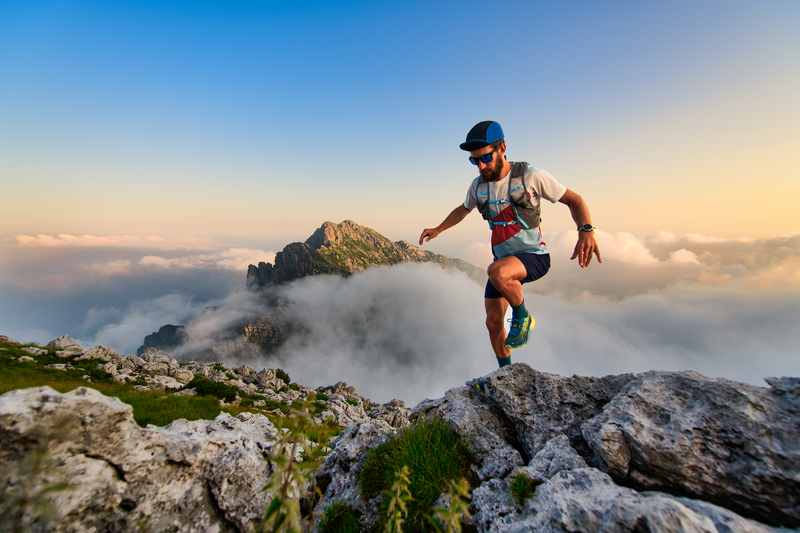
94% of researchers rate our articles as excellent or good
Learn more about the work of our research integrity team to safeguard the quality of each article we publish.
Find out more
HYPOTHESIS AND THEORY article
Front. Immunol. , 28 May 2020
Sec. Viral Immunology
Volume 11 - 2020 | https://doi.org/10.3389/fimmu.2020.01221
This article is part of the Research Topic Coronavirus Disease (COVID-19): Pathophysiology, Epidemiology, Clinical Management and Public Health Response View all 400 articles
Lactoferrin is a nutrient classically found in mammalian milk. It binds iron and is transferred via a variety of receptors into and between cells, serum, bile, and cerebrospinal fluid. It has important immunological properties, and is both antibacterial and antiviral. In particular, there is evidence that it can bind to at least some of the receptors used by coronaviruses and thereby block their entry. Of importance are Heparan Sulfate Proteoglycans (HSPGs) and the host receptor angiotensin-converting enzyme 2 (ACE2), as based on other activities lactoferrin might prevent severe acute respiratory syndrome coronavirus 2 (SARS-CoV-2) from attaching to the host cells. Lactoferrin (and more specifically enteric-coated LF because of increased bioavailability) may consequently be of preventive and therapeutic value during the present COVID-19 pandemic.
Lactoferrin (LF) or lactotransferrin has recently come under the spotlight, particularly with regards to the new coronavirus pandemic that started in 2019 (COVID-19). Diet and supplements support a well-functioning immune system, and favorably influence the body's ability to fight infection. Although LF is produced by the body itself, as a secretion by exocrine glands (such as maternal milk or tears) and secondary granules of human neutrophils (1), it can also be taken as a supplement, where it then acts as nutraceutical or functional food. Our particular focus is on its role as an oral supplement. Here we also collate some of the evidence that shows how LF may be an important nutrient to support host immunity, including as an antibacterial and antiviral agent, but particularly with the current COVID-19 pandemic in mind.
We summarize what is already known about LF, including its immunological properties, as well as its antibacterial and antiviral activities. We also discuss how LF uses Heparan Sulfate Proteoglycans (HSPGs) on cell surfaces to facilitate entry. This is of particular importance to coronaviruses, as these viruses are considered to bind to the host cell by attaching first to HSPGs using them as preliminary docking sites on the host cell surface. LF is known to interfere with some of the receptors used by coronaviruses, it may thus contribute usefully to the prevention and treatment of SARS CoV-2 infections. In COVID-19 infection, LF may therefore have a role to play, not only sequestering iron and inflammatory molecules that are severely increased during the cytokine burst, but also possibly in assisting by occupying receptors and HSPGs. LF might also prevent virus accumulation by the host cell, as well as rolling activity and entering of the virus via the host receptor angiotensin-converting enzyme 2 (ACE2). It has been 20 years since the discovery of ACE2, and since its discovery it has been found to be expressed in numerous tissues, including the lungs and the cardiovascular system (2). During 2020, there has been a renewed interest in this receptor, due to the interactions of novel coronaviruses and their interactions with ACE2 (3–5). South and co-workers in 2020 also investigated whether ACE2 blockade is a suitable option to attenuate COVID-19 (5). The use of recombinant human ACE2 (rhACE2) as ACE receptor competitor for binding has also been investigated (6, 7). There is also interest in the therapeutic targeting of HSPGs, and Hondermarck and co-workers suggested that is seems an easy way to inhibit SARS-Cov-2 infectivity (8). Here we also suggest that LF might be used as both a preventive and therapeutic supplement in the COVID-19 pandemic, by preventing interactions between the virus and both HSPGs and possibly ACE2. We summarize the layout of this paper in Figure 1.
Figure 1. Overview of this review of lactoferrin (LF). We discuss (1) discovery and structure of LF; (2) LF membrane receptors and some of the bacteria, their products and viruses that might also bind to these receptors, (3) including how acute respiratory syndrome coronavirus 2 (SARS-CoV-2) (causing COVID-19) may interact with host cells (see Figure 6 and Conclusion for a detailed discussion); (4) and how LF assists with host immunity. Diagram created with BioRender (www.biorender.com).
Human LF is a cationic glycosylated protein consisting of 691 amino acids (9) folded into two globular lobes (80 kDa bi-lobal glycoprotein) (10), that are connected by an α-helix (11, 12). Bovine LF contains 689 amino acids (13). LF was first discovered and isolated from bovine milk in 1939 (14), and is a member of the transferrin family (60% amino acid sequence identity with serum transferrin) (11). LF and transferrin have similar amino acid compositions, secondary structures (including their disulphide linkages), and tertiary structures, although they differ in terms of biological functions (11, 15, 16) (see Figure 2). There are also three different isoforms: LF-α is the iron-binding isoform, while LF- β and LF-g both have ribonuclease activity but do not bind iron (11, 17). When it is iron-rich it is referred to hololactoferrin and when iron-free apolactoferrin (18). The tertiary structures of the two forms are significantly different: apolactoferrin is characterized by an open conformation of the N-lobe and a closed conformation of the C-lobe, while both lobes are closed in the hololactoferrin (18). Human LF and bovine LF possess high sequence homology and have very similar antibacterial, antifungal, antiviral, antiparasitic, anti-inflammatory, and immunomodulatory activities (19–21). Consequently, it is common to give the bovine form rather than say a recombinant human form as a supplement. Bovine LF is also deemed a “generally recognized as safe” substance by the Food and Drug Administration (FDA, USA), and is commercially available in large quantities (19).
Figure 2. Crystal structures of bovine lactoferrin (PDB code = 1BLF), human lactoferrin (1B0L), and rabbit serum transferrin (1JNF). Adapted from Vogel (10). Pink spheres represent ferric iron (Fe3+) binding sites.
Due to its similarities to transferrin, which is the main iron transporting molecule in serum (22, 23), α-LF possesses iron binding capabilities (24, 25), and it can chelate two ferric irons (Fe3+) (26). LF binds one ferric iron atom in each of its two lobes; however, an important attribute is that it does not release its iron, even at pH 3.5. This is of importance as this property assures iron sequestration in infected tissues where the pH is commonly acidic (27). In the context of its iron-binding capabilities, it means that when it binds ferric and siderophore-bound iron, it limits the availability of essential iron to microbes (27).
In healthy individuals, iron is largely intracellular and sequestered within ferritin or as a co-factor of cytochromes and FeS proteins, and as haem complexed to hemoglobin within erythrocytes. Circulating iron is rapidly bound by transferrin (28, 29). When erythrocytes lyse and hemoglobin or haem is released into the circulation, their hemoglobin is captured by haptoglobin, and haem by hemopexin (30). Here, circulating serum ferroxidase ceruloplasmin is of importance, as LF can bind to ceruloplasmin, such that a direct transfer of ferric iron between the two proteins is possible (31). A direct transfer of ferric iron from ceruloplasmin to lactoferrin prevents both the formation of potentially toxic hydroxyl radicals (32) and the utilization of iron by pathogenic bacteria. LF is therefore an important player in preventing bacteria from acquiring and sequestering iron, which [with the possible exception of Borrelia burgdorferi (33)]; they require for growth and virulence. LF also acts as biomarker, as it is commonly upregulated when the host is suffering from various kinds of disease. See Table 1 for selected references.
Table 1. Lactoferrin as a major player in host defense and iron binding, and its use as biomarker for various diseases.
LF is thought to exert its main biological activities following interaction with receptors on target cells. There are in fact many LF receptors, though sometimes one is referred to as “the” lactoferrin receptor. They have been detected in multiple tissues and cell types including intestinal epithelial cells and lymphocytes (60, 61). Receptors that bind LF include CD14 (62), LDL receptor-related protein-1 (LRP-1/CD91) (63–65) intelectin-1 (omentin-1) (66), Toll-like receptor 2 and 4 (TLR4) (67) and cytokine receptor 4 (CXCR4) (68) (see Table 2). Importantly, LF also binds to heparan sulfate proteoglycans (HSPGs), which are cell-surface and extracellular matrix macromolecules that are composed of a core protein decorated with covalently linked glycosaminoglycan (GAG) chains (86, 87, 98, 99). See Table 2. Different receptors express at vastly different levels in different tissues; thus intelectin-1 is really expressed only in the intestine (https://www.proteinatlas.org/ENSG00000179914-ITLN1/tissue), while LRP1 is far more widely distributed https://www.proteinatlas.org/ENSG00000123384-LRP1/tissue. These multiple receptors arguably underpin the substantial and widespread effects that LF can induce, since only when multiple targets are hit simultaneously can one normally have major effects (103, 104).
