- 1Center for Autoimmune Musculoskeletal and Hematopoietic Diseases, The Feinstein Institutes for Medical Research, Manhasset, NY, United States
- 2Departments of Molecular Medicine and Pediatrics, Zucker School of Medicine at Hofstra-Northwell, Hempstead, NY, United States
Cytokines are soluble factors that play vital roles in systemic function due to their ability to initiate and mediate cell-to-cell communication. Another important mechanism of intercellular communication that has gained significant attention in the past 10 years is the release of extracellular vesicles (EVs). EVs are released by all cells during normal physiology, in states of resting and activation, as well as during disease. Accumulating evidence indicates that cytokines may be packaged into EVs, and the packaging of cytokines into EVs, along with their ultimate secretion, may also be regulated by cytokines. Importantly, the repertoire of biomolecules packaged into EVs is shaped by the biological state of the cell (resting vs. activated and healthy vs. disease) and the EV biogenesis pathway involved, thus providing mechanisms by which EV packaging and secretion may be modulated. Given the critical role of cytokines in driving acute and chronic inflammatory and autoimmune diseases, as well as their role in establishing the tumor immune microenvironment, in this review, we will focus on these disease settings and summarize recent progress and mechanisms by which cytokines may be packaged within and modulated by EVs, as a therapeutic option for regulating innate and adaptive immunity.
Introduction
Intercellular communication is an essential biological feature that is mediated through (1) cell-cell contact, (2) soluble factors (cytokines, growth factors, hormones, neurotransmitters) and (3) the more recently discovered extracellular vesicles (EVs) that carry cytosolic, nuclear and cell-surface proteins, lipids, nucleotides, microRNA, and metabolites (1, 2). These three mechanisms of intercellular communication help to ensure that homeostasis is maintained in a biological system and that the system can respond appropriately to conditions of stress and disease. Conversely, dysregulation of any of these mechanisms of intercellular communication may promote altered physiology leading to disease.
Cytokines are small, non-structural proteins with low molecular weights that are synthesized and secreted by immune cells: macrophages, B and T cells, dendritic cells, neutrophils, mast cells, as well as endothelial, epithelial, fibroblasts and stromal cells, as a mechanism to communicate with each other (3). As soluble factors, they are largely responsible for promoting and regulating an immune response by acting on receptors at the cell membrane. This results in the downstream regulation of signaling molecules that stimulate cells toward sites of inflammation, infection, and trauma (4). Thus, cytokines have significant roles in a variety of functions including cell activation, differentiation, proliferation, trafficking, inflammation, and tumorigenesis that affect every organ system in the body. Their pleiotropic function(s) as intercellular messengers allows them to act at the site they are produced (autocrine), on nearby cells (paracrine), or on distant cells and tissues (endocrine), which also enables them to be self-regulating (4, 5). Cytokines act as extracellular ligands for specific membrane receptors present on responsive target cells and thus must possess a high affinity for each other. The high affinity helps to explain why cytokines can exert their biological effects in picomolar concentrations (4). As such, it is not surprising that multiple mechanisms have evolved that allow for the fine-tuning of cytokine secretion that enables an effective but limited response. This level of control is necessary in order to prevent excessive and/or dysregulated release that could drive acute and chronic inflammatory and autoimmune diseases (5, 6). As a result, it is important to understand the secretory (exocytic) pathways and endocytic compartments involved in cytokine transport, along with the regulatory molecules and cellular machinery that determine the levels and timing of cytokine release [reviewed in (5–9)]. Although cytokines are considered soluble factors, recent data indicate that they can also function as membrane proteins and be packed and stored in secretory granules, lysosome-related organelles, or secretory lysosomes and later released at the cell surface (8). Accumulating evidence indicate that cytokines can also reach the extracellular space through EVs.
EVs are a heterogeneous collection of small membrane-bound organelles that are naturally released from all cells [recently reviewed in (10)]. Originally, they were described as small vesicles that selectively remove excess and unnecessary components of cells in order to maintain homeostasis. However, subsequent studies over the past 10 years reveal that EVs play an important and targeted, functional role in cell-to-cell communication (11). Studies from multiple labs show that the packaging of cellular components within EVs are determined, in part, by the cell type they are secreted from and the physiologic status of the parental cell (12–15); the latter involving mechanisms that can be manipulated to potentially alter the cellular components within EVs and the secretion of EVs.
Based on biochemical and microscopic characterization of EVs, they can be broadly separated into two classes—exosomes and microvesicles—that are primarily distinguished by the mechanisms of biogenesis, as well as size (11). Details of the mechanisms of EV biogenesis have recently been reviewed (10). Briefly, exosomes range in size between ~50 and 150 nm in diameter (~100 nm on average) and arise from the endo-lysosomal trafficking pathway during the formation of multivesicular bodies (MVBs). Exosomes are released extracellularly when MVBs fuse with the plasma membrane. Microvesicles, on the other hand, are organelles generated by pathways that direct the outward budding or shedding of the plasma membrane and range in size between ~50 nm to 1 μm. More recent data in the field of EVs indicate that these two classes also differ by the cellular components that are packaged inside, likely resulting in different biological functions (11). The unique profile of cellular components that are packaged in EVs and secreted from a cell represents a molecular, biological, and cellular code that contains information about the parental cell at the time of secretion and how the EVs may reprogram recipient, adjacent cells and tissues during normal homeostasis and disease (14). However, precise identification of the origin of EVs is made difficult by the fact that there is substantial commonality in size, external markers, and internal content between exosomes and microvesicles. As a result, it is often not possible to definitively establish the method of biogenesis of isolated EVs, underscoring the importance of clearly defining the parameters used to identify specific EV populations (16). In this review, we provide an overview of cellular states and mechanisms by which cytokines may be packaged within and their release controlled by EVs.
Packaging of Cytokines in EVs
While all innate immune cells have the capacity for constitutive exocytosis, their release can also occur through regulated secretory pathways [reviewed in (9, 14)]. The constitutive and induced secretion of cytokines as soluble factors provides a systemic release that helps to maintain normal homeostasis. Regulated secretion, on the other hand, provides the ability to orchestrate the rapid delivery of a concentrated amount of cytokines to a specific site in response to a specific signal (9). Recent work by Fitzgerald et al. revealed that cytokine packaging into EVs was a general biological phenomenon that occurs in vitro, ex vivo and in vivo from multiple cell types and tissues. Somewhat surprising, they found that all cytokines could be packaged into EVs. However, depending on the biological system and cell type, they reported that a cytokine could be released either in soluble or EV-associated form. Analysis across multiple biological systems (placental villous explants, tonsil explants, amnion explants, cervix explants, plasma, T cells, amniotic fluid, monocytes) revealed that 9 cytokines—Interleukin 6 (IL6), IL8, IL13, IL16, IP10, MCP1, MIP1α, MIP1β, and MIP3α–were more often found in soluble form. Conversely, 11 cytokines—IL2, IL4, IL12p70, IL17, IL21, IL22, IL33, IFNγ, ITAC, TGFβ, and TNFα–were found in greater levels in EVs. An interesting aspect of this study that is relevant to disease was the finding that cytokines packaged into EVs are not detected by standard cytokine assays, such as ELISA or other multiplexed immunoassays, since they are hidden from antibody detection by the EV membrane. Thus, methods to determine cytokine production from EVs will be important for our understanding of their role(s) in health and disease (12, 17).
What exactly is the biological meaning of packaging cytokines in EVs? Given that cytokines can exert their biological effects in picomolar concentrations, packaging cytokines into EVs is one mechanism whereby cytokine expression may be concentrated at the surface of other cells that might not otherwise be targeted by cytokines in soluble, circulating form. Further, EV packaging may facilitate cytokine delivery and targeting to distant cells. This could be mediated by binding of EV-surface cytokines to cells that express specific cytokine receptors. Another possibility is that EVs protect cytokines from environmental degradation. Indeed, Fitzgerald et al. found that EV-associated cytokines were protected from trypsin digestion, as compared to soluble cytokines (12). This protection extends to cytokines bound to the surface of EVs as well, since an 189 amino acid isoform of VEGF was found to associate with heparin on the surface of small cancer-derived EVs, resulting in reduced recognition by the VEGF antibody bevacizumab (18). Interestingly, these data suggest a mechanism by which vesicle surface-bound VEGF contributes to bevacizumab resistance in cancer patients that is likely different than soluble VEGF function. Further, synovial fibroblasts from patients with rheumatoid arthritis were shown to release EVs that express membrane-associated TNF that reduces the activation-induced cell death of CD4+ T cells (19). Differences in biologic function between soluble and membrane-bound cytokine receptors have been relatively well-characterized in the literature, showing that soluble receptors will often act as antagonists to membrane-bound forms (20, 21). However, comparatively little is still known regarding the different biologic functions of soluble vs. vesicle membrane-bound cytokines. As a result, the mechanism(s) by which cytokines are packaged in EVs, by internalization as vesicle cargo or expression on the vesicle surface, and how they are released from EVs, through lysis or uptake by a target cell, all contribute to the complex mechanisms of normal (healthy) and disease-related cytokine signaling.
