- 1Laboratory for Molecular Infection Medicine Sweden (MIMS) -The Nordic EMBL Partnership for Molecular Medicine, Umeå University, Umeå, Sweden
- 2Department of Molecular Biology, Umeå University, Umeå, Sweden
A crucial mechanism of intestinal defense includes the production and secretion of host defense peptides (HDPs). HDPs control pathogens and commensals at the intestinal interface by direct killing, by sequestering vital ions, or by causing bacterial cells to aggregate in the mucus layer. Accordingly, the combined activity of various HDPs neutralizes gut bacteria before reaching the mucosa and thus helps to maintain the homeostatic balance between the host and its microbes at the mucosal barrier. Defects in the mucosal barrier have been associated with various diseases that are on the rise in the Western world. These include metabolic diseases, such as obesity and type 2 diabetes, and inflammatory intestinal disorders, including ulcerative colitis and Crohn's disease, the two major entities of inflammatory bowel disease. While the etiology of these diseases is multifactorial, highly processed Western-style diet (WSD) that is rich in carbohydrates and fat and low in dietary fiber content, is considered to be a contributing lifestyle factor. As such, WSD does not only profoundly affect the resident microbes in the intestine, but can also directly alter HDP function, thereby potentially contributing to intestinal mucosal barrier dysfunction. In this review we aim to decipher the complex interaction between diet, microbiota, and HDPs. We discuss how HDP expression can be modulated by specific microbes and their metabolites as well as by dietary factors, including fibers, lipids, polyphenols and vitamins. We identify several dietary compounds that lead to reduced HDP function, but also factors that stimulate HDP production in the intestine. Furthermore, we argue that the effect of HDPs against commensal bacteria has been understudied when compared to pathogens, and that local environmental conditions also need to be considered. In addition, we discuss the known molecular mechanisms behind HDP modulation. We believe that a better understanding of the diet-microbiota-HDP interdependence will provide insights into factors underlying modern diseases and will help to identify potential dietary interventions or probiotic supplementation that can promote HDP-mediated intestinal barrier function in the Western gut.
Introduction
The human gut is the interface between the body and the environment and is colonized by a community of trillions of microorganisms, including bacteria, fungi, and archaea. While the small intestine is responsible for nutrient absorption, the large intestine can rather be considered as a bioreactor in which gut bacteria carry out different biological functions, such as processing of dietary fibers (1, 2), maturation and regulation of the immune system (3, 4), and production of metabolites that exhibit various metabolic and neurological effects (5–7). At the intestinal interface, the immune system has the challenging task of maintaining a stable microbiota by keeping beneficial commensal bacteria at bay and by recognizing and eliminating disease-causing microbes. When this equilibrium is lost, the microbiota composition enters a state termed “dysbiosis,” which has been associated with a wide array of diseases.
Several environmental factors are known to directly alter or disturb the microbial composition, including diet and medicine use (8), but also intrinsic host factors such as host defense peptides (HDPs) (9), and host genetics (10). Therefore, strict regulation of immune signals in response to intrinsic and extrinsic stimuli is prompted at the intestinal interface to maintain homeostasis.
The intestinal defense system is composed of the gut associated lymphoid tissue (GALT), formed by a single layer of intestinal epithelial cells that are arranged in crypts and villi, and the underlying mesenteric lymph nodes and lamina propria. Goblet cells are dispersed over the epithelial layer and secrete mucus that functions as a physical barrier to maintain microorganisms at a safe distance from the intestinal epithelium. Immunoglobulin A (IgA)-secreting B-cells contribute to controlling local microbial communities (11). Paneth cells are specialized small intestinal cells at the bottom of the crypts of Lieberkühn that specialize in the production of HDPs, and together with enterocytes, which produce HDPs in the small and large intestine, they represent the primary source of HDPs in the gut.
HDPs are mostly small cationic peptides with unique mechanisms of action and different specificity against Gram-positive and Gram-negative bacteria (12). These antimicrobial molecules are the effector molecules of the intestinal immunity with potent bactericidal activity that has mostly been tested against intestinal pathogens (13). On the contrary, much less is known about how HDPs affect commensal bacteria, and several studies suggest that antimicrobial activity against the resident microbiota is comparably low or even absent (14–16). Yet, two independent studies demonstrated that transgenic intestinal expression or oral application of human alpha-defensin 5 (HD5) in mice could shape the intestinal microbiota composition in vivo (9, 17). It is therefore possible that previous activity testings of HDPs in in vitro assays did not appropriately reflect the in vivo conditions, as already demonstrated for human beta-defensins 1 (HBD1) and the Paneth cell-derived human alpha defensin 6 (HD6), which gained activity under adjusted conditions that reflected the intestinal microenvironment (18, 19). However, we are only about to begin to understand how HDPs affect commensal microbes and how the functionality of this defense system can influence the way the host copes with its inner microbial world in the intestine.
The secretion of Paneth cell HDPs can occur in response to bacterial stimuli and is largely regulated by signals from the transcription factor 4 (TCF-4)/Wnt signaling pathway in Paneth cells, while epithelial-derived HDPs rather seem to be controlled through IL-22, derived from immune cells (12, 20, 21). Microbial ligands are recognized through pattern recognition receptors (PPRs) present in intestinal epithelial cells or immune cells. Upon recognition and activation, immune cells of the GALT send signals to Paneth cells, goblet cells and enterocytes to coordinate their function and maintain the epithelial barrier function (22). In addition, the presence of microbes and their metabolites seems to be implicated in the control of the antimicrobial programming at the intestine, as germ-free (GF) mice have reduced HDP expression (23) and since probiotic supplementation or microbial-metabolite enrichment stimulates HDP production (24–27).
Members of the gut microbiota can influence HDP expression, and diet is considered one of the most influencing factors determining gut microbiota composition. Accordingly, diet composition, and whether it is of animal or plant-based origin, has profound implications in defining the gut microbial composition. For example, a diet rich in plant-derived fiber is associated with increased diversity in microbial communities, and more specifically, with an increase in Bifidobacterium abundance, which has been shown to be a positive regulator of intestinal barrier function (28, 29). As for proteins, animal-derived proteins were shown to decrease the abundance of Firmicutes, a phylum that has been associated with obesity and high body mass index (30), whereas plant-derived proteins were shown to promote the growth of beneficial Bifidobacterium and Lactobacillus genera and reduce the abundance of pathogenic bacteria (31). A Western-style diet (WSD), characterized by its low dietary fiber but high-fat and high carbohydrate content, markedly changes the microbiota composition in humans and mice (29, 32–35). Moreover, a WSD promotes a pro-inflammatory response through different dietary components (e.g., cholesterol, saturated and non-saturated fatty acids) and can cause microbiota-induced mucus defects as a result of the reduced fiber content (29, 36–38). Importantly, various studies indicate that the increased consumption of WSD in our modern societies, often accompanied by food additives such as artificial sweeteners and emulsifiers, is likely one of the drivers for the worldwide increase in non-communicable diseases, including metabolic syndrome and inflammatory bowel disease (IBD) (3, 39–42).
In this review, we summarize recent findings linking the effect of microbiota, diet, and food availability on the HDP-mediated intestinal defense function during intestinal homeostasis. Moreover, a defective HDP function has been linked to modern diseases associated with a Western-lifestyle, including IBD and metabolic disease. In particular, reduced levels of human defensins have been described in ileal Crohn's disease (43–45). Therefore, we also aim to decipher possible interactions along the diet-HDP-microbiota axis (Figure 1), that could be relevant in Western diseases, in which gut microbiota can gain access to the host epithelium due to an impaired barrier function. In that context, modulation of the gut microbiota through diet has been much discussed as a therapeutic alternative to protect the intestinal epithelium. Thus, we pose the question whether an apple a day keeps the microbes away, and we chose this fruit for several reasons: an apple is an easy-accessible every-day product that does not only contain fibers and polyphenols to potentially support the growth of HDP-stimulating bacteria, as we discuss below, but it was also recently shown that apples carry thousands of bacteria (46). Thus, it is theoretically possible that this fruit could serve as a natural pre- and pro-biotic to strengthen antimicrobial HDP function in the gut.
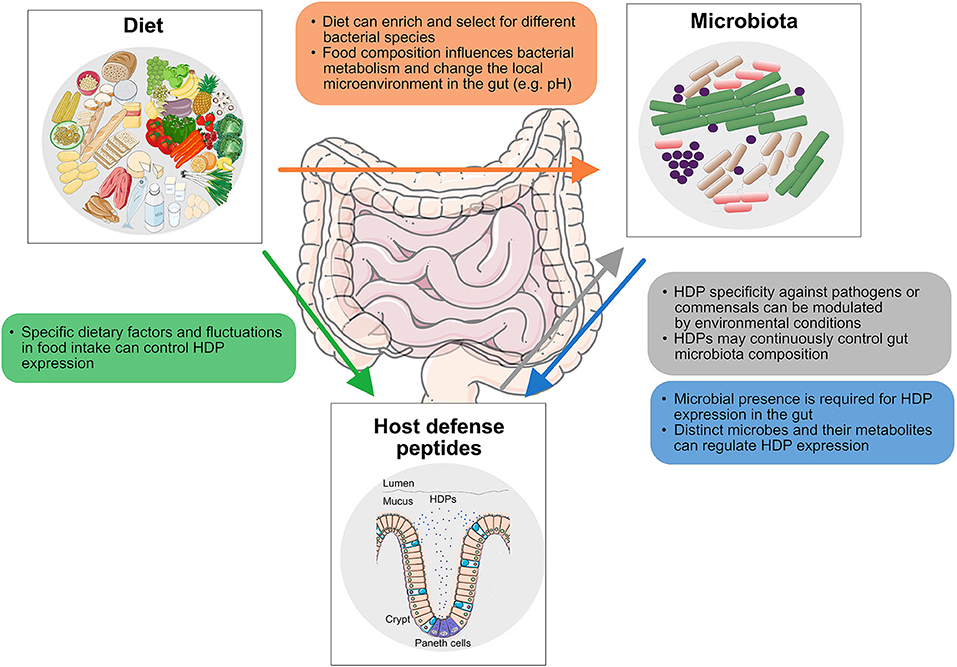
Figure 1. Interactions along the diet-HDP-microbiota axis discussed in this study. The impact of diet on gut microbiota composition (orange), the effect of diet on host defense peptide (HDP) expression (green), the activity of HDPs against gut microbes (gray), and the influence of bacteria and associated metabolites in HDP expression (blue) are displayed.
Microbiota In Health and Disease
The human microbiota is generally dominated by the Bacteroidetes (Bacteroides, Parabacteroides, Prevotella, Alistipes genera) and Firmicutes (Clostridium, Eubacterium, Blautia, Roseburia, Lactobacillus, Faecalibacterium, Ruminococcus, Streptococcus genera) phyla. Other phyla, such as Proteobacteria (Escherichia genus), Actinobacteria (Bifidobacterium genus), and Verrucomicrobia (Akkermansia genus), are less represented and ratios of these phyla vary highly between individuals (47). A classification into “enterotype” groups was previously proposed, based on the function and relative abundance of the Bacteroides, Prevotella, and Ruminococcus genera within an individual, but the authors also stressed the fact that non-abundant species can exert high-abundant functions (e.g., methanogens), and that high-abundant microbes should thus not be regarded as solely responsible for the entire functionality of the human intestinal microbiota (48). Consequently, enterotypes do not seem to be as discrete as previously suggested, as they can be confounded by environmental variables, the clustering model used and stability over time (49).
Numerous studies have collectively attempted to define what constitutes a healthy microbiota, as the gut microbiota of healthy and diseased individuals differs in its composition. For example, the microbiota has been implicated in several disorders, such as IBD (50–52), obesity (53–56), diabetes (57–59), allergic diseases (60, 61), Parkinson's disease (62), autism spectrum disorder (63), and atherosclerosis (64), among others. Although there is in most cases no solid evidence that changes in the microbiota may cause these diseases, these microbial associations have encouraged the effort of finding strategies to modulate the microbial community composition through dietary intervention to improve the symptoms accompanying these disorders. Yet, the establishment of a healthy “ideal” microbiota is complex, as many factors are known to influence its composition (65). Here, we will focus on two key factors that are continuously affecting the intestinal microbiota, namely diet, and HDPs.
Dietary Influence on Gut Microbiota Composition
The impact of different diets on the intestinal microbiota has been extensively reviewed in recent years (28, 30, 31, 66). The composition of the diet (defined by macronutrient ratio—carbohydrates, fats and proteins), the origin of these components (plant or animal-based) and the availability of different dietary factors are recognized as determinants of gut microbial metabolism and composition, with the potential to influence human health (67, 68) (Figure 2). The three major macronutrients carbohydrates, fats and proteins, can reach the colon after escaping the primary digestion in the small intestine when the intake surpasses the rate of digestion, or due to the biomolecules' intrinsic structural complexity (69–71). Therefore, the proportion of macronutrients present in, for example, a Western-style, protein-rich, vegan, vegetarian, or fiber-rich diet will have different effects on the colonic microbiota.
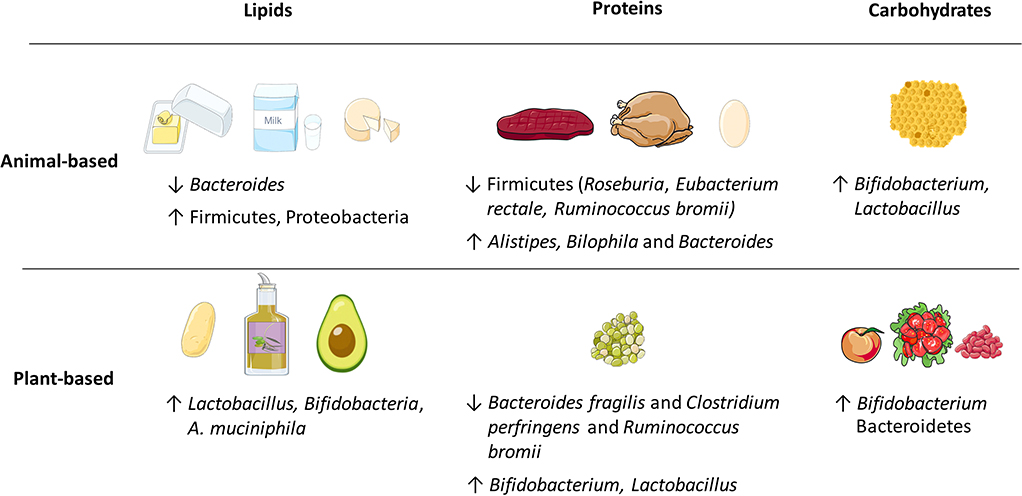
Figure 2. Examples of energy-delivering macronutrients, including lipids, proteins, and carbohydrates that produce various changes in the relative abundance of gut microbiota. The animal or plant-based origin dramatically influences the outcomes.
Fiber
The dietary compound that has been found to be the strongest contributor to gut microbial community structure is dietary fiber. Dietary fiber is almost exclusively of plant-based origin and can be found as soluble or insoluble carbohydrate polymers that are inaccessible to the human body due to the limited number (ca. 17) of carbohydrate-active enzymes (CAZymes) (2). In contrast, it is estimated that the gut microbiota is equipped with 11,000 CAZymes that carry out the hydrolysis of different sets of soluble fibers (72, 73), also known as microbiota-accessible carbohydrates (MACs) (2). As a result of bacterial fiber fermentation, short chain fatty acids (SCFAs), including acetate, propionate, and butyrate, and gases such as H2 and CO2, are produced by different gut bacteria in a complex cross-feeding network. Enterocytes utilize SCFAs as an energy substrate, and these metabolites have also been shown to improve the intestinal barrier integrity, regulate glucose homeostasis and lipid metabolism, and induce both anti-inflammatory and tolerogenic immune reactions (74). Conversely, insoluble fibers are not fermented by the microbiota and do not convey the aforementioned benefits.