Table 2. Receptors for lactoferrin, cells where these receptors are present, and other molecules and/or components that might bind to these receptors.
The entry of bacteria, bacterial products or viruses into host cells may also occur via some of these receptors. Such binding evokes signaling systems and pathways involving, amongst others, mitogen-activated protein kinase (MAPK) (105), NF-κB (106), activator protein 1 (AP-1) (107), and various interferon regulatory factors (IRFs) [for a comprehensive review see (108)]. During infection, activation of these signaling pathways results in a cellular response that shares multiple cytoplasmic components, leading ultimately to the activation of a complex biomolecular network. Phosphorylation of relevant substrates (e.g., enzymes, microtubules, histones, and transcription factors) plays a crucial role in determining the host's cellular response (109). Viruses (110, 111), as well as bacteria (112), interact with and bind to HSPGs, using this proteoglycan as entry into the cell (see also Figure 1). LF acts as an important element in host defense mechanisms by binding to these receptors, but also binding to HSPG on cells, since these are locations where binding to bacteria and their cell wall products as well as viruses occur. The membrane-penetrating peptide HIV-tat, released from HIV-infected cells, also enters surrounding cells using HSPGs (86, 98). This binding capacity allows LF to compete with such molecules for receptor occupancy (113, 114), and therefore plays a vital role in host immunity (20). LF can also serve to prevent nephrotoxicity, e.g., of cisplatin (115).
Small molecules, including pharmaceutical drugs, require solute carriers of the SLC family (116) to effect their uptake (117–124). Lactoferrin, as a protein, is far too large to exploit such a route, and instead passes from the stomach via epithelial cells and into the blood using endocytosis (125, 126), especially via Peyer's patches (127), and when it is encapsulated (“enterically formulated”) in liposomes (128–130). This uptake then occurs mostly via the lymphatic rather than the portal circulation (131, 132). LF can also enter, and be reabsorbed from, the bile (125). Blood LF can further be transported to the CNS via cerebrospinal fluid (133, 134) and via the Blood Brain Barrier (63, 133).
LF plays an important role in host defense, upon its release from the neutrophil (26). LF also enhances natural killer cell activity in immune defense (135) and can restrict the entry of the virus into host cells during infection. As part of the host's inflammatory response, leucocytes, including neutrophils, release LF from their granules, where it is normally stored. Activated neutrophils also release chromatin fibers, known as neutrophil extracellular traps (NETs), which trap and kill, amongst others, bacteria (1, 136). These NETs likewise modulate both acute and chronic inflammation (137, 138). NETs are also found in various autoimmune conditions such as rheumatoid arthritis, systemic lupus erythematosus (139, 140). Interestingly, 106 human neutrophils can release 15 μg of LF (26). In addition to DNA and histones, NET fibers contain extranuclear proteins and proteins such as elastase, myeloperoxidase (MPO), and LF (141). LF may also serve as an intrinsic inhibitor of NETs release into the circulation, and may therefore be central in controlling NETs release (1). See Figure 3.
Figure 3. Bacterial binding to various receptors, e.g., Toll-like receptors 2 and 4 (TLR2 and 4), as well as complement receptors, leads to protein arginine deiminase 4 (PAD4) activation, followed by chromatin decondensation, hypercitrullination of histones 3 and 4 in the nucleus, and nuclear membrane disruption. Granules also release lactoferrin. Neutrophil Extracellular Traps (NETs) and their protein constituents (including lactoferrin) are released from the neutrophil. Adapted from Jorch and Kubes (142) and Law and Gray (143). Bacteria are expelled and trapped in the NETs. Diagram created with BioRender (https://biorender.com/).
One of the most well-known characteristics of LF is that it is antibacterial (19, 144–148), antiviral (99, 149–151), antifungal (152–154), anti-inflammatory (26), and anti-carcinogenic (155). Its ability to of limit iron availability to microbes is one of its crucial amicrobial properties. Bacteria have, however, developed various ways to sequester iron (156). Figure 4 shows how bacteria acquire iron through receptor-mediated recognition of transferrin, hemopexin, hemoglobin, or hemoglobin-haptoglobin complexes and also LF (30). As well as binding it directly from the environment, bacterial siderophores can obtain iron by removing it from transferrin, lactoferrin, or ferritin (32). These siderophore-iron complexes are then recognized by receptors on the bacterium (30). Host innate immune functions are supported by the circulating protein, siderocalin, also known as Neutrophil gelatinase-associated lipocalin (NGAL), lipocalin2 or Lcn2 as it inhibits siderophore-mediated iron acquisition and release (30).
Figure 4. Ways by which bacteria acquire iron [adapted from (19, 30)]. Transferrin receptor, lactoferrin receptor, hemophore (Hp), hemophore receptor, and hemopexin. Siderophores remove iron from lactoferrin, ferritin and transferrin, and also from the environment. Stealth siderophores are modified in such a way as to prevent siderocalin binding. A primary bacterial defense against siderocalin involves the production of stealth siderophores. Modified from Rosa et al. and Skaar (19, 30). Diagram created with BioRender (https://biorender.com/).
Although LF has various means to counteract bacteria as part of its immune function (131), it is also capable of being hijacked to benefit the activities of bacteria. Thus, bacteria can also exploit LF by removing its bound ferric iron (19, 30). This process involves (1) synthesis of high-affinity ferric ion chelators by bacteria, (2) iron acquisition through LF or transferrin binding, mediated by bacterial-specific surface bacterial receptors, (3) or iron acquisition through bacterial reductases, which are able to reduce ferric to ferrous ions (19, 144–148).
Several Gram-negative pathogens including members of the genera Neisseria and Moraxella have evolved two-component systems that can extract iron from the host LF and transferrin (157). N. meningitidis is a principal cause of bacterial meningitis in children. While the majority of pathogenic bacteria employ siderophores to chelate and scavenge iron (158), Neisseria has evolved a series of protein transporters that directly hijack iron sequestered in host transferrin, lactoferrin, and hemoglobin (159). The system consists of a membrane-bound transporter that extracts and transports iron across the outer membrane (TbpA for transferrin and LbpA for lactoferrin), and a lipoprotein that delivers iron-loaded lactoferrin/transferrin to the transporter (TbpB for transferrin and LbpB for lactoferrin) (157). LbpB binds the N-lobe of lactoferrin, whereas TbpB binds the C-lobe of transferrin (157). However, more than 90% of LF in human milk is in the form of apolactoferrin (160), which competes with siderophilic bacteria for ferric iron, and disrupts the proliferation of these microbial and other pathogens. Similarly LF supplements may play an important role to counteract bacterial processes. LF is consequently a significant element of host defense (19), and its levels may vary in health and during disease. It is hence known to be a modulator of innate and adaptive immune responses (161).
LF has strong antiviral activity against a broad spectrum of both naked and enveloped DNA and RNA viruses (99, 149–151). LF inhibits the entry of viral particles into host cells, either by direct attachment to the viral particles or by blocking their cellular receptors (discussed in previous paragraphs) (149). Some of the viruses that LF prevents from entering host cells e.g., Herpes simplex virus (162), human papillomavirus (163), human immunodeficiency virus (HIV) (164), and rotavirus (165). These viruses typically utilize common molecules on the cell membrane to facilitate their invasion into cells, including HSPGs (Figure 1). HSPGs provide the first anchoring sites on the host cell surface, and help the virus make primary contact with these cells (99, 162). HSPGs can be either membrane bound, or in secretory vesicles and in the extracellular matrix (86). It has been shown that LF is able to prevent the internalization of some viruses by binding to HSPGs (86).
COVID-19 is caused by severe acute respiratory syndrome coronavirus 2 (SARS-CoV-2). Many COVID-19 patients develop acute respiratory distress syndrome (ARDS), which leads to pulmonary edema and lung failure, and have liver, heart, and kidney damages. These symptoms are associated with a cytokine storm (166, 167) manifesting elevated serum levels of interleukin (IL) IL-1β, IL-2, IL-7, IL-8, IL-9, IL-10, IL-17, granulocyte colony-stimulating factor (G-CSF), Granulocyte-Macrophage Colony Stimulating Factor (GM-CSF), interferon (IFN)γ, tumor necrosis factor (TNF)α, Interferon gamma-induced protein 10 (IP10), Monocyte Chemoattractant Protein-1 (MCP1), macrophage inflammatory protein 1(MIP1)A and MIP1B (168). IL-22, in collaboration with IL-17 and TNFα, induces antimicrobial peptides in the mucosal organs. IL-22 also upregulates mucins, fibrinogen, anti-apoptotic proteins, serum amyloid A, and LPS binding protein (169); therefore, IL-22 may contribute to the formation of life-threatening oedema with mucins and fibrin (170), seen in SARS-CoV-22 and SARS-CoV patients (168).
The 2003 SARS-CoV strain, that also causes severe acute respiratory syndrome, attaches to host cells via host receptor ACE2 (171). This type I integral membrane protein receptor is a well-known receptor for respiratory viruses, and is abundantly expressed in tissues lining the respiratory tract (111). During COVID-19 infection, SARS-CoV-2 also enters host cells via the ACE2 receptor (172). ACE2 is highly expressed on human lung alveolar epithelial cells, enterocytes of the small intestine, and the brush border of the proximal tubular cells of the kidney (99). HSPGs are also one of the preliminary docking sites on the host cell surface and play an important role in the process of SARS-CoV cell entry (99). There is no current confirmed information that SARS-CoV-2 binds to HSPGs, however, LF blocks the infection of SARS-CoV by binding to HSPGs (99). It is not presently known whether LF binds to ACE2, but it does bind to HSPGs (99). Whether SARS-CoV-2 also enters host cells via HPSGs in the same way, as does (the 2003) SARS-CoV clearly warrants further investigation.