Although the previous 5–10 years have shown rapid advancements in the field of EV research, there remain a number of unanswered questions regarding differential biological outcomes from cytokines (and other proteins) released by EVs into the microenvironment. For instance, Rana et al. reported that poly(I:C) could induce the release of both soluble and EV-secreted IL36γ from keratinocytes (22). The authors postulated that these two mechanisms of cytokine release may modulate both local and systemic immune responses to viruses and other pathogens. However, it remains unknown whether soluble and packaged IL36γ have different biological functions on target cells. Moreover, it is not currently known whether cytokine signaling in a target cell is altered dependent on how the target cell “sees” the cytokine. This lack of knowledge is partially due to the fact that multiple mechanisms exist for how target cells interact with EVs, thus adding to the complexity of our understanding of differential function [reviewed in (23)].
Cell Type and Physiologic Status Determine Cytokine Packaging
As alluded to above, Fitzgerald et al. recently reported that medium from cultured cells and tissue explants, as well as body fluids, contained different amounts of EVs with different levels and types of cytokines. Importantly, they found that the distribution of cytokines between soluble and EV-associated forms was largely dependent on the cellular system rather than the cytokine being secreted. For example, tissue explants that contain cells in close proximity to other cells normally found in their in vivo microenvironments tended to release more cytokines in soluble form than were found in T cell or monocyte suspensions or in plasma. Indeed, a greater proportion of EV-associated cytokines were found from the cells and plasma. However, upon stimulation of cells, they found that the number and pattern of cytokines packaged in EVs changed depending on the stimulus, suggesting that the packaging of cytokines in EVs is not simply the property of a particular cytokine, but rather a tightly controlled biological process. For instance, stimulation of tonsillar explants with pokeweed mitogen resulted in a drastic change in the pattern of cytokine release with a shift toward more soluble secretion rather than EV-associated secretion. In contrast, human primary monocytes stimulated with either LPS or polyI:C resulted in more EVs being secreted with different patterns of cytokines associated with EVs; distinct patterns of soluble vs. EV-associated cytokine secretion were also detected between the two stimuli (6, 12).
Stimulation of human umbilical cord blood-derived mast cells by cross-linkage of FcIgE receptors (FcεRI) induces the release of granule-associated mediators such as histamine, metabolites, and cytokines (24–26). Kandere-Grzybowska et al. found that stimulation of human mast cells with IL1 rather than FcεRI cross-linking resulted in the exclusion of IL6 from secretory granules, and instead found that IL6 was secreted in 40-80 nm vesicular structures (27). Similarly, a number of reports have been recently published showing that exosomes from the plasma of HIV-infected individuals have distinct levels and types of cytokines as compared to exosomes from healthy donors (28–30). Interestingly, in patients with diabetes, the association of specific cytokines with EVs was found to be strongly influenced by disease duration and treatment outcome (31). Altogether, these data support that EV-associated cytokine loading and secretion may be directed in a cell type- and stimuli-dependent manner.
Biological Activity of EV-Associated Cytokines
In the mid-1990s, EVs secreted from B cells were shown to have an immunological function in antigen presentation and as vesicles that can induce T cell responses (32–34). We now know that one of the mechanisms by which EVs elicit immunological function is that they can serve as alternate carriers for the delivery of cytokines. Immunologically, EVs maintain characteristics of the antigen presenting cell (APC) that they were derived from, exposing the extracellular domain of major histocompatibility complex (MHC) molecules at the vesicle surface. Thus, EVs released by APCs carrying surface MHC Class I and MHC Class II can directly stimulate CD8+ and CD4+ T cells, respectively [reviewed in (33)]. Of note, EVs are also generated from immunosuppressive APCs. For instance, autologous EVs isolated from plasma shortly after antigen (Ag) stimulation could be used to induce Ag-specific immunosuppression (35, 36). Further, EVs isolated from bronchoalveolar lavage fluid following Ag-specific exposure could be used to prevent Ag-specific allergic responses (37, 38). Last, EVs present in human breast milk and colostrum were found to increase the number of T regulatory (Treg) cells and thus could be used to suppress immune responses (37). In this context, pregnancy has been shown to alleviate the severity of some autoimmune diseases, such as rheumatoid (RA) arthritis and multiple sclerosis (MS) (39).
Given the small size of EVs, they are capable of crossing major biological barriers such as the blood-brain barrier, and thus provide interesting prospects for therapeutic packaging and regulation (40–42). It is now well-recognized that EVs have a wide range of pleiotropic functions in multiple biological processes. For example, in an in vitro model of cardiovascular disease, EVs isolated from TNFα-induced human vascular endothelial cells (HUVEC) were taken up by monocytes and un-induced HUVEC, promoting an inflammatory response (13, 43, 44). Hosseinkhani et al. reported a select increase in IL6, IL8, and ICAM1 levels in un-induced HUVEC after co-incubation with EVs isolated from TNFα-induced HUVEC, while THP1 cells showed an increase in ICAM1, MIP1β, CCL5, and CXCL10 levels (13). The change in THP1 inflammatory mediators by EVs led to an increase in monocyte adhesion and migratory function. Another interesting study reported that exosomes isolated from mesenchymal stem cells (MSCs) of human umbilical cord treated with interferon (IFN)γ or a combination of TGFβ plus IFNγ contained increased levels of TGFβ, IDO, IL10, and IFNγ that, when incubated with PBMCs, resulted in increased numbers of Tregs (45).
In HIV-positive individuals, cytokines were found to be markedly enriched in exosomes and exposure of these exosomes to purified naïve peripheral blood mononuclear cells (PBMCs) resulted in the induction of CD38 expression on naïve and central memory CD4+ and CD8+ T cells, likely contributing to viral propagation via activation of bystander cells (30). An independent study characterizing plasma EVs from HIV-positive individuals found increased oxidative stress markers that correlated with an IFN gene signature and immune activation (28). Another interesting immunologic function for EVs was discovered in the placenta as a mechanism to regulate immunity against the fetus during pregnancy. Holder et al. reported that macrophage-derived exosomes containing IL6 and IL8 were actively transported into the human placenta to stimulate placental cytokines (46).
EV-Associated Cytokines in Autoimmune Disease
Recent evidence supports that EVs can mediate immune stimulation or suppression and can drive inflammatory, autoimmune and infectious disease pathology (47–49). One of the mechanisms by which EVs can drive autoimmune disease is that they serve as carriers of pathogen-associated and damage-associated molecular patterns (PAMPs and DAMPs, respectively), as well as cytokines, autoantigens and tissue-degrading enzymes (48). Indeed, synovial EVs from patients with RA were found to contain citrullinated proteins and, in the autoimmune disease systemic lupus erythematosus (SLE), EVs could serve as autoantigens in the formation of immune complexes (50–54). In addition, cytokines, such as IL6, are highly implicated in the development and progression of multiple autoimmune diseases whose production can be regulated by EV packaging and secretion. The role of IL6 in autoimmune disease pathogenesis is due in part to its influence on CD4+ T cell lineage and regulation [reviewed in (55)]. We provide examples in the above sections of how IL6 packaging and secretion in EVs can be regulated by different stimuli.
Another cytokine that contributes to autoimmune disease pathogenesis is TNFα. High levels of circulating TNFα are a major driver of RA. Interestingly, a membrane-bound form of TNFα was recently detected from individuals with osteoarthritis (19). The premise that EVs package cytokines that contribute to the amplification of an immune response was supported by work from Obregon et al. revealing the presence of large amounts of TNFα packaged into EVs derived from LPS-activated dendritic cells (DCs). These EVs also contained MHC II, CD40, CD83, TNFR1, and TNFR2 and were internalized by epithelial cells that became activated to release cytokines and chemokines such as IL8, MCP1, MIP1β, RANTES, and TNFα (56). In another related study, Zhang et al. identified a membrane bound form of TNFα on exosomes produced from synovial fibroblasts of patients with RA. These exosomes were found to activate Akt and NFκB pathways and rendered T cells resistant to undergo apoptosis; the authors proposed that this contributed to T cell-mediated pathology in RA (19). Figure 1 provides an overview of these mechanisms through which EVs expressing TNFα modulate autoimmunity.
