- 1Department of Microbiology, Immunology and Infectious Diseases, Cumming School of Medicine, University of Calgary, Calgary, AB, Canada
- 2Bachelor of Health Sciences, Cumming School of Medicine, University of Calgary, Calgary, AB, Canada
- 3Department of Production Animal Health, Faculty of Veterinary Medicine, University of Calgary, Calgary, AB, Canada
Host defense peptides, abundantly secreted by colonic epithelial cells and leukocytes, are proposed to be critical components of an innate immune response in the colon against enteropathogenic bacteria, including Shigella spp., Salmonella spp., Clostridium difficile, and attaching and effacing Escherichia coli and Citrobacter rodentium. These short cationic peptides are bactericidal against both Gram-positive and -negative enteric pathogens, but may also exert killing effects on intestinal luminal microbiota. Simultaneously, these peptides modulate numerous cellular responses crucial for gut defenses, including leukocyte chemotaxis and migration, wound healing, cytokine production, cell proliferation, and pathogen sensing. This review discusses recent advances in our understanding of expression, mechanisms of action and microbicidal and immunomodulatory functions of major colonic host defense peptides, namely cathelicidins, β-defensins, and members of the Regenerating islet-derived protein III (RegIII) and Resistin-like molecule (RELM) families. In a theoretical framework where these peptides work synergistically, aspects of pathogenesis of infectious colitis reviewed herein uncover roles of host defense peptides aimed to promote epithelial defenses and prevent pathogen colonization, mediated through a combination of direct antimicrobial function and fine-tuning of host immune response and inflammation. This interactive host defense peptide network may decode how the intestinal immune system functions to quickly clear infections, restore homeostasis and avoid damaging inflammation associated with pathogen persistence during infectious colitis. This information is of interest in development of host defense peptides (either alone or in combination with reduced doses of antibiotics) as antimicrobial and immunomodulatory therapeutics for controlling infectious colitis.
Innate Immunity in Infectious Colitis and the Presence of Host Defense Peptides
Infectious diarrhea causes inflammation of the gastrointestinal tract, clinically manifested by diarrhea, dehydration and, in severe cases, death. Infectious diarrhea is a major cause of morbidity and mortality worldwide, particularly in developing countries (1). Diarrhea is regarded as the 8th leading cause of death, with children (<5 year of age) being responsible for over a quarter of deaths (2). Of the >1 million diarrheal deaths attributed to infectious agents, bacterial pathogens were collectively responsible for ~57% (2). Likewise, of the >2 billion global cases of diarrhea due to foodborne illnesses in 2010, 32% were due to bacteria (3). In addition, bacterial diarrhea is a main cause of illness in travelers seeking medical care after returning from developing nations (4).
The main genera of bacteria that cause infectious colitis are Escherichia, Salmonella, Campylobacter, Vibrio, Listeria, and Shigella. Increased emergence of antibiotic resistant bacterial strains have limited our ability to treat important enteric pathogens including Escherichia and Salmonella, and raises the possibility of increased prevalence and mortality due to intestinal bacterial infections (5–7). Indeed, antibiotic resistance is predicted to be a major future public health problem, with antibiotic resistant bacteria expected to cause > 10 million deaths globally by 2050 (8). Development and commercialization of new antibiotics is minimal and there are predictions that without substantial changes, bacterial resistance will continue to increase. Therefore, understanding innate immune mechanisms that aid in pathogen clearance and resolution are critical to understanding pathophysiology of infectious colitis and developing novel antimicrobial and immunomodulatory therapeutics.
The gastrointestinal tract has metabolic functions of digestion and nutrient absorption and also provides a barrier against large numbers of commensal or pathogenic microbes in the lumen. The colon employs multiple innate mechanisms to prevent and clear bacterial infections. For example, MUC2 mucin glycoprotein secreted by goblet cells and host defense peptides (HDPs) secreted by intestinal epithelial cells into the luminal environment compose the mucus layer, an acellular first line of defense. Intestinal epithelial cells, held together by apical junctional complexes, form a second line of defense to prevent penetration of bacteria into the lamina propria. If pathogenic bacteria are able to penetrate mucus and epithelial barriers, underlying leukocytes can protect the host by initiating inflammatory responses to clear invading pathogens.