Microbial fermentation is determined by the origin, chemical composition and physicochemical properties of the fibers present in food (28). As a rare example of animal-derived carbohydrate, honey includes a diverse mixture of mono- and disaccharides as well as complex carbohydrates. Although the effect will depend on the specific type, honey was shown to promote the growth of Bifidobacterium and Lactobacillus (75). Fibers that originate from plants—derived from either cereals, grains, vegetables, legumes or nuts—have unique chemical compositions and physicochemical properties (28). Therefore, the variety of fibers present in plant-based diets can support more diverse gut microbial communities (76, 77).
Fruits are another common source of plant-derived fibers. For example, complex pectins found in apples and wine can be degraded by Bacteroides thetaiotaomicron (B. thetaiotaomicron) (78), and kiwifruit supplements increased the abundance of Faecalibacterium prausnitzii (F. prausnitzii) in patients with constipation (79), while a crude extract of kiwi was shown to support the growth of Bifidobacterium and Bacteroides in vitro (80).
Clinical studies assessing the impact of different types of fibers on the microbiota report that Bifidobacterium spp. are enriched following consumption of diets with certain fibers, including galacto-oligosaccharides (GOS), inulin-type fructans, xylo-oligosaccharides, and arabinoxylan-oligosaccharides, and that microbes in the Bacteroidetes and Firmicutes phyla are differentially stimulated by soluble fibers from corn or polydextrose (28, 81). In addition, studies comparing the low-fiber diet of Westernized populations with the high-fiber diet of unindustrialized communities show dramatic differences in the microbiota composition between both populations (34, 35), including that the Westernized societies having decreased diversity and apparent loss of certain microbes that are present in the unindustrialized communities (82).
Lipids
A high-fat WSD, mainly containing saturated or trans-fat, is associated with a decrease in Bacteroides and an increase in Firmicutes and Proteobacteria relative abundance (34, 83–85). Conversely, mono- and polyunsaturated fat present in low levels in vegan/vegetarian diets increase the levels of lactic acid bacteria, Bifidobacteria, and Akkermansia muciniphila (A. muciniphila) (30, 31). In mice, both lard-based and palm-oil based HFDs increased the relative abundance of the Clostridiales and Bacteroidales classes in specific pathogen free (SPF) mice (86). However, no significant differences in microbiota composition were observed between both diets that mainly differ in their cholesterol content, where a lard-based diet contains 10 times more cholesterol than the palm-oil based HFD (86).
Agans et al. demonstrated in an in vitro multi-vessel analysis that distinct gut microbiota can utilize dietary fatty acids as a sole carbon source through β-oxidation and anaerobic respiration pathways (87). Thus, bacteria that possess fatty acid oxidation enzymes, for example Alistipes spp. and members of the Proteobacteria phylum (Bilophila, Escherichia/Shigella, Citrobacter, and Enterobacter spp.) were enriched in a medium containing only capric acid, palmitic acid, stearic acid, oleic acid, and linoleic acid (87). Interestingly, however, in the small intestine, where most of the macronutrient digestion and absorption occurs, the intestinal microbiota was also shown to be capable of regulating host dietary fat digestion and absorption in mice (88). In that study, consumption of a HFD increased the relative abundance of the Clostridiaceae family at the mucosa, most markedly in the jejunum and ileum, and one member of this family was shown to secrete an unknown metabolite capable of mediating lipid absorption (88). Thus, the mucosa-associated microbiota can be highly sensitive to dietary lipid changes and can play an important role in nutrient absorption.
Proteins
In addition to fiber fermentation, protein metabolism by bacteria can produce a small fraction of SCFAs too, but also more detrimental metabolites originating from animal diets (eggs, beef, pork). For example, the food-derived microbial metabolite trimethylamine N-oxide (TMAO) is linked to cardiovascular disease and atherosclerosis (89, 90). Moreover, a diet rich in animal protein is associated with a decrease in members of Firmicutes phylum that are known to metabolize plant polysaccharides (e.g., Roseburia, Eubacterium rectale, and Ruminococcus bromii) and with an increase in the levels of bile-tolerant bacteria (Alistipes, Bilophila, and Bacteroides) (67, 91). However, individuals consuming pea protein—a plant-based alternative for meat—displayed increased intestinal SCFA levels and a bloom in beneficial Bifidobacterium and Lactobacillus, while pathogenic Bacteroides fragilis and Clostridium perfringens levels were reduced (31). Furthermore, observations in protein supplementation studies showed an increase in the total amount of bacteria, as determined by absolute-abundance (92). This was proposed to be linked to the increased availability of nitrogen, an otherwise limited nutrient in the gut, as a result of the higher protein intake (92).
Other: Micronutrients and Food Additives
Besides the discussed macronutrients, micronutrients are increasingly acknowledged to influence the gut microbiota. Some of these compounds transit the small intestine, where a large number of digestible nutrients are already absorbed, and reach the colon intact, where they concentrate and interact with the microbiota (66, 93). Examples of micronutrients include polyphenols—naturally occurring plant metabolites—(e.g., lignans, isoflavones, stilbenes), trace elements and vitamins. Polyphenols in plant-based diets are generally considered to have a prebiotic effect, i.e., supporting the growth of beneficial bacteria such as Bifidobacterium and Lactobacillus (94–96), can be antimicrobial against different bacterial pathogens and can have anti-inflammatory effects (31, 97). Trace-elements, such as iron and zinc, have a low abundance in the gut and are thus competed for amongst pathogens and commensals, thereby also affecting gut microbial composition and/or favoring pathogen colonization (98–100). Other micronutrients such as vitamins B6 and B12 serve as cofactors for microbial enzymes and consequently, gut microbial species compete with the host for these diet-derived vitamins in the small intestine (101).
Remarkably, food additives that are often present in modern diets (e.g., non-caloric artificial sweeteners (NAS) such as sucralose, saccharin and aspartame) and emulsifiers [e.g., carboxymethyl cellulose (CMC) and polysorbate-80 (P80)], induce significant dysbiosis. The microbiota of NAS-consuming mice provoked an overgrowth of Bacteroides spp. and reduced levels of A. muciniphila (39). Emulsifier-treated mice had a reduction in the Bacteroidales population and an increase of the mucolytic bacterium Ruminococcus gnavus, which was accompanied by decreased SCFA production and the development of metabolic syndrome (40).
In summary, individual macronutrients and micronutrients can have distinct effects on gut microbiota composition, which in turn can have subsequent effects on human health. However, caution is prompted when linking a phylum to a specific diet, given the dynamic nature of the microbiome and due to the challenging task of disentangling which dietary effector in the complex composition of the diet is driving the observed changes (66). For example, the changes in gut microbial composition observed in HFDs could be biased by the low dietary fiber content and may not be a direct consequence of the fat content or composition. Indeed, Morrison et al. showed that switching from a regular chow diet to a refined low-fat/low soluble fiber diet was accountable for the change in the fecal community structure in mice (102). In contrast, a switch from the low-fat/low soluble fiber diet to a low soluble fiber/HFD kept the initial observed changes without further alteration. Remarkably, the authors observed expansion of Clostridia and Proteobacteria and a reduction of Bacteroidetes when switching from a chow diet to a diet low in fat and lacking soluble fibers; these alterations are typical of HFD interventions (102).
Intestinal HDPs as Key Effectors of Mucosal Barrier Function
Besides the nutrients derived from the ingested food, the host also plays an active role in shaping the gut microbial community. While the production of IgA and mucus as modulators of gut microbiota composition have been discussed elsewhere (103, 104), we will here focus on the release of HDPs, which due to their positive charge are retained in the intestinal mucus layer (105–107). Intestinal HDPs are a diverse group of proteins that possess unique mechanisms of action and spectrum of activity against microbes. In part, these mechanisms depend on the HDP localization in the intestine (Figure 3) and the specific regulatory mechanisms of expression and activation (12).
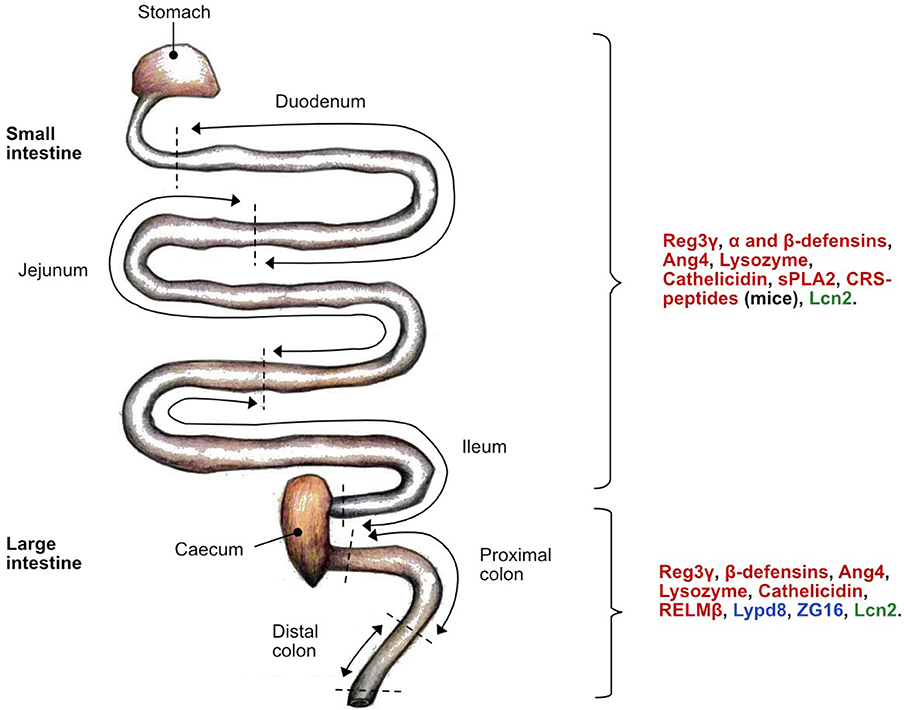
Figure 3. Location of host defense peptide (HDP) expression along the mice intestine under normal physiological conditions. The antimicrobial activity is specific to each HDP class: bactericidal (red), bacteriostatic (green) and aggregation (blue). α-defensins can also be referred to as Defas in mice. C-type lectin regenerating islet-derived protein 3 gamma (Reg3γ); Angiogenin 4 (Ang4); secretory phospholipase A2 group IIA (sPLA2); cryptdin-related sequence peptides (CRS-peptide)s; Lipocalin-2 (Lcn2); Ly6/PLAUR domain containing 8 protein (Lypd8); zymogen granule protein 16 (ZG16); resistin-like molecule beta (RELMβ).
Location
In the intestine, different epithelial cell subsets produce distinctive HDPs. Enterocytes produce the regenerating islet-derived protein 3-gamma (Reg3γ) and β-defensins in both the small and large intestine, and the Ly6/PLAUR domain containing 8 protein (Lypd8) exclusively in the large intestine (108). While the primary function of goblet cells relies on the production and secretion of MUC2, they also secrete resistin-like molecule beta (RELMβ), an HDP that is active predominantly in the colon (109, 110). However, the vast majority of HDPs are produced by small-intestinal Paneth cells and include lysozyme, α- and β-defensins (α-defensins are alternatively called cryptdins in mice), angiogenin-4 (Ang4), secretory phospholipase A2 group IIA (sPLA2), and Reg3γ. Although mature Paneth cell HDPs have been isolated from the large intestine, they probably also originate from small-intestinal Paneth cells (111).
Immune cells also contribute to the HDP repertoire with secretion of lipocalin-2 (Lcn2) by neutrophils and lysozyme by macrophages (112). Also, neutrophils produce human neutrophil peptides (HNPs), a class of α-defensins, which are only produced by humans and not by mice (113). To compensate for this, however, mice seemingly evolved and acquired an additional set of peptides closely related to α-defensins, called cryptdin-related sequence (CRS) peptides, that are also produced by Paneth cells (114).
Antimicrobial Activity of HDPs
Defensins possess a broad spectrum of antimicrobial activity, as in vitro studies demonstrated that they are bactericidal against Gram-positive and Gram-negative bacteria, fungi, viruses, and unicellular parasites (115). These small secreted peptides (30–40 amino acids long, 3 to 5 kDa) are characterized by six conserved cysteine residues that form three disulfide bridges (116). The in-sequence linkage of the cysteines distinguishes α- and β-defensins, the two largest defensin families (113). The cationic nature of these peptides advantageously attracts them to the negatively charged outer envelope of bacteria, produced by the presence of phospholipids in Gram-negatives and of teichoic acid in Gram-positives. In its majority, defensins are thought to act by disrupting the bacterial membrane integrity or via inhibition of the cell wall synthesis by interacting with lipid II (112, 113, 117). Interestingly, due to this cationic property, HDPs have recently been attributed different tumor killing capabilities, as the membrane of tumor cells have increased expression of negatively charged cell surface glycoproteins (118).
Mice possess a wide array of α-defensin and CRS-peptides in the intestine, both with many gene paralogs and high sequence similarity between different laboratory mouse strains, which complicates research on these molecules (119, 120). In addition, expression levels of mice defensins vary along the small intestine (23). α-defensins, also called cryptdins in mice, exhibit a variable spectrum of activity depending on their oxidation status (discussed below); for example displayed reduced α-defensin 4 a greater antimicrobial activity against 7 different commensal bacteria than the oxidized form (15). Furthermore, the antimicrobial activity spectrum of CRS-peptides relies on their characteristic ability to form covalent disulfide-bridged homo- and heterodimers, conferring different killing capabilities against commensal Enterococcus faecalis (E. faecalis) and Lactobacillus fermentum and the pathogens Streptococcus pyogenes, Listeria monocytogenes, Escherichia coli (E. coli) and Salmonella typhimurium (S. typhimurium) (114).
In humans, the most abundant intestinal HDPs are HD5 and HD6 (121). The potent antimicrobial activity of HD5 was shown against Staphylococcus aureus (S. aureus) and E. coli, which was comparable to the activity of HNP2 and HNP4, respectively (122). HD6 was shown to self-assemble and acquire a nanonet formation, capable of entrapping bacteria (123), and of disrupting the cell envelope of bacteria in a reducing environment (19). Other intestinal HDPs in humans include the β-defensins HBD1, HBD2, and HBD3. Interestingly, HBD1 seems to be constitutively expressed whereas HBD2 and HBD3 can be induced by microbial products (116, 124). HBD1 and HBD3 were found in human rectal-mucus extracts and their activity was not affected by binding to mucus (107). HBD3 possesses a broad spectrum of activity against facultative anaerobic commensal and pathogenic bacteria (19). Of note, HBD1 can exist as an oxidized, disulfide-bridged form and a reduced, linear peptide, which differ in their antimicrobial activity spectrum: reduced HBD1 exhibits a higher antimicrobial effect against the opportunistic pathogen Candida albicans (C. albicans) and different commensal Bifidobacterium and Lactobacillus strains when compared to its oxidized form (18). Recently, it was shown that even an HBD1-derived octapeptide fragment that was generated through digestion by gastrointestinal proteases caused cell wall and membrane defects as well as the disintegration of cytosolic structures of E. coli and C. albicans (125).