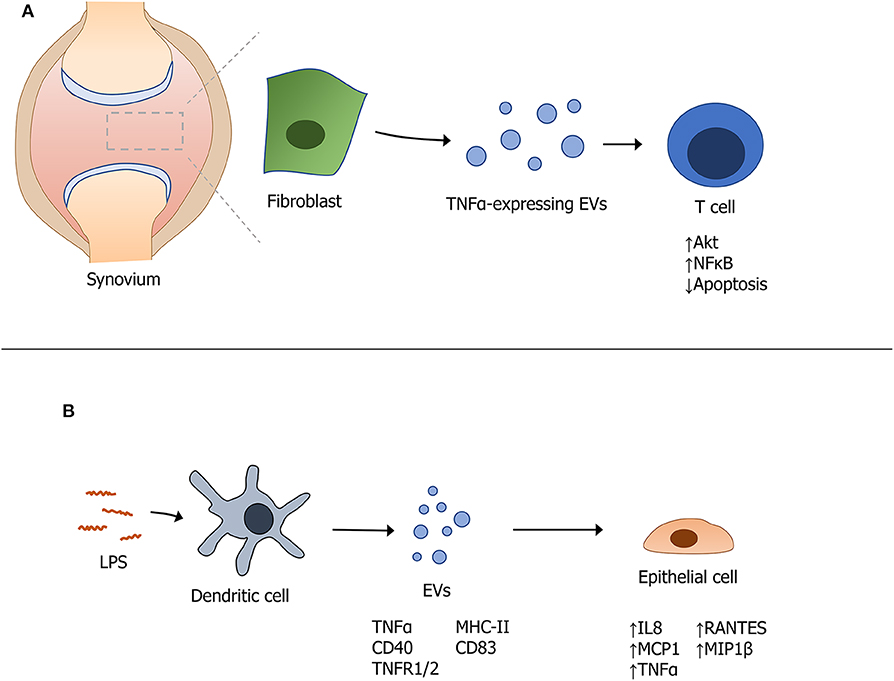
Figure 1. Modulation of autoimmunity by extracellular vesicles expressing TNFα. (A) Fibroblast-derived EVs containing TNFα modulate T cell function in the synovium of patients with rheumatoid arthritis. (B) Stimulation of dendritic cells with LPS induces the packaging and secretion of specific EV-associated cytokines that themselves, induce a downstream effect(s) on cytokine production from epithelial cells.
IL1β is a pro-inflammatory cytokine that has stimulatory effects and helps promote the differentiation of CD4+ T cells into T helper 1 (Th1) and Th17 lineages, both of which are known to contribute to autoimmune disease pathogenesis (57, 58). The release of the active form of IL1β follows a finely regulated process [(59); reviewed in (60)] and we now know that EV production plays a role in the maturation process of IL1β (61–66). This is dependent on the formation of the inflammasome, a multiprotein complex of innate immunity that is also involved in the secretion and loading of proteins associated with vesicles (67, 68). Different types of stimuli have been reported to promote inflammasome activation resulting in IL1β secretion via EVs, such as extracellular ATP that serves as a strong activator of the NRLP3 inflammasome, resulting in increased release of EVs (65, 69). Another stimulus is ionic fluxes that cause membrane polarization. It has been well-established that Ca2+ influx causes inflammasome activation and vesicular production. Ca2+ influx also induces the activation of different calcium-dependent proteins involved in membrane and cytoskeletal modification, thus facilitating the release of EVs (70). Last, a non-canonical route for inflammasome activation and the maturation of IL1β involves caspase 4/5, which directly recognize intracellular LPS. Caspase 4/5-mediated activation of the inflammasome strongly induces the release of IL1β, IL18, and other EV-associated cytokines (63).
High serum levels of type I (IFNα), II (IFNγ), and III (IFNλ1) are observed in patients with SLE and have been associated with high disease activity; thus, IFNs are considered to be key molecules in the pathogenesis of SLE (71–74). Interestingly, before EVs were identified as entities with physiologic function, it was well-known that IFNs were able to affect enveloped virus budding, release, and infectivity by increasing the expression of genes encoding restriction factors, such as ISG15 that has regulatory functions in EV packaging and secretion (75). ISG15 is an IFN stimulated gene (ISG) and an ubiquitin-like modifier (76–78). It has been identified in microvesicles and exosomes originating from TLR3 (polyI:C)-activated human brain microvascular endothelial cells (79). Importantly, ISG15 was found to ISGylate TSG101, which is a component of the ESCRT-I complex that mediates ESCRT-dependent EV secretion [reviewed in (10, 14, 80)]. Thus, not surprisingly, ISGylation was reported to influence exosome secretion. Villarroya-Beltri et al. revealed that type I IFNs trigger TSG101 modification via ISG15 that results in TSG101 degradation and impaired exosome secretion (81, 82). They reported that ISGylation of TSG101 triggers MVB co-localization with lysosomes, thus promoting the aggregation and degradation of MVB proteins, and the ultimate impairment of exosome secretion (81). Relevant to type I and II IFNs, the transcription factor interferon regulatory factor 1 (IRF1) was found to regulate select GTPases, such as Rab27a that is a key factor in EV secretion. Yang et al. found that IFNγ-induced IRF1 upregulation promoted Rab27a expression and EV secretion; conversely, knockdown of IRF1 or Rab27a resulted in reduced EV secretion (83). In addition to contributing to the regulation of EV secretion, IFNs also contribute to the packaging of its cellular components [reviewed in (6)].
EV-Associated Cytokines in Cancer
In cancer, tumor-derived EVs have been shown to play roles in immune evasion and metastatic progression (84–87). One of the first studies revealed that vaccination of mice with exosomes isolated from tumor peptide-pulsed DCs primed tumor-specific cytotoxic T cells and suppressed tumor growth in a T cell-dependent manner (88). Similarly, Seo et al. found that EVs released from activated CD8+ T cells of healthy mice were capable of attenuating tumor invasion and metastasis by apoptotic depletion of mesenchymal tumor stromal cells (89). Subsequent studies of EVs secreted from melanoma and prostate cancer cells revealed that they express programmed death-ligand 1 (PD-L1) on their surface, which suppresses the function of CD8+ T cells and facilitates tumor growth (90–93). The level of PD-L1 expression was found to correlate with disease stage, and was increased by IFNγ stimulation (94). Importantly, the associated suppression of CD8+ T cell response by exosomal PD-L1 could be abrogated by treatment with PD-1 or PD-L1 inhibitors to induce immune-mediated reduction of tumor growth.
Most solid tumors exhibit increased release of EVs, accompanied by alterations in their composition of proteins, lipids, and genetic material (95, 96). As a result, tumor-derived EVs have diverse effects on tumor growth, invasion, metastasis, and immune response, in part, through their modulation of cytokine production by cells of the innate and adaptive immune system (86). The complex interplay between the diverse array of cells in the tumor microenvironment and the pleiotropic factors that are secreted, is the subject of extensive current research, and our knowledge of exactly how these cells and mediators interact is incomplete. Nonetheless, it is clear that EVs promote tumor growth and progression in most solid tumors, highlighting the importance of these mediators in tumor-immune regulation.
Tumor-associated macrophages (TAMs) are major regulators of inflammation and immune response in the tumor microenvironment and are thus important targets of tumor-derived EVs. Crosstalk between tumor-derived EVs and macrophages can polarize them toward a more M2-like, pro-tumor TAM (97), which is associated with higher levels of the immunosuppressive cytokines IL10, IL4, and TGFβ. However, EVs can also promote tumor progression through an increase in pro-inflammatory functions of macrophages. Wu et al. found that exosomes secreted by gastric tumors were capable of inducing pro-inflammatory signaling in macrophages via activation of NF-κB, thereby promoting tumor growth and invasion (98). Similarly, breast cancer exosomes were found to induce macrophage-mediated secretion of the cytokines TNFα, IL6, and MCP1, which stimulate tumor progression and metastasis (99, 100). Increased IL6 production mediated by tumor-derived exosomes results in suppressed dendritic cell activity and attenuated immune response, resulting in enhanced tumor growth (101).
