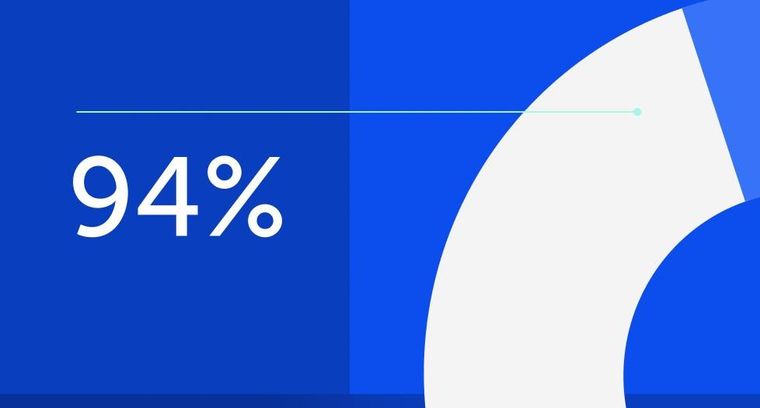
94% of researchers rate our articles as excellent or good
Learn more about the work of our research integrity team to safeguard the quality of each article we publish.
Find out more
REVIEW article
Front. Immunol., 09 June 2020
Sec. T Cell Biology
Volume 11 - 2020 | https://doi.org/10.3389/fimmu.2020.01090
The glycoprotein CD2 is a costimulatory receptor expressed mainly on T and NK cells that binds to LFA3, a cell surface protein expressed on e.g., antigen-presenting cells. CD2 has an important role in the formation and organization of the immunological synapse that is formed between T cells and antigen-presenting cells upon cell-cell conjugation and associated intracellular signaling. CD2 expression is upregulated on memory T cells as well as activated T cells and plays an important role in activation of memory T cells despite the coexistence of several other costimulatory pathways. Anti-CD2 monoclonal antibodies have been shown to induce immune modulatory effects in vitro and clinical studies have proven the safety and efficacy of CD2-targeting biologics. Investigators have highlighted that the lack of attention to the CD2/LFA3 costimulatory pathway is a missed opportunity. Overall, CD2 is an attractive target for monoclonal antibodies intended for treatment of pathologies characterized by undesired T cell activation and offers an avenue to more selectively target memory T cells while favoring immune regulation.
Costimulation is needed in addition to antigen-specific signaling through the T cell receptor CD3 complex (TCR/CD3) to achieve full T cell activation. Stimulation of T cells through TCR/CD3 in the absence of costimulation has been shown to induce anergy (1–3). An additional way to induce T cell activation and differentiation is via cytokines that bind corresponding receptors on the surface of T cells (4, 5). Recent decades have seen the development of biologics which target costimulatory cell surface molecules and thereby induce immune modulatory effects (6–8). Interestingly, blockade of a single costimulatory pathway with these biologics induced immune modulatory effects despite multiple costimulatory pathways working in tandem during T cell co-stimulation (9). This suggests that certain costimulatory pathways may have unique functions which cannot be compensated for by other pathways involved in co-stimulation. Therefore, blockade of individual costimulatory pathways has the potential to induce unique immune modulatory effects and intentionally direct T cell activation toward a desired outcome.
CD2 is a transmembrane glycoprotein of the immunoglobulin superfamily expressed on the surface of T cells, NK cells, thymocytes and dendritic cells (10, 11). The corresponding binding partner of CD2 is lymphocyte-associated antigen 3 (LFA3; also known as CD58) which is expressed on the surface of B cells, T cells, monocytes, granulocytes, thymic epithelial cells (10, 12). In addition, CD2 also binds to CD48, albeit with a relatively lower affinity (13). Although CD2 has been known for several decades to participate in a costimulatory pathway of T cell activation, studies of other costimulatory pathways with effects of greater magnitude in mice, have, until now, received considerably more attention by immunologists.
The CD2 antigen was identified as one of the earliest T cell markers (14, 15) and was shown to be important for thymocyte development (16). However, research was complicated by the fact that CD2 may not have the same role in murine immunity as in the immune system of higher animals. Notably, only a minor percentage of B cells express CD2 in humans (17) while CD2 is broadly expressed on murine B cells (18). Further, mice lack expression of LFA3 which is the main binding partner of CD2 in humans. Mice express CD48, however CD48 has a relatively lower affinity for CD2 and can bind to both CD2 and CD244 (13). Thus, the degree to which data about the role of CD2 in murine immunity can be extrapolated to a human context is uncertain and must be practiced cautiously. This difference made development of suitable animal models for research of CD2-targeting therapies resource-intensive as only transgenic rodents and primates were relevant pre-clinical models. Consequently, most research about CD2 immunobiology was conducted in vitro. However, the high degree of conservation of CD2 in rodents and higher mammals suggests an important role in all organisms as conservation without selective pressure is highly unlikely (19).
Recent clinical studies have shown the importance of this pathway for in T cell activation, especially in the transplantation setting. We have therefore considered it appropriate and timely to undertake the present review of the CD2 immunobiology, overlooking of which would represent a lost opportunity (9). Further, we consider potential therapeutic applications of CD2-targeting biologics.
Human CD2 consists of 327 amino acids (351 amino acids including signal sequence) and a protein weight of ~40 kDa. References to specific amino acids throughout this paper refer to the position in the sequence of human CD2 including the signal peptide [Uniprot ID P06729; (20)]. Fully-glycosylated CD2 can weigh up to 50 kDa (21). The extracellular domain (ECD) of CD2 (amino acids 25–209) consists of two Ig domains, as shown in Figure 1A (22), and is linked to a disordered proline-rich cytoplasmic tail via a transmembrane helix. The membrane-proximal domain of the CD2 ECD connects the adhesion (membrane-distal) domain to the cell membrane and may be involved in CD2 clustering following T cell activation (27, 28). The membrane-distal domain is responsible for LFA3 binding. The binding surface consists of a flat beta sheet with relatively poor surface complementarity to the adhesion domain of LFA3. Interaction of CD2 with LFA3 is mainly mediated by polar interactions, as illustrated in Figure 1B. The ECD of CD2 has three glycosylation sites (N89, N141, and N150) with one being situated in the membrane-distal domain (N89) and two in the membrane-proximal domain [N141 and N151; (29)]. In folded CD2, N89 is situated near several amino acids which are important for siplizumab binding [N42, K79, and T83; (26)] thus the N89-linked glycan may be part of siplizumab's binding site.
Figure 1. (A) Model of CD2 (green; Protein data bank code: PDB 1HNF) binding to LFA3 (blue; PDB 1CCZ). Model was created by structural alignment of PDB 1HNF [2.5 Å; (22)] and PDB 1CCZ [1.8 Å; (23)] with an X-ray crystallography structure of the adhesion domains of CD2 and LFA3 in their bound state [3.2 Å; PDB 1QA9; (24)] using PyMOLTM 2.3.2 software (Schrodinger LLC, New York). The extracellular domain (ECD) of CD2 consists of a membrane-distal and a membrane-proximal Ig domain which are connected by a flexible linker region. The membrane-proximal region is linked to a transmembrane helix while the membrane-distal domain binds to LFA3. The most well-characterized CD2 epitopes are T11.1 (orange), T11.2 (gray) and T11.3 (purple), as identified by Peterson and Seed (25) and Damschroder et al. (26). CD2 undergoes a conformational change upon T cell activation and/or LFA3 binding which exposes an epitope called CD2R. CD2R residues as identified by Li et al. (27) are shown in yellow. The distance between T cell and APC upon CD2-LFA3 association according to this model would be approximately 130 Ångström. (B) CD2 (green) and LFA3 (blue) adhesion domains (PDB 1QA9) mainly associate via polar interactions (dotted yellow lines) such as hydrogen bonds and salt bridges. Amino acids involved in polar interactions between CD2 and LFA3 are shown as sticks, the remainders of both chains are shown in ribbon representation.
There exist three well-characterized CD2 epitopes: T11.1, T11.2, and T11.3 (25, 30). T11.1 and T11.2 are located in the adhesion domain and are involved in LFA3 binding (16). T11.3 is part of the membrane-proximal domain (25) and exposure of this epitope increases upon T cell activation or CD2 clustering (27). T11.3 overlaps with another CD2 epitope named CD2R. The name of CD2R derives from its exposure being restricted to certain conformational states of CD2. While the T11.3 residues identified by Peterson and Seed (25) are not identical to the residues of CD2R as identified by Li et al. (27), both are located in the same region of CD2, namely in the membrane-proximal domain and the flexible linker region. The narrow distance between cells created by CD2-LFA3 as modeled in Figure 1A is approximately 130 Å, similar to the distance created by TCR bound to peptide-loaded major histocompatibility complex (TCR-pMHC) and CD4-MHC (31). This is essential for the kinetic segregation of activating and inhibiting membrane molecules upon formation of the immunological synapse [IS; (32)]. Presumably, siplizumab binds to T11.2 and T11.3 while Alefacept binds to T11.1 and T11.2 like LFA3. Alefacept, but not siplizumab, compete for CD2 binding with LFA3 (26).
Characteristic of cell-cell adhesion proteins, CD2 and LFA3 interact with relatively low affinity for each other (Kd = 9–22 μM) and a fast dissociation rate (33). Similar observations have been made for CD28/CD80 [Kd = 4 μM; (34)], LFA-1/ICAM-1 [Kd = 0.5 μM; (35)], CD2/CD48 [Kd = 100 μM; (36)] and CD40/CD40L [Kd = 0.2 μM; (37)]. A relatively low affinity and a high dissociation rate may facilitate cellular scanning but in the absence of further specific interactions of e.g., TCR with p-MHC (T cells) or of CD16 with an Fc-fragment (NK cells) no stable IS will be formed. Even though costimulatory molecules have a relatively low affinity for each other, association of thousands of costimulatory membrane molecules may still result in considerable cell-cell avidity. Furthermore, both CD2/LFA3 affinity and LFA1/ICAM1 affinity increase following cellular activation, providing another mechanism by which cell-cell avidity can be increased upon specific cell-cell conjugation (38–40).
The proline-rich intracellular domain (ICD) of CD2 is highly conserved across mammalian species. Considering how much time has passed since their divergence from a common ancestor, this degree of conservation indicates that CD2 fulfills an important function in mammalian immunity (19). As illustrated in Figure 2, the intracellular tail of human CD2 contains five potential src homology 3 domain (SH3)-binding sites [PxxP or PxxxP; (49, 50)], of which the first and second can also function as glycine-tyrosine-phenylalanine (GYF)-binding sites [PPPPGHR; (41, 51)]. The cytoplasmic tail of CD2 has been shown to interact with fyn kinase and lck kinase (41–43), CD2-binding protein 1 [CD2BP1; (52)], CD2BP2 (41, 49, 51), Cbl-interacting protein of 85 kDa [CIN85; (45, 53)], CD2BP3 [Variant of CIN85; (52)], and Cas ligand with multiple SH3 domains (CMS, also called CD2 adaptor protein or CD2AP). Further, while no direct interaction has been proven, CD2 has been reported to precipitate with TCR/CD3 and PI3K (47, 48).