Of particular interest, and in the context of this paper, is the set of interactions between SARS-CoV-2 and host platelets. This is of importance, as COVID-19 infection, can cause hyperinflammation due to a cytokine storm (166). Pathogens like the influenza virus and Francisella tularensis, do trigger life-threatening cytokine storms (173). Such a cytokine storm will significantly affect platelets, as platelets have many receptors where these inflammatory molecules may bind (173) (see Figure 5). Circulating cytokines and inflammagens will hyperactivate platelets, causing low platelet count (thrombocytopenia), and a significant chance of hypercoagulation. Thrombocytopenia is associated with increased risk of severe disease and mortality in patients with COVID-19, and thus serves as clinical indicator of worsening illness during hospitalization (174, 175). Patients with type 2 diabetes are also particularly prone to increased levels of circulating inflammatory cytokines and hypercoagulation (76). COVID-19 patients without other comorbidities but with diabetes are at higher risk of severe pneumonia, excessive uncontrolled inflammatory responses and a hypercoagulable state (176). Guo and co-workers in 2020 also found that serum levels of IL-6, C-reactive protein, serum ferritin, and D-dimer, were significantly higher in diabetic patients compared with those without, suggesting that patients with diabetes are more susceptible to an inflammatory storm eventually leading to rapid deterioration of the patient with COVID-19 (140). Acute pulmonary embolism has also been reported in COVID-19 infection (177). Focal accumulation of activated platelets within the oedematous area ex vivo correlated well with the size of the pulmonary embolism (178). Interestingly, anticoagulant therapy, mainly with (intravenous) heparin (and mainly with low molecular weight heparin, LMWH), appears to be associated with better prognosis in severe COVID-19 patients (179).
Figure 5. Simplified platelet signaling and receptor activation during disease with main dysregulated molecules thrombin, fibrin(ogen), von Willebrand Factor (vWF) interleukins (IL) like IL-1α, IL-1β, and IL17A and cytokines like TNF-α. Diagram created with BioRender (https://biorender.com/).
In COVID-19 infection, LF may have a role to play in not only sequestering iron and inflammatory molecules that are severely increased during the cytokine burst, but also possibly in assisting in occupying receptors and HSPGs to prevent virus binding. Receptor occupancy is an important characteristic of LF, when taken as supplement. Furthermore, it may assist in preventing thrombocytopenia, and hypercoagulation, both prominent features of COVID-19 infection.
There is little doubt that oral LF can be of health benefit to the host, and while it is not considered to be absolutely necessary for mammalian life (so it is not a vitamin), it is reasonable to class it as a nutraceutical along with a variety of other molecules such as those mentioned in various papers (180, 181). As a nutraceutical, the bioavailability of LF would clearly be an important consideration in its use for the prevention or treatment of COVID-19. Enteric coating of LF capsules has been proposed as a measure to maximize the uptake of LF by the receptors located in the brush-border of the small intestine (182). Enteric coating allows LF release some distance from LF-degrading pepsin activities in the stomach, allowing it to remain intact, in the form capable of binding small intestinal LF receptors for uptake and eventual transfer into the systemic circulation (182). In a rodent study, the “absorption” of enteric-formulated LF was approximately 10-fold higher than that of regular LF introduced into the stomach of experimental animals (128). In view of these investigations, the authors of this paper regard enteric-coated LF as superior to regular LF supplements with respect to bioavailability and potential application for the prevention or therapy for coronaviruses such as the SARS-Cov-2 involved in COVID-19.
There is considerable LF availability in various forms and sources. Table 3 shows some of the sources and the references to research where it has been used to treat various conditions.
Table 3. Lactoferrin sources as supplements, and examples where it has been used to treat various conditions.
Lactoferrin clearly has immunological benefits, as well as having an important antibacterial and antiviral role. Because it is known to interfere with some of the receptors used by coronaviruses, it may contribute usefully to the prevention and treatment of coronavirus infections. Figure 6 shows a possible scheme on how LF might interfere with SARS-CoV-2 binding. The binding of LF to HSPGs prevents the first contact between virus and host cells and thus prevents subsequent infection (99). HSPGs themselves are not sufficient for SARS-CoV entry. However, in SARS-CoV infections, the HSPGs play an important role in the process of cell entry (99). The anchoring sites provided by HSPGs permit initial contact between the virus and host cells and the concentration of virus particles on cell surface. SARS-CoV bound to HSPGs then rolls onto the cell membrane and scans for specific entry receptors, which leads to subsequent cell entry (99). LF enhances natural killer cell activity and stimulates neutrophil aggregation and adhesion in immune defense (135) and can restrict the entry of the virus into host cells during infection. We suggest that this process might be the same for COVID-19 (see Figure 6 for a visual representation), thereby offering useful strategies for prevention and treatment. Currently, there is also a renewed interest in ACE2 and HSPG blocking, as discussed in the introduction (5–8). LF may therefore be an excellent supplement to take, not only as a contribution to prevention but perhaps as a therapy in the event COVID-19 is diagnosed.
Figure 6. Possible action of (1) lactoferrin by occupying binding sites of (2) SARS-CoV-2 that causes COVID-19. (3) Entry into host cells occur when SARS-CoV-2 first attaches to Heparan sulfate proteoglycans (HSPGs). This attachment initiates the first contact between the cell and the virus, concentrating the virus on the cell surface, (4) followed attaching of the virus to the host receptor (ACE2) and association and entering are then facilitated via clathrin-coated pits (5) Virus replication can then happen inside the cell. (6) One of the characteristics of Lactoferrin, is that it attaches to HSPGs. (7) Currently we do not know if ACE2 is also a receptor for lactoferrin. (8) Lactoferrin may block the entry of SARS-CoV-2 into the host cell, by occupying HPSGs, thereby preventing SARS-CoV-2 initial attachment and accumulation on the host cell membrane. COVID-19 infection template adjusted from www.biorender.com.
The original contributions presented in the study are included in the article/supplementary material, further inquiries can be directed to the corresponding author/s.
Another recent review (196) has also highlighted the potential utility of lactoferrin as an antiviral.
EP wrote the paper. DK edited and wrote part of the paper. EH provided clinical input and edited the paper. All authors approved submission of the paper.
We thank the Medical Research Council of South Africa (MRC) (Self-Initiated Research Program: A0X331) and the UK BBSRC (grant BB/L025752/1) for supporting this collaboration.
The authors declare that the research was conducted in the absence of any commercial or financial relationships that could be construed as a potential conflict of interest.
LF, Lactoferrin; lactotransferrin; SARS-CoV, acute respiratory syndrome coronavirus; LRP-1/CD91, LDL receptor-related protein-1; TLR2 and 4, Toll-like receptor 2 and 4; CXCR4, cytokine receptor 4; GAG, glycosaminoglycan; AP-1, activator protein 1; NF-κB, NF-kappa beta; IRF, Interferon regulatory factor; MAPK, Mitogen-activated protein kinase; HSPG, Heparan sulfate proteoglycans; ACE2, Angiotensin-converting enzyme 2; IL, Interleukin; G-CSF, Granulocyte colony-stimulating factor; GM-CSF, Granulocyte-Macrophage Colony Stimulating Factor; IFN, Interferon; TNFα, Tumor necrosis factor alpha; IP10, Interferon gamma-induced protein 10; MCP1, Monocyte Chemoattractant Protein-1; (MIP1) A and B, Macrophage inflammatory protein 1 (A and B); LMWH, Low molecular weight heparin; vWF, von Willebrand Factor; PAD4, protein arginine deiminase 4; NETS, Neutrophil extracellular traps.
1. Okubo K, Kamiya M, Urano Y, Nishi H, Herter JM, Mayadas T, et al. Lactoferrin suppresses neutrophil extracellular traps release in inflammation. EBioMedicine. (2016) 10:204–15. doi: 10.1016/j.ebiom.2016.07.012
2. Gheblawi M, Wang K, Viveiros A, Nguyen Q, Zhong JC, Turner AJ, et al. Angiotensin-converting enzyme 2: SARS-CoV-2 receptor and regulator of the renin-angiotensin system: celebrating the 20th anniversary of the discovery of ACE2. Circ Res. (2020) 126:1456–74. doi: 10.1161/CIRCRESAHA.120.317015
3. Kai H, Kai M. Interactions of coronaviruses with ACE2, angiotensin II, and RAS inhibitors-lessons from available evidence and insights into COVID-19. Hypertens Res. (2020) 1–7. doi: 10.1038/s41440-020-0455-8. [Epub ahead of print].
4. Yang J, Li H, Hu S, Zhou Y. ACE2 correlated with immune infiltration serves as a prognostic biomarker in endometrial carcinoma and renal papillary cell carcinoma: implication for COVID-19. Aging. (2020) 12:6518–35. doi: 10.18632/aging.103100
5. South AM, Diz DI, Chappell MC. COVID-19, ACE2, and the cardiovascular consequences. Am J Physiol Heart Circ Physiol. (2020) 318:H1084–90. doi: 10.1152/ajpheart.00217.2020
6. Zhang J, Xie B, Hashimoto K. Current status of potential therapeutic candidates for the COVID-19 crisis. Brain Behav Immun. (2020). doi: 10.1016/j.bbi.2020.04.046. [Epub ahead of print].
7. Monteil V, Kwon H, Prado P, Hagelkrüys A, Wimmer RA, Stahl M, et al. Inhibition of SARS-CoV-2 infections in engineered human tissues using clinical-grade soluble human ACE2. Cell. (2020). doi: 10.1016/j.cell.2020.04.004. [Epub ahead of print].
8. Hondermarck H, Bartlett NW, Nurcombe V. The role of growth factor receptors in viral infections: an opportunity for drug repurposing against emerging viral diseases such as COVID-19? FASEB Bioadv. (2020) 2:296–303. doi: 10.1096/fba.2020-00015
9. Anderson BF, Baker HM, Norris GE, Rumball SV, Baker EN. Apolactoferrin structure demonstrates ligand-induced conformational change in transferrins. Nature. (1990) 344:784–7. doi: 10.1038/344784a0
10. Vogel HJ. Lactoferrin, a bird's eye view. Biochem Cell Biol. (2012) 90:233–44. doi: 10.1139/o2012-016
11. Karav S, German JB, Rouquié C, Le Parc A, Barile D. Studying lactoferrin N-glycosylation. Int J Mol Sci. (2017) 18:E870. doi: 10.3390/ijms18040870
12. Karav S. Selective deglycosylation of lactoferrin to understand glycans' contribution to antimicrobial activity of lactoferrin. Cell Mol Biol. (2018) 64:52–7. doi: 10.14715/cmb/2018.64.9.8
13. Moore SA, Anderson BF, Groom CR, Haridas M, Baker EN. Three-dimensional structure of diferric bovine lactoferrin at 2.8 A resolution. J Mol Biol. (1997) 274:222–36. doi: 10.1006/jmbi.1997.1386
14. Sorensen M, Sorensen S. Compte Rendu des Travaux du Laboratoire de Carlsberg. Copenhague: The Proteins in Whey, Hagerup in Komm (1939).