Tumor-derived exosomes also promote tumor growth through the stimulation of myeloid-derived suppressor cells (MDSCs), which have immunosuppressive effects in tumors. Multiple cancer types have been found to secrete exosomes containing heat shock proteins, Hsp72 and Hsp90, which activate Stat3 in MDSCs via IL6 and promote immunosuppression and tumor growth (85). Exosomes isolated from B16 melanoma tumors in mice were shown to stimulate MDSCs to produce TNFα, MCP1, and IL6 in a MyD88-dependent manner, which promotes immunosuppression, tumor growth, and metastasis (23). Not surprisingly, these same pro-inflammatory cytokines were implicated in ovarian cancer, in which exosomes isolated from the body fluids of patients induced production of IL1β, TNFα, and IL6 by THP-1 monocytes (102).
Last, tumor-derived EVs have been implicated in the development of pre-metastatic niche (PMN) formation in a variety of cancers [recently reviewed in (103)]. Results from pancreatic cancer, breast cancer, ovarian cancer, and melanoma, among others, highlights the importance of EVs in regulating intercellular communication at sites distant from the primary tumor (104). For example, in a well-characterized model of pancreatic cancer, tumors were found to secrete exosomes containing macrophage migration inhibitory factor (MIF), which induces TGFβ signaling in Kupffer cells in the liver. This resulted in increased production of fibronectin by hepatic stellate cells, creating an environment that is more permissive to metastatic colonization by tumor cells (105). In response to the hypoxic microenvironment that is present in most solid tumors, many types of cancers were found to promote endothelial growth through the release of pro-angiogenic factors, such as VEGF, that can also be packaged inside exosome (18, 106, 107). A summary of EV-mediated regulation of cytokine production by cells in the tumor microenvironment is provided in Figure 2.
Modulating EV Secretion as a Mechanism to Control Cytokine Release
Although EVs are released in resting cells, stimulating events, such as cell activation, leads to increased intracellular calcium levels, resulting in cellular membrane remodeling and enhanced EV secretion (108). Pharmacologic modulation of EV output can be achieved through treatment with agents that interfere with cytoskeletal remodeling that is required for the formation of MVBs and trafficking of proteins into vesicles for their subsequent release (10, 80). Calpains are a family of calcium-dependent cysteine proteases that are important for unconventional protein secretion and inflammasome activation (65). Inhibition of calpain with a small-molecule inhibitor, such as MDL28170, blocks vesicular formation and the subsequent release of EVs (65). Given the role of caspase 4/5 in inflammasome activation and release of IL1β-associated EVs, the use of a caspase 4 inhibitor was found to block EV secretion from LPS-stimulated human macrophages (63). Treatment of cells/tissues with the microbial metabolite Manumycin A, a farnesyltransferase inhibitor, resulted in decreased EV biogenesis and secretion via modulation of ESCRT machinery (12, 109). A similar pharmacologic approach is to inhibit the formation of MVBs by inhibiting sphingomyelinase activity. Sphingomyelinases are required for the inward budding and eventual release of MVBs through an ESCRT-independent pathway. GW4869 is a neutral sphingomyelinase inhibitor that inhibits vesicle formation (110). Last, simvastatin was recently identified as an inhibitor of EV secretion based on the rationale that cholesterol is necessary for the formation of vesicle membranes. However, simvastatin's function as an HMG-CoA reductase inhibitor does not entirely explain the mechanism, as supplementation with mevalonate did not fully restore EV output to baseline levels (111). Given that different mechanisms of EV biogenesis exist, we may utilize this knowledge to selectively target (inhibit) specific populations of EVs while leaving other subsets of EVs untouched.
As our current understanding of the mechanisms that differentially regulate the packaging of cytokines in EVs from resting and activated cells expands, this knowledge may be also used to preferentially drive the packaging of distinct groups of cytokines into EVs for therapeutic use. For instance, DCs can be stimulated to secrete EVs that induce the differentiation of immunosuppressive Tregs for the treatment of autoimmune disease (112–116). The regulation of T cell differentiation to immunosuppressive states is already under consideration for the treatment of autoimmune disease (49, 117, 118). Last, determination of the molecular machinery required for EV-associated cytokine secretion, such as ESCRT-dependent or -independent and autophagy-dependent, will provide critical information on select treatments that may target specific pathways [recently reviewed in (119)].
Engineering EVs to Therapeutically Deliver Cytokines
EV encapsulation of cytokines may facilitate their delivery and targeting to distant cells (34). Recent work has demonstrated the feasibility of engineering EVs to take up proteins as cargo (120–122), presenting a number of techniques by which EVs could be artificially generated to carry cytokine payloads to distant sites. An advantage to this method is that it does not require a priori knowledge of the biogenesis pathway resulting in EV cargo loading and secretion. Alternatively, EVs may be targeted to specific cells via binding of EV surface cytokines to cells that express the specific cytokine receptor (123). Sialic-acid binding immunoglobulin lectins, C-type lectins, lactadherin, MHC I, and II receptors, transferrin receptors, tetraspanins, and viral proteins have all been identified as molecules that may promote EV targeting (124–129). Thus, enrichment of exosomes on the basis of their surface ligand expression or ligand enrichment on engineered EVs may be used to induce or inhibit signaling events in recipient cells or to develop receptor-mediated tissue (and cell) targeting (80). Here, we provide two examples of how EVs can be therapeutically modulated for packaging of specific cytokines that drive an immune response. The first example is treatment of bone marrow-derived mast cells with IL4 to drive secretion of exosomes that express MHC II, CD86, LFA-1, and ICAM1, resulting in activation of the adaptive immune arm by inducing proliferation of B and T cells in vitro and in vivo (130). The second example is from engineering tumor cells to overexpress CD40L, resulting in tumor-derived exosomes that overexpress CD40L to promote dendritic cell maturation, resulting in increased T cell proliferation and antitumor activity in vivo (131).
While technological advances in isolating, characterizing, and now engineering EVs to deliver therapeutic payloads and immune modulators are being made [recently reviewed in (80)], it is not until the biological mechanisms by which cytokines are selectively packaged into EVs and the molecular machinery required for secretion determined, that we will be able to fully harness the potential of this natural, physiologic mechanism for cytokine modulation in the context of disease.
Author Contributions
BB and CS planned and prepared the manuscript.
Funding
This work was funded in part by the Department of Defense BCRP award W81XWH-19-1-0113 and CDMRP LRP award W81XWH-18-1-0674 to BB.
Conflict of Interest
The authors declare that the research was conducted in the absence of any commercial or financial relationships that could be construed as a potential conflict of interest.