Figure 2. Schematic illustration of adaptor molecules binding to the intracellular tail domain (ICD) of human CD2 (Green). The ICD of CD2 is connected to the extracellular domain (ECD) of CD2 via a transmembrane helix in the cell membrane (red). The ICD of CD2 contains five SH3 binding domains (Two proline residues separated by two or three amino acids and flanked by a basic amino acid residue; #1–#5), of which two can also act as GYF-binding motifs (#1 and #2). Fyn kinase and CD2-binding protein 2 (CD2BP2) have been shown to bind to #1 and #2 (41). Additionally, Fyn kinase binds to #4 and/or #5 (42). Lck kinase has been shown to interact with #1, #2 and #4 in rat CD2 ICD (43). CD2BP1, CD2BP3, Cbl-interacting protein of 85 kDa (CIN85) and Cas ligand with multiple SH3 domains (CMS; Human form of CD2-associated protein CD2AP) bind to #4 (44, 45). The C-terminal amino acid N327 has been shown to be essential for CD2-LFA3 affinity regulation but no binding partner has been identified (38, 46). The ICD of CD2 precipitated with the T cell receptor complex (TCR/CD3) and Phosphatidylinositol-3 kinase (PI3K) but no direct interaction and binding motif has been identified (47, 48). Further direct interactions between the cytoplasmic tail of CD2 and intracellular or membrane proteins may exist but have yet to be identified.
Intracellularly, CD2 influences rearrangement of the actin cytoskeleton and agonistic cell signaling. These two functions seem to be spatially separated as a fraction of CD2 molecules transitions to lipid rafts in the cell membrane upon cell activation where it co-localizes with other signaling molecules important in transducing TCR/CD3- and costimulatory receptor-mediated signaling, e.g., activated protein tyrosine kinases (41, 54, 55). In contrast, molecules associated with connecting CD2 and the actin cytoskeleton remain outside of lipid rafts (41, 52).
Lateral mobility of CD2 in the plasma membrane of T cells has been shown to decrease following activation (39, 56, 57). Immobilization of CD2 is likely caused by crosslinking of the cytoplasmic tail with the actin cytoskeleton (56). As depicted in Figure 2, CMS, CIN85, and CD2BP3 compete for binding to the fourth SH3-binding domain in the cytoplasmic tail of CD2 (52). CMS and CIN85 connect CD2 to the actin cytoskeleton in several ways. Both have been shown to associate with the actin capping protein capZ (45, 58) and adaptor protein p130Cas (53, 59). Further, CMS was reported to associate with the actin regulatory protein cortactin (60) and directly with F-actin (61). Interestingly, Tibaldi and Reinherz (52) showed that degradation of CIN85 and CD2BP3 increases following T cell activation and that CIN85 inhibits cell polarization while CD2BP3 does not seem to influence cell polarization. Given that CMS binding to CD2 seems activation-dependent (42), a model was proposed where CIN85 and CD2BP3 occupy SH3-binding domain 4 in the cytoplasmic tail of CD2 in resting T cells while their degradation frees up this site for binding by CMS upon cellular activation. Consequently, reorganization of the actin cytoskeleton and polarization of the microtubule organizing center (MTOC) toward the IS can occur. Indeed, CD2 and CMS facilitate polarization of the actin cytoskeleton and T cells expressing truncated CD2 mutants lacking the C-terminal 20 amino acids of the cytoplasmic tail do not display polarization toward the IS (42, 62). Furthermore, CD2BP1 also binds SH3-binding domain 4 and locates to the IS upon cell activation where it links CD2 or CMS and Wiskott-Aldrich syndrome protein (WASp) to facilitate actin cytoskeleton rearrangement (54).
The TCR/CD3 complex, particularly the CD3 zeta chain is involved in transducing CD2-mediated activation signaling (28, 63–66). However, no direct association between CD2 and TCR/CD3 has been proven, even though they have been reported to co-immunoprecipitate (47). Considering the evidence discussed above, co-localization of CD2 and TCR/CD3 may, at least in part, be mediated through adaptor proteins and the actin cytoskeleton. Future research should investigate the interaction of CD2 and the actin cytoskeleton through adaptor proteins more closely, as it seems to play an important role in IS formation (28) and transport of lytic granules [CTLs; (62)]. Another unanswered question is whether CD2 similarly influences transport of secretory cytokines.
The network of CD2-mediated activation signaling overlaps partly with TCR/CD3-mediated signaling and is dependent on protein tyrosine kinases like lck and fyn (55, 67, 68, 70). Indeed, at least part of CD2-mediated signaling seems to depend on CD3ζ expression (28, 65, 66). Similar to TCR/CD3, CD2 elicits activation signaling through the lck/CD3ζ/ZAP70/LAT/SLP76/Ras-MAPK pathway (28, 43, 69, 70). Additionally, CD2 can induce activation signaling via the PLCγ1/calmodulin/calcineurin/NFAT and PLCγ1/Vav1/PKCθ/Dok/FAK/Pyk2/JNK axes (57, 68, 71). Preliminary evidence analyzing the kinome of CD2-mediated signaling in CTLs confirmed these findings and elucidated a broad network of CD2-induced signaling which influences IS assembly, cell polarization, T cell activation and metabolic regulation which overlapped only partially with known TCR/CD3- or CD28-mediated signaling pathways (62). Generally, CD2 costimulation has been shown to act additively with TCR/CD3- and CD28-mediated signaling and to induce strong mTOR signaling but weak NF-κB activity when compared to CD28 costimulation (62, 69, 71, 72). While the majority of the literature investigating CD2 signaling has been conducted in T cells, there are indications that CD2-mediated signaling in NK cells utilizes the same pathways as T cells (73–75).
The role of CD2BP2 in CD2-mediated signaling is largely unexplored. CD2BP2 binds proline rich regions one and two in the cytoplasmic tail of CD2. Evidence surrounding the role of CD2BP2 in CD2-mediated signaling suggests that it specifically enhances CD2-mediated activation signaling but not TCR/CD3-mediated signaling (36). The nature of how CD2BP2 acts in intracellular signaling is poorly.
Even though costimulatory signaling pathways were originally assumed to be largely redundant, there are differences in the quantity and quality of signaling that is elicited. Specifically, blockade of CD2/LFA3 co-stimulation may have distinct effects on cell metabolism and activation distinct from those observed in e.g., CD28/B7 co-stimulatory blockade.
CD2 is an important component in assembly of the IS upon T cell-APC conjugation (19, 32). The IS forms in the contact zone between T cells and APCs upon stable cell-cell interaction and consists of supramolecular activation clusters (SMACs). As shown in Figure 3, the central SMAC (cSMAC) is surrounded by the peripheral SMAC (pSMAC), which in turn is encircled by the distal SMAC (dSMAC). Together, they form a bull's eye pattern at the interface between T cells and APCs (76). CD2 is enriched in the IS upon T cell-APC conjugation (77) and appears to play an important role for IS organization (78). It is currently unclear how CD2 affects the location of other membrane molecules in the IS. This effect may be indirectly mediated via the influence of CD2 on actin cytoskeleton rearrangement. IS formation enables accumulation of agonistic signaling molecules at the T-cell-APC interface while excluding membrane molecules that downregulate T cell activation signaling, e.g., CD45, from the center of the IS (32, 78, 79). The relatively short distance between T cells and APCs created by CD2-LFA3 interaction, along with other costimulatory molecules, forms the basis of the kinetic segregation theory (32). This theory describes a model of T cell activation whereby the close contacts formed between T cells and APCs sterically exclude membrane-bound phosphatases with large ECDs (e.g., CD45) from cSMAC and pSMAC. Thus, phosphatases, which might otherwise counteract the relatively high baseline activity of intracellular kinases involved in immunoreceptor tyrosine-based activation motif (ITAM) phosphorylation are sterically excluded from the IS. Consequently, phosphorylation of ITAM domains on the intracellular side of the T cell membrane crosses a threshold which results in T cell activation. For proper IS formation both co-stimulation and specific TCR-MHC binding are required. Some CD2/LFA3 complexes locate to cSMAC, together with other molecules such as CD28/CD80/86 and T cell receptor/peptide MHC (TCR/pMHC) complexes (80). Further, preliminary evidence suggests that clusters of CD2/LFA3 complexes form a ring-like structure between dSMAC and pSMAC termed “corolla” (81).
Figure 3. Schematic illustration of the immunological synapse and spatial distribution of TCR/MHC and costimulatory molecules. Illustration is for explanatory purposes and relative sizes of different molecules are not necessarily to scale. Regions include central supramolecular activation cluster (cSMAC), peripheral SMAC (pSMAC), CD2/LFA3 corolla and distal SMAC (dSMAC). CD2 (green) is positioned in the T cell plasma membrane (light red) and locates to both the cSMAC and corolla. CD2 binds to lymphocyte-associated antigen 3 (LFA3; dark blue) which is located in the plasma membrane of the antigen-presenting cell (light blue). Among other molecules, TCR/pMHC and CD28/CD80/86 complexes also locate to the cSMAC. LFA-1/ICAM-1 complexes predominantly locate to the pSMAC. See main text for references.
It has been observed repeatedly that CD2, along with other molecules of the T cell signaling machinery, organizes into microdomains in the IS (28, 82). Upon complete IS formation and given the presence of LFA3, CD2 microclusters tend to reside in the periphery of the IS and it may be speculated that this clustering results from a combination of CD2 translocating to lipid rafts and clustering of CD2 molecules via the ECD of CD2 upon CD2R exposure. As mentioned above, upon cell activation a fraction of CD2 transitions to lipid rafts which are enriched in src family kinases, LAT and components of the T cell signaling machinery but do not contain proteins that connect CD2 and the actin cytoskeleton. It has been shown that clustering of CD2 in the T cell membrane can occur in the absence of the ICD of CD2 (28), possibly mediated by the CD2R epitope (27). However, preliminary evidence indicates that expression of the cytoplasmic tail of CD2 is required for corolla formation (81). A potential explanation for this phenomenon might be that while components of the IS are usually pulled toward cSMAC via centripetal actin-mediated pulling forces, clustered CD2 in lipid rafts may resist this pull more effectively than other IS components as it is not extensively cross-linked with the actin cytoskeleton. This is confirmed by reports that association of CD2 with lck and fyn is required for translocation of CD2 to lipid rafts upon activation (55). This hypothesis would agree with observations that new TCR/CD3 complexes entering the IS emanate from CD2 microdomains in the corolla in the periphery of the IS (81). Following this path of reasoning, CD2 not translocating to lipid rafts would be more extensively linked to the actin cytoskeleton and get pulled into cSMAC. Future research should aim to elucidate what mechanisms underlie the binary positioning of CD2 to the corolla or cSMAC.