15. Querinjean P, Masson PL, Heremans JF. Molecular weight, single-chain structure and amino acid composition of human lactoferrin. Eur J Biochem. (1971) 20:420–5. doi: 10.1111/j.1432-1033.1971.tb01408.x
16. Bluard-Deconinck JM, Masson PL, Osinski PA, Heremans JF. Amino acid sequence of cysteic peptides of lactoferrin and demonstration of similarities between lactoferrin and transferrin. Biochim Biophys Acta. (1974) 365:311–7. doi: 10.1016/0005-2795(74)90002-6
17. Furmanski P, Li ZP, Fortuna MB, Swamy CV, Das MR. Multiple molecular forms of human lactoferrin. Identification of a class of lactoferrins that possess ribonuclease activity and lack iron-binding capacity. J Exp Med. (1989) 170:415–29. doi: 10.1084/jem.170.2.415
18. Jameson GB, Anderson BF, Norris GE, Thomas DH, Baker EN. Structure of human apolactoferrin at 2.0 A resolution. Refinement and analysis of ligand-induced conformational change. Acta Crystallogr D Biol Crystallogr. (1998) 54:1319–35. doi: 10.1107/S0907444998004417
19. Rosa L, Cutone A, Lepanto MS, Paesano R, Valenti P. Lactoferrin: a natural glycoprotein involved in iron and inflammatory homeostasis. Int J Mol Sci. (2017) 18:1985. doi: 10.3390/ijms18091985
20. Teraguchi S, Wakabayashi H, Kuwata H, Yamauchi K, Tamura Y. Protection against infections by oral lactoferrin: evaluation in animal models. Biometals. (2004) 17:231–4. doi: 10.1023/B:BIOM.0000027697.83706.32
21. Togawa J, Nagase H, Tanaka K, Inamori M, Umezawa T, Nakajima A, et al. Lactoferrin reduces colitis in rats via modulation of the immune system and correction of cytokine imbalance. Am J Physiol Gastrointest Liver Physiol. (2002) 283:G187–95. doi: 10.1152/ajpgi.00331.2001
22. Ashall L, Horton CA, Nelson DE, Paszek P, Harper CV, Sillitoe K, et al. Pulsatile stimulation determines timing and specificity of NF-kappaB-dependent transcription. Science. (2009) 324:242–6. doi: 10.1126/science.1164860
23. Anderberg RJ, Meek RL, Hudkins KL, Cooney SK, Alpers CE, Leboeuf RC, et al. Serum amyloid A and inflammation in diabetic kidney disease and podocytes. Lab Invest. (2015) 95:250–62. doi: 10.1038/labinvest.2014.163
26. Lepanto MS, Rosa L, Paesano R, Valenti P, Cutone A. Lactoferrin in aseptic and septic inflammation. Molecules. (2019) 24:1323. doi: 10.3390/molecules24071323
28. Kell DB. Iron behaving badly: inappropriate iron chelation as a major contributor to the aetiology of vascular and other progressive inflammatory and degenerative diseases. BMC Med Genom. (2009) 2:2. doi: 10.1186/1755-8794-2-2
29. Kell DB, Pretorius E. Serum ferritin is an important inflammatory disease marker, as it is mainly a leakage product from damaged cells. Metallomics. (2014) 6:748–73. doi: 10.1039/C3MT00347G
30. Skaar EP. The battle for iron between bacterial pathogens and their vertebrate hosts. PLoS Pathog. (2010) 6:e1000949. doi: 10.1371/journal.ppat.1000949
31. White KN, Conesa C, Sánchez L, Amini M, Farnaud S, Lorvoralak C, et al. The transfer of iron between ceruloplasmin and transferrins. Biochim Biophys Acta. (2012) 1820:411–6. doi: 10.1016/j.bbagen.2011.10.006
32. Kell DB, Pretorius E. No effects without causes. The iron dysregulation and dormant microbes hypothesis for chronic, inflammatory diseases: evidence and consequences. Biol Rev. (2018) 93:1518–57. doi: 10.1111/brv.12407
33. Posey JE, Gherardini FC. Lack of a role for iron in the Lyme disease pathogen. Science. (2000) 288:1651–3. doi: 10.1126/science.288.5471.1651
34. Telang S. Lactoferrin: a critical player in neonatal host defense. Nutrients. (2018) 10:1228. doi: 10.3390/nu10091228
35. Chow BD, Reardon JL, Perry EO, Laforce-Nesbitt SS, Tucker R, Bliss JM. Host defense proteins in breast milk and neonatal yeast colonization. J Hum Lact. (2016) 32:168–73. doi: 10.1177/0890334415592402
36. Hettinga K, van Valenberg H, de Vries S, Boeren S, van Hooijdonk T, van Arendonk J, et al. The host defense proteome of human and bovine milk. PLoS ONE. (2011) 6:e19433. doi: 10.1371/journal.pone.0019433
37. Ballard O, Morrow AL. Human milk composition: nutrients and bioactive factors. Pediatr Clin North Am. (2013) 60:49–74. doi: 10.1016/j.pcl.2012.10.002
38. Woodman T, Strunk T, Patole S, Hartmann B, Simmer K, Currie A. Effects of lactoferrin on neonatal pathogens and Bifidobacterium breve in human breast milk. PLoS ONE. (2018) 13:e0201819. doi: 10.1371/journal.pone.0201819
39. Czosnykowska-Łukacka M, Orczyk-Pawiłowicz M, Broers B, Królak-Olejnik B. Lactoferrin in human milk of prolonged lactation. Nutrients. (2019) 11:E2350. doi: 10.3390/nu11102350
40. Lönnerdal B. Bioactive proteins in human milk: health, nutrition, and implications for infant formulas. J Pediatr. (2016) 173(Suppl):S4–9. doi: 10.1016/j.jpeds.2016.02.070
41. Cai X, Duan Y, Li Y, Wang J, Mao Y, Yang Z, et al. Lactoferrin level in breast milk: a study of 248 samples from eight regions in China. Food Funct. (2018) 9:4216–22. doi: 10.1039/C7FO01559C
42. Valenti P, Rosa L, Capobianco D, Lepanto MS, Schiavi E, Cutone A, et al. Role of lactobacilli and lactoferrin in the mucosal cervicovaginal defense. Front Immunol. (2018) 9:376. doi: 10.3389/fimmu.2018.00376
43. Cole AM. Innate host defense of human vaginal and cervical mucosae. Curr Top Microbiol Immunol. (2006) 306:199–230. doi: 10.1007/3-540-29916-5_8
44. Bard E, Laibe S, Bettinger D, Riethmuller D, Biichlé S, Seilles E, et al. New sensitive method for the measurement of lysozyme and lactoferrin for the assessment of innate mucosal immunity. Part I: time-resolved immunofluorometric assay in serum and mucosal secretions. Clin Chem Lab Med. (2003) 41:127–33. doi: 10.1515/CCLM.2003.021
45. Boesch AW, Zhao Y, Landman AS, Garcia MR, Fahey JV, Wira CR, et al. A multiplexed assay to detect antimicrobial peptides in biological fluids and cell secretions. J Immunol Methods. (2013) 397:71–6. doi: 10.1016/j.jim.2013.09.001
46. Laube DM, Yim S, Ryan LK, Kisich KO, Diamond G. Antimicrobial peptides in the airway. Curr Top Microbiol Immunol. (2006) 306:153–82. doi: 10.1007/3-540-29916-5_6
47. Vargas Buonfiglio LG, Borcherding JA, Frommelt M, Parker GJ, Duchman B, Vanegas Calderón OG, et al. Airway surface liquid from smokers promotes bacterial growth and biofilm formation via iron-lactoferrin imbalance. Respir Res. (2018) 19:42. doi: 10.1186/s12931-018-0743-x
48. Ward PP, Uribe-Luna S, Conneely OM. Lactoferrin and host defense. Biochem Cell Biol. (2002) 80:95–102. doi: 10.1139/o01-214
49. Delgado-Rizo V, Martínez-Guzmán MA, Iñiguez-Gutierrez L, García-Orozco A, Alvarado-Navarro A, Fafutis-Morris M. Neutrophil extracellular traps and its implications in inflammation: an overview. Front Immunol. (2017) 8:81. doi: 10.3389/fimmu.2017.00081
50. Lynge Pedersen AM, Belstrøm D. The role of natural salivary defences in maintaining a healthy oral microbiota. J Dent. (2019) 80(Suppl. 1):S3–12. doi: 10.1016/j.jdent.2018.08.010
51. van Leeuwen SJM, Potting CMJ, Huysmans M, Blijlevens NMA. Salivary changes before and after hematopoietic stem cell transplantation: a systematic review. Biol Blood Marrow Transplant. (2019) 25:1055–61. doi: 10.1016/j.bbmt.2019.01.026
52. Wang A, Duncan SE, Lesser GJ, Ray WK, Dietrich AM. Effect of lactoferrin on taste and smell abnormalities induced by chemotherapy: a proteome analysis. Food Funct. (2018) 9:4948–58. doi: 10.1039/C8FO00813B
53. Farah R, Haraty H, Salame Z, Fares Y, Ojcius DM, Said Sadier N. Salivary biomarkers for the diagnosis and monitoring of neurological diseases. Biomed J. (2018) 41:63–87. doi: 10.1016/j.bj.2018.03.004
54. Gleerup HS, Hasselbalch SG, Simonsen AH. Biomarkers for Alzheimer's disease in saliva: a systematic review. Dis Markers. (2019) 2019:4761054. doi: 10.1155/2019/4761054
55. Carro E, Bartolomé F, Bermejo-Pareja F, Villarejo-Galende A, Molina JA, Ortiz P, et al. Early diagnosis of mild cognitive impairment and Alzheimer's disease based on salivary lactoferrin. Alzheimers Dement. (2017) 8:131–8. doi: 10.1016/j.dadm.2017.04.002