References
1. Raposo G, Stoorvogel W. Extracellular vesicles: exosomes, microvesicles, and friends. J Cell Biol. (2013) 200:373–83. doi: 10.1083/jcb.201211138
2. Stahl PD, Raposo G. Extracellular vesicles: exosomes and microvesicles, integrators of homeostasis. Physiology. (2019) 34:169–77. doi: 10.1152/physiol.00045.2018
3. Gulati K, Guhathakurta S, Joshi J, Rai N, Ray A. Cytokines and their role in health and disease: a brief overview. MOJ Immunol. (2016) 4:1–9. doi: 10.15406/moji.2016.04.00121
4. Dinarello CA. Historical insights into cytokines. Eur J Immunol. (2007) 37(Suppl. 1):S34–45. doi: 10.1002/eji.200737772
5. Lacy P, Stow JL. Cytokine release from innate immune cells: association with diverse membrane trafficking pathways. Blood. (2011) 118:9–18. doi: 10.1182/blood-2010-08-265892
6. Aiello A, Giannessi F, Percario ZA, Affabris E. An emerging interplay between extracellular vesicles and cytokines. Cytokine Growth Factor Rev. (2020) 51:49–60. doi: 10.1016/j.cytogfr.2019.12.003
7. Burgoyne RD, Morgan A. Secretory granule exocytosis. Physiol Rev. (2003) 83:581–632. doi: 10.1152/physrev.00031.2002
8. Ferro-Novick S, Brose N. Nobel 2013 Physiology or medicine: traffic control system within cells. Nature. (2013) 504:98. doi: 10.1038/504098a
9. Stow JL, Murray RZ. Intracellular trafficking and secretion of inflammatory cytokines. Cytokine Growth Factor Rev. (2013) 24:227–39. doi: 10.1016/j.cytogfr.2013.04.001
10. Jadli AS, Ballasy N, Edalat P, Patel VB. Inside(sight) of tiny communicator: exosome biogenesis, secretion, and uptake. Mol Cell Biochem. (2020) 467:77–94. doi: 10.1007/s11010-020-03703-z
11. van Niel G, D'Angelo G, Raposo G. Shedding light on the cell biology of extracellular vesicles. Nat Rev Mol Cell Biol. (2018) 19:213–28. doi: 10.1038/nrm.2017.125
12. Fitzgerald W, Freeman ML, Lederman MM, Vasilieva E, Romero R, Margolis L. A system of cytokines encapsulated in extracellular vesicles. Sci Rep. (2018) 8:8973. doi: 10.1038/s41598-018-27190-x
13. Hosseinkhani B, Kuypers S, van den Akker NMS, Molin DGM, Michiels L. Extracellular vesicles work as a functional inflammatory mediator between vascular endothelial cells and immune cells. Front Immunol. (2018) 9:1789. doi: 10.3389/fimmu.2018.01789
14. Leidal AM, Debnath J. Unraveling the mechanisms that specify molecules for secretion in extracellular vesicles. Methods. (2020) 177:15–26. doi: 10.1016/j.ymeth.2020.01.008
15. Leidal AM, Huang HH, Marsh T, Solvik T, Zhang D, Ye J, et al. The LC3-conjugation machinery specifies the loading of RNA-binding proteins into extracellular vesicles. Nat Cell Biol. (2020) 22:187–99. doi: 10.1038/s41556-019-0450-y
16. Thery C, Witwer KW, Aikawa E, Alcaraz MJ, Anderson JD, Andriantsitohaina R, et al. Minimal information for studies of extracellular vesicles 2018 (MISEV2018): a position statement of the International Society for Extracellular Vesicles and update of the MISEV2014 guidelines. J Extracell Vesicles. (2018) 7:1535750. doi: 10.1080/20013078.2018.1535750
17. Tkach M, Thery C. Communication by extracellular vesicles: where we are and where we need to go. Cell. (2016) 164:1226–32. doi: 10.1016/j.cell.2016.01.043
18. Ko SY, Lee W, Kenny HA, Dang LH, Ellis LM, Jonasch E, et al. Cancer-derived small extracellular vesicles promote angiogenesis by heparin-bound, bevacizumab-insensitive VEGF, independent of vesicle uptake. Commun Biol. (2019) 2:386. doi: 10.1038/s42003-019-0609-x
19. Zhang HG, Liu C, Su K, Yu S, Zhang L, Zhang S, et al. A membrane form of TNF-alpha presented by exosomes delays T cell activation-induced cell death. J Immunol. (2006) 176:7385–93. doi: 10.4049/jimmunol.176.12.7385
20. Levine SJ. Molecular mechanisms of soluble cytokine receptor generation. J Biol Chem. (2008) 283:14177–81. doi: 10.1074/jbc.R700052200
21. Rose-John S, Scheller J, Elson G, Jones SA. Interleukin-6 biology is coordinated by membrane-bound and soluble receptors: role in inflammation and cancer. J Leukoc Biol. (2006) 80:227–36. doi: 10.1189/jlb.1105674
22. Rana AA, Lucs AV, DeVoti J, Blanc L, Papoin J, Wu R, et al. Poly(I:C) induces controlled release of IL-36γ from keratinocytes in the absence of cell death. Immunol Res. (2015) 63:228–35. doi: 10.1007/s12026-015-8692-7
23. Liu Y, Xiang X, Zhuang X, Zhang S, Liu C, Cheng Z, et al. Contribution of MyD88 to the tumor exosome-mediated induction of myeloid derived suppressor cells. Am J Pathol. (2010) 176:2490–9. doi: 10.2353/ajpath.2010.090777
24. Galli SJ. New concepts about the mast cell. N Engl J Med. (1993) 328:257–65. doi: 10.1056/nejm199301283280408
25. Kobayashi H, Ishizuka T, Okayama Y. Human mast cells and basophils as sources of cytokines. Clin Exp Allergy. (2000) 30:1205–12. doi: 10.1046/j.1365-2222.2000.00808.x
26. Metcalfe DD, Baram D, Mekori YA. Mast cells. Physiol Rev. (1997) 77:1033–79. doi: 10.1152/physrev.1997.77.4.1033
27. Kandere-Grzybowska K, Letourneau R, Kempuraj D, Donelan J, Poplawski S, Boucher W, et al. IL-1 induces vesicular secretion of IL-6 without degranulation from human mast cells. J Immunol. (2003) 171:4830–6. doi: 10.4049/jimmunol.171.9.4830
28. Chettimada S, Lorenz DR, Misra V, Dillon ST, Reeves RK, Manickam C, et al. Exosome markers associated with immune activation and oxidative stress in HIV patients on antiretroviral therapy. Sci Rep. (2018) 8:7227. doi: 10.1038/s41598-018-25515-4
29. Kodidela S, Ranjit S, Sinha N, McArthur C, Kumar A, Kumar S. Cytokine profiling of exosomes derived from the plasma of HIV-infected alcohol drinkers and cigarette smokers. PLoS ONE. (2018) 13:e0201144. doi: 10.1371/journal.pone.0201144
30. Konadu KA, Chu J, Huang MB, Amancha PK, Armstrong W, Powell MD, et al. Association of cytokines with exosomes in the plasma of HIV-1-seropositive individuals. J Infect Dis. (2015) 211:1712–6. doi: 10.1093/infdis/jiu676
31. Tokarz A, Konkolewska M, Kusnierz-Cabala B, Maziarz B, Hanarz P, Zurakowski A, et al. Retinopathy severity correlates with RANTES concentrations and CCR 5-positive microvesicles in diabetes. Folia Med Cracov. (2019) 59:95–112. doi: 10.24425/fmc.2019.131139
32. Raposo G, Nijman HW, Stoorvogel W, Liejendekker R, Harding CV, Melief CJ, et al. B lymphocytes secrete antigen-presenting vesicles. J Exp Med. (1996) 183:1161–72. doi: 10.1084/jem.183.3.1161
33. Robbins PD, Morelli AE. Regulation of immune responses by extracellular vesicles. Nat Rev Immunol. (2014) 14:195–208. doi: 10.1038/nri3622
34. Yuana Y, Sturk A, Nieuwland R. Extracellular vesicles in physiological and pathological conditions. Blood Rev. (2013) 27:31–9. doi: 10.1016/j.blre.2012.12.002
35. Kim SH, Bianco NR, Shufesky WJ, Morelli AE, Robbins PD. MHC class II+ exosomes in plasma suppress inflammation in an antigen-specific and Fas ligand/Fas-dependent manner. J Immunol. (2007) 179:2235–41. doi: 10.4049/jimmunol.179.4.2235
36. Ostman S, Taube M, Telemo E. Tolerosome-induced oral tolerance is MHC dependent. Immunology. (2005) 116:464–76. doi: 10.1111/j.1365-2567.2005.02245.x
37. Admyre C, Grunewald J, Thyberg J, Gripenback S, Tornling G, Eklund A, et al. Exosomes with major histocompatibility complex class II and co-stimulatory molecules are present in human BAL fluid. Eur Respir J. (2003) 22:578–83. doi: 10.1183/09031936.03.00041703