Even before the function or ligand of CD2 was known, its upregulation during the early stages of thymic selection suggested a role in T cell development (83–85). Antibodies to either CD2 or LFA-3 inhibited the binding of thymocytes to thymic epithelial cells (16, 86), revealing involvement of this interaction during thymocyte ontogeny. Furthermore, characterizations of mouse fetal thymocytes reported initial expression of CD2 around day 14 of gestation, followed by an increase to adult levels by day 19 (87–90), whereas CD2 expression in humans has been observed at 9.5 week of gestation (14, 91). Early studies in the mouse thymus demonstrated a correlation between CD2 expression and thymocyte maturation. Namely, CD4− CD8− double-negative (DN) thymocytes expressed low levels, more differentiated CD4+ CD8+ double-positive (DP) thymocytes intermediate levels, whereas the majority of mature CD4– or CD8-single positive (SP) thymocytes expressed high levels of CD2 (92). Later studies confirmed that CD2 is expressed during late stages of DN maturation into DP thymocytes and maintained on all thymocytes at subsequent developmental stages and ongoing thymic selection events (93). Interestingly, during this pathway into TCR alpha beta T cells, CD2 expression is closely correlated with rearrangement of the TCR beta chain (93), with reports that TCR beta rearrangement stimulates normal CD2 surface expression in developmentally arrested DN thymocytes (94).
However, early studies utilizing CD2 knockout mouse models, or treatment with antibodies against murine CD2, observed normal immunological phenotypes (87, 89, 95), and undermined the view that CD2 plays a major role in T cell development. Although CD2 is not required for the positive selection of T cells, subsequent studies have revealed subtle defects in thymocyte differentiation in mutant mice. CD2-deficient mice displayed altered TCR repertoire selection by affecting Vα gene segment usage in mature T lymphocytes (96). Thus, CD2 can decide the outcome of thymic selection by likely modulating TCR/CD3 stimulation. Notably, tracking CD2-deficient TCR transgenic T cells revealed differential effects of CD2 on thymic selection predicated on the selecting ligand (96–98). On the one hand, CD2-deficiency in T cells bearing TCR with lower affinity for selecting ligand increased the efficiency of positive selection (97). On the other hand, positive selection in TCR transgenic mice with higher affinity for selecting ligand was not perturbed, despite increases in the activation state of DP progenitors in CD2-deficiency (97). Thus, while T cell development was not disengaged in either model, the effect of CD2 on selection was highly variable. Later studies utilizing separate TCR transgenic mouse models confirmed a role for CD2 in thymic selection (96, 98). Specifically, CD2-deficiency in class I-restricted TCR transgene resulted in a differentiation defect during the transition from CD25+ to CD25− CD44− DN immature thymocyte akin the ones observed in mice which lack critical components of pre-TCR signaling (99, 100). Similar experiments in class II-restricted TCR transgenic mice observed a decrease in DP and CD4+ SP thymocytes on a CD2-deficient background (98).
Overall, it is likely that CD2 plays a role at multiple stages of thymic development of T lymphocytes. In addition, these effects of CD2 seems to be dependent upon the associated TCR. While most studies utilizing a variety of CD2-deficient mouse models reported a reduction in the frequency of DP thymocytes (96–98, 101), the observations in regards to thymus cellularity or proportion of other immature thymocyte populations are less consistent. The exact mechanisms of how CD2 functions during thymic development are yet to be determined. One interpretation is that CD2 supports the weak affinity of the pre-TCR signaling in DN cells, by increasing thymocyte to epithelial cell adhesion and/or directly affecting intracellular signaling. Experiments utilizing human CD2 transgenic mice, suggest a key role for the cytoplasmic tail in exerting effects during thymocyte maturation (101). Furthermore, CD2 might affect TCR to peptide-MHC affinity during positive and negative selection allowing immature thymocytes bearing TCRs with relatively high affinities for pMHCs to escape negative selection when CD2 is absent/blocked. The results of increased positive selection upon CD2-deletion for a low-affinity model TCR are surprising, given the opposite role of CD2 in the periphery, however independent studies have made similar findings for other costimulatory molecules such as CD5 and CD28 (102, 103). Investigating possible differences in CD2 cross-talk with the (pre-)TCR complex, in mature T lymphocytes compared to developing thymocytes, might help to elucidate the role of CD2 further. Additionally, re-examining CD2-deficient mouse models through the lens of modern transcriptomics methods that can generate gene expression trajectories paired with single-cell resolution TCR sequencing will provide a more complete view of how CD2 affects T cell differentiation and repertoire generation (104).
T cells are highly motile and scan their environment with the aim of specific antigen recognition, a process involving remodeling of the actin cytoskeleton (105). CD2 has been shown to be enriched in the uropod of scanning T cells along with TCR/CD3 and lipid rafts (106). This indicates an important role of CD2 in APC scanning by T cells prior to IS formation and T cell activation. While the mechanism is yet to be elucidated, CD2 may facilitate formation of the “pre-IS” during probing of APCs by T cells through its influence on T cell signaling or actin cytoskeleton rearrangement. This may reduce the minimum required affinity of TCR/CD3 for pMHC to induce stable cell-cell conjugation and IS formation. A strain of HCMV has been shown to downregulate cell surface expression of LFA3 in host cells and thereby evade CTL cytotoxicity (107). The fact that a pathogen seems to have evolved to evade the CD2/LFA3 pathway underlines importance of this costimulatory pathway in human immunity.
The function of CD2 during activation of naïve T cells has been studied in vivo using anti-CD2 antibodies (108, 109), as well as CD2-deficient mouse models (96–98). Anti-CD2 antibody treatment during priming resulted in a reduced T cell response (108), while also protecting T cells from apoptosis upon stimulation by an overly strong TCR signal (109). Interestingly, administration of a single dose of anti-CD2 monoclonal antibody resulted in a sustained hyporesponsiveness of T cells for up to 4 weeks (108), however the mechanism behind this finding has not been elucidated. In addition, naïve CD2-deficient TCR transgenic T cells displayed diminished activation, proliferation, and IFN-γ production upon priming (96–98), however the magnitude of the effect of CD2-deficincy was dependent on the TCR signal intensity. Specifically, T cells expressing TCRs that bind antigen ligands with high affinity are less reliant on CD2 signaling to mount a full immune response. Thus, during primary T cell-mediated immune responses, CD2 is a positive regulator of TCR signaling intensity, and could be crucial in regulating responsiveness to weaker TCR agonists.
The expression of CD2 on memory T cells is higher than on naïve T cells in humans (7, 81, 110) and non-human primates (111, 112). Moreover, CD2 expression is upregulated on activated T cells relative to resting T cells (7, 39). While most costimulatory pathways seem to lose effectiveness in memory and exhausted T cells, CD2-LFA3 retains its ability to contribute to T cell activation (113). Additionally, CD2/LFA3 costimulation seems to play an important role in the reversal of T cell anergy. Whereas, T cells can lose expression of T11.3 upon anergy induction, they regain T11.3 expression only upon extended activation with IL-2 (3). This suggests a role of CD2/LFA3 costimulation in memory T cell activation. Nonetheless, CD2− murine models have shown that CD2 does not seem to be necessary for generation, activation and maintenance of memory T cells (114).
Like naïve T cells, Tregs tend to express lower levels of CD2 when compared to memory T cells in both humans (110) and non-human primates (112). A notable exception are activated Tregs, which display similar CD2 expression to memory T cells (110). The lower expression of CD2 suggests a decreased importance of CD2/LFA3 costimulation in Tregs relative to other T cell subpopulations. Lastly, binding of CD2 by some monoclonal antibodies has been reported to induce apoptosis, suggesting a potential role of CD2 in T cell survival (115, 116). The importance of CD2/LFA3 costimulation for the survival and activation of Tregs and other T cell subpopulations should be investigated more closely.
Recent in vitro evidence has shown that CD2/LFA3 costimulation plays an important role in the differentiation of human T cells co-cultured with activated keratinocytes. CD2/LFA3 CoB reduced Signal transducer and activator of transcription 1 (STAT1) signaling and interferon γ production in activated T cells leading to reduced differentiation to a T helper 1 (Th1) phenotype (117, 118). In contrast, differentiation to the Th17 phenotype following stimulation with activated keratinocytes was not affected. Notably, activated keratinocytes expressed LFA3 but did not express B7, while T cells found in psoriatic lesions expressed CD2 but not CD28 (118). These findings indicate that CD2/LFA3 costimulation has an important role in the generation of Th1 cells following T cell activation by non-professional APCs in the epidermis. Potential differences in the role of CD2/LFA3 costimulation in the activation of peripheral and tissue-resident T cells should be investigated further.
Besides having an important role in NK cell conjugation to target cells (119), CD2 has been shown to recruit CD16 to the NK cell immunological synapse (NKIS) in spontaneous (antibody-independent) NK cell cytotoxicity. CD16 (also known as Fc γ receptor III; FcγRIII) is well-known for its central role in antibody-mediated NK cell cytotoxicity (120), however it still co-localizes to the NKIS with CD2 during spontaneous NK cytotoxicity (121, 122). Similar to what has been observed in T cells, accumulation of CD2 in the NKIS is facilitated by actin cytoskeleton rearrangement and CD2 has a predominantly peripheral positioning in the NKIS (121). NK cells of patients homozygous for a mutation of L66 in CD16 displayed normal ADCC activity but impaired spontaneous cytotoxicity (122, 123). This seemed to derive from an interaction between CD2 and CD16 that leads both to co-localize to the NKIS. L66 in CD16 is important for this interaction and mutation of L66 abrogated interaction between CD2 and CD16. Cells expressing L66H CD16 also display lower CD2 expression than NK cells expressing wild-type CD16 (122). This interaction likely serves to increase the accumulation of activating membrane molecules in the NKIS and thus cross the threshold required for NK cell activation. Given that L66 is situated in the extracellular domain of CD16, CD2, and CD16 may interact via their extracellular domains (122). It may be the case that CD2 serves as a connector between CD16 and the actin cytoskeleton, facilitating repositioning to the NKIS. Thus, similar to its role in T cell-APC conjugation, CD2 has not only an adhesive function on NK cells but also actively recruits activating membrane molecules to the IS, which lowers the threshold for activation.
NKG2C is an activating NK cell receptor which reacts with human cytomegalovirus [HCMV; (124, 125)]. Liu et al. (126) found evidence that the adaptive NK cell response in seropositive HCMV patients involves the synergistic interaction between CD2 and CD16 as well as CD2 and NKG2C. Conversely, no synergistic interaction was observed in HCMV seronegative patients. CD2 ligation with CD16 raised IFN-γ and TNF production, increased activating signaling through CD16 and enhanced antibody-dependent responses (126). These results point toward an important role of CD2 in the functional response of adaptive NK cells. It is especially notable that interaction of CD2 and CD16 enhanced antibody-mediated responses in adaptive NK cells as this was not seen in conventional NK cells as described in the previous paragraph. Similar to T cells, the importance of CD2 may be increased in memory responses of the NK cell compartment (126, 127). Lastly, CD2 seems to synergize with NKG2D in spontaneous cytotoxicity of human NK cells against xenogeneic cells (128) and play an important role in nanotube formation between NK cells and target cell (129).
The general function of CD2 in NK cells and T cells seem similar, however the published literature on the function of CD2 in NK cells to date is limited. Future research should investigate the function of CD2 in NK cell activation more closely to determine similarities and differences in the function of CD2 in NK cells and T cells. Differences in the function of CD2 in T and NK cells would be especially important to consider for therapeutic applications.