Among innate effectors in the colon, HDPs are short cationic peptides abundantly secreted into the lumen by leukocytes and the intestinal epithelium, with key functions in maintenance of gut homeostasis (Figure 1). In the colon, HDPs are mostly represented by cathelicidin, β-defensins, and members of the Regenerating islet-derived protein III (RegIII) and Resistin-like molecule (RELM) families. Known as broad-spectrum antimicrobials, their contribution to innate gut defenses is expected to extend beyond direct lytic effects on bacteria to include immune functions reported in vitro (e.g., recruitment of immune cells, wound healing, and cytokine production; Table 1) (9, 42, 43). These immunomodulatory functions have been mostly described for myeloid-derived immune cells (10, 44). A key question is the extent to which immunomodulatory effects of HDPs occur in the gut and their relevance in infectious diseases. There are indications that secreted colonic HDPs are not merely antimicrobial, but also contribute to orchestrated immune responses. Understanding these aspects of HDP function is necessary for identifying novel anti-inflammatory and anti-infective targets as alternatives to conventional antimicrobials. Herein, we review recent advances in our understanding of HDPs in gut innate immune defenses and their role in pathogenesis of infectious colitis.
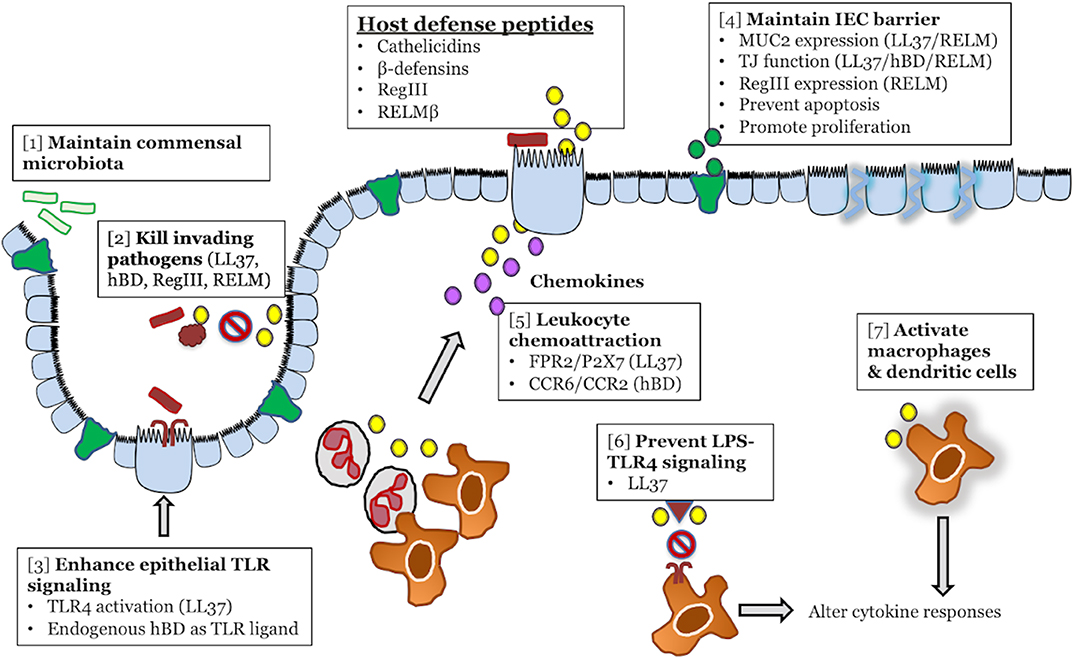
Figure 1. Colonic host defense peptides (HDPs) contribute to intestinal homeostasis and innate immune defense during infectious colitis through multiple overlapping mechanisms. HDPs secreted from intestinal epithelial cells (yellow) exert direct antibacterial effects on both the intestinal microbiota [1] and invading bacterial pathogens [2]. In terms of immunomodulatory functions, HDPs can enhance the immune signaling capacity of intestinal epithelial cells by forming complexes with LPS/TLR4 (LL-37), or by directly activating TLRs (β-defensins) [3]. HDPs might maintain intestinal epithelial cell barrier and prevention of pathogen invasion by increasing MUC2 secretion (green) and tight junction protein expression [4]. In the lamina propria, HPDs (yellow) secreted by epithelial cells or infiltrating leukocytes can directly chemoattract leukocytes from the blood (neutrophils, macrophages), through activation of FPR2, P2X7, CCR2, and CCR6, or promote the secretion of chemoattractant (CXCL8/IL-8) by epithelial cells (purple) [5]. In tissue resident macrophages, HDPs (yellow) can either promote anti-inflammatory responses by blocking LPS-TLR4 interaction [6] or activate macrophages and dendritic cells [7] to alter cytokine responses.