38. Prado N, Marazuela EG, Segura E, Fernandez-Garcia H, Villalba M, Thery C, et al. Exosomes from bronchoalveolar fluid of tolerized mice prevent allergic reaction. J Immunol. (2008) 181:1519–25. doi: 10.4049/jimmunol.181.2.1519
39. Sabapatha A, Gercel-Taylor C, Taylor DD. Specific isolation of placenta-derived exosomes from the circulation of pregnant women and their immunoregulatory consequences. Am J Reprod Immunol. (2006) 56:345–55. doi: 10.1111/j.1600-0897.2006.00435.x
40. Alvarez-Erviti L, Seow Y, Yin H, Betts C, Lakhal S, Wood MJ. Delivery of siRNA to the mouse brain by systemic injection of targeted exosomes. Nat Biotechnol. (2011) 29:341–5. doi: 10.1038/nbt.1807
41. Osorio-Querejeta I, Alberro A, Munoz-Culla M, Mager I, Otaegui D. Therapeutic potential of extracellular vesicles for demyelinating diseases; challenges and opportunities. Front Mol Neurosci. (2018) 11:434. doi: 10.3389/fnmol.2018.00434
42. Yang T, Martin P, Fogarty B, Brown A, Schurman K, Phipps R, et al. Exosome delivered anticancer drugs across the blood-brain barrier for brain cancer therapy in Danio rerio. Pharm Res. (2015) 32:2003–14. doi: 10.1007/s11095-014-1593-y
43. Gaceb A, Martinez MC, Andriantsitohaina R. Extracellular vesicles: new players in cardiovascular diseases. Int J Biochem Cell Biol. (2014) 50:24–8. doi: 10.1016/j.biocel.2014.01.018
44. Loyer X, Vion AC, Tedgui A, Boulanger CM. Microvesicles as cell-cell messengers in cardiovascular diseases. Circ Res. (2014) 114:345–53. doi: 10.1161/CIRCRESAHA.113.300858
45. Chen Z, Luo J, Zhang C, Ma Y, Sun S, Zhang T, et al. Mechanism of prolactin inhibition of miR-135b via methylation in goat mammary epithelial cells. J Cell Physiol. (2018) 233:651–62. doi: 10.1002/jcp.25925
46. Holder B, Jones T, Sancho Shimizu V, Rice TF, Donaldson B, Bouqueau M, et al. Macrophage exosomes induce placental inflammatory cytokines: a novel mode of maternal-placental messaging. Traffic. (2016) 17:168–78. doi: 10.1111/tra.12352
47. Bobrie A, Colombo M, Raposo G, Thery C. Exosome secretion: molecular mechanisms and roles in immune responses. Traffic. (2011) 12:1659–68. doi: 10.1111/j.1600-0854.2011.01225.x
48. Buzas EI, Gyorgy B, Nagy G, Falus A, Gay S. Emerging role of extracellular vesicles in inflammatory diseases. Nat Rev Rheumatol. (2014) 10:356–64. doi: 10.1038/nrrheum.2014.19
49. Katsiougiannis S. Extracellular vesicles: evolving contributors in autoimmunity. For Immunopathol Dis Therap. (2015) 6:163–70. doi: 10.1615/ForumImmunDisTher.2016016491
50. Cloutier N, Tan S, Boudreau LH, Cramb C, Subbaiah R, Lahey L, et al. The exposure of autoantigens by microparticles underlies the formation of potent inflammatory components: the microparticle-associated immune complexes. EMBO Mol Med. (2013) 5:235–49. doi: 10.1002/emmm.201201846
51. Nielsen CT, Ostergaard O, Stener L, Iversen LV, Truedsson L, Gullstrand B, et al. Increased IgG on cell-derived plasma microparticles in systemic lupus erythematosus is associated with autoantibodies and complement activation. Arthritis Rheum. (2012) 64:1227–36. doi: 10.1002/art.34381
52. Pisetsky DS. Microparticles as autoantigens: making immune complexes big. Arthritis Rheum. (2012) 64:958–61. doi: 10.1002/art.34377
53. Skriner K, Adolph K, Jungblut PR, Burmester GR. Association of citrullinated proteins with synovial exosomes. Arthritis Rheum. (2006) 54:3809–14. doi: 10.1002/art.22276
54. Ullal AJ, Reich CF III, Clowse M, Criscione-Schreiber LG, Tochacek M, Monestier M, Pisetsky DS. Microparticles as antigenic targets of antibodies to DNA and nucleosomes in systemic lupus erythematosus. J Autoimmun. (2011) 36:173–80. doi: 10.1016/j.jaut.2011.02.001
55. Jones BE, Maerz MD, Buckner JH. IL-6: a cytokine at the crossroads of autoimmunity. Curr Opin Immunol. (2018) 55:9–14. doi: 10.1016/j.coi.2018.09.002
56. Obregon C, Rothen-Rutishauser B, Gerber P, Gehr P, Nicod LP. Active uptake of dendritic cell-derived exovesicles by epithelial cells induces the release of inflammatory mediators through a TNF-alpha-mediated pathway. Am J Pathol. (2009) 175:696–705. doi: 10.2353/ajpath.2009.080716
57. Casanova JL, Abel L, Quintana-Murci L. Human TLRs and IL-1Rs in host defense: natural insights from evolutionary, epidemiological, and clinical genetics. Annu Rev Immunol. (2011) 29:447–91. doi: 10.1146/annurev-immunol-030409-101335
58. Santarlasci V, Cosmi L, Maggi L, Liotta F, Annunziato F. IL-1 and T helper immune responses. Front Immunol. (2013) 4:182. doi: 10.3389/fimmu.2013.00182
59. Rubartelli A, Cozzolino F, Talio M, Sitia R. A novel secretory pathway for interleukin-1 beta, a protein lacking a signal sequence. EMBO J. (1990) 9:1503–10.
60. Sitia R, Rubartelli A. The unconventional secretion of IL-1beta: Handling a dangerous weapon to optimize inflammatory responses. Semin Cell Dev Biol. (2018) 83:12–21. doi: 10.1016/j.semcdb.2018.03.011
61. Cypryk W, Lorey M, Puustinen A, Nyman TA, Matikainen S. Proteomic and bioinformatic characterization of extracellular vesicles released from human macrophages upon influenza A virus infection. J Proteome Res. (2017) 16:217–27. doi: 10.1021/acs.jproteome.6b00596
62. Cypryk W, Ohman T, Eskelinen EL, Matikainen S, Nyman TA. Quantitative proteomics of extracellular vesicles released from human monocyte-derived macrophages upon beta-glucan stimulation. J Proteome Res. (2014) 13:2468–77. doi: 10.1021/pr4012552
63. Lorey MB, Rossi K, Eklund KK, Nyman TA, Matikainen S. Global characterization of protein secretion from human macrophages following non-canonical caspase-4/5 inflammasome activation. Mol Cell Proteomics. (2017) 16(4 Suppl. 1):S187–99. doi: 10.1074/mcp.M116.064840
64. Singhto N, Kanlaya R, Nilnumkhum A, Thongboonkerd V. Roles of macrophage exosomes in immune response to calcium oxalate monohydrate crystals. Front Immunol. (2018) 9:316. doi: 10.3389/fimmu.2018.00316
65. Valimaki E, Cypryk W, Virkanen J, Nurmi K, Turunen PM, Eklund KK, et al. Calpain activity is essential for ATP-driven unconventional vesicle-mediated protein secretion and inflammasome activation in human macrophages. J Immunol. (2016) 197:3315–25. doi: 10.4049/jimmunol.1501840
66. Valimaki E, Miettinen JJ, Lietzen N, Matikainen S, Nyman TA. Monosodium urate activates Src/Pyk2/PI3 kinase and cathepsin dependent unconventional protein secretion from human primary macrophages. Mol Cell Proteomics. (2013) 12:749–63. doi: 10.1074/mcp.M112.024661
67. Cypryk W, Nyman TA, Matikainen S. From inflammasome to exosome-does extracellular vesicle secretion constitute an inflammasome-dependent immune response? Front Immunol. (2018) 9:2188. doi: 10.3389/fimmu.2018.02188
68. Jo EK, Kim JK, Shin DM, Sasakawa C. Molecular mechanisms regulating NLRP3 inflammasome activation. Cell Mol Immunol. (2016) 13:148–59. doi: 10.1038/cmi.2015.95
69. MacKenzie A, Wilson HL, Kiss-Toth E, Dower SK, North RA, Surprenant A. Rapid secretion of interleukin-1beta by microvesicle shedding. Immunity. (2001) 15:825–35. doi: 10.1016/s1074-7613(01)00229-1
70. Kalra H, Drummen GP, Mathivanan S. Focus on extracellular vesicles: introducing the next small big thing. Int J Mol Sci. (2016) 17:170. doi: 10.3390/ijms17020170