Organ transplantation and certain types of autoimmune disorders, conditions characterized by excessive activation of T cells and NK cells, constitute the natural target for CD2-LFA3 CoB. In organ transplantation, CD2-LFA3 CoB could e.g., be used in induction therapy and maintenance immunosuppression to prolong graft survival and minimize the risk for allograft rejection. A substantial part of CD2-LFA3 CoB clinical studies have been conducted using depleting anti-CD2 mAbs which provides two MoAs: Depletion of CD2-expressing cells and CD2/LFA3 costimulatory blockade. There exists little evidence on direct immune modulation via CD2/LFA3 CoB in vivo. Research on non-depleting anti-CD2 agents should be conducted to elucidate the contribution of each MoA to the effects seen with depleting anti-CD2 agents.
Chavin et al. (130) showed that treatment with anti-CD2 mAb prolonged xenograft islet survival and cardiac allograft survival in mice. More recently, it was shown that treatment of transgenic mice expressing human CD2 in a transfer colitis model with anti-human CD2 mAb CB.219 reduced intestinal inflammation and prolonged survival (131). Interestingly, efficacy varied between different anti-CD2 mAbs with two other anti-human CD2 mAbs (Clones 35.1 or 8E5, respectively) showing markedly lower or no efficacy, depending on the assessed parameter. These findings suggest that differences in binding properties (i.e., epitope or affinity) may potentially influence the effect of CD2-LFA3 CoB. Further, addition of CD2-LFA3 CoB via the fusion protein Alefacept to a CoB-based regimen extended allograft survival in rhesus macaques and depleted peripheral effector memory T cells (111). It is difficult to deduce how much of the observed effects in these studies stem from CD2-LFA3 CoB and how much from depletion of CD2+ cells. In this regard, it should be noted that while anti-CD2 mAbs induced strong depletion of peripheral T cells (siplizumab or BTI-322 [the original rat IgG2b version of siplizumab]) in chimpanzees, only moderate T cell depletion was seen in secondary lymphoid tissue (132). Given incomplete depletion in secondary lymphoid tissue and assuming sufficient tissue distribution, CD2-LFA3 CoB could become relatively more important as an MoA of CD2-targeting biologics in lymph nodes and other tissues where depletion of CD2+ cells is milder than in peripheral blood. Notably, depletory anti-CD2 mAb treatment induced a relative enrichment of Tregs in cynomolgus macaques (112) and in mixed lymphocyte reactions using human peripheral blood mononuclear cells (110). The study conducted by Podesta et al. (110) is especially notable since it reported an enrichment of CD45RA− Tregs using a depletory anti-CD2 mAb. CD2 expression levels on CD45RA− Tregs tends to be similar to the expression level seen on memory T cells. These results indicate that there may be other mechanisms than depletion which contribute to Treg enrichment with anti-CD2 mAbs. The approach of combining depletion of disease-mediating cells and CoB of remaining or newly maturing T and NK cells opens an alternative view in the design of treatment strategies for pathologies characterized by an uncontrolled immune response.
Most clinical studies investigating CD2-LFA3 CoB have been conducted using two biologic drugs: (1) Alefacept, a fusion protein of the ECD of LFA3 and an IgG1 Fc-fragment; and (2) siplizumab (previously known as MEDI-507), a humanized anti-CD2 monoclonal IgG1 antibody. Alefacept has been clinically studied for treatment of psoriasis (133) and new-onset type I diabetes (134) and for use in transplant induction therapy (135, 136). Alefacept did not seem to elicit notable additional adverse events compared to control groups and induced selective (albeit modest) depletion of peripheral memory T cells. Similarly, siplizumab has been investigated for treatment of plaque psoriasis (137), transplant induction therapy (138, 139), stem cell transplantation (140), graft-vs.-host disease (141) and T cell malignancies (142). In addition, siplizumab has been used in a treatment protocol aimed at inducing renal allograft tolerance, in which kidney transplantation is augmented by donor bone marrow infusion, in order to induce transient mixed chimerism (143, 144). Donor-specific Tregs were found to be enriched in this cohort, possibly due to selective depletion by siplizumab (145, 146). The results of these studies have stimulated further development of siplizumab, in order to resume clinical efforts toward establishment of allograft tolerance and treatment of autoimmune conditions. Collectively, reported clinical data on anti-CD2 agents have shown that this is a safe treatment modality that can be used in a broad spectrum of patients. Interestingly, the CD2 activation epitope CD2R has been reported to be upregulated on T cells in the synovial fluid of rheumatoid arthritis (RA) patients and in the peripheral blood of patients with juvenile RA, systemic lupus erythematosus, ankylosing spondylitis, and Lyme disease (147). Thus, therapeutic anti-CD2R/T11.3 mAbs could potentially be used to more specifically target autoreactive T cells in these conditions. Lastly, Tregs isolated from the peripheral blood of multiple sclerosis patients tended to exhibit reduced suppressive function relative to Tregs isolated from heathy controls (148). CD2/LFA3 CoB may be able to augment peripheral Treg-mediated suppression of autoreactive T cells in these patients and thus promote peripheral tolerance. Clinical trials in the discussed autoimmune conditions are needed to evaluate the efficacy of CD2-targeting therapies in these patient groups.
To date, most clinical trials of CD2-LFA3 CoB have focused on peripheral T (and NK) cells, with limited attention being paid to depletion and/or CD2/LFA3 CoB of tissue-resident T and NK cells. Data about tissue-resident NK cells, e.g., in lymph nodes, and their response to CD2-LFA3 CoB may be especially interesting as the majority of lymph node NK cells have a CD56bright phenotype (149) which has been shown to express CD2 at markedly higher levels than CD56dim NK cells (150), the most common phenotype in peripheral blood. NK cell count in peripheral blood seems decreased in many autoimmune conditions. However, there seems to be a concurrent enrichment of NK cells in tissues affected by autoimmune disease, suggesting an increased migration from peripheral blood to tissue (151). CD56bright NK cells have been shown to have relatively little cytotoxic capacity but a relatively high cytokine secretion potential (149). CD2-LFA3 CoB treatments enabling more selective depletion and inhibition of T (and NK) cells could be desirable in a variety of clinical settings. Commercially available depletory agents such as anti-thymocyte globulin (ATG) and Alemtuzumab bind other cell types in addition to T and NK cells and accordingly do not allow for selective depletion or inhibition of T and NK cells. Use of CD2 as a target for depletion and/or CoB may enable a reduction in off-target effects compared to currently available depletory agents. Inhibition of spontaneous NK cell cytotoxicity by blocking the association of CD2 and CD16 and interfering with NK-target cell conjugation via the CD2-LFA3 pathway through anti-CD2 mAbs could be used in prevention or treatment of transplant rejection as well for treatment of autoimmune conditions deriving in part from spontaneous NK cell cytotoxicity. Effectiveness of inhibiting NK cell activation via CD2-LFA3 CoB in these conditions likely depends on the mode of NK cell activation inherent to the disease. If NK cell activation is triggered via autoantibodies the potential of CD2-LFA3 CoB is likely low because CD2-LFA3 co-stimulation does not seem important for ADCC (122). However, if NK cell activation is spontaneous CD2-LFA3 CoB may have an inhibitory effect, especially if association of CD2 and CD16 is prevented in addition to CD2-LFA3 binding.
Patients with CD2+ lymphomas may benefit from depletory anti-CD2 treatments. Administration of siplizumab to NOD/SCID mice inoculated with adult T cell leukemia prolonged survival given expression of intact Fcγ receptors (152). A phase I study of siplizumab added to chemotherapy for treatment of patients with peripheral T cell lymphoma resulted in 84.5% overall response rate (OR), with 62% of patients achieving complete remission (CR). However, long-term progression-free survival was not improved (142) and there was no significant increase in observed OR and CR rates over other available treatments of lymphoma patients (153). An alternative to anti-CD2 mAbs, CD2-targeting chimeric antigen receptor T cells could be employed for treatment of CD2+ lymphomas. Indeed, there exist efforts to engineer CART cell treatments against T cell antigens like CD2 or CD7, which are overexpressed in T-cell acute lymphoblastic leukemia. To maximize anti-tumor activity, the target antigen in these CART cells is deleted (154). Moreover, due to the broad expression of LFA3 on CD2- lymphomas and the important role of CD2 in T cell costimulation, investigators have highlighted the benefit of using CD2 costimulation on CART cells (155). Notably, even if the activation profile of CD2 is not desired, the extracellular and transmembrane domains of CD2 can be fused with intracellular domains of other stimulating cell surface antigens. This approach combines enhanced target cell-binding by CART cells with desired activation signaling to maximize therapeutic efficacy (156, 157). Other conditions exist which could potentially be treated with CD2+ cell depletion or CD2-LFA3 CoB, e.g., NK-type lymphoproliferative disease of granular lymphocytes (158) or TAP deficiency (159).
Although CD2 has traditionally been considered a non-essential adhesion molecule, evidence that has emerged since the last comprehensive review by Davis and van der Merwe (19), confirms their hypotheses about the multifunctionality of CD2 and its important roles in cell-cell adhesion, IS formation, IS architecture, IS composition and recruitment of intracellular kinases to the IS. Due to the increased expression of CD2 on activated and memory T cells as well as its importance for spontaneous NK cell cytotoxicity, CD2-targeting therapies could become a potent tool for modulating the activation of these cell types in transplant patients or individuals suffering from autoimmune disease. Thus, it seems likely that the recent renewal of interest in CD2 immunology that we have examined in this review will have important clinical applications in the near future.
CB and FC analyzed the literature and wrote the first manuscript. All authors were involved in reviewing and editing the manuscript.
CB, FC, FS, SB, DS, EB, and DB are employees of and/or consultants to ITB-MED. SB, DS, EB, and DB own shares in ITB-MED. HP is an intern at ITB-MED.
ADCC, Antibody-dependent cell cytotoxicity; APC, Antigen-presenting cell; CD, Cluster of differentiation; CD2AP, CD2-associated protein; CD2BP, CD2-binding protein; CD2R, CD2 restricted epitope; CIN85, Cbl-interacting protein of 85 kDa; CMS, Cas ligand with multiple SH3 domains; CoB, Costimulatory blockade; cSMAC, central supramolecular activation motif; CTL, Cytotoxic T lymphocyte; DN, CD4− CD8− double-negative; DP, CD4+ CD8+ double-positive; Dok, Docking protein; dSMAC, distal SMAC; ECD, Extracellular domain; FAK, Focal adhesion kinase Fcγ: Fragment crystallizable gamma; FcγR, Fragment crystallizable gamma receptor; GYF, glycine tyrosine phenylalanine; HCMV, Human cytomegalovirus; ICD, Intracellular domain; IS, Immunological synapse; ITAM, Immunoreceptor tyrosine-based activation motif; JNK, c-Jun N-terminal kinase LAT: Linker for activation of T cells; Lck, Lymphocyte-specific Protein Tyrosine Kinase; mAb, Monoclonal antibody; MAPK, Mitogen-activated protein kinase; MTOC, Microtubule-organizing center; mTOR, Mammalian target of rapamycin NCAM: Neural cell adhesion molecule; NFAT, Nuclear factor of activated T-cells; NF-κB, Nuclear factor κB; NKIS, NK cell immunological synapse; NOD/SCID, Non-obese diabetic/severe combined immunodeficiency; PDB, Protein data bank; PI3K, Phosphatidylinositol 3-kinase; PKCθ, Protein Kinase C θ; PLCγ1, Phospholipase C γ1; pMHC, peptide Major histocompatibility complex; pSMAC, peripheral SMAC; Pyk2, Protein Tyrosine Kinase 2 Beta; RA, Rheumatoid arthritis; SH3, Src homology 3; SLP76, SH2 Domain-Containing Leukocyte Protein of 76 KDa; STAT1, Signal transducer and activator of transcription 1; T1DM, Type I diabetes mellitus; TCR, T cell receptor; Th1, T helper 1; Th17, T helper 17; Vav1, Vav Guanine Nucleotide Exchange Factor 1; WASp, Wiskott-Aldrich Syndrome Protein; ZAP70, Zeta-Chain Associated Protein Kinase of 70 kDa.