56. Koshi R, Kotani K, Ohtsu M, Yoshinuma N, Sugano N. Application of lactoferrin and α1-antitrypsin in gingival retention fluid to diagnosis of periodontal disease. Dis Mark. (2018) 2018:4308291. doi: 10.1155/2018/4308291
57. Mizuhashi F, Koide K, Toya S, Takahashi M, Mizuhashi R, Shimomura H. Levels of the antimicrobial proteins lactoferrin and chromogranin in the saliva of individuals with oral dryness. J Prosthet Dent. (2015) 113:35–8. doi: 10.1016/j.prosdent.2013.12.028
58. Glimvall P, Wickström C, Jansson H. Elevated levels of salivary lactoferrin, a marker for chronic periodontitis? J Periodontal Res. (2012) 47:655–60. doi: 10.1111/j.1600-0765.2012.01479.x
59. Jalil RA, Ashley FP, Wilson RF, Wagaiyu EG. Concentrations of thiocyanate, hypothiocyanite, 'free' and 'total' lysozyme, lactoferrin and secretory IgA in resting and stimulated whole saliva of children aged 12–14 years and the relationship with plaque accumulation and gingivitis. J Periodontal Res. (1993) 28:130–6. doi: 10.1111/j.1600-0765.1993.tb01060.x
60. Jiang R, Lopez V, Kelleher SL, Lönnerdal B. Apo- and holo-lactoferrin are both internalized by lactoferrin receptor via clathrin-mediated endocytosis but differentially affect ERK-signaling and cell proliferation in Caco-2 cells. J Cell Physiol. (2011) 226:3022–31. doi: 10.1002/jcp.22650
61. Suzuki YA, Lopez V, Lönnerdal B. Mammalian lactoferrin receptors: structure and function. Cell Mol Life Sci. (2005) 62:2560–75. doi: 10.1007/s00018-005-5371-1
62. Rawat P, Kumar S, Sheokand N, Raje CI, Raje M. The multifunctional glycolytic protein glyceraldehyde-3-phosphate dehydrogenase (GAPDH) is a novel macrophage lactoferrin receptor. Biochem Cell Biol. (2012) 90:329–38. doi: 10.1139/o11-058
63. Fillebeen C, Descamps L, Dehouck MP, Fenart L, Benaïssa M, Spik G, et al. Receptor-mediated transcytosis of lactoferrin through the blood-brain barrier. J Biol Chem. (1999) 274:7011–7. doi: 10.1074/jbc.274.11.7011
64. Grey A, Banovic T, Zhu Q, Watson M, Callon K, Palmano K, et al. The low-density lipoprotein receptor-related protein 1 is a mitogenic receptor for lactoferrin in osteoblastic cells. Mol Endocrinol. (2004) 18:2268–78. doi: 10.1210/me.2003-0456
65. Ikoma-Seki K, Nakamura K, Morishita S, Ono T, Sugiyama K, Nishino H, et al. Role of LRP1 and ERK and cAMP signaling pathways in lactoferrin-induced lipolysis in mature rat adipocytes. PLoS ONE. (2015) 10:e0141378. doi: 10.1371/journal.pone.0141378
66. Shin K, Wakabayashi H, Yamauchi K, Yaeshima T, Iwatsuki K. Recombinant human intelectin binds bovine lactoferrin and its peptides. Biol Pharm Bull. (2008) 31:1605–8. doi: 10.1248/bpb.31.1605
67. Gao CH, Dong HL, Tai L, Gao XM. Lactoferrin-containing immunocomplexes drive the conversion of human macrophages from M2- into M1-like phenotype. Front Immunol. (2018) 9:37. doi: 10.3389/fimmu.2018.00037
68. Takayama Y, Aoki R, Uchida R, Tajima A, Aoki-Yoshida A. Role of CXC chemokine receptor type 4 as a lactoferrin receptor. Biochem Cell Biol. (2017) 95:57–63. doi: 10.1139/bcb-2016-0039
69. Patel P, Shah J. Role of vitamin D in Amyloid clearance via LRP-1 upregulation in Alzheimer's disease: a potential therapeutic target? J Chem Neuroanat. (2017) 85:36–42. doi: 10.1016/j.jchemneu.2017.06.007
70. Tamaki C, Ohtsuki S, Terasaki T. Insulin facilitates the hepatic clearance of plasma amyloid beta-peptide (1 40) by intracellular translocation of low-density lipoprotein receptor-related protein 1 (LRP-1) to the plasma membrane in hepatocytes. Mol Pharmacol. (2007) 72:850–5. doi: 10.1124/mol.107.036913
71. Yan FL, Zheng Y, Zhao FD. Effects of ginkgo biloba extract EGb761 on expression of RAGE and LRP-1 in cerebral microvascular endothelial cells under chronic hypoxia and hypoglycemia. Acta Neuropathol. (2008) 116:529–35. doi: 10.1007/s00401-008-0435-6
72. Watanabe T, Watanabe-Kominato K, Takahashi Y, Kojima M, Watanabe R. Adipose tissue-derived omentin-1 function and regulation. Compr Physiol. (2017) 7:765–81. doi: 10.1002/cphy.c160043
73. Tang AT, Choi JP, Kotzin JJ, Yang Y, Hong CC, Hobson N, et al. Endothelial TLR4 and the microbiome drive cerebral cavernous malformations. Nature. (2017) 545:305–10. doi: 10.1038/nature22075
74. Vogel S, Thein SL. Platelets at the crossroads of thrombosis, inflammation and haemolysis. Br J Haematol. (2018) 180:761–7. doi: 10.1111/bjh.15117
75. Olumuyiwa-Akeredolu OO, Page MJ, Soma P, Pretorius E. Platelets: emerging facilitators of cellular crosstalk in rheumatoid arthritis. Nat Rev Rheumatol. (2019) 15:237–48. doi: 10.1038/s41584-019-0187-9
76. Pretorius E. Platelets as potent signaling entities in type 2 diabetes mellitus. Trends Endocrinol Metab. (2019) 30:532–45. doi: 10.1016/j.tem.2019.05.003
77. García-Culebras A, Durán-Laforet V, Peña-Martínez C, Moraga A, Ballesteros I, Cuartero MI, et al. Role of TLR4 (Toll-Like receptor 4) in N1/N2 neutrophil programming after stroke. Stroke. (2019) 50:2922–32. doi: 10.1161/STROKEAHA.119.025085
78. Page MJ, Pretorius E. A champion of host defense: a generic large-scale cause for platelet dysfunction and depletion in infection. Semin Thromb Hemost. (2020) 46:302–19. doi: 10.1055/s-0040-1708827
79. Assinger A, Laky M, Badrnya S, Esfandeyari A, Volf I. Periodontopathogens induce expression of CD40L on human platelets via TLR2 and TLR4. Thromb Res. (2012) 130:e73–8. doi: 10.1016/j.thromres.2012.04.017
80. He Y, Lawlor NT, Newburg DS. Human milk components modulate Toll-like receptor-mediated inflammation. Adv Nutr. (2016) 7:102–11. doi: 10.3945/an.115.010090
81. Zhang G, Han J, Welch EJ, Ye RD, Voyno-Yasenetskaya TA, Malik AB, et al. Lipopolysaccharide stimulates platelet secretion and potentiates platelet aggregation via TLR4/MyD88 and the cGMP-dependent protein kinase pathway. J Immunol. (2009) 182:7997–8004. doi: 10.4049/jimmunol.0802884
82. De Filippo K, Rankin SM. CXCR4, the master regulator of neutrophil trafficking in homeostasis and disease. Eur J Clin Invest. (2018) 48(Suppl. 2):e12949. doi: 10.1111/eci.12949
83. Seo YD, Jiang X, Sullivan KM, Jalikis FG, Smythe KS, Abbasi A, et al. Mobilization of CD8(+) T cells via CXCR4 blockade facilitates PD-1 checkpoint therapy in human pancreatic cancer. Clin Cancer Res. (2019) 25:3934–45. doi: 10.1158/1078-0432.CCR-19-0081
84. Sanui T, Takeshita M, Fukuda T, Haraguchi A, Aida Y, Nishimura F. Anti-CD14 antibody-treated neutrophils respond to lps: possible involvement of CD14 upregulated by anti-CD14 antibody binding. Immunol Invest. (2017) 46:190–200. doi: 10.1080/08820139.2016.1238925
85. Palipane M, Snyder JD, LeMessurier KS, Schofield AK, Woolard SN, Samarasinghe AE. Macrophage CD14 impacts immune defenses against influenza virus in allergic hosts. Microb Pathog. (2019) 127:212–9. doi: 10.1016/j.micpath.2018.12.008
86. Sarrazin S, Lamanna WC, Esko JD. Heparan sulfate proteoglycans. Cold Spring Harbor Perspect Biol. (2011) 3:a004952. doi: 10.1101/cshperspect.a004952
87. Milewska A, Zarebski M, Nowak P, Stozek K, Potempa J, Pyrc K. Human coronavirus NL63 utilizes heparan sulfate proteoglycans for attachment to target cells. J Virol. (2014) 88:13221–30. doi: 10.1128/JVI.02078-14
88. Seong K-J, Lee H-G, Kook MS, Ko H-M, Jung J-Y, Kim W-J. Epigallocatechin-3-gallate rescues LPS-impaired adult hippocampal neurogenesis through suppressing the TLR4-NF-κB signaling pathway in mice. Korean J Physiol Pharmacol. (2016) 20:41–51. doi: 10.4196/kjpp.2016.20.1.41
89. Liu Q, Zerbinatti CV, Zhang J, Hoe HS, Wang B, Cole SL, et al. Amyloid precursor protein regulates brain apolipoprotein E and cholesterol metabolism through lipoprotein receptor LRP1. Neuron. (2007) 56:66–78. doi: 10.1016/j.neuron.2007.08.008
90. Kanekiyo T, Cirrito JR, Liu CC, Shinohara M, Li J, Schuler DR, et al. Neuronal clearance of amyloid-beta by endocytic receptor LRP1. J Neurosci. (2013) 33:19276–83. doi: 10.1523/JNEUROSCI.3487-13.2013
91. McMahon CM, Isabella CR, Windsor IW, Kosma P, Raines RT, Kiessling LL. Stereoelectronic effects impact glycan recognition. J Am Chem Soc. (2020) 142:2386–95. doi: 10.1021/jacs.9b11699