Cathelicidins
Cathelicidins are small, cationic, amphipathic peptides produced by epithelial cells, macrophages, and polymorphonuclear leukocytes (44, 45). These peptides are synthesized as pro-peptide precursors with a highly conserved N-terminal region (cathelin domain) and a highly variable antimicrobial cathelicidin peptide domain in their C-termini. Cleavage of the C-terminal domain from the holoprotein (e.g., by serine proteases) is required for antimicrobial activity. Humans have a single cathelicidin gene (cathelicidin antimicrobial peptide, CAMP), which yields a 37 amino acid peptide (leucine-leucine, LL-37) generated by extracellular cleavage of the C-terminus (46). The murine counterpart is cathelicidin-related-antimicrobial-peptide (CRAMP), encoded by the gene Camp (formerly Cnlp) (47).
In the colon, cathelicidins are mostly secreted by neutrophils and epithelial cells (42, 48). Differentiated colonic epithelial cells at the peak of crypts constitutively secrete cathelicidins (42, 48, 49), which are normally present in intestinal mucus of healthy individuals (50). Protective roles of cathelicidin in infectious colitis have been demonstrated in mice homozygous for null mutations in Camp (Camp−/−). These mice had exacerbated diarrhea, destructive colitis, and increased pathogen burden after challenge by chemical (43) or infectious (Clostridium difficile) (51) agents. Accordingly, Camp−/− mice were more susceptible to infection with attaching/effacing bacteria including C. rodentium (42) and E. coli O157:H7 (11). Consistent with these findings, upregulation of endogenous cathelicidin ameliorated colitis caused by enteropathogenic E. coli in rabbits (52).
Signaling pathways that regulate cathelicidin synthesis in the colon respond to both bacterial and endogenous stimuli. Regarding bacterial stimuli, colonic epithelium produced cathelicidins in response to bacterial by-products, such as short chain fatty acids (e.g., butyrate) (48, 53) via MEK-ERK signaling (49, 54). Bacterial DNA also stimulated cathelicidin in colonic lamina propria macrophages through TLR9 (43). This mechanism was observed in vivo when intracolonic exposure to E. coli genomic DNA upregulated cathelicidin expression in mice via TLR9 signaling (43). Similar to bacterial DNA, double-stranded RNA mimic poly(I:C) induced cathelicidin expression from intestinal epithelial cells via PI3-kinase-PKCζ-Sp1 signaling independent of MAPK pathways (55). MAPK signaling was also required for cathelicidin expression from colonic epithelial cells exposed to a combination of IL-1β and purified MUC2 (56).
Direct antibacterial activity was the first function identified for cathelicidins (57) with most studies focusing on the role of LL-37 against E. coli. Whereas, multiple antibacterial mechanisms may occur simultaneously, a principal bactericidal action of cathelicidins is membrane pore formation followed by direct bacterial death. LL-37 recognizes negatively charged lipids, a major component of Gram-negative bacterial membranes (58, 59). Binding of LL-37 to the bacterial surface leads to formation of transmembrane pores that induce bacterial cell lysis (60, 61). This pore formation depends on the alpha-helical amphipathic structure of LL-37, which shape its interactions with negatively-charged and hydrophobic targets on bacterial membranes. Because the structure of LL-37 is highly dependent on environmental factors (e.g., pH and anion concentration), the ability of cathelicidins to kill E. coli by transmembrane pore formation may be affected in physiological conditions (61).