1. Jenkins MK, Ashwell JD, Schwartz RH. Allogeneic non-T spleen cells restore the responsiveness of normal T cell clones stimulated with antigen and chemically modified antigen- presenting cells. J Immunol. (1988) 140:3324.
2. Mueller DL, Jenkins MK, Schwartz RH. Clonal expansion versus functional clonal inactivation: a costimulatory signalling pathway determines the outcome of T Cell antigen receptor occupancy. Annu Rev Immunol. (1989) 7:445–80. doi: 10.1146/annurev.iy.07.040189.002305
3. Boussiotis VA, Freeman GJ, Griffin JD, Gray GS, Gribben JG, Nadler LM. CD2 is involved in maintenance and reversal of human alloantigen-specific clonal anergy. J Exp Med. (1994) 180:1665–73. doi: 10.1084/jem.180.5.1665
4. Wurster AL, Rodgers VL, Satoskar AR, Whitters MJ. Interleukin 21 is a T helper (Th) cell 2 cytokine that specifically inhibits the differentiation of naive th cells into interferon γ-producing Th1 cells. J Exp Med. (2002) 196:969–77. doi: 10.1084/jem.20020620
5. Freeman BE, Hammarlund E, Raue H-P, Slifka MK. Regulation of innate CD8 T-cell activation mediated by cytokines. Proc Natl Acad Sci USA. (2012) 109:9971–6. doi: 10.1073/pnas.1203543109
6. Larsen CP, Pearson TC, Adams AB, Tso P. Rational development of LEA29Y (belatacept), a high-affinity variant of CTLA4-Ig with potent immunosuppressive properties. Am J Transplant. (2005) 5:443. doi: 10.1111/j.1600-6143.2005.00749.x
7. Lo DJ, Weaver TA, Stempora L, Mehta AK. Selective targeting of human alloresponsive CD8 effector memory T cells based on CD2 expression. Am J Transplant. (2011) 11:22. doi: 10.1111/j.1600-6143.2010.03317.x
8. Wekerle T, Grinyó JM. Belatacept: from rational design to clinical application. Transpl Int. (2012) 25:139–50. doi: 10.1111/j.1432-2277.2011.01386.x
9. Kean LS, Turka LA, Blazar BR. Advances in targeting co-inhibitory and co-stimulatory pathways in transplantation settings: the Yin to the Yang of cancer immunotherapy. Immunol Rev. (2017) 276:192–212. doi: 10.1111/imr.12523
10. Krensky AM, Sanchez-Madrid F, Robbins E, Nagy JA. The functional significance, distribution, and structure of LFA-1, LFA-2, and LFA-3: cell surface antigens associated with CTL-target interactions. J Immunol. (1983) 131:611–6.
11. Matsui T, Connolly JE, Michnevitz M, Chaussabel D, Yu C-I, Glaser C, et al. CD2 distinguishes two subsets of human plasmacytoid dendritic cells with distinct phenotype and functions. J Immunol. (2009) 182:6815–23. doi: 10.4049/jimmunol.0802008
12. Springer TA, Dustin ML, Kishimoto TK, Marlin SD. The lymphocyte function associated LFA-1, CD2, and LFA-3 molecules: cell adhesion receptors of the immune system. Annual Rev Immunol. (1987) 5:223–52. doi: 10.1146/annurev.iy.05.040187.001255
13. McArdel SL, Terhorst C, Sharpe AH. Roles of CD48 in regulating immunity and tolerance. Clin Immunol. (2016) 164:10–20. doi: 10.1016/j.clim.2016.01.008
14. Haynes BF, Singer KH, Denning SM, Martin ME. Analysis of expression of CD2, CD3, and T cell antigen receptor molecules during early human fetal thymic development [published erratum appears. J Immunol. (1989) 142:1410.
15. Suthanthiran M. Transmembrane signalling via the T cell antigen receptor heterodimer and the CD2 antigen. A synergistic pathway for activation of T cells. Transplantation. (1989) 47:348. doi: 10.1097/00007890-198902000-00033
16. Vollger LW, Tuck DT, Springer TA, Haynes BF, Singer KH. Thymocyte binding to human thymic epithelial cells is inhibited by monoclonal antibodies to CD-2 and LFA-3 antigens. J Immunol. (1987) 138:358–63.
17. Kingma DW, Imus P, Xie XY, Jasper G. CD2 is expressed by a subpopulation of normal B cells and is frequently present in mature B-cell neoplasms. Cytometry. (2002) 50:243–8. doi: 10.1002/cyto.10131
18. Yagita H, Asakawa J, Tansyo S, Nakamura T. Expression and function of CD2 during murine thymocyte ontogeny. Eu J Immunol. (1989) 19:2211–7. doi: 10.1002/eji.1830191206
19. Davis SJ, van der Merwe PA. The structure and ligand interactions of CD2: implications for T-cell function. Immunol Today. (1996) 17:177–87. doi: 10.1016/0167-5699(96)80617-7
20. UniProt: a worldwide hub of protein knowledge. Nucleic Acids Res. (2019) 47:D506–15. doi: 10.1093/nar/gky1049
21. Sayre PH, Reinherz EL. Structure and function of the erythrocyte receptor CD2 on human T lymphocytes: a review. Scand J Rheumatol. (1988) 17:131–44. doi: 10.3109/03009748809102963
22. Bodian DL, Jones EY, Harlos K, Stuart DI, Davis SJ. Crystal structure of the extracellular region of the human cell adhesion molecule CD2 at 2.5å resolution. Structure. (1994) 2:755–66. doi: 10.1016/S0969-2126(94)00076-X
23. Ikemizu S, Sparks LM, Merwe PA, van der Harlos K, Stuart DI, Jones EY, et al. Crystal structure of the CD2-binding domain of CD58 (lymphocyte function-associated antigen 3) at 1.8-Å resolution. PNAS. (1999) 96:4289–94. doi: 10.1073/pnas.96.8.4289
24. Wang J, Smolyar A, Tan K, Liu J, Kim M, Sun ZJ, et al. Structure of a heterophilic adhesion complex between the human CD2 and CD58 (LFA-3) counterreceptors. Cell. (1999) 97:791–803. doi: 10.1016/S0092-8674(00)80790-4
25. Peterson A, Seed B. Monoclonal antibody and ligand binding sites of the T cell erythrocyte receptor (CD2). Nature. (1987) 329:842–6. doi: 10.1038/329842a0
26. Damschroder MM, Kozhich AA, Woods RM, Cheng L. Analysis of human and primate CD2 molecules by protein sequence and epitope mapping with anti-human CD2 antibodies. Mol Immunol. (2004) 41:985–1000. doi: 10.1016/j.molimm.2004.05.004
27. Li J, Smolyar A, Sunder-Plassmann R, Reinherz EL. Ligand-induced conformational change within the CD2 ectodomain accompanies receptor clustering: implication for molecular lattice formation. J Mol Biol. (1996) 263:209–26. doi: 10.1006/jmbi.1996.0570
28. Kaizuka Y, Douglass AD, Vardhana S, Dustin ML. The coreceptor CD2 uses plasma membrane microdomains to transduce signals in T cells. J Cell Biol. (2009) 185:521. doi: 10.1083/jcb.200809136
29. Polley A, Orłowski A, Danne R, Gurtovenko AA. Glycosylation and lipids working in concert direct CD2 ectodomain orientation and presentation. J Phys Chem Lett. (2017) 8:1060–6. doi: 10.1021/acs.jpclett.6b02824
30. Meuer SC, Hussey RE, Fabbi M, Fox D, Acuto O, Fitzgerald KA, et al. An alternative pathway of T-cell activation: a functional role for the 50 kd T11 sheep erythrocyte receptor protein. Cell. (1984) 36:897–906. doi: 10.1016/0092-8674(84)90039-4
31. Anton van der Merwe P, McNamee PN, Davies EA, Barclay AN. Topology of the CD2–CD48 cell-adhesion molecule complex: implications for antigen recognition by T cells. Curr Biol. (1995) 5:74–84. doi: 10.1016/S0960-9822(95)00019-4
32. Davis SJ, Merwe PA. The kinetic-segregation model: TCR triggering and beyond. Nat Immunol. (2006) 7:803–9. doi: 10.1038/ni1369
33. van der Merwe PA, Barclay AN, Mason DW, Davies EA. Human cell-adhesion molecule CD2 binds CD58 (LFA-3) with a very low affinity and an extremely fast dissociation rate but does not bind CD48 or CD59. Biochemistry. (1994) 33:10149–60. doi: 10.1021/bi00199a043
34. van der Merwe PA, Bodian DL, Daenke S, Linsley P, Davis SJ. CD80 (B7-1) binds both CD28 and CTLA-4 with a low affinity and very fast kinetics. J Exp Med. (1997) 185:393–404. doi: 10.1084/jem.185.3.393
35. Tominaga Y, Kita Y, Satoh A, Asai S, Kato K, Ishikawa K, et al. Affinity and kinetic analysis of the molecular interaction of ICAM-1 and leukocyte function-associated antigen-1. J Immunol. (1998) 161:4016–22.