71. Bengtsson AA, Sturfelt G, Truedsson L, Blomberg J, Alm G, Vallin H, et al. Activation of type I interferon system in systemic lupus erythematosus correlates with disease activity but not with antiretroviral antibodies. Lupus. (2000) 9:664–71. doi: 10.1191/096120300674499064
72. Munroe ME, Lu R, Zhao YD, Fife DA, Robertson JM, Guthridge JM, et al. Altered type II interferon precedes autoantibody accrual and elevated type I interferon activity prior to systemic lupus erythematosus classification. Ann Rheum Dis. (2016) 75:2014–21. doi: 10.1136/annrheumdis-2015-208140
73. Oke V, Brauner S, Larsson A, Gustafsson J, Zickert A, Gunnarsson I, et al. IFN-lambda1 with Th17 axis cytokines and IFN-alpha define different subsets in systemic lupus erythematosus (SLE). Arthritis Res Ther. (2017) 19:139. doi: 10.1186/s13075-017-1344-7
74. Rana A, Minz RW, Aggarwal R, Anand S, Pasricha N, Singh S. Gene expression of cytokines (TNF-alpha, IFN-gamma), serum profiles of IL-17 and IL-23 in paediatric systemic lupus erythematosus. Lupus. (2012) 21:1105–12. doi: 10.1177/0961203312451200
75. Hotter D, Kirchhoff F. Interferons and beyond: Induction of antiretroviral restriction factors. J Leukoc Biol. (2018) 103:465–77. doi: 10.1002/JLB.3MR0717-307R
76. Dos Santos PF, Mansur DS. Beyond ISGlylation: functions of free intracellular and extracellular ISG15. J Interferon Cytokine Res. (2017) 37:246–53. doi: 10.1089/jir.2016.0103
77. Dzimianski JV, Scholte FEM, Bergeron E, Pegan SD. ISG15: it's complicated. J Mol Biol. (2019) 431:4203–16. doi: 10.1016/j.jmb.2019.03.013
78. Perng YC, Lenschow DJ. ISG15 in antiviral immunity and beyond. Nat Rev Microbiol. (2018) 16:423–39. doi: 10.1038/s41579-018-0020-5
79. Sun L, Wang X, Zhou Y, Zhou RH, Ho WZ, Li JL. Exosomes contribute to the transmission of anti-HIV activity from TLR3-activated brain microvascular endothelial cells to macrophages. Antiviral Res. (2016) 134:167–71. doi: 10.1016/j.antiviral.2016.07.013
80. Kalluri R, LeBleu VS. The biology, function, and biomedical applications of exosomes. Science. (2020) 367:eaau6977. doi: 10.1126/science.aau6977
81. Villarroya-Beltri C, Baixauli F, Mittelbrunn M, Fernandez-Delgado I, Torralba D, Moreno-Gonzalo O, et al. ISGylation controls exosome secretion by promoting lysosomal degradation of MVB proteins. Nat Commun. (2016) 7:13588. doi: 10.1038/ncomms13588
82. Villarroya-Beltri C, Guerra S, Sanchez-Madrid F. ISGylation - a key to lock the cell gates for preventing the spread of threats. J Cell Sci. (2017) 130:2961–9. doi: 10.1242/jcs.205468
83. Yang MQ, Du Q, Goswami J, Varley PR, Chen B, Wang RH, et al. Interferon regulatory factor 1-Rab27a regulated extracellular vesicles promote liver ischemia/reperfusion injury. Hepatology. (2018) 67:1056–70. doi: 10.1002/hep.29605
84. Berchem G, Noman MZ, Bosseler M, Paggetti J, Baconnais S, Le Cam E, et al. Hypoxic tumor-derived microvesicles negatively regulate NK cell function by a mechanism involving TGF-beta and miR23a transfer. Oncoimmunology. (2016) 5:e1062968. doi: 10.1080/2162402X.2015.1062968
85. Chalmin F, Ladoire S, Mignot G, Vincent J, Bruchard M, Remy-Martin JP, et al. Membrane-associated Hsp72 from tumor-derived exosomes mediates STAT3-dependent immunosuppressive function of mouse and human myeloid-derived suppressor cells. J Clin Invest. (2010) 120:457–71. doi: 10.1172/jci40483
86. Greening DW, Gopal SK, Xu R, Simpson RJ, Chen W. Exosomes and their roles in immune regulation and cancer. Semin Cell Dev Biol. (2015) 40:72–81. doi: 10.1016/j.semcdb.2015.02.009
87. Xiang X, Poliakov A, Liu C, Liu Y, Deng ZB, Wang J, et al. Induction of myeloid-derived suppressor cells by tumor exosomes. Int J Cancer. (2009) 124:2621–33. doi: 10.1002/ijc.24249
88. Zitvogel L, Regnault A, Lozier A, Wolfers J, Flament C, Tenza D, et al. Eradication of established murine tumors using a novel cell-free vaccine: dendritic cell-derived exosomes. Nat Med. (1998) 4:594–600. doi: 10.1038/nm0598-594
89. Seo N, Shirakura Y, Tahara Y, Momose F, Harada N, Ikeda H, et al. Activated CD8(+) T cell extracellular vesicles prevent tumour progression by targeting of lesional mesenchymal cells. Nat Commun. (2018) 9:435. doi: 10.1038/s41467-018-02865-1
90. Chen G, Huang AC, Zhang W, Zhang G, Wu M, Xu W, et al. Exosomal PD-L1 contributes to immunosuppression and is associated with anti-PD-1 response. Nature. (2018) 560:382–6. doi: 10.1038/s41586-018-0392-8
91. Fan Y, Che X, Qu J, Hou K, Wen T, Li Z, et al. Exosomal PD-L1 retains immunosuppressive activity and is associated with gastric cancer prognosis. Ann Surg Oncol. (2019) 26:3745–55. doi: 10.1245/s10434-019-07431-7
92. Kim DH, Kim H, Choi YJ, Kim SY, Lee JE, Sung KJ, et al. Exosomal PD-L1 promotes tumor growth through immune escape in non-small cell lung cancer. Exp Mol Med. (2019) 51:94. doi: 10.1038/s12276-019-0295-2
93. Yang Y, Li CW, Chan LC, Wei Y, Hsu JM, Xia W, et al. Exosomal PD-L1 harbors active defense function to suppress T cell killing of breast cancer cells and promote tumor growth. Cell Res. (2018) 28:862–4. doi: 10.1038/s41422-018-0060-4
94. Theodoraki MN, Yerneni SS, Hoffmann TK, Gooding WE, Whiteside TL. Clinical significance of PD-L1(+) exosomes in plasma of head and neck cancer patients. Clin Cancer Res. (2018) 24:896–905. doi: 10.1158/1078-0432.Ccr-17-2664
95. Rabinowits G, Gercel-Taylor C, Day JM, Taylor DD, Kloecker GH. Exosomal microRNA: a diagnostic marker for lung cancer. Clin Lung Cancer. (2009) 10:42–6. doi: 10.3816/CLC.2009.n.006
96. Silva J, Garcia V, Rodriguez M, Compte M, Cisneros E, Veguillas P, et al. Analysis of exosome release and its prognostic value in human colorectal cancer. Genes Chromosomes Cancer. (2012) 51:409–18. doi: 10.1002/gcc.21926
97. Shinohara H, Kuranaga Y, Kumazaki M, Sugito N, Yoshikawa Y, Takai T, et al. Regulated polarization of tumor-associated macrophages by mir-145 via colorectal cancer-derived extracellular vesicles. J Immunol. (2017) 199:1505–15. doi: 10.4049/jimmunol.1700167
98. Wu L, Zhang X, Zhang B, Shi H, Yuan X, Sun Y, et al. Exosomes derived from gastric cancer cells activate NF-kappaB pathway in macrophages to promote cancer progression. Tumour Biol. (2016) 37:12169–80. doi: 10.1007/s13277-016-5071-5
99. Chow A, Zhou W, Liu L, Fong MY, Champer J, Van Haute D, et al. Macrophage immunomodulation by breast cancer-derived exosomes requires Toll-like receptor 2-mediated activation of NF-kappaB. Sci Rep. (2014) 4:5750. doi: 10.1038/srep05750
100. Maji S, Chaudhary P, Akopova I, Nguyen PM, Hare RJ, Gryczynski I, et al. Exosomal annexin II promotes angiogenesis and breast cancer metastasis. Mol Cancer Res. (2017) 15:93–105. doi: 10.1158/1541-7786.Mcr-16-0163
101. Yu S, Liu C, Su K, Wang J, Liu Y, Zhang L, et al. Tumor exosomes inhibit differentiation of bone marrow dendritic cells. J Immunol. (2007) 178:6867–75. doi: 10.4049/jimmunol.178.11.6867
102. Bretz NP, Ridinger J, Rupp AK, Rimbach K, Keller S, Rupp C, et al. Body fluid exosomes promote secretion of inflammatory cytokines in monocytic cells via Toll-like receptor signaling. J Biol Chem. (2013) 288:36691–702. doi: 10.1074/jbc.M113.512806