Other antimicrobial mechanisms of cathelicidins include binding LPS to cross bacterial outer membranes into the periplasmic space, where LL-37 binds and immobilizes peptidoglycan to impede cell wall biogenesis and growth (62). Aditionally, there is a large influx of LL-37 into the bacterial cell after permeabilization of outer and cytoplasmic membranes that rigidifies the cytoplasm and halts motion of chromosomal DNA and ribosomes, thereby arresting E. coli growth (63). The polycationic nature of LL-37 allows it to form a network of electrostatic bonds with polyanionic DNA and ribosomes, preventing proper diffusion of cellular components (63). However, some of these antibacterial effects may be simply bacteriostatic and may not be effective at the population level. In high-density E. coli cultures exposed to LL-37, a sub-population of non-growing bacterial cells absorb massive amounts of LL-37 to deplete it from the surrounding environment, enabling a second sub-population to continue growing (64). Unlike LL-37, which interacts directly with microbial cell surfaces [e.g., E. histolytica (56)], other cathelicidins seem to internalize within bacterial cells and trigger non-lytic mechanisms. For example, porcine proline rich cathelicidin (PR-39), abundant in myeloid cells in pigs, crosses the cell membrane and likely kills pathogens by blocking bacterial DNA and peptide synthesis (65).
In an attempt to establish infection, intestinal pathogens may actively dampen cathelicidin defenses by multiple strategies. One strategy is to decrease cathelicidin expression in the colon during bacterial colonization. Cathelicidin production was decreased in colonic epithelium and leukocytes of shigellosis patients during early infection, where both live Shigella and bacterial plasmids blocked transcription of cathelicidin mRNA (66). Cathelicidin was also transcriptionally suppressed in colonic epithelial cells by exotoxins of Vibrio cholera and E. coli (cholera toxin and labile toxin, respectively) (67). Thus, cathelicidin silencing is likely a key virulence mechanism used by bacterial pathogens to facilitate intestinal colonization. Another evasion strategy is to repel direct killing by cathelicidins. While cathelicidins displayed in vitro killing activity against multiple strains of E. coli (11, 68), Salmonella enterica serovar Typhimurium resisted killing by cathelicidin through modulation of its outer membrane properties (69). Of note, despite these findings, the real relevance of cathelicidin antimicrobial activity in the gut is still controversial. Cathelicidins showed broad in vitro antibacterial activity (either bactericidal or bacteriostatic) against both Gram-positive and -negative bacteria (12, 70–73) (Table 2). However, this antibacterial activity is often abolished under physiological conditions, including presence of high salt concentrations (68, 85), serum (86), plasma alipoprotein-A1 (87), and sugars (88). Antibacterial activity of certain cathelicidins [synthetic cathelicidin (C18G), protegrin, magainin-like peptide] could be further inhibited by bacterial surface modifications, e.g., lipid A acylation by Salmonella spp. (89). Therefore, it is still questionable if cathelicidins undertake extensive antimicrobial activities in the colonic lumen. It is possible that microbicidal activities are restricted to certain conditions or niches (e.g., deeper in intestinal crypts, within the inner mucus layer) where cathelicidins can reach high concentrations and/or overcome inhibitory physiological effects.
On the other hand, there is growing evidence that immunomodulation is a critical function of cathelicidins in gut homeostasis. Such immunomodulation can be achieved by signaling through both colonic epithelial cells and immune cells, often at concentrations lower than is required for antimicrobial activity (Figure 1, Table 1). A first role of cathelicidins in gut innate immuninty could be enhancement of Toll-like receptor (TLR) sensing and prevention of pathogen invasion into colonic epithelial cells. For instance, human adenocarcinoma colonic epithelial (HT-29) cells exposed to a combination of synthetic LL-37 and LPS had increased TLR4 gene and protein expression (13). Such TLR4 activation is expected to increase production of pro-inflammatory cytokines, since LL-37 was required for CXCL8 and IL-1β production from colonocytes exposed to bacterial stimuli (13, 14). Moreover, the combination of cathelicidin and LPS prevented invasion of Salmonella enterica serovar Typhimurium into HT-29 cells (14). Although the synergistic effect between cathelicidins and LPS has not be tested in vivo, HT-29 cells served to examine colonic epithelial cell responses, as they constitutively express TLR4 and secrete CXCL8 in response to LPS as do primary intestinal epithelial cells (90). In support of this presumptive role in the colonic mucosa, LL-37 primed inflammatory responses in airway epithelial cells during Pseudomonas aeruginosa infection, promoting IL-1β and IL-18 secretion in an NLRP3 and caspase-1 dependent fashion (91).