Further HDP classes include Reg proteins, angiogenins, and Lcn2. Reg3γ in mice (or the human homolog Reg3A, previously known as human HIP/PAP), present antimicrobial activity against Gram-positive bacteria via formation of an hexameric pore structure in the bacterial membranes (126). Interestingly, angiogenins have been attributed to different functions, including tumorigenesis, cell growth, and apoptosis, and Ang4 was shown to be bactericidal against Gram-positive E. faecalis and L. monocytogenes through a yet unknown mechanism of action (127, 128). Lcn2 is mainly secreted by neutrophils and prevents bacterial growth by sequestering iron-scavenging siderophores (129). Additionally, in the absence of Lcn2, mice were more susceptible to bacterial colonization, not only owing to the reduction of the bactericidal effect but also by altering the migration of neutrophils and reducing the expression of cytokines by macrophages (130).
ZG16, Lypd8, and RELMβ have been described as peptides that maintain the spatial segregation between the gut microbiota and the hosts' epithelial cell surface in the colonic mucus layer (108, 110, 131). ZG16 is a highly abundant protein in the colon that intertwines with the mucin polymeric network and contributes to space separation by binding to Gram-positive bacteria (131). Lypd8, a highly glycosylated glycosylphosphatidylinositol-anchored protein, binds flagellated bacteria and was recently shown to inhibit the attachment of Citrobacter rodentium to epithelial cells through competitive binding of bacterial intimin, thereby interrupting its interaction with the translocated intimin receptor (Tir) in the host (108, 132). Lypd8 thus specializes in inhibiting the colonization of intestinal pathogens. RELMβ controls the levels of Gram-negative Proteobacteria in the inner colonic mucus layer of mice by forming pores into the bacterial cell membrane (110). Of note, the human homolog (hRETN) was shown to be specifically bactericidal against Gram-negative pathogens but lacked activity against commensal bacteria such as E. faecalis and B. thetaiotaomicron (110). Additionally, RELMβ is inducible by the microbiota and was shown to be elevated during intestinal inflammation and potentially also aging, as observed in 104 weeks old mice (133, 134).
Altogether, there is reason to believe that HDPs in the small intestine are mainly aiming to “kill” bacteria, whereas those in the large intestine have a more spatial segregation function and are not necessarily bactericidal.
Transcriptional Regulation of HDPs
Key to the antimicrobial effect of HDPs are the regulatory cues behind their function, which can occur at the level of granule-release, expression, and activation. For example, defensin exocytosis from Paneth cell granules is mediated by the ATG16L1 and ATG5 proteins of the autophagy pathway (135), and can also occur in response to bacterial stimuli (21), which will be discussed later. In the crypts of the small intestine, stem cells differentiate into Paneth cells via the Wnt/β-catenin signaling pathway under the activity of the transcription factor TCF-4 (20, 136). Studies performed in mice show that the Paneth cell maturation process is accompanied by the appearance of α-defensins and CRS-peptides during the weaning period (23, 137). Moreover, TCF-4 can control the expression of α-defensins and CRS-peptides in mice and humans—as the promotor region of these genes has binding sites for TCF-4, suggesting their baseline release levels occur in parallel to Paneth cell differentiation (20, 119). Recently, the pro-inflammatory cytokine interferon gamma (IFN-γ) was also identified as a potent inducer of Paneth cell degranulation and goblet cell mucus production in a model of murine primary organoid culture (138).
Although several studies report that Paneth cells and intestinal epithelial cells can directly respond to bacterial stimuli, immune cell-derived signals are the main effectors triggering HDP expression (138). Upon recognition of microbe-associated molecular patterns (MAMPs) by Toll-like receptors (TLRs) present in innate lymphoid cells (ILCs) and dendritic cells, downstream signaling orchestrates an inflammatory response and integrates different signals oriented to control the expression of different HDPs. Activation of the NLRP6 inflammasome signaling by the microbiota—as shown in GF vs. SPF mice—directed the release of IL-18, which induced the HDPs intelectin 1a (ITLN1), RELMβ, and Ang4, implicating IL-18 as a regulator of the antimicrobial program of the colonic mucosa (27). Likewise, IL-25, a Th2 cytokine mainly known for its anti-helminth function, was shown to also induce the expression of Ang4 in an IL-23 dependent manner (139). Furthermore, IL-22 has been demonstrated to induce Reg3β, Reg3γ, β-defensins, Lcn2, and Ang4 (12, 12, 140–144), as well as mucin production (145). The cellular sources of IL-22 include type 3 innate lymphoid (ILC3), natural killer (NK), Th17 and Th22 cells as well as dendritic cells (146–148).
Whereas, Reg3γ is induced by the microbiota-dependent inflammatory signals of the TLR-MyD88-IL-22 axis (24, 120, 143), evidence for a role of MyD88 in α-defensin regulation is conflicting. Castillo et al. reported that MyD88 is not involved in defensin regulation, as the total defensin copy number in the small intestine of Myd88−/− mice, was not different from the Myd88+/+ control group (120). Conversely, Menendez et al. showed a drop in ileal defensin (Defa) expression in MyD88−/− mice, using a relative expression approach (149). Likewise, Liang et al. showed that MyD88−/− mice had reduced gene expression of IL-22, and of Mmp7, the coding gene for the matrix metalloproteinase-7 (Mmp7) which is a key enzyme that is required to activate mouse α-defensins (discussed below), and diminished mature α-defensins under normal conditions (150). These last observations suggest that MyD88 regulates Mmp7 expression, and therefore the post-transcriptional activation of α-defensins (150). Thus, MyD88 may not regulate transcription of Defa genes directly, but affect α-defensins rather indirectly through regulation of Mmp7. However, this explanation does not provide reasoning to the transcriptional downregulation of Defa observed by Menendez et al. (149). Finally, most recently the TIR domain-containing adaptor molecule 1 (TRIF or TICAM1) was described as a key homeostatic regulator of epithelial barrier function by controlling the expression and protein levels of Mmp7, Reg3γ, and Defa1 (151). In this study, MyD88−/− mice again had no influence on Defa1 expression and showed only a slight reduction in Mmp7 expression (151).
Post-transcriptional Regulation of HDPs
On the activation level, environmental conditions and the presence of different proteases can determine the activity of defensins. HDPs with membrane-lysing capacities can be toxic to eukaryotic cells. Because of this, defensins are secreted as pro-peptides that are processed and activated in the gut lumen by Mmp7, also known as matrilysin, in mice and by trypsin in humans (152, 153). Cutting off the pro-region will activate their antimicrobial function, and once in its mature form, some peptides can resist proteolysis, as was shown for α-defensin 4 (111). In the gut, HDPs can be further processed by other proteases such as gelatinase (GelE) or serine protease E (SprE), secreted by E. faecalis, or chymotrypsin and neutrophil elastase, produced by the host (111).
Another form of regulation of HDP activity relies on the local microenvironment in the intestine. Defensins form disulfide bridges that can modulate the antimicrobial effect, depending on whether they are in their reduced (linear/open) or oxidized (closed) forms, as exemplified for α-defensin 4 (15). Redox-potential and pH differ between the bottom of the intestinal crypts (where most HDPs are secreted) and the gut lumen and can thus shape the tertiary structure of α-defensins. Furthermore, the enzymatic thioredoxin system in the gut is a host-dependent mechanism to control redox reactions, and this system mediated the reduction of HBD1, thereby revealing its potent antimicrobial activity against common anaerobic gut bacteria (18). Of note, antimicrobial activity of α- and β-defensins was differently modulated when conditions were adjusted for pH and redox-potential, and was independent of bacterial genus, cell wall composition, or defensin class (19). In this manner, a reducing environment exposed a bactericidal effect of HD6, while the nanonet conformation was maintained under these conditions (19). Importantly, in the gut the reduced or oxidized conformations will be subject to protease activity to either activate or deactivate them (125, 154). While oxidized HBD1 and HD5 were resistant to protein digestion, HBD1red was readily digested in vitro and generated a C-terminal octapeptide (125). This octapeptide gradually lost its activity in acidic conditions, further highlighting the influence of environmental regulation on HDP activity (125). Similarly, HD5red was efficiently degraded by host proteases and produced ten new fragments, some of which exhibited an antimicrobial effect against commensal bacteria, and thus greatly increased the known spectrum of activity of this peptide (154). In contrast, HD6red was unaffected by host proteases, mainly due to its characteristic nanonet formation (154).
In summary, the antimicrobial activity of intestinal HDPs is controlled by a complex interplay between transcriptional and post-transcriptional signals that are central to maintaining homeostasis in the gut (12). Some of these regulatory factors are under the influence of the host, but other factors tightly depend on the presence and the composition of the gut microbiota. While HDPs have historically been considered to protect the host against pathogens, many studies preferentially included pathogenic bacteria and fungi in their antibiotic activity tests. It is thus possible that the activity against anaerobic, commensal bacteria is underestimated, due to a study bias and due to the selection of simple testing conditions that did not resemble the conditions in the gut. In fact, only by modulating some of the environmental parameters in the activity tests, the antimicrobial effect of several HDPs against commensal bacteria could be revealed (15, 18, 19, 154). It is thus required to keep these factors in mind in order to increase our understanding of the function of these peptides in shaping microbial communities in the gut.
Impact of HDPs on Intestinal Microbiota
Intestinal HDPs protect the host against microbial intruders in the gut and have the potential to shape the intestinal microbiota (155) (Table 1). Specifically, the HDP family of defensins has been shown to exert noticeable effects on gut microbiota composition. Transgenic mice expressing HD5 on top of their indigenous HDP repertoire were shown to have an expansion of the Bacteroidetes and a reduction of the Firmicutes phyla in the small intestine (9). Interestingly, a fragment produced after proteolysis of HD5 (HD51−9), shifted the fecal microbiota composition and influenced the microbial diversity in the small intestine, specifically increasing Akkermansia and a member of the Ruminococcaceae family and decreasing Intestimonas from the Clostridiaceae family (154). Furthermore, administration of HD5 in a murine model of diet-induced obesity reversed dyslipidemia and improved the overall glucose regulation (17). The latter was partly attributed to HD5-induced changes in the fecal microbiota, namely an increase in Bifidobacterium and Alloprevotella abundance, that correlated with improved metabolic parameters (17). However, the effect on microbiota composition in the small intestine was less marked. Thus, both the transgenic expression and administration of defensins can shape the microbial communities in the mouse intestine.
The impact of mice defensins on gut microbial communities was also studied in mice lacking Mmp7 (152). Mmp7−/− mice have an increase in the levels of Firmicutes (mainly Clostridia) and a significantly lower proportion and abundance of Bacteroides in the small intestinal microbiota, which is contrary to the result observed in mice expressing HD5 (9). As both the HD5 transgenic and the Mmp7−/− mice models showed no effect on the total bacterial numbers, the defensin function seems likely restricted to shaping the composition of the microbiota rather than controlling its abundance in the small intestinal lumen. Interestingly, and in contrast to the small intestine, the caecal and colonic microbiota of Mmp7−/− mice were not different from their wild-type controls (111). These findings were attributed to the existence of other host and microbial proteases present in the large intestine that could convert inactive defensin precursors into active peptides, thereby explaining the lack of an effect on the microbiota composition at this intestinal site (111).
However, as discussed above, the overall impact of HDPs on gut microbiota community structure is expected to be reflected by the potential antimicrobial effect against gut commensals. And indeed, administration of HD5 increased Bifidobacterium relative abundance (17), while transgenic expression of HD5 on top of the own mouse antimicrobial arsenal led to increased relative abundance of Bacteroidetes (9). This suggests that defensins can promote the growth of selected microbial taxa by specifically eliminating other microbes. While some HDPs, such as hRETN, human LL37 or mouse cathelicidin related antimicrobial peptide (CRAMP) show no antimicrobial effect against commensal microbes (16, 110), defensins have anti-commensal activity that was dependent on an environment that resembled the conditions in the gut (15, 18, 19). Regardless of this, most in vitro studies have investigated the antimicrobial effect against isolated pathogens and not against complex communities of commensals. Accordingly, these findings have contributed to build up the notion that HDPs do not affect commensals. Therefore, more work is required to determine the effect of several HDP classes with adjusted microenvironmental conditions and to truly understand the impact of HDPs on the gut microbiota community.
Altered HDP Expression in Microbiota-Associated Diseases
An indication that HDP modulation of gut microbiota composition might also be true in humans is based on the fact that gut-associated inflammatory disorders are commonly accompanied by dysbiotic communities, and that ileal Crohn's disease, a form of IBD, can be linked to Paneth cell dysfunction (156–158). Accordingly, in two German cohorts patients with ileal Crohn's disease had reduced levels of HD5 and HD6, which was even more pronounced in patients carrying a mutation in the intracellular nucleotide binding oligomerization domain 2 (NOD2) receptor (43, 44). However, in another cohort in Australia, reduced levels of HD5 were associated with inflammation and not with the NOD2 genetic status (45). Yet, despite that Paneth cells are the predominant cell types expressing NOD2 at the intestinal mucosa, it is unclear whether mutations in NOD2 are indeed causing the altered expression of the human defensins in ileal Crohn's disease (159).
In addition to NOD2, other genetic risk factors that may affect Paneth cell function in Crohn's disease include mutations in ATG16L1 (160), which is part of the autophagy pathway and implicated in Paneth cell degranulation, or in the transcription factor X-box binding protein-1 (Xbp1), a key regulator of the endoplasmic reticulum (ER) stress response (161). Mutations in Xbp1 can result in ER stress, defects in Paneth cell granule morphology and reduced lysozyme levels, leading to intestinal inflammation (162).
Besides patients with ileal Crohn's disease, patients with obesity (BMI>35) evidenced defective Paneth cell secretions, which was linked to the activation of the unfolded protein response (UPR) during ER stress (163). Even though the morphology and number of Paneth cells were normal, these individuals had reduced HD5 and lysozyme protein levels, despite having an increased gene expression of these HDPs, suggesting the presence of transcriptional arrest (163). Similarly in mice, an obesogenic diet was associated with Paneth- and goblet cell abnormalities, a worsened colonic inflammation and expansion of Atopobium spp. and Proteobacteria in the fecal microbiota (164). Taken together, a defective antimicrobial defense that cannot sufficiently control microbial communities in the gut may likely be a contributing factor in the pathogenesis of ileal Crohn's disease and obesity. However, it is still not fully clear if defects in defensin expression or secretion precede or follow the onset of disease.
Direct Effect of Diet on HDP Expression
We have described the implications of different dietary compounds on the microbial communities in the gut. To add to this function, evidence of a direct effect of diet (i.e., the presence of certain dietary components or the impact of complex diets) and nutritional status (i.e., fasting or starvation condition) on HDP function, has been accumulating over the past decade. Takakuwa et al. tested 20 amino acids in mice-derived enteroids to investigate their defensin-inducing capacity in vitro. The release of Defa1 was strongly induced by leucine, and to a lesser extent, by tryptophan (165). Their observations thus suggest a direct role of distinct amino acids in the induction of defensin expression by intestinal cells. Another study explored how diets supplemented with kidney bean flour, which is rich in fiber and phenolic compounds, influenced colonic barrier function in a mouse model of colitis. When compared with a basal control diet, the dietary flour intervention increased SCFAs (acetate, butyrate, and propionate) and up-regulated MUC1 and RELMβ in unchallenged mice (166). This effect was more pronounced after the induction of colitis and at the same time, the bean flour treatment decreased the expression of pro-inflammatory cytokines and improved colitis symptoms (166). Moreover, Bentley-Hewitt et al. demonstrated that in vitro fermented kiwifruit products significantly increased the production of HBD1 and HBD2 by intestinal epithelial cells. This effect, which was mainly mediated by the production of SCFAs and was not observed after treatment with the digested kiwifruit lacking fermentation products, suggests that the HDP modulating effect was exerted by fermentation products and not directly by the digested kiwifruit (167).