92. Kell DB, Pretorius E. On the translocation of bacteria and their lipopolysaccharides between blood and peripheral locations in chronic, inflammatory diseases: the central roles of LPS and LPS-induced cell death. Integr Biol. (2015) 7:1339–77. doi: 10.1039/c5ib00158g
93. Singer-Englar T, Barlow G, Mathur R. Obesity, diabetes, and the gut microbiome: an updated review. Expert Rev Gastroenterol Hepatol. (2019) 13:3–15. doi: 10.1080/17474124.2019.1543023
94. Lv X, Wang H, Su A, Xu S, Chu Y. Herpes simplex virus type 2 infection triggers AP-1 transcription activity through TLR4 signaling in genital epithelial cells. Virol J. (2018) 15:173. doi: 10.1186/s12985-018-1087-3
95. Chen B, Molecular mechanism of HIV-1 entry. Trends Microbiol. (2019) 27:878–91. doi: 10.1016/j.tim.2019.06.002
96. Mehrbod P, Ande SR, Alizadeh J, Rahimizadeh S, Shariati A, Malek H, et al. The roles of apoptosis, autophagy and unfolded protein response in arbovirus, influenza virus, HIV infections. Virulence. (2019) 10:376–413. doi: 10.1080/21505594.2019.1605803
97. Lee ACY, Zhang AJX, Chu H, Li C, Zhu H, Mak WWN, et al. H7N9 influenza A virus activation of necroptosis in human monocytes links innate and adaptive immune responses. Cell Death Dis. (2019) 10:442. doi: 10.1038/s41419-019-1684-0
98. Frankel AD, Pabo CO. Cellular uptake of the tat protein from human immunodeficiency virus. Cell. (1988) 55:1189–93. doi: 10.1016/0092-8674(88)90263-2
99. Lang J, Yang N, Deng J, Liu K, Yang P, Zhang G, et al. Inhibition of SARS pseudovirus cell entry by lactoferrin binding to heparan sulfate proteoglycans. PLoS ONE. (2011) 6:e23710. doi: 10.1371/journal.pone.0023710
100. Naskalska A, Dabrowska A, Szczepanski A, Milewska A, Jasik KP, Pyrc K. Membrane protein of human coronavirus NL63 is responsible for interaction with the adhesion receptor. J Virol. (2019) 93:e00355-19. doi: 10.1128/JVI.00355-19
101. Cagno V, Tseligka ED, Jones ST, Tapparel C. Heparan sulfate proteoglycans and viral attachment: true receptors or adaptation bias? Viruses. (2019) 11:E596. doi: 10.3390/v11070596
102. Szczepanski A, Owczarek K, Bzowska M, Gula K, Drebot I, Ochman M, et al. Canine respiratory coronavirus, bovine coronavirus, and human coronavirus OC43: receptors and attachment factors. Viruses. (2019) 11:E328. doi: 10.3390/v11040328
103. Cornish-Bowden A, Hofmeyr J-HS, Cárdenas ML. Strategies for manipulating metabolic fluxes in biotechnology. Bioorg Chem. (1995) 23:439–49. doi: 10.1006/bioo.1995.1030
104. Kell DB, Knowles JD. The role of modeling in systems biology. In: Szallasi Z, Stelling J, Periwal V, editors. System Modeling in Cellular Biology: From Concepts to Nuts and Bolts. Cambridge: MIT Press (2006). p. 3–18.
105. Liu C, Tang X, Zhang W, Li G, Chen Y, Guo A, et al. 6-Bromoindirubin-3'-oxime suppresses LPS-induced inflammation via inhibition of the TLR4/NF-κB and TLR4/MAPK signaling pathways. Inflammation. (2019) 42:2192–204. doi: 10.1007/s10753-019-01083-1
106. Zhou P, She Y, Dong N, Li P, He H, Borio A, et al. Alpha-kinase 1 is a cytosolic innate immune receptor for bacterial ADP-heptose. Nature. (2018) 561:122–6. doi: 10.1038/s41586-018-0433-3
107. Srivastava M, Saqib U, Banerjee S, Wary K, Kizil B, Muthu K, et al. Inhibition of the TIRAP-c-Jun interaction as a therapeutic strategy for AP1-mediated inflammatory responses. Int Immunopharmacol. (2019) 71:188–97. doi: 10.1016/j.intimp.2019.03.031
108. Futosi K, Fodor S, Mócsai A. Neutrophil cell surface receptors and their intracellular signal transduction pathways. Int Immunopharmacol. (2013) 17:638–50. doi: 10.1016/j.intimp.2013.06.034
109. Dreyfuss JL, Regatieri CV, Jarrouge TR, Cavalheiro RP, Sampaio LO, Nader HB. Heparan sulfate proteoglycans: structure, protein interactions and cell signaling. An Acad Bras Cienc. (2009) 81:409–29. doi: 10.1590/S0001-37652009000300007
110. Christianson HC, Belting M. Heparan sulfate proteoglycan as a cell-surface endocytosis receptor. Matrix Biol. (2014) 35:51–5. doi: 10.1016/j.matbio.2013.10.004
111. Milewska A, Nowak P, Owczarek K, Szczepanski A, Zarebski M, Hoang A, et al. Entry of human coronavirus NL63 into the cell. J Virol. (2018) 92:e01933–17. doi: 10.1128/JVI.01933-17
112. Xu D, Olson J, Cole JN, van Wijk XM, Brinkmann V, Zychlinsky A, et al. Heparan sulfate modulates neutrophil and endothelial function in antibacterial innate immunity. Infect Immun. (2015) 83:3648–56. doi: 10.1128/IAI.00545-15
113. Elass-Rochard E, Legrand D, Salmon V, Roseanu A, Trif M, Tobias PS, et al. Lactoferrin inhibits the endotoxin interaction with CD14 by competition with the lipopolysaccharide-binding protein. Infect Immun. (1998) 66:486–91. doi: 10.1128/IAI.66.2.486-491.1998
114. Baveye S, Elass E, Mazurier J, Spik G, Legrand D. Lactoferrin: a multifunctional glycoprotein involved in the modulation of the inflammatory process. Clin Chem Lab Med. (1999) 37:281–6. doi: 10.1515/CCLM.1999.049
115. Kimoto Y, Nishinohara M, Sugiyama A, Haruna A, Takeuchi T. Protective effect of lactoferrin on cisplatin-induced nephrotoxicity in rats. J Vet Med Sci. (2013) 75:159–64. doi: 10.1292/jvms.12-0154
116. Hediger MA, Clemencon B, Burrier RE, Bruford EA. The ABCs of membrane transporters in health and disease (SLC series): introduction. Mol Aspects Med. (2013) 34:95–107. doi: 10.1016/j.mam.2012.12.009
117. Dobson PD, Kell DB. Carrier-mediated cellular uptake of pharmaceutical drugs: an exception or the rule? Nat Rev Drug Disc. (2008) 7:205–20. doi: 10.1038/nrd2438
118. Kell DB. What would be the observable consequences if phospholipid bilayer diffusion of drugs into cells is negligible? Trends Pharmacol Sci. (2015) 36:15–21. doi: 10.1016/j.tips.2014.10.005
119. Kell DB. The transporter-mediated cellular uptake of pharmaceutical drugs is based on their metabolite-likeness and not on their bulk biophysical properties: towards a systems pharmacology. Perspect Sci. (2015) 6:66–83. doi: 10.1016/j.pisc.2015.06.004
120. Kell DB, Dobson PD, Bilsland E, Oliver SG. The promiscuous binding of pharmaceutical drugs and their transporter-mediated uptake into cells: what we (need to) know and how we can do so. Drug Disc Today. (2013) 18:218–39. doi: 10.1016/j.drudis.2012.11.008
121. Kell DB, Dobson PD, Oliver SG. Pharmaceutical drug transport: the issues and the implications that it is essentially carrier-mediated only. Drug Disc Today. (2011) 16:704–14. doi: 10.1016/j.drudis.2011.05.010
122. Kell DB, Oliver SG. How drugs get into cells: tested and testable predictions to help discriminate between transporter-mediated uptake and lipoidal bilayer diffusion. Front Pharmacol. (2014) 5:231. doi: 10.3389/fphar.2014.00231
123. Superti-Furga G, Lackner D, Wiedme T, Ingles-Prieto A, Barbosa B, Girardi E, et al. The RESOLUTE consortium: unlocking SLC transporters for drug discovery. Nat Rev Drug Discov. (2020). doi: 10.1038/d41573-020-00056-6. [Epub ahead of print]
124. Girardi E, César-Razquin A, Lindinger S, Papakostas K, Konecka J, Hemmerich J, et al. A widespread role for SLC transmembrane transporters in resistance to cytotoxic drugs. Nat Chem Biol. (2020) 16:469–78. doi: 10.1038/s41589-020-0483-3
125. Harada E, Itoh Y, Sitizyo K, Takeuchi T, Araki Y, Kitagawa H. Characteristic transport of lactoferrin from the intestinal lumen into the bile via the blood in piglets. Comp Biochem Physiol A Mol Integr Physiol. (1999) 124:321–7. doi: 10.1016/S1095-6433(99)00122-1
126. Matsuzaki T, Nakamura M, Nogita T, Sato A. Cellular uptake and release of intact lactoferrin and its derivatives in an intestinal enterocyte model of Caco-2 cells. Biol Pharm Bull. (2019) 42:989–95. doi: 10.1248/bpb.b19-00011
127. Talukder MJ, Takeuchi T, Harada E. Characteristics of lactoferrin receptor in bovine intestine: higher binding activity to the epithelium overlying Peyer's patches. J Vet Med A Physiol Pathol Clin Med. (2003) 50:123–31. doi: 10.1046/j.1439-0442.2003.00512.x
128. Takeuchi T, Jyonotsuka T, Kamemori N, Kawano G, Shimizu H, Ando K, et al. Enteric-formulated lactoferrin was more effectively transported into blood circulation from gastrointestinal tract in adult rats. Exp Physiol. (2006) 91:1033–40. doi: 10.1113/expphysiol.2006.034876