Other immunomodulatory roles of cathelicidins in the gut include regulation of the intestinal epithelial barrier. This is important because the epithelial barrier plays a critical role in colonic histopathological changes and diarrhea that characterize infectious colitis. The epithelial paracellular barrier is largely maintained by tight junctions (TJs), and is critical for water absorption and restricting invasion of enteric luminal bacteria. TJs are disrupted in the colon exposed to enteric pathogens (92), but cathelicidins induced TJ gene expression in mammalian enterocytes and porcine intestines (9, 93). In addition, LL-37 prevented disruption of the TJ protein ZO-1 during S. enterica serovar Typhimurium infection in colonic epithelial (T84) cells (14). Effects of cathelicidins are not restricted to gut epithelium, as LL-37 induces upregulation of tight junction proteins and increases epithelial barrier function in keratinocytes (94). Although significance of cathelicidins in maintaining the gut barrier is incompletely understood, these functions might contribute to the increased pathogen burden and histopathological damage in Camp−/− mice infected with C. rodentium (42).
The colonic epithelial barrier is also maintained by the mucus layer, mainly composed of MUC2 mucin derived from intestinal goblet cells (56). MUC2 mucin limited in vitro colonization by pathogenic E. coli (95) and mechanically expelled pathogens from the gut to prevent C. rodentium propagation (96). The colonic mucus barrier is comprised of a firmly attached inner layer devoid of bacteria and a more loosely attached outer layer which contains large numbers of commensal bacteria (97). Camp−/− mice had a thinner colonic mucus layer and were more easily penetrated by E. coli O157:H7 (11), demonstrating the importance of cathelicidin for forming an effective mucus barrier. Moreover, stimulation of HT-29 cells with LL-37 induced gene expression of mucins MUC1 and MUC2 via the P2X purinoceptor 7 (P2X7) and MAP kinase pathway (9, 15). This function has been demonstrated in other epithelia, where stimulation of airway epithelial (NCI-H292) cells with LL-37 resulted in MUC5AC production through EGFR activation (98). Thus, it has been postulated that cathelicidins and mucin coexist as first line defenders in the intestinal lumen. Moreover, the more compact inner mucus layer could retain cationic peptides due to its overall negative charge and provides a gradient of antimicrobial HDPs that separates commensal microbiota from the epithelium (50). Indeed, cathelicidins are implicated in maintaining the colonic microbiota. Camp−/− mice display a different colonic microbiota in comparison to Camp+/+ mice, mostly associated with increased populations of Odoribacter lanues, Desulfovibrio piger, and Desulfomicrobium orale in Camp−/− mice (99).
It is known cathelicidins can act as direct chemoattractants to promote leukocyte influx to the site of infection; a role that could be critical in infectious colitis (100, 101). In leukocytes, cathelicidins signal through a range of receptors, including P2X7 and Formyl Peptide Receptor 2 (FPR2) that recognize extracellular ATP and N-formyl peptides, respectively. Accordingly, LL-37 inhibited neutrophil apoptosis by signaling through P2X7 and PI3K pathway (102), and chemoattracted FPR2-expressing peripheral blood monocytes, neutrophils and CD4+ T cells (16). The inhibition of apoptosis in neutrophils was abrogated by blocking P2X7 and the PI3K pathway (102), while the chemoattractant function of cathelicidin was inhibited by both a specific FPR2 inhibitor and the G-protein coupled receptor inhibitor pertussis toxin (16). Moreover, LL-37 directly activated CD11b/CD18 to increase monocyte migration (103) and phagocytosis of LL-37 coated bacteria (104), indicating a role of cathelicidin in leukocyte phagocytosis and migration through CD11b/CD18. Indirect activation of CD11b/CD18 by LL-37 and CRAMP on monocytes can also occur through activation of FPR2 (105). Cathelicidins can promote additional antimicrobial functions in neutrophils, such as induction of neutrophil extracellular trap (NET) formation. Stimulation of human neutrophils with both LL-37 and CRAMP resulted in an increase in NET formation (17, 18). Further research is needed to define the importance of these chemoattractant and pro-phagoctytic effects of cathelicidins in gut physiology and defenses in infectious colitis.