Food Availability
In addition to individual components of the diet (i.e., food quality), food availability (i.e., food quantity) has also been linked to HDP function. Mice deprived of food for 48 h had reduced expression of the Paneth cell antimicrobial peptides lysozyme, defensins, and Reg3γ, which was confirmed on the protein level for the precursor of Reg3γ and lysozyme (168). Although the numbers of Paneth cells were not changed, the physiology of their granules was altered. Furthermore, a 2-fold increase in bacterial translocation to the mesenteric lymph nodes was observed in the starved mice (168). Interestingly, the same authors showed opposite results in a second study, in which they evaluated the impact of total parenteral nutrition on Paneth cell function and regulation of intestinal homeostasis in rats. Here, the absence of enteric food caused an up-regulation of lysozyme and rat α-defensins 5 and 8 (169). Remarkably, the authors noted an inverse correlation between lysozyme expression and Firmicutes abundance in the small intestine, implying a link between HDP function and the small intestinal microbiota (169). Regarding the contrasting results, the authors speculated that the main difference between both studies was that the rats fed with parenteral nutrition could still respond to changes in the microbiota, as the Paneth cells were still functional, as opposed to the altered granule physiology observed in starved mice (169). Indeed, Liang et al. expanded these observations in a longer mouse starvation study of 72 h and identified that the starved group showed a drop in Reg3β, Reg3γ and Mmp7 gene expression after the first 48 h, and later an increased expression of Mmp7 at 72 h (150). The authors investigated the V-shaped pattern of expression of Mmp7 and were able to demonstrate that an initial drop in the microbial population caused the decreased expression 48 h post-starvation through Myd88-IL-22 signaling, and that the recovered expression 72 h post-starvation was regulated by the transcriptional repressor Hes1, controlled by the mTOR nutrient-sensing transcriptional regulator in response to the nutrient fluctuations (150). They additionally demonstrate that mTOR controlled Hes1 translation by sensing amino acids and glucose (150). Recently, intestinal neurons secreting vasoactive intestinal peptide (VIP) in response to food consumption were also implicated in the ILC3-mediated regulation of Reg3γ expression (170). Although the molecular food-sensing mechanism causing a VIPergic response remains unknown, this study evidences a link between neural-immune regulation of HDP expression and food intake.
Taken together, these studies suggest that microbiota as well as nutritional status play a role in HDP-modulation. Accordingly, these observations highlight the importance of the presence of food for the correct functioning of the antimicrobial program in the small intestine, as starvation has been associated with increased risk of bacterial translocation in patients receiving parenteral nutrition (171).
The Effect of High Fat Diet (HFD) on HDP Expression
HFD is a general term for a diet with increased fat content. However, the amount and composition of fat content, as well as other dietary compounds can vary between studies, as can the length of the intervention. Such variations have the potential to change HDP expression pattern in the small intestine to varying degrees. Table 2 summarizes various mice and rat studies that investigated how different HFD treatments affect the expression of selected HDPs. Diets with 20–60% fat content substantially modify HDP expression patterns in the small intestine, whereas short term treatments (between 2 and 20 weeks) lowered HDP expression, which was in some cases also confirmed on protein levels. At the same time, longer treatments (>20 weeks) seemed to shift this pattern toward higher HDP expression levels.
HFDs, which also include the high fat/high carbohydrate WSDs, contain components that can directly (e.g., cholesterol or saturated fatty acids) or indirectly (e.g., TMAO) trigger inflammation (37). Specifically, saturated fatty acids present in WSDs, such as palmitic or stearic acid, induce ER stress, an insult that is sensed by macrophages (180). Also, a recent study demonstrated that HFD feeding caused a 50% increase in Lgr5+ intestinal stem cell numbers but at the same time a 23% reduction in Paneth cell numbers (181). Thus, we hypothesize that the initial reduction in HDP expression upon HFD feeding can be caused by the reduced numbers of Paneth cells (Table 2) (176, 179), and in addition by further detrimental effects, such as ER stress, which was previously linked to obesity and HDP malfunction (163). After prolonged treatment—for example, 5-month HFD intervention—a chronic inflammatory response is initiated in the gut that is reflected by the increased HDP expression.
Different pathways were suggested to control the dietary regulation of HDP expression. Tomas et al. focused on the pathological effects of a HFD in the small intestine, a region with key nutritional functions, and which seemed to have been understudied in the context of obesity, diabetes and metabolic syndrome (Table 2) (172). Among different detrimental effects, the authors identified that HFD treatment led to a downregulation of HDPs and the cystic fibrosis transmembrane conductance regulator (Cftr), as well as the peroxisome proliferator-activated receptor (Ppar)-γ, a nuclear receptor involved in lipid sensing and mucosal defense regulation (172, 182, 183). In addition, mice fed the HFD had increased numbers of bacteria in the intervillous zone in the small intestine—an otherwise sterile area. This aberrant colonization of bacteria in the villi was associated with the defective antimicrobial response in the ileum, and indeed, the administration of rosiglitazone, a Ppar-γ agonist, restored HDP expression levels; thus revealing the role of this receptor in controlling the antimicrobial function in the intestine (172).
While research on high-fat diets naturally focuses on the role of fat, these dietary compounds can also interact with other dietary factors. Su et al. demonstrated that both dietary fat and vitamin D can modulate Defa and Mmp7 expression in mice. Vitamin D is a dietary component that supports mucosal barrier function when supplied in adequate amounts (184, 185). HFD treatment reduced the expression of Defa5, Defa1, Defb1, and the protein levels of Defa1 and Mmp7, and the reduction in HDP expression was even stronger in mice fed a diet low in vitamin D (Table 2) (173). Remarkably, the combination of a high-fat and vitamin D-deficient diet (called “the double hit model”) caused hepatic steatosis and insulin resistance. This effect was attributed to a state of dysbiosis in the small intestine, as a consequence of the low antimicrobial response. Accordingly, this hypothesis was confirmed by the administration of synthetic HD5 in the double hit model, which corrected the expression of HDPs, resolved the systemic inflammation and improved the observed insulin resistance (173).
Importantly, dietary supplements in the context of HFDs revealed additional factors with the potential of modulating mucosal barrier function. These factors included the polyphenol rutin and the prebiotics inulin and oligofructose (Table 2) (175, 178). While a 20 week HFD treatment elevated the expression of HDPs, both the administration of rutin or rutin and inulin reduced their transcription back to base expression levels (178). In addition, the administration of oligofructose significantly increased the expression level of Reg3γ (>50-fold), which was otherwise reduced by a HFD (175). These examples implicate polyphenols and prebiotics as keepers of intestinal homeostasis by correcting the aberrant expression of HDPs. As prebiotic interventions will promote the growth of beneficial bacteria, their HDP-modulatory effect might operate via modulation of microbial communities, which will be discussed in detail below.
Finally, a more indirect effect of HFD on HDP expression has been described recently. Upon consumption of a (high-fat) meal the host secretes bile acids to emulsify the fatty acids and facilitate their absorption. To test the effect of bile acids in mice, dietary supplementation with the primary bile-acid chenodeoxycholic acid (CDCA) was shown to induce the transcript levels of Defa20, Reg3β, and Reg3γ, and stimulate the production of Reg3β, and Reg3γ in different cell types along the villi in the ileum (186). While the effect on the microbiota was minimal and only increased relative abundance of Bacteroidetes, the increase in antimicrobial defenses protected the host from enteric Salmonella and Citrobacter infection, two microbes that are otherwise bile-resistant (186). Although the mechanism behind the induction could not be identified, this implicates bile acids as indirect effector molecules from the host that can regulate HDP expression and microbiota composition.
Altogether, food availability and specific dietary factors have the potential to significantly influence the intestinal antimicrobial defense system, an effect that is only starting to be understood. While most studies observed HDPs at the expression level, protein levels are expected to reveal more information about this function. Also, most of these studies were performed in mice and the effect of these factors in human trials remains to be demonstrated.
Effect of Microbes and Their Metabolites on HDP Expression
Dietary factors elicit different responses that control microbial communities in the gut. Nevertheless, both the presence or absence of the microbiota as well as specific individual microbes have been linked to modulation of HDP expression. It is well-known that germ-free (GF) mice, which are completely devoid of a microbiota, undergo an incomplete development of the immune system (187, 188). As such, early studies of GF mice intestines revealed a decrease in expression of Reg3γ and CRS4C, as determined by total transcript copy number, when compared to conventional mice (23). Likewise, conventional mice had higher expression of Reg3γ, Reg3β, RELMβ, and CRS4C4 when compared to GF mice or to mice in which the microbiota had been depleted by an aggressive antibiotic regime (14). Similarly, observations in antibiotic depleted mice further corroborated the decreased expression of Mmp7 and Reg3β in the ileum and of Ang4, Pla2g2a, RELMβ, Reg3γ, and Reg3β in the colon (150, 189). Altogether, these observations seem to be a result of immature immune development in the absence of HDP-stimulating microbes.
Microbes That Stimulate HDP Expression
Different studies have linked the enrichment of a particular microbe or the administration of a probiotic bacterium to increased expression of HDPs. For example, B. thetaiotaomicron has been shown to stimulate the production of Reg3γ and Ang4 in the small intestine and CRAMP in the colon (24, 127, 190). Moreover, B. thetaiotaomicron mediated the colonization resistance against C. albicans via induction of HIF-1α (190), a transcription factor involved in the activation of innate immune effectors that also regulates CRAMP in the murine gut and of HBD1 in humans (191, 192). Interestingly, this microbe has been described to be resistant to HDPs and could therefore survive the antimicrobial stimulation (16, 24). Similar to B. thetaiotaomicron, supplementation with A. muciniphila increased the expression of Reg3γ in the mouse colon when mice were fed a control diet, but not during treatment with a HFD, in which ileal Pla2g2a, Defa, and Lyz1 were not stimulated by A. muciniphila (193). Also, monocolonization of mice with the probiotic Bifidobacterium breve NCC2950 induced in vivo and in vitro expression of Reg3γ, an effect that was mediated by the MyD88-Ticam axis of the TLR signaling pathway (194). Furthermore, Cazorla et al. isolated intestinal fluid from mice treated with the probiotic strains L. casei CRL431 and L. paracasei CNCM-I and showed that the extracted fluid had enhanced antimicrobial activity against S. typhimurium and S. aureus (195). Although this study did not measure HDP expression, the probiotic treatment increased the Paneth cell numbers in the crypts, hence suggesting an overall increase in the antimicrobial peptide function in the gut (195). In the same line, the probiotic strain E. coli Nissle 1917 evidenced a strong induction of HBD2 in in vitro studies (196), an effect that could be confirmed in vivo in a small human study, in which administration of an E. coli probiotic preparation (Symbioflor® 2) for 2–3 weeks increased the levels of this peptide in the feces (25). While the in vitro HBD2-inducing effect was caused by flagellin of E. coli Nissle 1917 (197), the E.coli strains in the probiotic preparation did not produce flagellin, thus suggesting that multiple mechanisms can mediate this effect and that they differ between individual strains (25).
Microbial-Derived Components and Molecules Implicated in HDP Control
Specific microbial-derived molecules and metabolites have been associated with regulation of HDP function by stimulating their expression or by promoting their release. Early studies demonstrated that Paneth cells can respond directly to bacterial stimuli, either live or dead bacteria, lipopolysaccharide (LPS) or membrane components (21). This observation was performed in isolated crypt cells and shed light on defensin secretion capabilities. However, since a mucus layer covers the small intestinal epithelium, the probability of microbial derivates reaching the bottom of the crypts is expected to be low. Yet, the described Paneth cell response is likely a back-up response that could take place in the presence of severe mucosal damage or during abnormal microbial growth at this site. Still, isolated crypts may contain exposed basolateral receptors and are perhaps more responsive to bacterial stimuli. Accordingly, using mouse-derived small intestinal organoids, Farin et al. showed that Paneth cell degranulation did not occur after stimulation with bacterial agents, but rather after direct induction with IFN-γ or a supernatant derived from stimulated iNKT cull culture (138). Similarly, the SCFA butyrate was shown to directly enhance the production of Defa1 in isolated crypts from the small intestine (165), but it is unclear whether Paneth cells could be stimulated by this fermentation product in the small intestine in vivo, where the concentration of butyrate is relatively low (165, 198). Furthermore, by using a human-derived reporter cancer cell line, Sugi et al. investigated the transcription of Defa5 after challenge with the bacterial ligands LPS, the synthetic lipopeptide P3CSK4 or with the bacterial metabolites acetate, lactate, butyrate, and propionate. Among these molecules, lactate strongly suppressed the transcription of Defa5 while propionate and butyrate were suppressive only at a high concentration (9 mM) (198). However, this intestinal cell line represents absorptive epithelial cells, and their defensin expression capacities are lower when compared to Paneth cells. Nevertheless, lactate was found in high concentrations in the small intestine, suggesting it could suppress the transcription of Defa5 in vivo and thereby prevent the release of pyrogenic molecules and the probable aberrant activation of inflammation (198).
In addition to SCFA-mediated HDP regulation, microbial-derived tryptophan catabolites (e.g., indole or indole-3-aldehyde) can bind the aryl hydrocarbon receptor (AhR) and activate IL-22 secretion (199). The AhR is a transcriptional factor expressed by several immune cell types, including RORgt+ ILC3s, that is crucial for the control of intestinal homeostasis in a ligand dependent manner (200). Binding of microbial AhR ligands induced IL-22 secretion by ILCs and stimulated the expression of the HDPs Lcn-2, S100A8, and S100A9, which was shown to be protective against C. albicans infection (26).
As described in section 3, Levy et al. demonstrated the IL-18 mediated induction of colonic ITLN1, RELMβ and angiogenins (27). By using colonic explants and colonic spheroids, the authors identified the microbiota-associated metabolite taurine, a bile acid conjugate, as the most potent activator of IL-18, which stimulated HDP expression via the activation of the NLRP6 inflammasome. Conversely, histamine and spermine (polyamine) featured the strongest suppression of IL-18. Furthermore, when taurine was administered to mice with dextran sodium sulfate (DSS)-induced colitis, this metabolite greatly improved colitis severity and weight loss, stressing its in vivo importance in the context of disease (27).
Consequently, the members of the microbiota play an essential role in the innate immune development and regulation of HDP function against pathogen infection. This effect may be mediated by the presence of beneficial microbes or by their associated metabolites. Identifying microbes or metabolites that have high potential to influence HDPs will aid in the selection of potential new probiotic strains. Furthermore, the use of such microbial metabolites could be exploited as a “postbiotic” treatment to control the interactions between host and microbiota (27).
Diet-Microbiota-Host Defense Interaction
Diet composition can define microbial communities in the intestine and the antimicrobial programming in the intestinal epithelium. Given the close interactions between microbes and HDPs, there is undoubtedly a close interrelationship of diet, HDPs, and the intestinal microbiota (Figure 4), in which it is difficult to define the extent to which each component is regulating each other.