36. Arulanandam AR, Moingeon P, Concino MF, Recny MA, Kato K, Yagita H, et al. A soluble multimeric recombinant CD2 protein identifies CD48 as a low affinity ligand for human CD2: divergence of CD2 ligands during the evolution of humans and mice. J Exp Med. (1993) 177:1439–50. doi: 10.1084/jem.177.5.1439
37. Malmborg Hager A-C, Ellmark P, Borrebaeck C, Furebring C. Affinity and epitope profiling of mouse anti-CD40 monoclonal antibodies. Scand J Immunol. (2003) 57:517. doi: 10.1046/j.1365-3083.2003.01271.x
38. Hahn WC, Bierer BE. Separable portions of the CD2 cytoplasmic domain involved in signaling and ligand avidity regulation. J Exp Med. (1993) 178:1831–6. doi: 10.1084/jem.178.5.1831
39. Zhu D-M, Dustin ML, Cairo CW, Thatte HS, Golan DE. Mechanisms of cellular avidity regulation in CD2-CD58-Mediated T Cell adhesion. ACS Chem Biol. (2006) 1:649–58. doi: 10.1021/cb6002515
40. Walling BL, Kim M. LFA-1 in T Cell migration and differentiation. Front Immunol. (2018) 9:952. doi: 10.3389/fimmu.2018.00952
41. Freund C, Kühne R, Yang H, Park S, Reinherz EL, Wagner G. Dynamic interaction of CD2 with the GYF and the SH3 domain of compartmentalized effector molecules. EMBO J. (2002) 21:5985–95. doi: 10.1093/emboj/cdf602
42. Dustin ML, Olszowy MW, Holdorf AD, Li J. A novel adaptor protein orchestrates receptor patterning and cytoskeletal polarity in T-cell contacts. Cell. (1998) 94:667–77. doi: 10.1016/S0092-8674(00)81608-6
43. Bell GM, Fargnoli J, Bolen JB, Kish L, Imboden JB. The SH3 domain of p56lck binds to proline-rich sequences in the cytoplasmic domain of CD2. J Exp Med. (1996) 183:169–78. doi: 10.1084/jem.183.1.169
44. Li J, Nishizawa K, An W, Hussey RE, Lialios FE, Salgia R, et al. A cdc15-like adaptor protein (CD2BP1) interacts with the CD2 cytoplasmic domain and regulates CD2-triggered adhesion. EMBO J. (1998) 17:7320–36. doi: 10.1093/emboj/17.24.7320
45. Hutchings NJ, Clarkson N, Chalkley R, Barclay AN. Linking the T cell surface protein CD2 to the actin-capping protein CAPZ via CMS and CIN85. J Biol Chem. (2003) 278:22396. doi: 10.1074/jbc.M302540200
46. Hahn WC, Rosenstein Y, Calvo V, Burakoff SJ, Bierer BE. A distinct cytoplasmic domain of CD2 regulates ligand avidity and T-cell responsiveness to antigen. Proc Natl Acad Sci USA. (1992) 89:7179–83. doi: 10.1073/pnas.89.15.7179
47. Brown MH, Cantrell DA, Brattsand G, Crumpton MJ, Gullberg M. The CD2 antigen associates with the T-cell antigen receptor CD3 antigen complex on the surface of human T lymphocytes. Nature. (1989) 339:551–3. doi: 10.1038/339551a0
48. Kivens WJ, Hunt Mobley JL, Zell T. Identification of a proline-rich sequence in the CD2 cytoplasmic domain critical for regulation of integrin-mediated adhesion and activation of phosphoinositide 3-kinase. Mol Cell Biol. (1998) 18:5291. doi: 10.1128/MCB.18.9.5291
49. Nishizawa K, Freund C, Li J, Wagner G. Identification of a proline-binding motif regulating CD2-triggered T lymphocyte activation. Proc Natl Acad Sci USA. (1998) 95:14897–902. doi: 10.1073/pnas.95.25.14897
50. Moncalián G, Cárdenes N, Deribe YL, Spínola-Amilibia M. Atypical polyproline recognition by the CMS N-terminal Src homology 3 domain. J Biol Chem. (2006) 281:38845. doi: 10.1074/jbc.M606411200
51. Freund C, Dötsch V, Nishizawa K, Reinherz EL, Wagner G. The GYF domain is a novel structural fold that is involved in lymphoid signaling through proline-rich sequences. Nat Struct Biol. (1999) 6:656–60. doi: 10.1038/10712
52. Tibaldi EV. CD2BP3, CIN85 and the structurally related adaptor protein CMS bind to the same CD2 cytoplasmic segment, but elicit divergent functional activities. Int Immunol. (2003) 15:313–29. doi: 10.1093/intimm/dxg032
53. Kirsch KH, Georgescu M-M, Ishimaru S, Hanafusa H. CMS: An adapter molecule involved in cytoskeletal rearrangements. Proc Natl Acad Sci USA. (1999) 96:6211–6. doi: 10.1073/pnas.96.11.6211
54. Badour K, Zhang J, Shi F, McGavin MKH. The wiskott-aldrich syndrome protein acts downstream of CD2 and the CD2AP and PSTPIP1 adaptors to promote formation of the immunological synapse. Immunity. (2003) 18:141–54. doi: 10.1016/S1074-7613(02)00516-2
55. Nunes RJ, Castro MAA, Gonçalves CM, Bamberger M. Protein interactions between CD2 and Lck are required for the lipid raft distribution of CD2. J Immunol. (2008) 180:988. doi: 10.4049/jimmunol.180.5.3613-b
56. Liu SJ, Hahn WC, Bierer BE, Golan DE. Intracellular mediators regulate CD2 lateral diffusion and cytoplasmic Ca2 mobilization upon CD2-mediated T cell activation. Biophys J. (1995) 68:459–70. doi: 10.1016/S0006-3495(95)80207-9
57. Liu S-QJ, Golan DE. T-cell stimulation through the T-cell receptor/CD3 complex regulates CD2 lateral mobility by a calcium/calmodulin-dependent mechanism. Biophys J. (1999) 76:1679–92. doi: 10.1016/S0006-3495(99)77327-3
58. Bruck S, Huber TB, Ingham RJ, Kim K. Identification of a novel inhibitory actin-capping protein binding motif in CD2-associated protein. J Biol Chem. (2006) 281:19196. doi: 10.1074/jbc.M600166200
59. Dikic I. CIN85/CMS family of adaptor molecules. FEBS Lett. (2002) 529:110–5. doi: 10.1016/S0014-5793(02)03188-5
60. Zhao J, Bruck S, Cemerski S, Zhang L. CD2AP links cortactin and capping protein at the cell periphery to facilitate formation of Lamellipodia. Mol Cell Biol. (2013) 33:38–47. doi: 10.1128/MCB.00734-12
61. Lehtonen S, Zhao F, Lehtonen E. CD2-associated protein directly interacts with the actin cytoskeleton. Am J Physiol Renal Physiol. (2002) 283:F734. doi: 10.1152/ajprenal.00312.2001
62. Zurli V, Montecchi T, Heilig R, Poschke I, Volkmar M, Wimmer G, et al. Phosphoproteomics of CD2 signaling reveals AMPK-dependent regulation of lytic granule polarization in cytotoxic T cells. Sci. Signal. (2020) 13:eaaz1965. doi: 10.1126/scisignal.aaz1965
63. Spruyt LL, Glennie MJ, Beyers AD, Williams AF. Signal transduction by the CD2 antigen in T cells and natural killer cells: requirement for expression of a functional T cell receptor or binding of antibody Fc to the Fc receptor, Fc gamma RIIIA (CD16). J Exp Med. (1991) 174:1407–15. doi: 10.1084/jem.174.6.1407
64. Howard FD, Moingeon P, Moebius U, McConkey DJ. The CD3 zeta cytoplasmic domain mediates CD2-induced T cell activation. J Exp Med. (1992) 176:139–45. doi: 10.1084/jem.176.1.139
65. Moingeon P, Lucich J, Mcconkey D, Letourneur F. CD3 zeta dependence of the CD2 pathway of activation in T lymphocytes and natural killer cells. Proc Natl Acad Sci USA. (1992) 89:1492–6. doi: 10.1073/pnas.89.4.1492
66. Steeg C, von Bonin A, Mittrücker HW, Malissen B. CD2-mediated signaling in T cells lacking the zeta-chain-specific immune receptor tyrosine-based activation (ITAM) motif. Eur J Immunol. (1997) 27:2233–8. doi: 10.1002/eji.1830270917
67. Sunder-Plassmann R, Reinherz EL. A p56lck-independent pathway of CD2 signaling involves jun kinase. J Biol Chem. (1998) 273:24249–57. doi: 10.1074/jbc.273.37.24249
68. Fukai I, Hussey RE, Sunder-Plassmann R, Reinherz EL. A critical role for p59fyn in CD2-based signal transduction. Eur J Immunol. (2000) 30:3507–15. doi: 10.1002/1521-4141(2000012)30:12<3507::AID-IMMU3507>3.0.CO;2-O
69. Kalland ME, Oberprieler NG, Vang T, Taskén K. T cell-signaling network analysis reveals distinct differences between CD28 and CD2 costimulation responses in various subsets and in the MAPK pathway between resting and activated regulatory T cells. J Immunol. (2011) 187:5233–45. doi: 10.4049/jimmunol.1101804
70. Skånland SS, Moltu K, Berge T, Aandahl EM. T-cell co-stimulation through the CD2 and CD28 co-receptors induces distinct signalling responses. Biochem J. (2014) 460:399–410. doi: 10.1042/BJ20140040
71. Parra E, Varga M, Hedlund G, Kalland T. Costimulation by B7-1 and LFA-3 targets distinct nuclear factors that bind to the interleukin-2 promoter: B7-1 negatively regulates LFA-3-induced NF-AT DNA binding. Mol Cell Biol. (1997) 17:1314. doi: 10.1128/MCB.17.3.1314
72. Jutz S, Leitner J, Schmetterer K, Doel-Perez I. Assessment of costimulation and coinhibition in a triple parameter T cell reporter line: simultaneous measurement of NF-κB, NFAT and AP-1. J Immunol Methods. (2016) 430:10–20. doi: 10.1016/j.jim.2016.01.007
73. Umehara H, Huang JY, Kono T, Tabassam FH, Okazaki T, Bloom ET, et al. Involvement of protein tyrosine kinase p72syk and phosphatidylinositol 3-kinase in CD2-mediated granular exocytosis in the natural killer cell line, NK3.3. J Immunol. (1997) 159:1200–7.
74. Umehara H, Inoue H, Huang J, Kono T. Role for adapter proteins in costimulatory signals of CD2 and IL-2 on NK cell activation. Mol Immunol. (2002) 38:587–96. doi: 10.1016/S0161-5890(01)00099-2
75. Inoue H, Miyaji M, Kosugi A, Nagafuku M, Okazaki T, Mimori T, et al. Lipid rafts as the signaling scaffold for NK cell activation: tyrosine phosphorylation and association of LAT with phosphatidylinositol 3-kinase and phospholipase C-gamma following CD2 stimulation. Eur J Immunol. (2002) 32:2188–98. doi: 10.1002/1521-4141(200208)32:8<2188::AID-IMMU2188>3.0.CO;2-T
76. Dustin ML. Modular design of immunological synapses and kinapses. Cold Spring Harbor Perspect Biol. (2009) 1:a002873. doi: 10.1101/cshperspect.a002873
77. Singleton K, Parvaze N, Dama KR, Chen KS, Jennings P, Purtic B, et al. A large T cell invagination with CD2 enrichment resets receptor engagement in the immunological synapse. J Immunol. (2006) 177:4402–13. doi: 10.4049/jimmunol.177.7.4402
78. Zaru R, Cameron TO, Stern LJ, Müller S. Cutting Edge: TCR engagement and triggering in the absence of large-scale molecular segregation at the T cell-APC contact site. J Immunol. (2002) 168:4287–91. doi: 10.4049/jimmunol.168.9.4287
79. Chang VT, Fernandes RA, Ganzinger KA, Lee SF. Initiation of T cell signaling by CD45 segregation at “close contacts.” Nature Immunol. (2016) 17:574–82. doi: 10.1038/ni.3392
80. Siokis A, Robert PA, Demetriou P, Dustin ML. F-actin-driven CD28-CD80 localization in the immune synapse. Cell Rep. (2018) 24:1151–62. doi: 10.1016/j.celrep.2018.06.114
81. Demetriou P, Abu-Shah E, McCuaig S, Mayya V, Valvo S, Korobchevskaya K, et al. CD2 expression acts as a quantitative checkpoint for immunological synapse structure and T-cell activation. Pre-print bioRxiv. (2019) 589440. doi: 10.1101/589440
82. Douglass AD, Vale RD. Single-molecule microscopy reveals plasma membrane microdomains created by protein-protein networks that exclude or trap signaling molecules in T cells. Cell. (2005) 121:937–50. doi: 10.1016/j.cell.2005.04.009
83. Kamoun M, Kadin ME, Martin PJ, Nettleton J. A novel human T cell antigen preferentially expressed on mature T cells and shared by both well and poorly differentiated B cell leukemias and lymphomas. J Immunol. (1981) 127:987–91.