One intriguing aspect of cathelicidins is their pleiotropic nature, exerting either pro-inflammatory effects or attenuating inflammation depending on the environment. Cathelicidins inhibit LPS-induced pro-inflammatory responses in leukocytes. LL-37 inhibited the LPS-induced secretion of TNF-α from phagocytic THP-1 cells (10) by blocking binding of LPS to CD14 (19). This LPS neutralization was also important for preventing LPS-induced apoptosis in endothelial cells (106) and LPS/ATP-induced macrophage pyroptosis (20). Likewise, LL-37 lowered TNF-α, Cxcl-1, and IL-1β expression in both cultured murine macrophages and mammary epithelial cells exposed to the pathogenic algae Prototheca bovis (107). On the other hand, cathelicidins seem to promote inflammatory responses in intestinal epithelium (13, 14). Cathelicidin exhibited pro-inflammatory functions in intestinal epithelial cells exposed to LPS or S. enterica, including increased TLR4 expression, increased CXCL8 expression, and increased IL-1β (13, 14). It is likely that pro- or anti-inflammatory immunomodulatory function of cathelicidin is cell-type specific, and depends on the receptors expressed by either leukocytes or epithelial cells, infection status, the class of infecting pathogen, and the surrounding cytokine milieu.
The inter-species activity of cathelicidins is of interest for understanding the ontogeny of these ancenstral defenses and the development of therapeutics. Although all cathelicidins share a highly conserved N-terminal cathelin domain, the C-terminal antimicrobial domain is highly variable, both in sequence identity and secondary structure (45). For example, LL-37 and CRAMP share <70% sequence identity, however, they are still considered homologous. This is because both LL-37 and CRAMP share similar structure (α-helical and net charge of +6), antimicrobial capabilities (12, 108), and show interspecies functional capacity (109, 110). Both peptides comparably regulated chemokine expression and TLR 4 activity in myeloid cells (12, 21, 22) and neutrophil recruitment via FPR2 (16, 23). Moreover, mice infected with H1N1 influenza A virus had enhanced survival and reduced viral titer upon treatment with nebulized LL-37 (109). Likewise, intranasal administration of LL-37 increased inflammatory responses in sinonasal tissue of mice (110), while CRAMP was chemoattractant for human monocytes, neutrophils, and macrophages (23). However, an analysis of the interspecies functionality of 12 cathelicidins from 6 different species showed varying immunomodulatory activity results. While cathelicidins from all species demonstrated antimicrobial and LPS neutralizing function, there was large variability in the peptides' abilities to induce cytokine secretion from RAW264.7 macrophages (12). Thus, although they share common aspects, each cathelicidin should be studied as a unique peptide with specific activities in each host.
Given the antimicrobial and immunomodulatory characteristics of endogenous cathelicidins, the use of exogenous cathelicidin peptides (or their derivatives) as therapeutics for infectious colitis is appealing. Systemically administered exogenous cathelicidins were shown to attenuate colitis and reduce Salmonella burden in mice (111), while intracolonic CRAMP administration attenuated murine C. difficle colitis (51). Furthermore, intraperitoneal injection of porcine cathelicidin PR-39 in EHEC-infected mice improved survival, attenuated inflammatory cell infiltration and pro-inflammatory cytokine production (IL-1β, TNF-α, and IL-6) in the colon, and restored jejunal tight junction formation (112). Treatment of EHEC-infected mice with a cathelicidin derived from the snake Bungarus fascia (cathelicidin-WA) was similarly effective as the antibiotic enrofloxacin for increasing survival, reducing histopathological colonic damage and attenuating inflammatory colonic IL-6 production (113). Moreover, cathelicidin-WA was more effective than enrofloxacin for restoring jejunal mucus thickness and goblet cell number in EHEC-infected mice (93). A CRAMP-vancomycin conjugate demonstrated increased antibacterial activity against Gram-negative bacteria when compared to vancomycin or CRAMP alone, or to a 1:1 mixture of vancomycin and CRAMP (114). Synthetic HDPs derived from bovine cathelicidin peptide sequences with direct bactericidal and immunomodulatory functions (named immune defense regulator peptides, IDRs) have been developed for the treatment of diverse bacterial infections (115). IDR-HH2,−1002, and−1018 stimulated neutrophil functions including chemokine secretion, expression of adhesion molecules and release of antimicrobial HDPs, resulting in increased neutrophil killing of E. coli (116). IDR-1002 showed anti-inflammatory functions in a murine ear sterile inflammation model, decreasing IL-6, MCP-1, and KC production in PMA inflamed ears (117). Treatment of P. aeruginosa infected mice with IDR-1002 showed decreased bacterial burden and associated inflammation, including decreased IL-6 and MCP-1 in bronchoalveolar lavage fluid (118). Additionally, RAW 264.7 macrophages pre-treated with IDR-1002 and then stimulated with LPS showed reduced TNF-α and COX-2 expression (119). IDRs could also be combined with conventional antibiotics; IDR-1018 demonstrated anti-biofilm activity against P. aeruginosa and synergistic capabilities with antibiotics to kill biofilms of P. aeruginosa, E. coli, Acinetobacter baumannii, and S. enteria, among others (24). Thus, cathelicidins show promise as a potential future therapeutic against infectious colitis to reduce or replace antibiotics.