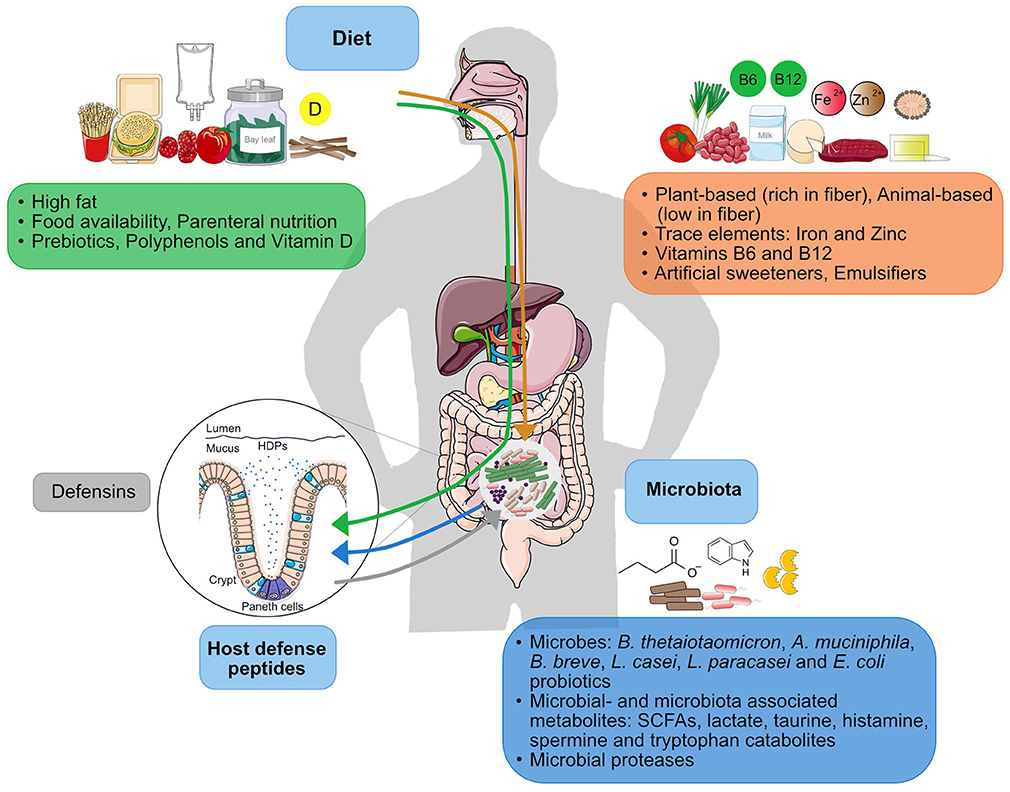
Figure 4. Factors within the diet-microbiota-HDP axis. The continuous interplay between microbiota and HDP (blue and gray arrows) is constantly influenced by diet. Effect of dietary factors on the microbiota is represented by the orange arrow and the effect of dietary factors on HDP function is represented by the green arrow.
The microbiota is a dynamic community and different types of diets change the microbiota composition. As we discussed, plant-based components seem to enrich beneficial microbes with the potential of improving immune function, whereas HFDs tend to enrich pro-inflammatory Proteobacteria communities. In both cases, however, HDP expression is also altered, but it is unclear if diet-dependent alterations of the microbiota composition can at least in part be caused by modulation of HDP expression. Most HFDs studies discussed here report that both the changes in HDP expression (Table 2) and microbiota alterations take place at the same time. However, by looking at different time-points, Guo et al. found that HFD-mediated alterations of the microbiota preceded changes in the levels of circulating inflammatory cytokines (Table 2) (177). Thus, HFD may presumably first change microbiota composition which in turn influences the immune response. In addition, diet composition and microbial metabolism will likely affect environmental conditions such as redox-potential and pH that can further mediate defensin function (18, 19). As a result of microbial fermentation, the pH in the gut becomes more acidic (from 6.5 to 5.5 as determined in-vitro), which can directly influence the activity of HDPs and favor the growth of butyrate-producing Firmicutes, such as Roseburia spp., while reducing the proliferation of the acid-sensitive Bacteroides spp. (201, 202).
Microbes can regulate HDP function directly through their structural components, through proteolytic activation/deactivation, and via their metabolites. However, defining the causative contribution of complex microbial communities on HDP expression is more challenging. Yet, a dysbiotic microbiota obtained from the caecum of SPF mice with ileitis was shown to transmit the inflammatory phenotype to genetically susceptible GF mice, which also led to a reduced expression of lysozyme, but not Defa2 (203). The transferred dysbiotic microbiota was characterized by an increase in the relative abundance of Clostridiales and decreased abundance of Porphyromonadacaeae (order of Bacteroidales), suggesting a pro-inflammatory potential of these taxa (203). Remarkably, it was the caecal microbiota that could induce the defect in the small intestine, which is unexpected, as the small intestine possess its own characteristic microbial community.
When attempting to understand the role of microbes in controlling HDP function, the mucosa-associated microbiota is expected to have an even stronger effect on the host as the luminal microbiota, since it is in closer interaction with the epithelium. Furthermore, in the case of defensin function, the ileal microbiota is expected to have a stronger influence than the colonic community. Indeed, Su et al. observed in their HFD experiment a reduced HDP expression (Table 2) that was accompanied by changes in microbiota composition in the ileum (173). They observed a pronounced increase in Proteobacteria with members Campylobacterales and Helicobacteraceae (including the hepatotoxic Helicobacter hepaticus), a mild increase in Firmicutes and a reduction in Bacteroidetes. Thus, a closer examination of the missing microbial communities mentioned in these studies could help in identifying microbes with distinct HDP modulating function.
Concluding Remarks
We are only beginning to understand how certain dietary components and microbes can signal to the epithelium and mediate HDP function. We discussed that the nutrient-sensing signaling mediators Ppar-γ, AhR, VDR, mTOR, and VIP-producing neurons are involved in diet-associated HDP control, while immune mediators in the TLR-Myd88/TRIF signaling pathway as well as the cytokines IFN-γ, IL-22, and IL-18 shape the microbe-dependent HDP regulation. However, more research is required to define the individual contribution of these different mediators to the complex regulation of intestinal HDP expression, and we believe that identifying strategies to fine-tune diet-HDP-microbiota interplay is a promising approach to strengthen the innate defenses in HDP-related disorders. In addition, further studies are required to understand whether HDPs can modulate and kill commensal bacteria in the gut or whether their activity is indeed limited to kill pathogenic microorganisms.
So, does an apple a day also keep the microbes away? Unfortunately, our current knowledge does not allow us to answer this question, but instead prompts us to ask other questions: Do we in fact want the microbes away from the intestinal mucosa, and thus eliminate beneficial microbes that stimulate HDPs? Or can we specifically fine-tune our defenses to target only pathogens and not commensals? Thus, rather than just relying on an apple, a diverse diet with different proportions of macronutrients and micronutrients is likely the food of choice to maintain a stable microbiota community that is separated from the host through a balanced HDP-microbiota homeostasis.
Author Contributions
The manuscript was written and edited by FP-B and BS. FP-B generated the figures included in this study using templates from Servier Medical Art by Servier licensed under a Creative Commons Attribution 3.0 Unported License.
Funding
BS is supported by a Starting Grant from the Swedish Research Council (dnr 2018-02095) and a group leader package from the Laboratory for Molecular Infection Medicine Sweden (MIMS) -The Nordic EMBL Partnership for Molecular Medicine at Umeå University, Sweden. The article processing charge is covered by an institutional library agreement from Umeå University. None of the funders had any influence on the content of this review.
Conflict of Interest
The authors declare that the research was conducted in the absence of any commercial or financial relationships that could be construed as a potential conflict of interest.
References
1. Koropatkin NM, Cameron EA, Martens EC. How glycan metabolism shapes the human gut microbiota. Nat Rev Microbiol. (2012) 10:323–35. doi: 10.1038/nrmicro2746
2. Sonnenburg ED, Sonnenburg JL. Starving our microbial self: the deleterious consequences of a diet deficient in microbiota-accessible carbohydrates. Cell Metab. (2014) 20:779–86. doi: 10.1016/j.cmet.2014.07.003
3. Nagai M, Obata Y, Takahashi D, Hase K. Fine-tuning of the mucosal barrier and metabolic systems using the diet-microbial metabolite axis. Int Immunopharmacol. (2016) 37:79–86. doi: 10.1016/j.intimp.2016.04.001
4. Thaiss CA, Zmora N, Levy M, Elinav E. The microbiome and innate immunity. Nature. (2016) 535:65–74. doi: 10.1038/nature18847
5. Schroeder BO, Bäckhed F. Signals from the gut microbiota to distant organs in physiology and disease. Nat Med. (2016) 22:1079–89. doi: 10.1038/nm.4185
6. Sonnenburg JL, Bäckhed F. Diet-microbiota interactions as moderators of human metabolism. Nature. (2016) 535:56–64. doi: 10.1038/nature18846
7. Strandwitz P. Neurotransmitter modulation by the gut microbiota. Brain Res. (2018) 1693:128–33. doi: 10.1016/j.brainres.2018.03.015
8. Zhernakova A, Kurilshikov A, Bonder MJ, Tigchelaar EF, Schirmer M, Vatanen T, et al. Population-based metagenomics analysis reveals markers for gut microbiome composition and diversity. Science. (2016) 352:565–9. doi: 10.1126/science.aad3369
9. Salzman NH, Hung K, Haribhai D, Chu H, Karlsson-Sjöberg J, Amir E, et al. Enteric defensins are essential regulators of intestinal microbial ecology. Nat Immunol. (2010) 11:76–82. doi: 10.1038/ni.1825
10. Goodrich JK, Davenport ER, Clark AG, Ley RE. The relationship between the human genome and microbiome comes into view. Annu Rev Genet. (2017) 51:413–33. doi: 10.1146/annurev-genet-110711-155532
11. Blander JM, Longman RS, Iliev ID, Sonnenberg GF, Artis D. Regulation of inflammation by microbiota interactions with the host. Nat Immunol. (2017) 18:851–60. doi: 10.1038/ni.3780
12. Mukherjee S, Hooper LV. Antimicrobial Defense of the Intestine. Immunity. (2015) 42:28–39. doi: 10.1016/j.immuni.2014.12.028
13. Salzman NH, Ghosh D, Huttner KM, Paterson Y, Bevins CL. Protection against enteric salmonellosis in transgenic mice expressing a human intestinal defensin. Nature. (2003) 422:522–6. doi: 10.1038/nature01520
14. Vaishnava S, Behrendt CL, Ismail AS, Eckmann L, Hooper LV. Paneth cells directly sense gut commensals and maintain homeostasis at the intestinal host-microbial interface. Proc Natl Acad Sci USA. (2008) 105:20858–63. doi: 10.1073/pnas.0808723105
15. Masuda K, Sakai N, Nakamura K, Yoshioka S, Ayabe T. Bactericidal activity of mouse α-defensin cryptdin-4 predominantly affects noncommensal bacteria. J Innate Immun. (2011) 3:315–26. doi: 10.1159/000322037
16. Cullen TW, Schofield WB, Barry NA, Putnam EE, Rundell EA, Trent MS, et al. Antimicrobial peptide resistance mediates resilience of prominent gut commensals during inflammation. Science. (2015) 347:170–5. doi: 10.1126/science.1260580
17. Larsen IS, Fritzen AM, Carl CS, Agerholm M, Damgaard MTF, Holm JB, et al. Human paneth cell α-defensin-5 treatment reverses dyslipidemia and improves glucoregulatory capacity in diet-induced obese mice. Am J Physiol-Endocrinol Metab. (2019) 317:E42–52. doi: 10.1152/ajpendo.00019.2019
18. Schroeder BO, Wu Z, Nuding S, Groscurth S, Marcinowski M, Beisner J, et al. Reduction of disulphide bonds unmasks potent antimicrobial activity of human β-defensin 1. Nature. (2011) 469:419–23. doi: 10.1038/nature09674
19. Schroeder BO, Ehmann D, Precht JC, Castillo PA, Küchler R, Berger J, et al. Paneth cell α-defensin 6 (HD-6) is an antimicrobial peptide. Mucosal Immunol. (2015) 8:661–71. doi: 10.1038/mi.2014.100
20. Es JH van, Jay P, Gregorieff A, Gijn ME van, Jonkheer S, Hatzis P, et al. Wnt signalling induces maturation of paneth cells in intestinal crypts. Nat Cell Biol. (2005) 7:381–6. doi: 10.1038/ncb1240
21. Ayabe T, Satchell DP, Wilson CL, Parks WC, Selsted ME, Ouellette AJ. Secretion of microbicidal α-defensins by intestinal paneth cells in response to bacteria. Nat Immunol. (2000) 1:113–8. doi: 10.1038/77783
22. Goto Y. Epithelial cells as a transmitter of signals from commensal bacteria and host immune cells. Front Immunol. (2019) 10:2057. doi: 10.3389/fimmu.2019.02057
23. Karlsson J, Pütsep K, Chu H, Kays RJ, Bevins CL, Andersson M. Regional variations in Paneth cell antimicrobial peptide expression along the mouse intestinal tract. BMC Immunol. (2008) 9:37. doi: 10.1186/1471-2172-9-37
24. Cash HL. Symbiotic bacteria direct expression of an intestinal bactericidal lectin. Science. (2006) 313:1126–30. doi: 10.1126/science.1127119
25. Möndel M, Schroeder BO, Zimmermann K, Huber H, Nuding S, Beisner J, et al. Probiotic E. coli treatment mediates antimicrobial human β-defensin synthesis and fecal excretion in humans. Mucosal Immunol. (2009) 2:166–72. doi: 10.1038/mi.2008.77
26. Zelante T, Iannitti RG, Cunha C, De Luca A, Giovannini G, Pieraccini G, et al. Tryptophan catabolites from microbiota engage aryl hydrocarbon receptor and balance mucosal reactivity via interleukin-22. Immunity. (2013) 39:372–85. doi: 10.1016/j.immuni.2013.08.003
27. Levy M, Thaiss CA, Zeevi D, Dohnalová L, Zilberman-Schapira G, Mahdi JA, et al. Microbiota-modulated metabolites shape the intestinal microenvironment by regulating nlrp6 inflammasome signaling. Cell. (2015) 163:1428–43. doi: 10.1016/j.cell.2015.10.048
28. Holscher HD. Dietary fiber and prebiotics and the gastrointestinal microbiota. Gut Microbes. (2017) 8:172–84. doi: 10.1080/19490976.2017.1290756
29. Schroeder BO, Birchenough GMH, Ståhlman M, Arike L, Johansson MEV, Hansson GC, et al. Bifidobacteria or fiber protects against diet-induced microbiota-mediated colonic mucus deterioration. Cell Host Microbe. (2018) 23:27–40.e7. doi: 10.1016/j.chom.2017.11.004
30. Tomova A, Bukovsky I, Rembert E, Yonas W, Alwarith J, Barnard ND, et al. The effects of vegetarian and vegan diets on gut microbiota. Front Nutr. (2019) 6:47. doi: 10.3389/fnut.2019.00047
31. Singh RK, Chang H-W, Yan D, Lee KM, Ucmak D, Wong K, et al. Influence of diet on the gut microbiome and implications for human health. J Transl Med. (2017) 15:73. doi: 10.1186/s12967-017-1175-y