84. Howard FD, Ledbetter JA, Wong J, Bieber CP. A human T lymphocyte differentiation marker defined by monoclonal antibodies that block E-rosette formation. J Immunol. (1981) 126:2117–22.
85. Reinherz EL. A molecular basis for thymic selection: regulation of T11 induced thymocyte expansion by the T3-Ti antigen/MHC receptor pathway. Immunol Today. (1985) 6:75–9. doi: 10.1016/0167-5699(85)90019-2
86. Denning SM, Tuck DT, Vollger LW, Springer TA. Monoclonal antibodies to CD2 and lymphocyte function-associated antigen 3 inhibit human thymic epithelial cell-dependent mature thymocyte activation. J Immunol. (1987) 139:2573–8.
87. Kyewski BA, Jenkinson EJ, Kingston R, Altevogt P. The effects of anti-CD2 antibodies on the differentiation of mouse thymocytes. Eur J Immunol. (1989) 19:951–4. doi: 10.1002/eji.1830190526
88. Sen J, Arceci RJ, Jones W, Burakoff SJ. Expression and ontogeny of murine CD2. Eur J Immunol. (1989) 19:1297–302. doi: 10.1002/eji.1830190722
89. Yagita H, Nakamura T, Asakawa J-I, Matsuda H, Tansyo S, Iigo Y, et al. CD2 expression in murine B cell lineage. Int Immunol. (1989) 1:94–8. doi: 10.1093/intimm/1.1.94
90. Owen MJ, Jenklnson EJ, Brown MH, Sewell WA. Murine CD2 gene expression during fetal thymus ontogeny. Eur J Immunol. (1988) 18:187–9. doi: 10.1002/eji.1830180129
91. Haynes BF, Heinly CS. Early human T cell development: analysis of the human thymus at the time of initial entry of hematopoietic stem cells into the fetal thymic microenvironment. J Exp Med. (1995) 181:1445–58. doi: 10.1084/jem.181.4.1445
92. Duplay P, Lancki D, Allison JP. Distribution and ontogeny of CD2 expression by murine T cells. J Immunol. (1989) 142:2998–3005.
93. Rodewald HR, Awad K, Moingeon P, D'Adamio L, Rabinowitz D, Shinkai Y, et al. Fc gamma RII/III and CD2 expression mark distinct subpopulations of immature CD4-CD8- murine thymocytes: in vivo developmental kinetics and T cell receptor beta chain rearrangement status. J Exp Med. (1993) 177:1079–92. doi: 10.1084/jem.177.4.1079
94. Groettrup M, Baron A, Griffiths G, Palacios R, von Boehmer H. T cell receptor (TCR) beta chain homodimers on the surface of immature but not mature alpha, gamma, delta chain deficient T cell lines. EMBO J. (1992) 11:2735–45. doi: 10.1002/j.1460-2075.1992.tb05339.x
95. Killeen N, Stuart SG, Littman DR. Development and function of T cells in mice with a disrupted CD2 gene. EMBO J. (1992) 11:4329–36. doi: 10.1002/j.1460-2075.1992.tb05532.x
96. Sasada T, Reinherz EL. A critical role for CD2 in both thymic selection events and mature T cell function. J Immunol. (2001) 166:2394–403. doi: 10.4049/jimmunol.166.4.2394
97. Teh S-J, Killeen N, Tarakhovsky A, Littman DR. CD2 regulates the positive selection and function of antigen-specific CD4–CD8 T cells. Blood. (1997) 89:1308–18. doi: 10.1182/blood.V89.4.1308
98. Sasada T, Yang H, Reinherz EL. CD2 facilitates differentiation of CD4 Th cells without affecting Th1/Th2 polarization. J Immunol. (2002) 168:1113–22. doi: 10.4049/jimmunol.168.3.1113
99. Mombaerts P, Clarke AR, Rudnicki MA, Iacomini J. Mutations in T-cell antigen receptor genes alpha and beta block thymocyte development at different stages. Nature. (1992) 360:225–31. doi: 10.1038/360225a0
100. Fehling HJ, Krotkova A, Saint-Ruf C, von Boehmer H. Crucial role of the pre-T-cell receptor alpha gene in development of alpha beta but not gamma delta T cells. Nature. (1995) 375:795–8. doi: 10.1038/375795a0
101. Melton E, Sarner N, Torkar M, van der Merwe PA. Transgene-encoded human CD2 acts in a dominant negative fashion to modify thymocyte selection signals in mice. Eur J Immunol. (1996) 26:2952–63. doi: 10.1002/eji.1830261222
102. Tarakhovsky A, Kanner SB, Hombach J, Ledbetter JA. A role for CD5 in TCR-mediated signal transduction and thymocyte selection. Science. (1995) 269:535–7. doi: 10.1126/science.7542801
103. Vacchio M, Williams J, Hodes R. A novel role for CD28 in thymic selection: elimination of CD28/B7 interactions increases positive selection. Eur J Immunol. (2005) 35:418–27. doi: 10.1002/eji.200424918
104. Park J-E, Botting RA, Domínguez Conde C, Popescu D-M. A cell atlas of human thymic development defines T cell repertoire formation. Science. (2020) 367:eaay3224. doi: 10.1126/science.aay3224
105. Dupré L, Houmadi R, Tang C, Rey-Barroso J. T lymphocyte migration: an action movie starring the actin and associated actors. Front Immunol. (2015) 6:586. doi: 10.3389/fimmu.2015.00586
106. Tibaldi EV, Salgia R, Reinherz EL. CD2 molecules redistribute to the uropod during T cell scanning: Implications for cellular activation and immune surveillance. Proc Natl Acad Sci USA. (2002) 99:7582. doi: 10.1073/pnas.112212699
107. Wang ECY, Pjechova M, Nightingale K, Vlahava V-M, Patel M, Ruckova E, et al. Suppression of costimulation by human cytomegalovirus promotes evasion of cellular immune defenses. Proc Natl Acad Sci USA. (2018) 115:4998–5003. doi: 10.1073/pnas.1720950115
108. Gückel B, Berek C, Lutz M, Altevogt P. Anti-CD2 antibodies induce T cell unresponsiveness in vivo. J Exp Med. (1991) 174:957. doi: 10.1084/jem.174.5.957
109. Fortner KA, Russell JQ, Budd RC. Down-modulation of CD2 delays deletion of superantigen-responsive T cells. Eur J Immunol. (1998) 28:70–9. doi: 10.1002/(SICI)1521-4141(199801)28:01<70::AID-IMMU70>3.0.CO;2-9
110. Podestà MA, Binder C, Sellberg F, DeWolf S. Siplizumab selectively depletes effector memory T cells and promotes a relative expansion of alloreactive regulatory T cells in vitro. Am J Transpl. (2019) 20:88–100. doi: 10.1111/ajt.15533
111. Weaver TA, Charafeddine AH, Agarwal A, Turner AP, Russell M, Leopardi FV, et al. Alefacept promotes co-stimulation blockade based allograft survival in nonhuman primates. Nat Med. (2009) 15:746–9. doi: 10.1038/nm.1993
112. Berglund E, Alonso-Guallart P, Danton M, Sellberg F, Binder C, Fröbom R, et al. Safety and pharmacodynamics of anti-CD2 monoclonal antibody treatment in cynomolgus macaques- an experimental study. Transpl Int. (2019) 33:98–107. doi: 10.1111/tri.13524
113. Leitner J, Herndler-Brandstetter D, Zlabinger GJ, Grubeck-Loebenstein B. CD58/CD2 is the primary costimulatory pathway in human CD28-CD8 T cells. J Immunol. (2015) 195:477–87. doi: 10.4049/jimmunol.1401917
114. Evans CF, Rall GF, Killeen N, Littman D. CD2-deficient mice generate virus-specific cytotoxic T lymphocytes upon infection with lymphocytic choriomeningitis virus. J Immunol.(1993) 151:6259–64.
115. Dumont C, Déas O, Mollereau B, Hebib C. Potent apoptotic signaling and subsequent unresponsiveness induced by a single CD2 mAb (BTI-322) in activated human peripheral T cells. J Immunol. (1998) 160:3797–804.
116. Hammond SA, Fuhrmann S, Roff S, Kiener PA, Coats S, Kinch MS. Mechanistic evaluation of siplizumab (MEDI-507) activity on normal and malignant T-lymphocytes. Blood. (2006) 108:2504–4. doi: 10.1182/blood.V108.11.2504.2504
117. Gonsky R, Deem RL, Young HA, Targan SR. CD2 mediates activation of the IFN-γ intronic STAT binding region in mucosal T cells. Eur J Immunol. (2003) 33:1152–62. doi: 10.1002/eji.200322875
118. Orlik C, Deibel D, Küblbeck J, Balta E. Keratinocytes costimulate naive human T cells via CD2: a potential target to prevent the development of proinflammatory Th1 cells in the skin. Cell Mol Immunol. (2020) 17:380–94. doi: 10.1038/s41423-019-0261-x
119. Paul S, Lal G. The molecular mechanism of natural killer cells function and its importance in cancer immunotherapy. Front Immunol. (2017) 8:1124. doi: 10.3389/fimmu.2017.01124
120. Watzl C. How to trigger a killer: modulation of natural killer cell reactivity on many levels. Adv Immunol. (2014) 124:137–70. doi: 10.1016/B978-0-12-800147-9.00005-4
121. Orange JS, Harris KE, Andzelm MM, Valter MM. The mature activating natural killer cell immunologic synapse is formed in distinct stages. Proc Natl Acad Sci USA. (2003) 100:14151–56. doi: 10.1073/pnas.1835830100
122. Grier JT, Forbes LR, Monaco-Shawver L, Oshinsky J, Atkinson TP, Moody C, et al. Human immunodeficiency-causing mutation defines CD16 in spontaneous NK cell cytotoxicity. J Clin Invest. (2012) 122:3769–80. doi: 10.1172/JCI64837
123. Jawahar S, Moody C, Chan M, Finberg R, Geha R, Chatila T. Natural Killer (NK) cell deficiency associated with an epitope-deficient Fc receptor type IIIA (CD16-II). Clin Exp Immunol. (1996) 103:408-13. doi: 10.1111/j.1365-2249.1996.tb08295.x
124. Beziat V, Liu LL, Malmberg JA, Ivarsson MA. NK cell responses to cytomegalovirus infection lead to stable imprints in the human KIR repertoire and involve activating KIRs. Blood. (2013) 121: 2678–88. doi: 10.1182/blood-2012-10-459545
125. Wu Z, Sinzger C, Frascaroli G, Reichel J. Human cytomegalovirus-induced NKG2Chi CD57hi natural killer cells are effectors dependent on humoral antiviral immunity. J Virol. (2013) 87:7717–25. doi: 10.1128/JVI.01096-13
126. Liu LL, Landskron J, Ask EH, Enqvist M. Critical role of CD2 Co-stimulation in adaptive natural killer cell responses revealed in NKG2C-deficient humans. Cell Rep. (2016) 15:1088–99. doi: 10.1016/j.celrep.2016.04.005
127. Rölle A, Halenius A, Ewen E-M, Cerwenka A, Hengel H, Momburg F. CD2-CD58 interactions are pivotal for the activation and function of adaptive natural killer cells in human cytomegalovirus infection. Eur J Immunol. (2016) 46:2420–5. doi: 10.1002/eji.201646492
128. Kim T-J, Kim N, Kim E-O, Choi J-R, Bluestone JA, Lee K-M. Suppression of human anti-porcine natural killer cell xenogeneic responses by combinations of monoclonal antibodies specific to CD2 and NKG2D and extracellular signal-regulated kinase kinase inhibitor. Immunology. (2010) 130:545–55. doi: 10.1111/j.1365-2567.2010.03253.x
129. Comerci CJ, Mace EM, Banerjee PP, Orange JS. CD2 promotes human natural killer cell membrane nanotube formation. PLoS ONE. (2012) 7:e47664. doi: 10.1371/journal.pone.0047664
130. Chavin KD, Lau HT, Bromberg JS. Prolongation of allograft and xenograft survival in mice by anti-CD2 monoclonal antibodies. Transplantation. (1992) 54:286–91. doi: 10.1097/00007890-199208000-00018
131. Erben U, Pawlowski NN, Doerfel K, Loddenkemper C, Hoffmann JC, Siegmund B, et al. Targeting human CD2 by the monoclonal antibody CB.219 reduces intestinal inflammation in a humanized transfer colitis model. Clin Immunol. (2015) 157:16–25. doi: 10.1016/j.clim.2015.01.004
132. Sellberg F, Berglund D, Binder C, Hope J. Pharmacokinetic and pharmacodynamic study of a clinically effective anti-CD2 monoclonal antibody. Scand J Immunol. (2019) 91:e12839. doi: 10.1111/sji.12839
133. Jenneck C, Novak N. The safety and efficacy of alefacept in the treatment of chronic plaque psoriasis. Therapeut Clin Risk Manage. (2007) 3:411.