129. Ishikado A, Imanaka H, Takeuchi T, Harada E, Makino T. Liposomalization of lactoferrin enhanced it's anti-inflammatory effects via oral administration. Biol Pharm Bull. (2005) 28:1717–21. doi: 10.1248/bpb.28.1717
130. Roseanu A, Florian PE, Moisei M, Sima LE, Evans RW, Trif M. Liposomalization of lactoferrin enhanced its anti-tumoral effects on melanoma cells. Biometals. (2010) 23:485–92. doi: 10.1007/s10534-010-9312-6
131. Takeuchi T, Kitagawa H, Harada E. Evidence of lactoferrin transportation into blood circulation from intestine via lymphatic pathway in adult rats. Exp Physiol. (2004) 89:263–70. doi: 10.1113/expphysiol.2003.026633
132. Wakabayashi H, Kuwata H, Yamauchi K, Teraguchi S, Tamura Y. No detectable transfer of dietary lactoferrin or its functional fragments to portal blood in healthy adult rats. Biosci Biotechnol Biochem. (2004) 68:853–60. doi: 10.1271/bbb.68.853
133. Kamemori N, Takeuchi T, Sugiyama A, Miyabayashi M, Kitagawa H, Shimizu H, et al. Trans-endothelial and trans-epithelial transfer of lactoferrin into the brain through BBB and BCSFB in adult rats. J Vet Med Sci. (2008) 70:313–5. doi: 10.1292/jvms.70.313
134. Talukder MJ, Takeuchi T, Harada E. Receptor-mediated transport of lactoferrin into the cerebrospinal fluid via plasma in young calves. J Vet Med Sci. (2003) 65:957–64. doi: 10.1292/jvms.65.957
135. Reghunathan R, Jayapal M, Hsu LY, Chng HH, Tai D, Leung BP, et al. Expression profile of immune response genes in patients with Severe Acute Respiratory Syndrome. BMC Immunol. (2005) 6:2. doi: 10.1186/1471-2172-6-2
136. Brinkmann V, Reichard U, Goosmann C, Fauler B, Uhlemann Y, Weiss DS, et al. Neutrophil extracellular traps kill bacteria. Science. (2004) 303:1532–5. doi: 10.1126/science.1092385
137. F.Castanheira VS, Kubes P. Neutrophils and NETs in modulating acute and chronic inflammation. Blood. (2019) 133:2178–85. doi: 10.1182/blood-2018-11-844530
138. Hahn J, Knopf J, Maueröder C, Kienhöfer D, Leppkes M, Herrmann M. Neutrophils and neutrophil extracellular traps orchestrate initiation and resolution of inflammation. Clin Exp Rheumatol. (2016) 34:6–8. Available online at: https://www.clinexprheumatol.org/abstract.asp?a=10879
139. Lee KH, Kronbichler A, Park DD, Park Y, Moon H, Kim H, et al. Neutrophil extracellular traps (NETs) in autoimmune diseases: a comprehensive review. Autoimmun Rev. (2017) 16:1160–73. doi: 10.1016/j.autrev.2017.09.012
140. Papayannopoulos V. Neutrophil extracellular traps in immunity and disease. Nat Rev Immunol. (2018) 18:134–47. doi: 10.1038/nri.2017.105
141. Urban CF, Ermert D, Schmid M, Abu-Abed U, Goosmann C, Nacken W, et al. Neutrophil extracellular traps contain calprotectin, a cytosolic protein complex involved in host defense against Candida albicans. PLoS Pathog. (2009) 5:e1000639. doi: 10.1371/journal.ppat.1000639
142. Jorch SK, Kubes P. An emerging role for neutrophil extracellular traps in noninfectious disease. Nat Med. (2017) 23:279–87. doi: 10.1038/nm.4294
143. Law SM, Gray RD. Neutrophil extracellular traps and the dysfunctional innate immune response of cystic fibrosis lung disease: a review. J Inflamm. (2017) 14:29. doi: 10.1186/s12950-017-0176-1
144. Petrik M, Zhai C, Haas H, Decristoforo C. Siderophores for molecular imaging applications. Clin Transl Imaging. (2017) 5:15–27. doi: 10.1007/s40336-016-0211-x
145. Beddek AJ, Schryvers AB. The lactoferrin receptor complex in Gram negative bacteria. Biometals. (2010) 23:377–86. doi: 10.1007/s10534-010-9299-z
146. Pogoutse AK, Moraes TF. Iron acquisition through the bacterial transferrin receptor. Crit Rev Biochem Mol Biol. (2017) 52:314–26. doi: 10.1080/10409238.2017.1293606
147. Wandersman C, Stojiljkovic I. Bacterial heme sources: the role of heme, hemoprotein receptors and hemophores. Curr Opin Microbiol. (2000) 3:215–20. doi: 10.1016/S1369-5274(00)00078-3
148. Huang W, Wilks A. Extracellular heme uptake and the challenge of bacterial cell membranes. Annu Rev Biochem. (2017) 86:799–823. doi: 10.1146/annurev-biochem-060815-014214
149. Redwan EM, Uversky VN, El-Fakharany EM, Al-Mehdar H. Potential lactoferrin activity against pathogenic viruses. C R Biol. (2014) 337:581–95. doi: 10.1016/j.crvi.2014.08.003
150. Chen JM, Fan YC, Lin JW, Chen YY, Hsu WL, Chiou SS. Bovine lactoferrin inhibits dengue virus infectivity by interacting with heparan sulfate, low-density lipoprotein receptor, and DC-SIGN. Int J Mol Sci. (2017) 18:E1957. doi: 10.3390/ijms18091957
151. Carvalho CAM, Casseb SMM, Goncalves RB, Silva EVP, Gomes AMO, Vasconcelos PFC. Bovine lactoferrin activity against Chikungunya and Zika viruses. J Gen Virol. (2017) 98:1749–54. doi: 10.1099/jgv.0.000849
152. Fernandes KE, Carter DA. The antifungal activity of lactoferrin and its derived peptides: mechanisms of action and synergy with drugs against fungal pathogens. Front Microbiol. (2017) 8:2. doi: 10.3389/fmicb.2017.00002
153. Liao H, Liu S, Wang H, Su H, Liu Z. Enhanced antifungal activity of bovine lactoferrin-producing probiotic Lactobacillus casei in the murine model of vulvovaginal candidiasis. BMC Microbiol. (2019) 19:7. doi: 10.1186/s12866-018-1370-x
154. Andrés MT, Acosta-Zaldívar M, Fierro JF. Antifungal mechanism of action of lactoferrin: identification of H+-ATPase (P3A-type) as a new apoptotic-cell membrane receptor. Antimicrob Agents Chemother. (2016) 60:4206–16. doi: 10.1128/AAC.03130-15
155. Wang B, Timilsena YP, Blanch E, Adhikari B. Lactoferrin: structure, function, denaturation and digestion. Crit Rev Food Sci Nutr. (2019) 59:580–96. doi: 10.1080/10408398.2017.1381583
156. Nairz M, Schroll A, Sonnweber T, Weiss G. The struggle for iron - a metal at the host-pathogen interface. Cell Microbiol. (2010) 12:1691–702. doi: 10.1111/j.1462-5822.2010.01529.x
157. Brooks CL, Arutyunova E, Lemieux MJ. The structure of lactoferrin-binding protein B from Neisseria meningitidis suggests roles in iron acquisition and neutralization of host defences. Acta Crystallogr F Struct Biol Commun. (2014) 70:1312–7. doi: 10.1107/S2053230X14019372
158. Weinberg ED. Iron availability and infection. Biochim Biophys Acta. (2009) 1790:600–5. doi: 10.1016/j.bbagen.2008.07.002
159. Schryvers AB, Stojiljkovic I. Iron acquisition systems in the pathogenic Neisseria. Mol Microbiol. (1999) 32:1117–23. doi: 10.1046/j.1365-2958.1999.01411.x
160. Fransson GB, Lönnerdal B. Iron in human milk. J Pediatr. (1980) 96:380–4. doi: 10.1016/S0022-3476(80)80676-7
161. Legrand D. Overview of lactoferrin as a natural immune modulator. J Pediatr. (2016) 173(Suppl.):S10–5. doi: 10.1016/j.jpeds.2016.02.071
162. Belting M. Heparan sulfate proteoglycan as a plasma membrane carrier. Trends Biochem Sci. (2003) 28:145–51. doi: 10.1016/S0968-0004(03)00031-8
163. Drobni P, Näslund J, Evander M. Lactoferrin inhibits human papillomavirus binding and uptake in vitro. Antiviral Res. (2004) 64:63–8. doi: 10.1016/S0166-3542(04)00123-8
164. Puddu P, Borghi P, Gessani S, Valenti P, Belardelli F, Seganti L. Antiviral effect of bovine lactoferrin saturated with metal ions on early steps of human immunodeficiency virus type 1 infection. Int J Biochem Cell Biol. (1998) 30:1055–62. doi: 10.1016/S1357-2725(98)00066-1
165. Superti F, Siciliano R, Rega B, Giansanti F, Valenti P, Antonini G. Involvement of bovine lactoferrin metal saturation, sialic acid and protein fragments in the inhibition of rotavirus infection. Biochim Biophys Acta. (2001) 1528:107–15. doi: 10.1016/S0304-4165(01)00178-7
166. Mehta P, McAuley DF, Brown M, Sanchez E, Tattersall RS, Manson JJ. COVID-19: consider cytokine storm syndromes and immunosuppression. Lancet. (2020) 395:1033–4. doi: 10.1016/S0140-6736(20)30628-0
167. Kell DB, Pretorius E. To what extent are the terminal stages of sepsis, septic shock, systemic inflammatory response syndrome, and multiple organ dysfunction syndrome actually driven by a prion/amyloid form of fibrin? Semin Thromb Hemost. (2018) 44: 224–38.