103. Guo Y, Ji X, Liu J, Fan D, Zhou Q, Chen C, et al. Effects of exosomes on pre-metastatic niche formation in tumors. Mol Cancer. (2019) 18:39. doi: 10.1186/s12943-019-0995-1
104. Peinado H, Zhang H, Matei IR, Costa-Silva B, Hoshino A, Rodrigues G, et al. Pre-metastatic niches: organ-specific homes for metastases. Nat Rev Cancer. (2017) 17:302–17. doi: 10.1038/nrc.2017.6
105. Costa-Silva B, Aiello NM, Ocean AJ, Singh S, Zhang H, Thakur BK, et al. Pancreatic cancer exosomes initiate pre-metastatic niche formation in the liver. Nat Cell Biol. (2015) 17:816–26. doi: 10.1038/ncb3169
106. Ribeiro MF, Zhu H, Millard RW, Fan GC. Exosomes function in pro- and anti-angiogenesis. Curr Angiogenes. (2013) 2:54–9. doi: 10.2174/22115528113020020001
107. Thompson CA, Purushothaman A, Ramani VC, Vlodavsky I, Sanderson RD. Heparanase regulates secretion, composition, and function of tumor cell-derived exosomes. J Biol Chem. (2013) 288:10093–9. doi: 10.1074/jbc.C112.444562
108. Cocucci E, Racchetti G, Meldolesi J. Shedding microvesicles: artefacts no more. Trends Cell Biol. (2009) 19:43–51. doi: 10.1016/j.tcb.2008.11.003
109. Datta A, Kim H, Lal M, McGee L, Johnson A, Moustafa AA, et al. Manumycin A suppresses exosome biogenesis and secretion via targeted inhibition of Ras/Raf/ERK1/2 signaling and hnRNP H1 in castration-resistant prostate cancer cells. Cancer Lett. (2017) 408:73–81. doi: 10.1016/j.canlet.2017.08.020
110. Menck K, Sonmezer C, Worst TS, Schulz M, Dihazi GH, Streit F, et al. Neutral sphingomyelinases control extracellular vesicles budding from the plasma membrane. J Extracell Vesicles. (2017) 6:1378056. doi: 10.1080/20013078.2017.1378056
111. Kulshreshtha A, Singh S, Ahmad M, Khanna K, Ahmad T, Agrawal A, et al. Simvastatin mediates inhibition of exosome synthesis, localization and secretion via multicomponent interventions. Sci Rep. (2019) 9:16373. doi: 10.1038/s41598-019-52765-7
112. Bianco NR, Kim SH, Ruffner MA, Robbins PD. Therapeutic effect of exosomes from indoleamine 2,3-dioxygenase-positive dendritic cells in collagen-induced arthritis and delayed-type hypersensitivity disease models. Arthritis Rheum. (2009) 60:380–9. doi: 10.1002/art.24229
113. Kim SH, Bianco N, Menon R, Lechman ER, Shufesky WJ, Morelli AE, et al. Exosomes derived from genetically modified DC expressing FasL are anti-inflammatory and immunosuppressive. Mol Ther. (2006) 13:289–300. doi: 10.1016/j.ymthe.2005.09.015
114. Kim SH, Lechman ER, Bianco N, Menon R, Keravala A, Nash J, et al. Exosomes derived from IL-10-treated dendritic cells can suppress inflammation and collagen-induced arthritis. J Immunol. (2005) 174:6440–8. doi: 10.4049/jimmunol.174.10.6440
115. Veerman RE, Gucluler Akpinar G, Eldh M, Gabrielsson S. Immune cell-derived extracellular vesicles - functions and therapeutic applications. Trends Mol Med. (2019) 25:382–94. doi: 10.1016/j.molmed.2019.02.003
116. Yin W, Ouyang S, Li Y, Xiao B, Yang H. Immature dendritic cell-derived exosomes: a promise subcellular vaccine for autoimmunity. Inflammation. (2013) 36:232–40. doi: 10.1007/s10753-012-9539-1
117. Wang GJ, Liu Y, Qin A, Shah SV, Deng ZB, Xiang X, et al. Thymus exosomes-like particles induce regulatory T cells. J Immunol. (2008) 181:5242–8. doi: 10.4049/jimmunol.181.8.5242
118. Yang C, Robbins PD. Immunosuppressive exosomes: a new approach for treating arthritis. Int J Rheumatol. (2012) 2012:573528. doi: 10.1155/2012/573528
119. Catalano M, O'Driscoll L. Inhibiting extracellular vesicles formation and release: a review of EV inhibitors. J Extracell Vesicles. (2020) 9:1703244. doi: 10.1080/20013078.2019.1703244
120. Armstrong JP, Holme MN, Stevens MM. Re-engineering extracellular vesicles as smart nanoscale therapeutics. ACS Nano. (2017) 11:69–83. doi: 10.1021/acsnano.6b07607
121. Mizrak A, Bolukbasi MF, Ozdener GB, Brenner GJ, Madlener S, Erkan EP, et al. Genetically engineered microvesicles carrying suicide mRNA/protein inhibit schwannoma tumor growth. Mol Ther. (2013) 21:101–8. doi: 10.1038/mt.2012.161
122. Wang M, Altinoglu S, Takeda YS, Xu Q. Integrating protein engineering and bioorthogonal click conjugation for extracellular vesicle modulation and intracellular delivery. PLoS ONE. (2015) 10:e0141860. doi: 10.1371/journal.pone.0141860
123. Broughton SE, Hercus TR, Lopez AF, Parker MW. Cytokine receptor activation at the cell surface. Curr Opin Struct Biol. (2012) 22:350–9. doi: 10.1016/j.sbi.2012.03.015
124. Calzolari A, Raggi C, Deaglio S, Sposi NM, Stafsnes M, Fecchi K, et al. TfR2 localizes in lipid raft domains and is released in exosomes to activate signal transduction along the MAPK pathway. J Cell Sci. (2006) 119(Pt 21):4486–98. doi: 10.1242/jcs.03228
125. Dasgupta SK, Abdel-Monem H, Niravath P, Le A, Bellera RV, Langlois K, et al. Lactadherin and clearance of platelet-derived microvesicles. Blood. (2009) 113:1332–9. doi: 10.1182/blood-2008-07-167148
126. Huang H, Hao S, Li F, Ye Z, Yang J, Xiang J. CD4+ Th1 cells promote CD8+ Tc1 cell survival, memory response, tumor localization and therapy by targeted delivery of interleukin 2 via acquired pMHC I complexes. Immunology. (2007) 120:148–59. doi: 10.1111/j.1365-2567.2006.02452.x
127. Perez-Hernandez D, Gutierrez-Vazquez C, Jorge I, Lopez-Martin S, Ursa A, Sanchez-Madrid F, et al. The intracellular interactome of tetraspanin-enriched microdomains reveals their function as sorting machineries toward exosomes. J Biol Chem. (2013) 288:11649–61. doi: 10.1074/jbc.M112.445304
128. Saunderson SC, Dunn AC, Crocker PR, McLellan AD. CD169 mediates the capture of exosomes in spleen and lymph node. Blood. (2014) 123:208–16. doi: 10.1182/blood-2013-03-489732
129. Simons M, Raposo G. Exosomes–vesicular carriers for intercellular communication. Curr Opin Cell Biol. (2009) 21:575–81. doi: 10.1016/j.ceb.2009.03.007
130. Skokos D, Le Panse S, Villa I, Rousselle JC, Peronet R, David B, et al. Mast cell-dependent B and T lymphocyte activation is mediated by the secretion of immunologically active exosomes. J Immunol. (2001) 166:868–76. doi: 10.4049/jimmunol.166.2.868
Keywords: extracellular vesicles, biogenesis, secretion, trafficking, therapeutics, intercellular, communication
Citation: Barnes BJ and Somerville CC (2020) Modulating Cytokine Production via Select Packaging and Secretion From Extracellular Vesicles. Front. Immunol. 11:1040. doi: 10.3389/fimmu.2020.01040
Received: 06 March 2020; Accepted: 29 April 2020;
Published: 29 May 2020.
Edited by:
Erwan Mortier, INSERM U1232 Centre de Recherche en Cancérologie et Immunologie Nantes Angers (CRCINA), FranceReviewed by:
Alanna Sedgwick, University of Notre Dame, United StatesNadia Lampiasi, Institute of Biomedicine and Molecular Immunology Alberto Monroy (IBIM), Italy
Copyright © 2020 Barnes and Somerville. This is an open-access article distributed under the terms of the Creative Commons Attribution License (CC BY). The use, distribution or reproduction in other forums is permitted, provided the original author(s) and the copyright owner(s) are credited and that the original publication in this journal is cited, in accordance with accepted academic practice. No use, distribution or reproduction is permitted which does not comply with these terms.
*Correspondence: Betsy J. Barnes, YmJhcm5lczFAbm9ydGh3ZWxsLmVkdQ==