β-defensins
β-defensins are small cationic HDPs characterized by their cysteine-rich nature and disulphide bridges. There are at least 48 human β-defensin (hBD) genes (120), including hBD-1, -2, -3, and -4 that are highly expressed in the colon (25, 121). In mice, murine β-defensin (mBD)-1, -4, and -14 have been proposed as orthologous to hBD-1, -2, and -3, respectively (120). In terms of gut regulation, hBD-1 is constitutively expressed in colonic epithelium but does not appear to be upregulated by inflammatory signals (26), whereas hBD-2, -3, and -4 are minimally expressed in healthy colonic epithelium but are induced during inflammation (27–29).
Specific pro-inflammatory cytokines regulate colonic β-defensins. For example, IL-1α/β, and TNF-α enhanced expression of hBD-2 in intestinal epithelial cells without affecting hBD-1 expression (26). Such β-defensin induction occurred mostly through NF-κB (26). Likewise, activation of TLR2 and TLR4 directly activated hBD-2 expression in colonic epithelial cells through NF-κB and AP-1 (30), as well as activation of Nucleotide-binding Oligomerization Domain-like Receptor 2 (NOD2) (31). NF-κB-independent mechanisms have also been involved in β-defensin synthesis. Corticosteroids increased β-defensin expression independent of NF-κB in intestinal epithelial (Caco-2) cells (32). Additionally, hBD-3 was upregulated independently of NF-κB in human colonic epithelial cells exposed to extracts from medicinal plants (andrographolide, oridonin, and isoliquiritigen) (122). This upregulation of β-defensin increased bactericidal activity against Listeria monocytogenes and, bacteriostatic activity against S. enterica in supernatants from human colonic epithelial cells (122). The colonic mucus layer is also important in hBD-2 regulation. The major colonic secretory mucin, MUC2, upregulated hBD-2 in HT-29 cells in response to IL-1β (75). Moreover, mice genetically deficient in Muc2 (Muc2−/−) had decreased expression of mBD-4 and mBD-14 (75). Interestingly, fully glycosylated mature MUC2 reduced antibacterial activity of hBD-2 against pathogenic (EPEC) and commensal E. coli, indicating mucin may protect enteric bacteria from killing by β-defensins (75). These results are particularly important for ulcerative colitis patients, who commonly have diminished or disrupted intestinal mucus layers, suffer from dysbiosis, and are more prone to Clostridium difficile infection (123, 124).
Direct antibacterial functions of β-defensins are attributed to a disruption of microbial membranes by pore formation, causing release of intracellular contents and death (125). β-defensin homologs have a broad range of antibacterial activity (Table 2). For instance, hBD-2 has bactericidal activity against Gram-negative bacteria (i.e., E. coli, P. aeruginosa, Helicobacter pylori) and fungicidal activity against yeast (Candida albicans), but is merely bacteriostatic against the Gram-positive bacterium Staphylococcus aureus (76, 77). Conversely, hBD-3 is directly bactericidal against S. aureus and vancomycin-resistant Enterococcus faecium (VRE) (78). hBD-4 has bactericidal activity against both Gram-negative E. coli and P. aeruginosa in addition to Gram-positive S. aureus (79). Interestingly, reduction of disulphide-bridges in hBD-1 increases its bactericidal activity against C. albicans and Gram-positive commensal bacteria (29). Noteworthy, hBD-1 may have additional antibacterial functions beyond direct bacterial lysis; hBD-1 forms an entrapping net that abolished bacterial translocation across polycarbonate membranes and would prevent bacterial invasion (74).