134. Rigby MR, Harris KM, Pinckney A, DiMeglio LA. Alefacept provides sustained clinical and immunological effects in new-onset type 1 diabetes patients. J Clin Invest. (2015) 125:3285–96. doi: 10.1172/JCI81722
135. Rostaing L, Charpentier B, Glyda M, Rigotti P. Alefacept combined with tacrolimus, mycophenolate mofetil and steroids in de novo kidney transplantation: a randomized controlled trial. Am J Transplant. (2013) 13:1724–33. doi: 10.1111/ajt.12303
136. Stenger EO, Chiang K-Y, Haight A, Qayed M. Use of alefacept for preconditioning in multiply transfused pediatric patients with nonmalignant diseases. Biol Blood Marrow Transplant. (2015) 21:1845–52. doi: 10.1016/j.bbmt.2015.06.005
137. Langley RG, Papp K, Bissonnette R, Toth D. Safety profile of intravenous and subcutaneous siplizumab, an anti-CD2 monoclonal antibody, for the treatment of plaque psoriasis: results of two randomized, double-blind, placebo-controlled studies. Int J Dermatol. (2010) 49:818–28. doi: 10.1111/j.1365-4632.2010.04512.x
138. Koenecke C, Shaffer J, Alexander SI, Preffer F. NK cell recovery, chimerism, function, and recognition in recipients of haploidentical hematopoietic cell transplantation following nonmyeloablative conditioning using a humanized anti-CD2 mAb, Medi-507. Exp Hematol. (2003) 31:911–23. doi: 10.1016/S0301-472X(03)00224-8
139. Pruett TL, McGory RW, Wright FH, Pescovitz MD. Safety profile, pharmacokinetics, and pharmacodynamics of siplizumab, a humanized anti-CD2 monoclonal antibody, in renal allograft recipients. Transplant Proc. (2009) 41:3655–61. doi: 10.1016/j.transproceed.2009.06.226
140. Spitzer TR, McAfee SL, Dey BR, Colby C. Nonmyeloablative haploidentical stem-cell transplantation using anti-CD2 monoclonal antibody (MEDI-507)-based conditioning for refractory hematologic malignancies. Transplantation. (2003) 75:1748–51. doi: 10.1097/01.TP.0000064211.23536.AD
141. Adkins D, Ratanatharathorn V, Yang H, White B. Safety profile and clinical outcomes in a phase I, placebo-controlled study of siplizumab in acute graft-versus-host disease. Transplantation. (2009) 88:198–202. doi: 10.1097/TP.0b013e3181abfbf7
142. Roswarski J, Roschewski M, Lucas A, Melani C. Phase I dose escalation study of the anti-CD2 monoclonal antibody, siplizumab, with DA-EPOCH-R in aggressive peripheral T-cell lymphomas. Leukemia Lymphoma. (2018) 59:1466–9. doi: 10.1080/10428194.2017.1387908
143. Kawai T, Cosimi AB, Spitzer TR, Tolkoff-Rubin N. HLA-mismatched renal transplantation without maintenance immunosuppression. N Engl J Med. (2008) 358:353–61. doi: 10.1056/NEJMoa071074
144. Kawai T, Sachs DH, Sprangers B, Spitzer TR. Long-term results in recipients of combined HLA-mismatched kidney and bone marrow transplantation without maintenance immunosuppression. Am J Transplantat. (2014) 14:1599–611. doi: 10.1111/ajt.12731
145. Sprangers B, DeWolf S, Savage TM, Morokata T. Origin of enriched regulatory T cells in patients receiving combined kidney-bone marrow transplantation to induce transplantation tolerance. Am J Transplant. (2017) 17:2020–32. doi: 10.1111/ajt.14251
146. Savage TM, Shonts BA, Obradovic A, Dewolf S. Early expansion of donor-specific Tregs in tolerant kidney transplant recipients. JCI insight. (2018) 3:e124086. doi: 10.1172/jci.insight.124086
147. Potocnik AJ, Menninger H, Yang SY, Pirner K, Krause A, Burmester GR, et al. Expression of the CD2 activation epitope T11-3 (CD2R) on T cells in rheumatoid arthritis, juvenile rheumatoid arthritis, systemic lupus erythematosus, ankylosing spondylitis, and Lyme disease: phenotypic and functional analysis. Scand J Immunol. (1991) 34:351–8. doi: 10.1111/j.1365-3083.1991.tb01556.x
148. Baecher-Allan CM, Costantino CM, Cvetanovich GL, Ashley CW. CD2 costimulation reveals defective activity by human CD4 CD25(hi) regulatory cells in patients with multiple sclerosis. J Immunol. (2011) 186:3317–26. doi: 10.4049/jimmunol.1002502
149. Fehniger TA, Cooper MA, Nuovo GJ, Cella M. CD56bright natural killer cells are present in human lymph nodes and are activated by T cell-derived IL-2: a potential new link between adaptive and innate immunity. Blood. (2003) 101:3052–7. doi: 10.1182/blood-2002-09-2876
150. Angelo LS, Banerjee PP, Monaco-Shawver L, Rosen JB. Practical NK cell phenotyping and variability in healthy adults. Immunol Res. (2015) 62:341–56. doi: 10.1007/s12026-015-8664-y
151. Fogel LA, Yokoyama WM, French AR. Natural killer cells in human autoimmune disorders. Arthritis Res Therapy. (2013) 15:216. doi: 10.1186/ar4232
152. Zhang Z, Zhang M, Ravetch JV, Goldman C. Effective therapy for a murine model of adult T-cell leukemia with the humanized anti-CD2 monoclonal antibody, MEDI-507. Blood. (2003) 102:284–88. doi: 10.1182/blood-2002-11-3601
153. Bartlett NL, Wilson WH, Jung SH, Hsi ED. Dose-adjusted EPOCH-R compared with R-CHOP as frontline therapy for diffuse large B-cell lymphoma: clinical outcomes of the phase iii intergroup trial alliance/CALGB 50303. J Clin Oncol. (2019) 37:1790–9. doi: 10.1200/JCO.18.01994
154. Cooper ML, DiPersio JF. Chimeric antigen receptor T cells (CAR-T) for the treatment of T-cell malignancies. Best Pract Res Clin Haematol. (2019) 32:101097. doi: 10.1016/j.beha.2019.101097
155. Cheadle EJ, Rothwell DG, Bridgeman JS, Sheard VE. Ligation of the CD2 co-stimulatory receptor enhances IL-2 production from first-generation chimeric antigen receptor T cells. Gene Therapy. (2012) 19:1114–20. doi: 10.1038/gt.2011.192
156. Hanada K, Bagadia P, Wang Q, Griffith K, Yang J. Augmenting adoptive T cell therapy through universal chimeric costimulators. J Immunother Cancer. (2013) 1:P14. doi: 10.1186/2051-1426-1-S1-P14
157. Posey AD, June CH. CD2, the first identified T cell co-stimulator, demonstrates more effective chimeric antigen receptor activity over CD28 and 4-1BB. Mol Ther. (2015) 23:S83. doi: 10.1016/S1525-0016(16)33816-3
158. Pascal V, Schleinitz N, Brunet C, Ravet S. Comparative analysis of NK cell subset distribution in normal and lymphoproliferative disease of granular lymphocyte conditions. Eur J Immunol. (2004) 34:2930–40. doi: 10.1002/eji.200425146
Keywords: CD2, CD58, LFA-3 (lymphocyte functional antigen-3), T cell activation, costimulation, costimulation blockade, Alefacept, siplizumab
Citation: Binder C, Cvetkovski F, Sellberg F, Berg S, Paternina Visbal H, Sachs DH, Berglund E and Berglund D (2020) CD2 Immunobiology. Front. Immunol. 11:1090. doi: 10.3389/fimmu.2020.01090
Received: 06 February 2020; Accepted: 05 May 2020;
Published: 09 June 2020.
Edited by:
Michael Loran Dustin, University of Oxford, United KingdomReviewed by:
Paul E. Love, National Institutes of Health (NIH), United StatesCopyright © 2020 Binder, Cvetkovski, Sellberg, Berg, Paternina Visbal, Sachs, Berglund and Berglund. This is an open-access article distributed under the terms of the Creative Commons Attribution License (CC BY). The use, distribution or reproduction in other forums is permitted, provided the original author(s) and the copyright owner(s) are credited and that the original publication in this journal is cited, in accordance with accepted academic practice. No use, distribution or reproduction is permitted which does not comply with these terms.
*Correspondence: Christian Binder, Y2hyaXN0aWFuLmJpbmRlckBpZ3AudXUuc2U=
Disclaimer: All claims expressed in this article are solely those of the authors and do not necessarily represent those of their affiliated organizations, or those of the publisher, the editors and the reviewers. Any product that may be evaluated in this article or claim that may be made by its manufacturer is not guaranteed or endorsed by the publisher.
Research integrity at Frontiers
Learn more about the work of our research integrity team to safeguard the quality of each article we publish.