32. Sonnenburg ED, Smits SA, Tikhonov M, Higginbottom SK, Wingreen NS, Sonnenburg JL. Diet-induced extinctions in the gut microbiota compound over generations. Nature. (2016) 529:212–15. doi: 10.1038/nature16504
33. Yatsunenko T, Rey FE, Manary MJ, Trehan I, Dominguez-Bello MG, Contreras M, et al. Human gut microbiome viewed across age and geography. Nature. (2012) 486:222–7. doi: 10.1038/nature11053
34. De Filippo C, Cavalieri D, Di Paola M, Ramazzotti M, Poullet JB, Massart S, et al. Impact of diet in shaping gut microbiota revealed by a comparative study in children from Europe and rural Africa. Proc Natl Acad Sci USA. (2010) 107:14691–6. doi: 10.1073/pnas.1005963107
35. Schnorr SL, Candela M, Rampelli S, Centanni M, Consolandi C, Basaglia G, et al. Gut microbiome of the Hadza hunter-gatherers. Nat Commun. (2014) 5:3654. doi: 10.1038/ncomms4654
36. Desai MS, Seekatz AM, Koropatkin NM, Kamada N, Hickey CA, Wolter M, et al. A dietary fiber-deprived gut microbiota degrades the colonic mucus barrier and enhances pathogen susceptibility. Cell. (2016) 167:1339–53.e21. doi: 10.1016/j.cell.2016.10.043
37. Christ A, Lauterbach M, Latz E. Western diet and the immune system: an inflammatory connection. Immunity. (2019) 51:794–811. doi: 10.1016/j.immuni.2019.09.020
38. Murphy EA, Velazquez KT, Herbert KM. Influence of high-fat-diet on gut microbiota: a driving force for chronic disease risk. Curr Opin Clin Nutr Metab Care. (2016) 18:515–20. doi: 10.1097/MCO.0000000000000209
39. Suez J, Korem T, Zeevi D, Zilberman-Schapira G, Thaiss CA, Maza O, et al. Artificial sweeteners induce glucose intolerance by altering the gut microbiota. Nature. (2014) 514:181–6. doi: 10.1038/nature13793
40. Chassaing B, Koren O, Goodrich JK, Poole AC, Srinivasan S, Ley RE, et al. Dietary emulsifiers impact the mouse gut microbiota promoting colitis and metabolic syndrome. Nature. (2015) 519:92–6. doi: 10.1038/nature14232
41. Gentschew L, Ferguson LR. Role of nutrition and microbiota in susceptibility to inflammatory bowel diseases. Mol Nutr Food Res. (2012) 56:524–35. doi: 10.1002/mnfr.201100630
42. Cândido FG, Valente FX, Grześkowiak ŁM, Moreira APB, Rocha DMUP, Alfenas R de CG. Impact of dietary fat on gut microbiota and low-grade systemic inflammation: mechanisms and clinical implications on obesity. Int J Food Sci Nutr. (2018) 69:125–43. doi: 10.1080/09637486.2017.1343286
43. Wehkamp J. NOD2 (CARD15) mutations in Crohn's disease are associated with diminished mucosal -defensin expression. Gut. (2004) 53:1658–64. doi: 10.1136/gut.2003.032805
44. Wehkamp J, Salzman NH, Porter E, Nuding S, Weichenthal M, Petras RE, et al. Reduced paneth cell -defensins in ileal Crohn's disease. Proc Natl Acad Sci USA. (2005) 102:18129–34. doi: 10.1073/pnas.0505256102
45. Simms LA, Doecke JD, Walsh MD, Huang N, Fowler EV, Radford-Smith GL. Reduced -defensin expression is associated with inflammation and not NOD2 mutation status in ileal Crohn's disease. Gut. (2008) 57:903–10. doi: 10.1136/gut.2007.142588
46. Wassermann B, Müller H, Berg G. An apple a day: which bacteria do we eat with organic and conventional apples? Front Microbiol. (2019) 10:1629. doi: 10.3389/fmicb.2019.01629
47. Eckburg PB. Diversity of the human intestinal microbial flora. Science. (2005) 308:1635–8. doi: 10.1126/science.1110591
48. Arumugam M, Raes J, Pelletier E, Paslier DL, Yamada T, Mende DR, et al. Enterotypes of the human gut microbiome. Nature. (2011) 473:174–80. doi: 10.1038/nature09944
49. Knights D, Ward TL, McKinlay CE, Miller H, Gonzalez A, McDonald D, et al. Rethinking “Enterotypes.” Cell Host Microbe. (2014) 16:433–7. doi: 10.1016/j.chom.2014.09.013
50. Manichanh C. Reduced diversity of faecal microbiota in Crohn's disease revealed by a metagenomic approach. Gut. (2006) 55:205–11. doi: 10.1136/gut.2005.073817
51. Lane ER, Zisman T, Suskind D. The microbiota in inflammatory bowel disease: current and therapeutic insights. J Inflamm Res. (2017) 10:63–73. doi: 10.2147/JIR.S116088
52. Stange EF, Schroeder BO. Microbiota and mucosal defense in IBD: an update. Expert Rev Gastroenterol Hepatol. (2019) 13:963–76. doi: 10.1080/17474124.2019.1671822
53. Ley RE, Backhed F, Turnbaugh P, Lozupone CA, Knight RD, Gordon JI. Obesity alters gut microbial ecology. Proc Natl Acad Sci USA. (2005) 102:11070–5. doi: 10.1073/pnas.0504978102
54. Turnbaugh PJ, Ley RE, Mahowald MA, Magrini V, Mardis ER, Gordon JI. An obesity-associated gut microbiome with increased capacity for energy harvest. Nature. (2006) 444:1027–31. doi: 10.1038/nature05414
55. Ridaura VK, Faith JJ, Rey FE, Cheng J, Duncan AE, Kau AL, et al. Gut microbiota from twins discordant for obesity modulate metabolism in mice. Science. (2013) 341:1241214. doi: 10.1126/science.1241214
56. Verdam FJ, Fuentes S, de Jonge C, Zoetendal EG, Erbil R, Greve JW, et al. Human intestinal microbiota composition is associated with local and systemic inflammation in obesity: obese gut microbiota and inflammation. Obesity. (2013) 21:E607–15. doi: 10.1002/oby.20466
57. Wen L, Ley RE, Volchkov PY, Stranges PB, Avanesyan L, Stonebraker AC, et al. Innate immunity and intestinal microbiota in the development of type 1 diabetes. Nature. (2008) 455:1109–13. doi: 10.1038/nature07336
58. Larsen N, Vogensen FK, van den Berg FWJ, Nielsen DS, Andreasen AS, Pedersen BK, et al. Gut microbiota in human adults with type 2 diabetes differs from non-diabetic adults. PLoS ONE. (2010) 5:e9085. doi: 10.1371/journal.pone.0009085
59. Qin J, Li Y, Cai Z, Li S, Zhu J, Zhang F, et al. A metagenome-wide association study of gut microbiota in type 2 diabetes. Nature. (2012) 490:55–60. doi: 10.1038/nature11450
60. Hong P-Y, Lee BW, Aw M, Shek LPC, Yap GC, Chua KY, et al. Comparative analysis of fecal microbiota in infants with and without eczema. PLoS ONE. (2010) 5:e9964. doi: 10.1371/journal.pone.0009964
61. Fujimura KE, Lynch SV. Microbiota in allergy and asthma and the emerging relationship with the gut microbiome. Cell Host Microbe. (2015) 17:592–602. doi: 10.1016/j.chom.2015.04.007
62. Unger MM, Spiegel J, Dillmann K-U, Grundmann D, Philippeit H, Bürmann J, et al. Short chain fatty acids and gut microbiota differ between patients with Parkinson's disease and age-matched controls. Parkinsonism Relat Disord. (2016) 32:66–72. doi: 10.1016/j.parkreldis.2016.08.019
63. Ding HT, Taur Y, Walkup JT. Gut microbiota and autism: key concepts and findings. J Autism Dev Disord. (2017) 47:480–9. doi: 10.1007/s10803-016-2960-9
64. Jonsson AL, Bäckhed F. Role of gut microbiota in atherosclerosis. Nat Rev Cardiol. (2017) 14:79–87. doi: 10.1038/nrcardio.2016.183
65. Kolodziejczyk AA, Zheng D, Elinav E. Diet-microbiota interactions and personalized nutrition. Nat Rev Microbiol. (2019) 17:742–53. doi: 10.1038/s41579-019-0256-8
66. Reese AT, Carmody RN. Thinking outside the cereal box: noncarbohydrate routes for dietary manipulation of the gut microbiota. Appl Environ Microbiol. (2018) 85:e02246–18. doi: 10.1128/AEM.02246-18
67. David LA, Maurice CF, Carmody RN, Gootenberg DB, Button JE, Wolfe BE, et al. Diet rapidly and reproducibly alters the human gut microbiome. Nature. (2014) 505:559–63. doi: 10.1038/nature12820
68. Oliphant K, Allen-Vercoe E. Macronutrient metabolism by the human gut microbiome: major fermentation by-products and their impact on host health. Microbiome. (2019) 7:91. doi: 10.1186/s40168-019-0704-8
69. Wong JMW, Jenkins DJA. Carbohydrate digestibility and metabolic effects. J Nutr. (2007) 137:2539S−46S. doi: 10.1093/jn/137.11.2539S
70. Morales P, Fujio S, Navarrete P, Ugalde JA, Magne F, Carrasco-Pozo C, et al. Impact of dietary lipids on colonic function and microbiota: an experimental approach involving orlistat-induced fat malabsorption in human volunteers. Clin Transl Gastroenterol. (2016) 7:e161. doi: 10.1038/ctg.2016.20
71. Yao CK, Muir JG, Gibson PR. Review article: insights into colonic protein fermentation, its modulation and potential health implications. Aliment Pharmacol Ther. (2016) 43:181–96. doi: 10.1111/apt.13456
72. Lombard V, Golaconda Ramulu H, Drula E, Coutinho PM, Henrissat B. The carbohydrate-active enzymes database (CAZy) in 2013. Nucleic Acids Res. (2014) 42:D490–5. doi: 10.1093/nar/gkt1178
73. Bhattacharya T, Ghosh TS, Mande SS. Global profiling of carbohydrate active enzymes in human gut microbiome. PLOS ONE. (2015) 10:e0142038. doi: 10.1371/journal.pone.0142038
74. Koh A, De Vadder F, Kovatcheva-Datchary P, Bäckhed F. From dietary fiber to host physiology: short-chain fatty acids as key bacterial metabolites. Cell. (2016) 165:1332–45. doi: 10.1016/j.cell.2016.05.041
75. Mohan A, Quek S-Y, Gutierrez-Maddox N, Gao Y, Shu Q. Effect of honey in improving the gut microbial balance. Food Qual Saf. (2017) 1:207–15. doi: 10.1093/fqs/fyx015
76. Elleuch M, Bedigian D, Roiseux O, Besbes S, Blecker C, Attia H. Dietary fibre and fibre-rich by-products of food processing: characterisation, technological functionality and commercial applications: a review. Food Chem. (2011) 124:411–21. doi: 10.1016/j.foodchem.2010.06.077
77. McRorie JW, Fahey GC. A review of gastrointestinal physiology and the mechanisms underlying the health benefits of dietary fiber: matching an effective fiber with specific patient needs. Clin Nurs Stud. (2013) 1, 82–92. doi: 10.5430/cns.v1n4p82
78. Ndeh D, Rogowski A, Cartmell A, Luis AS, Baslé A, Gray J, et al. Complex pectin metabolism by gut bacteria reveals novel catalytic functions. Nature. (2017) 544:65–70. doi: 10.1038/nature21725
79. Blatchford P, Stoklosinski H, Eady S, Wallace A, Butts C, Gearry R, et al. Consumption of kiwifruit capsules increases Faecalibacterium prausnitzii abundance in functionally constipated individuals: a randomised controlled human trial. J Nutr Sci. (2017) 6:e52. doi: 10.1017/jns.2017.52
80. Parkar SG, Rosendale D, Paturi G, Herath TD, Stoklosinski H, Phipps JE, et al. In vitro utilization of gold and green kiwifruit oligosaccharides by human gut microbial populations. Plant Foods Hum Nutr. (2012) 67:200–7. doi: 10.1007/s11130-012-0293-1
81. Swanson KS, de Vos WM, Martens EC, Gilbert JA, Menon RS, Soto-Vaca A, et al. Effect of fructans, prebiotics and fibres on the human gut microbiome assessed by 16S rRNA-based approaches: a review. Benef Microbes. (2020) 11:101–29. doi: 10.3920/BM2019.0082
82. Segata N. Gut microbiome: westernization and the disappearance of intestinal diversity. Curr Biol. (2015) 25:R611–13. doi: 10.1016/j.cub.2015.05.040
83. Turnbaugh PJ, Bäckhed F, Fulton L, Gordon JI. Diet-induced obesity is linked to marked but reversible alterations in the mouse distal gut microbiome. Cell Host Microbe. (2008) 3:213–23. doi: 10.1016/j.chom.2008.02.015
84. Hildebrandt MA, Hoffmann C, Sherrill-Mix SA, Keilbaugh SA, Hamady M, Chen Y, et al. High-fat diet determines the composition of the murine gut microbiome independently of obesity. Gastroenterology. (2009) 137:1716–24.e2. doi: 10.1053/j.gastro.2009.08.042
85. Zhang C, Zhang M, Pang X, Zhao Y, Wang L, Zhao L. Structural resilience of the gut microbiota in adult mice under high-fat dietary perturbations. ISME J. (2012) 6:1848–57. doi: 10.1038/ismej.2012.27
86. Kübeck R, Bonet-Ripoll C, Hoffmann C, Walker A, Müller VM, Schüppel VL, et al. Dietary fat and gut microbiota interactions determine diet-induced obesity in mice. Mol Metab. (2016) 5:1162–74. doi: 10.1016/j.molmet.2016.10.001
87. Agans R, Gordon A, Kramer DL, Perez-Burillo S, Rufián-Henares JA, Paliy O. Dietary fatty acids sustain the growth of the human gut microbiota. Appl Environ Microbiol. (2018) 84:e01525–18. doi: 10.1128/AEM.01525-18
88. Martinez-Guryn K, Hubert N, Frazier K, Urlass S, Musch MW, Ojeda P, et al. Small intestine microbiota regulate host digestive and absorptive adaptive responses to dietary lipids. Cell Host Microbe. (2018) 23:458–69.e5. doi: 10.1016/j.chom.2018.03.011
89. Randrianarisoa E, Lehn-Stefan A, Wang X, Hoene M, Peter A, Heinzmann SS, et al. Relationship of serum trimethylamine N-Oxide (TMAO) levels with early atherosclerosis in humans. Sci Rep. (2016) 6:26745. doi: 10.1038/srep26745
90. Qi J, You T, Li J, Pan T, Xiang L, Han Y, et al. Circulating trimethylamine N-oxide and the risk of cardiovascular diseases: a systematic review and meta-analysis of 11 prospective cohort studies. J Cell Mol Med. (2018) 22:185–94. doi: 10.1111/jcmm.13307
91. Hentges DJ, Maier BR, Burton GC, Flynn MA, Tsutakawa RK. Effect of a high-beef diet on the fecal bacterial flora of humans. Cancer Res. (1977) 37:568–71.