168. Wu D, Yang XO. TH17 responses in cytokine storm of COVID-19: an emerging target of JAK2 inhibitor Fedratinib. J Microbiol Immunol Infect. (2020). doi: 10.1016/j.jmii.2020.03.005. [Epub ahead of print]
169. Zenewicz LA. IL-22: there is a gap in our knowledge. Immunohorizons. (2018) 2:198–207. doi: 10.4049/immunohorizons.1800006
170. Tse GM, To KF, Chan PK, Lo AW, Ng KC, Wu A, et al. Pulmonary pathological features in coronavirus associated severe acute respiratory syndrome (SARS). J Clin Pathol. (2004) 57:260–5. doi: 10.1136/jcp.2003.013276
171. Wan Y, Shang J, Graham R, Baric RS, Li F. Receptor recognition by the novel coronavirus from wuhan: an analysis based on decade-long structural studies of SARS coronavirus. J Virol. (2020) 94:e00127-20. doi: 10.1128/JVI.00127-20
172. Baig AM, Khaleeq A, Ali U, Syeda H. Evidence of the COVID-19 virus targeting the CNS: tissue distribution, host-virus interaction, and proposed neurotropic mechanisms. ACS Chem Neurosci. (2020) 11:995–8. doi: 10.1021/acschemneuro.0c00122
173. D'Elia RV, Harrison K, Oyston PC, Lukaszewski RA, Clark GC. Targeting the “cytokine storm” for therapeutic benefit. Clin Vaccine Immunol. (2013) 20:319–27. doi: 10.1128/CVI.00636-12
174. Lippi G, Plebani M, Henry BM. Thrombocytopenia is associated with severe coronavirus disease 2019 (COVID-19) infections: a meta-analysis. Clin Chim Acta. (2020) 506:145–8. doi: 10.1016/j.cca.2020.03.022
175. Zhang G, Zhang J, Wang B, Zhu X, Wang Q, Qiu S. Analysis of clinical characteristics and laboratory findings of 95 cases of 2019 novel coronavirus pneumonia in Wuhan, China: a retrospective analysis. Respir Res. (2020) 21:74. doi: 10.1186/s12931-020-01338-8
176. Guo W, Li M, Dong Y, Zhou H, Zhang Z, Tian C, et al. Diabetes is a risk factor for the progression and prognosis of COVID-19. Diabetes Metab Res Rev. (2020) e3319. doi: 10.1002/dmrr.3319. [Epub ahead of print].
177. Danzi GB, Loffi M, Galeazzi G, Gherbesi E. Acute pulmonary embolism and COVID-19 pneumonia: a random association? Eur Heart J. (2020). doi: 10.1093/eurheartj/ehaa254. [Epub ahead of print].
178. Heidt T, Ehrismann S, Hövener JB, Neudorfer I, Hilgendorf I, Reisert M, et al. Molecular imaging of activated platelets allows the detection of pulmonary embolism with magnetic resonance imaging. Sci Rep. (2016) 6:25044. doi: 10.1038/srep25044
179. Tang N, Bai H, Chen X, Gong J, Li D, Sun Z. Anticoagulant treatment is associated with decreased mortality in severe coronavirus disease 2019 patients with coagulopathy. J Thromb Haemost. (2020) 18:1094–99. doi: 10.1111/jth.14851
180. Ames BN. Prolonging healthy aging: longevity vitamins and proteins. Proc Natl Acad Sci USA. (2018) 115:10836–44. doi: 10.1073/pnas.1809045115
181. Borodina I, Kenny LC, McCarthy CM, Paramasivan K, Pretorius R, Roberts TJ, et al. The biology of ergothioneine, an antioxidant nutraceutical. Nutr Res Rev. (2020) 1−28. doi: 10.1017/S0954422419000301. [Epub ahead of print].
182. Kawakami H, Park H, Park S, Kuwata H, Shephard RJ, Aoyagi Y. Effects of enteric-coated lactoferrin supplementation on the immune function of elderly individuals: a randomised, double-blind, placebo-controlled trial. Int Dairy J. (2015) 47:79–85. doi: 10.1016/j.idairyj.2015.02.001
183. Bellamy W, Takase M, Yamauchi K, Wakabayashi H, Kawase K, Tomita M. Identification of the bactericidal domain of lactoferrin. Biochim Biophys Acta. (1992) 1121:130–6. doi: 10.1016/0167-4838(92)90346-F
184. Pammi M, Suresh G. Enteral lactoferrin supplementation for prevention of sepsis and necrotizing enterocolitis in preterm infants. Cochrane Database Syst Rev. (2017) 6:CD007137. doi: 10.1002/14651858.CD007137.pub5
185. Cooper CA, Nelson KM, Maga EA, Murray JD. Consumption of transgenic cows' milk containing human lactoferrin results in beneficial changes in the gastrointestinal tract and systemic health of young pigs. Transgenic Res. (2013) 22:571–8. doi: 10.1007/s11248-012-9662-7
186. Wang M, Sun Z, Yu T, Ding F, Li L, Wang X, et al. Large-scale production of recombinant human lactoferrin from high-expression, marker-free transgenic cloned cows. Sci Rep. (2017) 7:10733. doi: 10.1038/s41598-017-11462-z
187. Russo R, Edu A, De Seta. F. Study on the effects of an oral lactobacilli and lactoferrin complex in women with intermediate vaginal microbiota. Arch Gynecol Obstet. (2018) 298:139–45. doi: 10.1007/s00404-018-4771-z
188. Patras KA, Ha AD, Rooholfada E, Olson J, Ramachandra Rao SP, Lin AE, et al. Augmentation of urinary lactoferrin enhances host innate immune clearance of uropathogenic Escherichia coli. J Innate Immun. (2019) 11:481–95. doi: 10.1159/000499342
189. Chanda W, Joseph TP, Wang W, Padhiar AA, Zhong M. The potential management of oral candidiasis using anti-biofilm therapies. Med Hypotheses. (2017) 106:15–8. doi: 10.1016/j.mehy.2017.06.029
190. Sessa R, Di Pietro M, Filardo S, Bressan A, Mastromarino P, Biasucci AV, et al. Lactobacilli-lactoferrin interplay in Chlamydia trachomatis infection. Pathog Dis. (2017) 75. doi: 10.1093/femspd/ftx054
191. Morita Y, Ishikawa K, Nakano M, Wakabayashi H, Yamauchi K, Abe F, et al. Effects of lactoferrin and lactoperoxidase-containing food on the oral hygiene status of older individuals: a randomized, double blinded, placebo-controlled clinical trial. Geriatr Gerontol Int. (2017) 17:714–21. doi: 10.1111/ggi.12776
192. Sangermano R, Pernarella S, Straker M, Lepanto MS, Rosa L, Cutone A, et al. The treatment of black stain associated with of iron metabolism disorders with lactoferrin: a litterature search and two case studies. Clin Ter. (2019) 170:e373–81. doi: 10.7417/CT.2019.2163
193. Cutone A, Lepanto MS, Rosa L, Scotti MJ, Rossi A, Ranucci S, et al. Aerosolized bovine lactoferrin counteracts infection, inflammation and iron dysbalance in a cystic fibrosis mouse model of Pseudomonas aeruginosa chronic lung infection. Int J Mol Sci. (2019) 20:E2128. doi: 10.3390/ijms20092128
194. Marshall LJ, Oguejiofor W, Price R, Shur J. Investigation of the enhanced antimicrobial activity of combination dry powder inhaler formulations of lactoferrin. Int J Pharm. (2016) 514:399–406. doi: 10.1016/j.ijpharm.2016.09.034
195. Oda H, Miyakawa M, Mizuki M, Misawa Y, Tsukahara T, Tanaka M, et al. Effects of lactoferrin on subjective skin conditions in winter: a preliminary, randomized, double-blinded, placebo-controlled trial. Clin Cosmet Investig Dermatol. (2019) 12:875–80. doi: 10.2147/CCID.S228153
Keywords: lactoferrin, coronaviruses, iron, membrane receptors, HSPGs
Citation: Kell DB, Heyden EL and Pretorius E (2020) The Biology of Lactoferrin, an Iron-Binding Protein That Can Help Defend Against Viruses and Bacteria. Front. Immunol. 11:1221. doi: 10.3389/fimmu.2020.01221
Received: 09 April 2020; Accepted: 15 May 2020;
Published: 28 May 2020.
Edited by:
Slobodan Paessler, University of Texas Medical Branch at Galveston, United StatesReviewed by:
Elena Martinelli, Population Council, United StatesCopyright © 2020 Kell, Heyden and Pretorius. This is an open-access article distributed under the terms of the Creative Commons Attribution License (CC BY). The use, distribution or reproduction in other forums is permitted, provided the original author(s) and the copyright owner(s) are credited and that the original publication in this journal is cited, in accordance with accepted academic practice. No use, distribution or reproduction is permitted which does not comply with these terms.
*Correspondence: Douglas B. Kell, ZGJrQGxpdi5hYy51aw==; Etheresia Pretorius, cmVzaWFwQHN1bi5hYy56YQ==
†ORCID: Douglas B. Kell orcid.org/0000-0001-5838-7963
Etheresia Pretorius orcid.org/0000-0002-9108-2384
Disclaimer: All claims expressed in this article are solely those of the authors and do not necessarily represent those of their affiliated organizations, or those of the publisher, the editors and the reviewers. Any product that may be evaluated in this article or claim that may be made by its manufacturer is not guaranteed or endorsed by the publisher.
Research integrity at Frontiers
Learn more about the work of our research integrity team to safeguard the quality of each article we publish.