92. Reese AT, Pereira FC, Schintlmeister A, Berry D, Wagner M, Hale LP, et al. Microbial nitrogen limitation in the mammalian large intestine. Nat Microbiol. (2018) 3:1441–50. doi: 10.1038/s41564-018-0267-7
93. Selma MV, Espín JC, Tomás-Barberán FA. Interaction between phenolics and gut microbiota: role in human health. J Agric Food Chem. (2009) 57:6485–501. doi: 10.1021/jf902107d
94. Ozdal T, Sela DA, Xiao J, Boyacioglu D, Chen F, Capanoglu E. The reciprocal interactions between polyphenols and gut microbiota and effects on bioaccessibility. Nutrients. (2016) 8:78. doi: 10.3390/nu8020078
95. Lee HC, Jenner AM, Low CS, Lee YK. Effect of tea phenolics and their aromatic fecal bacterial metabolites on intestinal microbiota. Res Microbiol. (2006) 157:876–84. doi: 10.1016/j.resmic.2006.07.004
96. Queipo-Ortuño MI, Boto-Ordóñez M, Murri M, Gomez-Zumaquero JM, Clemente-Postigo M, Estruch R, et al. Influence of red wine polyphenols and ethanol on the gut microbiota ecology and biochemical biomarkers. Am J Clin Nutr. (2012) 95:1323–34. doi: 10.3945/ajcn.111.027847
97. Sun H, Chen Y, Cheng M, Zhang X, Zheng X, Zhang Z. The modulatory effect of polyphenols from green tea, oolong tea and black tea on human intestinal microbiota in vitro. J Food Sci Technol. (2018) 55:399–407. doi: 10.1007/s13197-017-2951-7
98. Kortman GAM, Boleij A, Swinkels DW, Tjalsma H. Iron availability increases the pathogenic potential of Salmonella typhimurium and other enteric pathogens at the intestinal epithelial interface. PLoS ONE. (2012) 7:e29968. doi: 10.1371/journal.pone.0029968
99. Reed S, Neuman H, Moscovich S, Glahn RP, Koren O, Tako E. Chronic zinc deficiency alters chick gut microbiota composition and function. Nutrients. (2015) 7:9768–84. doi: 10.3390/nu7125497
100. Bäumler AJ, Sperandio V. Interactions between the microbiota and pathogenic bacteria in the gut. Nature. (2016) 535:85–93. doi: 10.1038/nature18849
101. Biesalski HK. Nutrition meets the microbiome: micronutrients and the microbiota: nutrition meets the microbiome. Ann N Y Acad Sci. (2016) 1372:53–64. doi: 10.1111/nyas.13145
102. Morrison KE, Jašarević E, Howard CD, Bale TL. It's the fiber, not the fat: significant effects of dietary challenge on the gut microbiome. Microbiome. (2020) 8:15. doi: 10.1186/s40168-020-0791-6
103. Pabst O, Slack E. IgA and the intestinal microbiota: the importance of being specific. Mucosal Immunol. (2020) 13:12–21. doi: 10.1038/s41385-019-0227-4
104. Schroeder BO. Fight them or feed them: how the intestinal mucus layer manages the gut microbiota. Gastroenterol Rep. (2019) 7:3–12. doi: 10.1093/gastro/goy052
105. Dupont A, Heinbockel L, Brandenburg K, Hornef MW. Antimicrobial peptides and the enteric mucus layer act in concert to protect the intestinal mucosa. Gut Microbes. (2014) 5:761–5. doi: 10.4161/19490976.2014.972238
106. Meyer-Hoffert U, Hornef MW, Henriques-Normark B, Axelsson L-G, Midtvedt T, Pütsep K, et al. Secreted enteric antimicrobial activity localises to the mucus surface layer. Gut. (2008) 57:764–71. doi: 10.1136/gut.2007.141481
107. Antoni L, Nuding S, Weller D, Gersemann M, Ott G, Wehkamp J, et al. Human colonic mucus is a reservoir for antimicrobial peptides. J Crohns Colitis. (2013) 7:e652–64. doi: 10.1016/j.crohns.2013.05.006
108. Okumura R, Kurakawa T, Nakano T, Kayama H, Kinoshita M, Motooka D, et al. Lypd8 promotes the segregation of flagellated microbiota and colonic epithelia. Nature. (2016) 532:117–21. doi: 10.1038/nature17406
109. Artis D, Wang ML, Keilbaugh SA, He W, Brenes M, Swain GP, et al. RELMbeta/FIZZ2 is a goblet cell-specific immune-effector molecule in the gastrointestinal tract. Proc Natl Acad Sci USA. (2004) 101:13596–600. doi: 10.1073/pnas.0404034101
110. Propheter DC, Chara AL, Harris TA, Ruhn KA, Hooper LV. Resistin-like molecule β is a bactericidal protein that promotes spatial segregation of the microbiota and the colonic epithelium. Proc Natl Acad Sci USA. (2017) 114:11027–33. doi: 10.1073/pnas.1711395114
111. Mastroianni JR, Costales JK, Zaksheske J, Selsted ME, Salzman NH, Ouellette AJ. Alternative luminal activation mechanisms for paneth cell α-defensins. J Biol Chem. (2012) 287:11205–12. doi: 10.1074/jbc.M111.333559
112. Bevins CL, Salzman NH. Paneth cells, antimicrobial peptides and maintenance of intestinal homeostasis. Nat Rev Microbiol. (2011) 9:356–68. doi: 10.1038/nrmicro2546
113. Ganz T. Defensins: antimicrobial peptides of innate immunity. Nat Rev Immunol. (2003) 3:710–20. doi: 10.1038/nri1180
114. Hornef MW, Pütsep K, Karlsson J, Refai E, Andersson M. Increased diversity of intestinal antimicrobial peptides by covalent dimer formation. Nat Immunol. (2004) 5:836–43. doi: 10.1038/ni1094
115. Wang G. Human antimicrobial peptides and proteins. Pharmaceuticals. (2014) 7:545–94. doi: 10.3390/ph7050545
116. Harder J, Gläser R, Schröder J-M. Human antimicrobial proteins effectors of innate immunity. J Endotoxin Res. (2007) 13:317–38. doi: 10.1177/0968051907088275
117. Lehrer RI, Lichtenstein AK, Ganz T. Defensins: antimicrobial and cytotoxic peptides of mammalian cells. Annu Rev Immunol. (1993) 11:105–28. doi: 10.1146/annurev.iy.11.040193.000541
118. Baxter AA, Lay FT, Poon IKH, Kvansakul M, Hulett MD. Tumor cell membrane-targeting cationic antimicrobial peptides: novel insights into mechanisms of action and therapeutic prospects. Cell Mol Life Sci. (2017) 74:3809–25. doi: 10.1007/s00018-017-2604-z
119. Andersson ML, Karlsson-Sjöberg JMT, Pütsep KL-A. CRS-peptides: unique defense peptides of mouse Paneth cells. Mucosal Immunol. (2012) 5:367–76. doi: 10.1038/mi.2012.22
120. Castillo PA, Nonnecke EB, Ossorio DT, Tran MTN, Goley SM, Lönnerdal B, et al. An experimental approach to rigorously assess paneth cell α-defensin (Defa) mRNA expression in C57BL/6 mice. Sci Rep. (2019) 9:13115. doi: 10.1038/s41598-019-49471-9
121. Wehkamp J, Chu H, Shen B, Feathers RW, Kays RJ, Lee SK, et al. Paneth cell antimicrobial peptides: topographical distribution and quantification in human gastrointestinal tissues. FEBS Lett. (2006) 580:5344–50. doi: 10.1016/j.febslet.2006.08.083
122. Ericksen B, Wu Z, Lu W, Lehrer RI. Antibacterial activity and specificity of the six human -defensins. Antimicrob Agents Chemother. (2005) 49:269–75. doi: 10.1128/AAC.49.1.269-275.2005
123. Chu H, Pazgier M, Jung G, Nuccio S-P, Castillo PA, de Jong MF, et al. Human -defensin 6 promotes mucosal innate immunity through self-assembled peptide nanonets. Science. (2012) 337:477–81. doi: 10.1126/science.1218831
124. O'Neil DA, Porter EM, Elewaut D, Anderson GM, Eckmann L, Ganz T, et al. Expression and regulation of the human β-defensins hBD-1 and hBD-2 in intestinal epithelium. J Immunol. (1999) 163:6718–24.
125. Wendler J, Schroeder BO, Ehmann D, Koeninger L, Mailänder-Sánchez D, Lemberg C, et al. Proteolytic degradation of reduced human beta defensin 1 generates a novel antibiotic octapeptide. Sci Rep. (2019) 9:3640. doi: 10.1038/s41598-019-40216-2
126. Mukherjee S, Zheng H, Derebe MG, Callenberg KM, Partch CL, Rollins D, et al. Antibacterial membrane attack by a pore-forming intestinal C-type lectin. Nature. (2014) 505:103–7. doi: 10.1038/nature12729
127. Hooper LV, Stappenbeck TS, Hong CV, Gordon JI. Angiogenins: a new class of microbicidal proteins involved in innate immunity. Nat Immunol. (2003) 4:269–73. doi: 10.1038/ni888
128. Sheng J, Xu Z. Three decades of research on angiogenin: a review and perspective. Acta Biochim Biophys Sin. (2016) 48:399–410. doi: 10.1093/abbs/gmv131
129. Berger T, Togawa A, Duncan GS, Elia AJ, You-Ten A, Wakeham A, et al. Lipocalin 2-deficient mice exhibit increased sensitivity to Escherichia coli infection but not to ischemia-reperfusion injury. Proc Natl Acad Sci USA. (2006) 103:1834–9. doi: 10.1073/pnas.0510847103
130. Wang Q, Li S, Tang X, Liang L, Wang F, Du H. Lipocalin 2 protects against Escherichia coli infection by modulating neutrophil and macrophage function. Front Immunol. (2019) 10:2594. doi: 10.3389/fimmu.2019.02594
131. Bergström JH, Birchenough GMH, Katona G, Schroeder BO, Schütte A, Ermund A, et al. Gram-positive bacteria are held at a distance in the colon mucus by the lectin-like protein ZG16. Proc Natl Acad Sci USA. (2016) 113:13833–8. doi: 10.1073/pnas.1611400113
132. Okumura R, Kodama T, Hsu C-C, Sahlgren BH, Hamano S, Kurakawa T, et al. Lypd8 inhibits attachment of pathogenic bacteria to colonic epithelia. Mucosal Immunol. (2020) 13:75–85. doi: 10.1038/s41385-019-0219-4
133. He W, Wang M-L, Jiang H-Q, Steppan CM, Shin ME, Thurnheer MC, et al. Bacterial colonization leads to the colonic secretion of RELMbeta/FIZZ2, a novel goblet cell-specific protein. Gastroenterology. (2003) 125:1388–97. doi: 10.1016/j.gastro.2003.07.009
134. Tremblay S, Côté NML, Grenier G, Duclos-Lasnier G, Fortier L-C, Ilangumaran S, et al. Ileal antimicrobial peptide expression is dysregulated in old age. Immun Ageing. (2017) 14:19. doi: 10.1186/s12979-017-0101-8
135. Cadwell K, Liu JY, Brown SL, Miyoshi H, Loh J, Lennerz JK, et al. A key role for autophagy and the autophagy gene Atg16l1 in mouse and human intestinal paneth cells. Nature. (2008) 456:259–63. doi: 10.1038/nature07416
136. Andreu P. Crypt-restricted proliferation and commitment to the Paneth cell lineage following Apc loss in the mouse intestine. Development. (2005) 132:1443–51. doi: 10.1242/dev.01700
137. Ménard S, Förster V, Lotz M, Gütle D, Duerr CU, Gallo RL, et al. Developmental switch of intestinal antimicrobial peptide expression. J Exp Med. (2008) 205:183–93. doi: 10.1084/jem.20071022
138. Farin HF, Karthaus WR, Kujala P, Rakhshandehroo M, Schwank G, Vries RGJ, et al. Paneth cell extrusion and release of antimicrobial products is directly controlled by immune cell-derived IFN-γ. J Exp Med. (2014) 211:1393–405. doi: 10.1084/jem.20130753
139. Noor Z, Burgess SL, Watanabe K, Petri WA. Interleukin-25 mediated induction of angiogenin-4 is interleukin-13 dependent. PLOS ONE. (2016) 11:e0153572. doi: 10.1371/journal.pone.0153572
140. Wolk K, Kunz S, Witte E, Friedrich M, Asadullah K, Sabat R. IL-22 increases the innate immunity of tissues. Immunity. (2004) 21:241–54. doi: 10.1016/j.immuni.2004.07.007
141. Zheng Y, Valdez PA, Danilenko DM, Hu Y, Sa SM, Gong Q, et al. Interleukin-22 mediates early host defense against attaching and effacing bacterial pathogens. Nat Med. (2008) 14:282–9. doi: 10.1038/nm1720
142. Raffatellu M, George MD, Akiyama Y, Hornsby MJ, Nuccio S-P, Paixao TA, et al. Lipocalin-2 resistance of Salmonella enterica serotype Typhimurium confers an advantage during life in the inflamed intestine. Cell Host Microbe. (2009) 5:476–86. doi: 10.1016/j.chom.2009.03.011
143. Vaishnava S, Yamamoto M, Severson KM, Ruhn KA, Yu X, Koren O, et al. The antibacterial lectin regiiiγ promotes the spatial segregation of microbiota and host in the intestine. Science. (2011) 334:255–8. doi: 10.1126/science.1209791
144. Walker CR, Hautefort I, Dalton JE, Overweg K, Egan CE, Bongaerts RJ, et al. Intestinal intraepithelial lymphocyte-enterocyte crosstalk regulates production of bactericidal angiogenin 4 by paneth cells upon microbial challenge. PLOS ONE. (2013) 8:e84553. doi: 10.1371/journal.pone.0084553
145. Sugimoto K, Ogawa A, Mizoguchi E, Shimomura Y, Andoh A, Bhan AK, et al. IL-22 ameliorates intestinal inflammation in a mouse model of ulcerative colitis. J Clin Invest. (2008) 118:534–44. doi: 10.1172/JCI33194
146. Kinnebrew MA, Buffie CG, Diehl GE, Zenewicz LA, Leiner I, Hohl TM, et al. Intestinal CD103+ CD11b+ lamina propria dendritic cells instruct intestinal epithelial cells to express antimicrobial proteins in response to toll-like receptor 5 activation. Immunity. (2012) 36:276–87. doi: 10.1016/j.immuni.2011.12.011
147. Dudakov JA, Hanash AM, van den Brink MRM. Interleukin-22: immunobiology and pathology. Annu Rev Immunol. (2015) 33:747–85. doi: 10.1146/annurev-immunol-032414-112123
148. Ngo VL, Abo H, Maxim E, Harusato A, Geem D, Medina-Contreras O, et al. A cytokine network involving IL-36γ, IL-23, and IL-22 promotes antimicrobial defense and recovery from intestinal barrier damage. Proc Natl Acad Sci USA. (2018) 115:E5076–85. doi: 10.1073/pnas.1718902115
149. Menendez A, Willing BP, Montero M, Wlodarska M, So CC, Bhinder G, et al. Bacterial stimulation of the TLR-MyD88 pathway modulates the homeostatic expression of ileal paneth cell α-defensins. J Innate Immun. (2013) 5:39–49. doi: 10.1159/000341630
150. Liang S, Guo X-K, Ou J, Huang R, Xue Q, Zhang B, et al. Nutrient sensing by the intestinal epithelium orchestrates mucosal antimicrobial defense via translational control of hes1. Cell Host Microbe. (2019) 25:706–18.e7. doi: 10.1016/j.chom.2019.03.012
151. Stockinger S, Duerr CU, Fulde M, Dolowschiak T, Pott J, Yang I, et al. TRIF signaling drives homeostatic intestinal epithelial antimicrobial peptide expression. J Immunol. (2014) 193:4223–34. doi: 10.4049/jimmunol.1302708
152. Wilson CL, Ouellette AJ, Satchell DP, Ayabe T, López-Boado YS, Stratman JL, et al. Regulation of intestinal α-defensin activation by the metalloproteinase matrilysin in innate host defense. Science. (1999) 286:113–7. doi: 10.1126/science.286.5437.113
153. Ghosh D, Porter E, Shen B, Lee SK, Wilk D, Drazba J, et al. Paneth cell trypsin is the processing enzyme for human defensin-5. Nat Immunol. (2002) 3:583–90. doi: 10.1038/ni797
154. Ehmann D, Wendler J, Koeninger L, Larsen IS, Klag T, Berger J, et al. Paneth cell α-defensins HD-5 and HD-6 display differential degradation into active antimicrobial fragments. Proc Natl Acad Sci USA. (2019) 116:3746–51. doi: 10.1073/pnas.1817376116
155. Salzman NH. Paneth cell defensins and the regulation of the microbiome: Détente at mucosal surfaces. Gut Microbes. (2010) 1:401–6. doi: 10.4161/gmic.1.6.14076
156. Filipp D, Brabec T, Voboril M, Dobeš J. Enteric α-defensins on the verge of intestinal immune tolerance and inflammation. Semin Cell Dev Biol. (2019) 88:138–46. doi: 10.1016/j.semcdb.2018.01.007
157. Salzman NH, Bevins CL. Dysbiosis-a consequence of paneth cell dysfunction. Semin Immunol. (2013) 25:334–41. doi: 10.1016/j.smim.2013.09.006
158. Liu T-C, Gurram B, Baldridge MT, Head R, Lam V, Luo C, et al. Paneth cell defects in Crohn's disease patients promote dysbiosis. JCI Insight. (2016) 1:86907. doi: 10.1172/jci.insight.86907
159. Wehkamp J, Stange EF. An update review on the paneth cell as key to ileal Crohn's disease. Front Immunol. (2020) 11:646. doi: 10.3389/fimmu.2020.00646
160. Hampe J, Franke A, Rosenstiel P, Till A, Teuber M, Huse K, et al. A genome-wide association scan of nonsynonymous SNPs identifies a susceptibility variant for Crohn disease in ATG16L1. Nat Genet. (2007) 39:207–11. doi: 10.1038/ng1954
161. Kaser A, Lee A-H, Franke A, Glickman JN, Zeissig S, Tilg H, et al. XBP1 links er stress to intestinal inflammation and confers genetic risk for human inflammatory bowel disease. Cell. (2008) 134:743–56. doi: 10.1016/j.cell.2008.07.021
162. Adolph TE, Tomczak MF, Niederreiter L, Ko H-J, Böck J, Martinez-Naves E, et al. Paneth cells as a site of origin for intestinal inflammation. Nature. (2013) 503:272–6. doi: 10.1038/nature12599
163. Hodin CM, Verdam FJ, Grootjans J, Rensen SS, Verheyen FK, Dejong CH, et al. Reduced paneth cell antimicrobial protein levels correlate with activation of the unfolded protein response in the gut of obese individuals. J Pathol. (2011) 225:276–84. doi: 10.1002/path.2917
164. Lee J-C, Lee H-Y, Kim TK, Kim M-S, Park YM, Kim J, et al. Obesogenic diet-induced gut barrier dysfunction and pathobiont expansion aggravate experimental colitis. PLOS ONE. (2017) 12:e0187515. doi: 10.1371/journal.pone.0187515
165. Takakuwa A, Nakamura K, Kikuchi M, Sugimoto R, Ohira S, Yokoi Y, et al. Butyric acid and leucine induce α-defensin secretion from small intestinal paneth cells. Nutrients. (2019) 11:2817. doi: 10.3390/nu11112817
166. Monk JM, Zhang CP, Wu W, Zarepoor L, Lu JT, Liu R, et al. White and dark kidney beans reduce colonic mucosal damage and inflammation in response to dextran sodium sulfate. J Nutr Biochem. (2015) 26:752–60. doi: 10.1016/j.jnutbio.2015.02.003
167. Bentley-Hewitt KL, Blatchford PA, Parkar SG, Ansell J, Pernthaner A. Digested and fermented green kiwifruit increases human β-defensin 1 and 2 production in vitro. Plant Foods Hum Nutr. (2012) 67:208–14. doi: 10.1007/s11130-012-0305-1
168. Hodin CM, Lenaerts K, Grootjans J, de Haan JJ, Hadfoune M, Verheyen FK, et al. Starvation compromises paneth cells. Am J Pathol. (2011) 179:2885–93. doi: 10.1016/j.ajpath.2011.08.030
169. Hodin CM, Visschers RGJ, Rensen SS, Boonen B, Damink SWMO, Lenaerts K, et al. Total parenteral nutrition induces a shift in the firmicutes to bacteroidetes ratio in association with paneth cell activation in rats. J Nutr. (2012) 142:2141–7. doi: 10.3945/jn.112.162388
170. Talbot J, Hahn P, Kroehling L, Nguyen H, Li D, Littman DR. Feeding-dependent VIP neuron-ILC3 circuit regulates the intestinal barrier. Nature. (2020) 579:575–80. doi: 10.1038/s41586-020-2039-9
171. MacFie J, Reddy BS, Gatt M, Jain PK, Sowdi R, Mitchell CJ. Bacterial translocation studied in 927 patients over 13 years. BJS Br J Surg. (2006) 93:87–93. doi: 10.1002/bjs.5184
172. Tomas J, Mulet C, Saffarian A, Cavin J-B, Ducroc R, Regnault B, et al. High-fat diet modifies the PPAR-γ pathway leading to disruption of microbial and physiological ecosystem in murine small intestine. Proc Natl Acad Sci USA. (2016) 113:E5934–43. doi: 10.1073/pnas.1612559113
173. Su D, Nie Y, Zhu A, Chen Z, Wu P, Zhang L, et al. Vitamin D signaling through induction of paneth cell defensins maintains gut microbiota and improves metabolic disorders and hepatic steatosis in animal models. Front Physiol. (2016) 7:498. doi: 10.3389/fphys.2016.00498
174. Srugo SA, Bloise E, Nguyen TT-TN, Connor KL. Impact of maternal malnutrition on gut barrier defense: implications for pregnancy health and fetal development. Nutrients. (2019) 11:1375. doi: 10.3390/nu11061375
175. Everard A, Lazarevic V, Gaïa N, Johansson M, Ståhlman M, Backhed F, et al. Microbiome of prebiotic-treated mice reveals novel targets involved in host response during obesity. ISME J. (2014) 8:2116–30. doi: 10.1038/ismej.2014.45
176. Andres SF, Santoro MA, Mah AT, Keku JA, Bortvedt AE, Blue RE, et al. Deletion of intestinal epithelial insulin receptor attenuates high-fat diet-induced elevations in cholesterol and stem, enteroendocrine, and paneth cell mRNAs. Am J Physiol-Gastrointest Liver Physiol. (2015) 308:G100–11. doi: 10.1152/ajpgi.00287.2014
177. Guo X, Li J, Tang R, Zhang G, Zeng H, Wood RJ, et al. High fat diet alters gut microbiota and the expression of paneth cell-antimicrobial peptides preceding changes of circulating inflammatory cytokines. Mediat Inflamm. (2017) 2017:1–9. doi: 10.1155/2017/9474896
178. Guo X, Tang R, Yang S, Lu Y, Luo J, Liu Z. Rutin and its combination with inulin attenuate gut dysbiosis, the inflammatory status and endoplasmic reticulum stress in paneth cells of obese mice induced by high-fat diet. Front Microbiol. (2018) 9:2651. doi: 10.3389/fmicb.2018.02651
179. Huang C, Chen J, Wang J, Zhou H, Lu Y, Lou L, et al. Dysbiosis of intestinal microbiota and decreased antimicrobial peptide level in paneth cells during hypertriglyceridemia-related acute necrotizing pancreatitis in rats. Front Microbiol. (2017) 8:776. doi: 10.3389/fmicb.2017.00776
180. Erbay E, Babaev VR, Mayers JR, Makowski L, Charles KN, Snitow M, et al. Reducing endoplasmic reticulum stress through a macrophage lipid chaperone alleviates atherosclerosis. Nat Med. (2009) 15:1383–91. doi: 10.1038/nm.2067
181. Beyaz S, Mana MD, Roper J, Kedrin D, Saadatpour A, Hong S-J, et al. High-fat diet enhances stemness and tumorigenicity of intestinal progenitors. Nature. (2016) 531:53–8. doi: 10.1038/nature17173
182. Peyrin-Biroulet L, Beisner J, Wang G, Nuding S, Oommen ST, Kelly D, et al. Peroxisome proliferator-activated receptor gamma activation is required for maintenance of innate antimicrobial immunity in the colon. Proc Natl Acad Sci USA. (2010) 107:8772–7. doi: 10.1073/pnas.0905745107
183. Wahli W, Michalik L. PPARs at the crossroads of lipid signaling and inflammation. Trends Endocrinol Metab. (2012) 23:351–63. doi: 10.1016/j.tem.2012.05.001
184. Kong J, Zhang Z, Musch MW, Ning G, Sun J, Hart J, et al. Novel role of the vitamin D receptor in maintaining the integrity of the intestinal mucosal barrier. Am J Physiol-Gastrointest Liver Physiol. (2008) 294:G208–16. doi: 10.1152/ajpgi.00398.2007
185. Cantorna MT, Lin Y-D, Arora J, Bora S, Tian Y, Nichols RG, et al. Vitamin D regulates the microbiota to control the numbers of RORγt/FoxP3+ regulatory t cells in the colon. Front Immunol. (2019) 10:1772. doi: 10.3389/fimmu.2019.01772
186. Tremblay S, Romain G, Roux M, Chen X-L, Brown K, Gibson DL, et al. Bile acid administration elicits an intestinal antimicrobial program and reduces the bacterial burden in two mouse models of enteric infection. Infect Immun. (2017) 85:e00942–16. doi: 10.1128/IAI.00942-16
187. Boman HG. Innate immunity and the normal microflora. Immunol Rev. (2000) 173:5–16. doi: 10.1034/j.1600-065X.2000.917301.x
188. Round JL, Mazmanian SK. The gut microbiome shapes intestinal immune responses during health and disease. Nat Rev Immunol. (2009) 9:313–23. doi: 10.1038/nri2515
189. Reikvam DH, Erofeev A, Sandvik A, Grcic V, Jahnsen FL, Gaustad P, et al. Depletion of murine intestinal microbiota: effects on gut mucosa and epithelial gene expression. PLoS ONE. (2011) 6:e17996. doi: 10.1371/journal.pone.0017996
190. Fan D, Coughlin LA, Neubauer MM, Kim J, Kim MS, Zhan X, et al. Activation of HIF-1α and LL-37 by commensal bacteria inhibits Candida albicans colonization. Nat Med. (2015) 21:808–14. doi: 10.1038/nm.3871
191. Peyssonnaux C, Datta V, Cramer T, Doedens A, Theodorakis EA, Gallo RL, et al. HIF-1α expression regulates the bactericidal capacity of phagocytes. J Clin Invest. (2005) 115:1806–15. doi: 10.1172/JCI23865
192. Kelly CJ, Glover LE, Campbell EL, Kominsky DJ, Ehrentraut SF, Bowers BE, et al. Fundamental role for HIF-1α in constitutive expression of human β defensin-1. Mucosal Immunol. (2013) 6:1110–8. doi: 10.1038/mi.2013.6
193. Everard A, Belzer C, Geurts L, Ouwerkerk JP, Druart C, Bindels LB, et al. Cross-talk between Akkermansia muciniphila and intestinal epithelium controls diet-induced obesity. Proc Natl Acad Sci USA. (2013) 110:9066–71. doi: 10.1073/pnas.1219451110
194. Natividad JMM, Hayes CL, Motta J-P, Jury J, Galipeau HJ, Philip V, et al. Differential induction of antimicrobial regiii by the intestinal microbiota and Bifidobacterium breve NCC2950. Appl Environ Microbiol. (2013) 79:7745–54. doi: 10.1128/AEM.02470-13
195. Cazorla SI, Maldonado-Galdeano C, Weill R, De Paula J, Perdigón GDV. Oral administration of probiotics increases paneth cells and intestinal antimicrobial activity. Front Microbiol. (2018) 9:736. doi: 10.3389/fmicb.2018.00736
196. Wehkamp J, Harder J, Wehkamp K, Meissner BW, Schlee M, Enders C, et al. NF-κB- and AP-1-Mediated Induction of human beta defensin-2 in intestinal epithelial cells by Escherichia coli nissle 1917: a novel effect of a probiotic bacterium. Infect Immun. (2004) 72:5750–8. doi: 10.1128/IAI.72.10.5750-5758.2004
197. Schlee M, Wehkamp J, Altenhoefer A, Oelschlaeger TA, Stange EF, Fellermann K. Induction of human β-defensin 2 by the probiotic Escherichia coli nissle 1917 is mediated through flagellin. Infect Immun. (2007) 75:2399–407. doi: 10.1128/IAI.01563-06
198. Sugi Y, Takahashi K, Kurihara K, Nakano K, Kobayakawa T, Nakata K, et al. α-Defensin 5 gene expression is regulated by gut microbial metabolites. Biosci Biotechnol Biochem. (2017) 81:242–8. doi: 10.1080/09168451.2016.1246175
199. Roager HM, Licht TR. Microbial tryptophan catabolites in health and disease. Nat Commun. (2018) 9:3294. doi: 10.1038/s41467-018-05470-4
200. Levy M, Blacher E, Elinav E. Microbiome, metabolites and host immunity. Curr Opin Microbiol. (2017) 35:8–15. doi: 10.1016/j.mib.2016.10.003
201. Walker AW, Duncan SH, Leitch ECM, Child MW, Flint HJ. pH and peptide supply can radically alter bacterial populations and short-chain fatty acid ratios within microbial communities from the human colon. Appl Environ Microbiol. (2005) 71:3692–700. doi: 10.1128/AEM.71.7.3692-3700.2005
202. Duncan SH, Louis P, Thomson JM, Flint HJ. The role of pH in determining the species composition of the human colonic microbiota. Environ Microbiol. (2009) 11:2112–22. doi: 10.1111/j.1462-2920.2009.01931.x
Keywords: antimicrobial peptides, defensins, microbiota, diet, prebiotics and probiotics, high-fat diet, intestinal barrier function, gut bacteria
Citation: Puértolas-Balint F and Schroeder BO (2020) Does an Apple a Day Also Keep the Microbes Away? The Interplay Between Diet, Microbiota, and Host Defense Peptides at the Intestinal Mucosal Barrier. Front. Immunol. 11:1164. doi: 10.3389/fimmu.2020.01164
Received: 28 February 2020; Accepted: 12 May 2020;
Published: 09 June 2020.
Edited by:
Mark Hulett, La Trobe University, AustraliaReviewed by:
Maryam Dadar, Razi Vaccine and Serum Research Institute, IranAmy Alexandra Baxter, La Trobe University, Australia
Copyright © 2020 Puértolas-Balint and Schroeder. This is an open-access article distributed under the terms of the Creative Commons Attribution License (CC BY). The use, distribution or reproduction in other forums is permitted, provided the original author(s) and the copyright owner(s) are credited and that the original publication in this journal is cited, in accordance with accepted academic practice. No use, distribution or reproduction is permitted which does not comply with these terms.
*Correspondence: Bjoern O. Schroeder, Ympvcm4uc2Nocm9kZXJAdW11LnNl