β-defensins have chemoattractant function, although these roles in colitis are poorly defined. Both human (hBD-1, -2, and -3) and murine (mBD-4 and -14) β-defensins induce chemotaxis in leukocytes in a CCR6 dependent fashion (126–129). Moreover, hBD-2 and -3, and the orthologous mBD-4 and -14 induce migration of monocytes, macrophages and neutrophils through interactions with CCR2 (25). β-defensin immunomodulatory function also includes maturation and activation of leukocytes. mBD-2 activates immature dendritic cells, functioning as a TLR4 ligand and upregulating co-stimulatory molecules toward to a TH1 polarized response (130). In addition, hBD-3 activates monocytes in a TLR1/2 dependent fashion (131), whereas hBD-2 and -3 increase pro-inflammatory cytokine release from TLR-stimulated macrophages by ATP release and P2X7 activation (132). Interestingly, hBD-1, -2, and -3 all stimulate cytokine release from human peripheral blood mononuclear cells, with each β-defensin stimulating a unique array of cytokines (133). The presence of hBD-1 in human plasma (134), and expression of hBD-1 and -2 by human monocytes indicates systemic functions of β-defensins (135).
At the gut mucosa, β-defensins regulate epithelial cell responses including proliferation and migration, which are critical for resolution of injury, infection, and inflammation. hBD-2 signals through CCR6 on colonic epithelial (Caco-2 and T84) cells to induce actin cytoskeleton rearrangements and promote cell migration (136). Likewise, hBD-2 increased cell migration, induced MUC2 transcription and protected against apoptosis in Caco-2 and HT-29 cells (137). Studies in other epithelia infer hBDs may additionally have a role in regulation of intestinal epithelial permeability. hBD-3 improved keratinocyte barrier function through upregulation of tight junction proteins (138). Overall, protective mechanisms of β-defensins during intestinal infection include direct bacterial killing and regulatory functions on immune and intestinal epithelial cells.
Regenerating Islet-Derived Protein (Reg) III
The Regenerating islet-derived protein III (RegIII) proteins are C-type lectins, ~16–17 kD (139), with the capacity of binding bacterial carbohydrate motifs, independent of calcium, to mediate pore formation in bacterial membranes (140). The Reg gene family was originally identified from the Reg gene expressed in rat pancreatic regenerating islets (141). A large Reg gene family was later characterized and separated into 4 subgroups (I-IV), based on DNA and protein sequence similarity (142, 143). In humans, the Reg family consists of 5 genes [REGIα, REGIβ, Hepatocarcinomal-Intestine-Pancreas/Pancreatitis-Associated Protein (HIP/PAP), REGIIIγ, and REGIV], whereas 7 Reg genes are present in mice (RegI, RegII, RegIIIα, RegIIIβ, RegIIIγ, RegIIIδ, and RegIV) (144). In intestines, RegIII genes are the most prevalent Reg, with higher expression in the small intestine (83, 145, 146). Mice express 4 RegIII family members (RegIIIα, -β, -γ, and -δ), whereas humans express 2 RegIII genes (HIP/PAP (REGIIIα) and REGIIIγ) with certain homologies (147, 148). Human HIP/PAP and murine RegIIIγ are orthologous and share 67% homology, whereas human REGIIIγ shares 68% homology with murine RegIIIβ (149).
RegIII gene expression is increased during intestinal inflammation, as observed in IBD patients and mice afflicted with DSS-induced colitis (HIP/PAP and RegIIIγ) (145). RegIII expression in non-hematopoietic cells is mainly induced by activation of pattern recognition receptors and MyD88 signaling. Such innate upregulation of RegIIIγ in intestinal epithelium conferred protection against L. monocytogenes infection (150). Likewise, oral LPS upregulated RegIIIγ through TLR4 in antibiotic-treated mice, providing increased resistance to vancomycin-resistant Enterococcus (VRE) (151). Specialized intracellular nucleotide-binding oligomerization domain-like receptors (NOD-like receptors) also regulate RegIII. Mice deficient in Nod (Nod1−/−/Nod2−/−) had decreased expression of colonic RegIIIγ, associated with increased susceptibility to DSS-induced colitis (152).
Expression of RegIIIγ and RegIIIβ in colonic epithelial cells can also be regulated by IL-22 via STAT3 (153, 154). Indeed, mice genetically deficient in STAT3 have delayed wound healing during DSS-colitis, associated with decreased RegIIIγ and RegIIIβ expression in intestinal epithelial cells (155). Likewise, enteric infections could regulate RegIII via IL-22. RegIIIγ is upregulated during C. rodentium infection in response to IL-22 (154), whereas the TLR5 ligand flagellin increased expression of RegIIIγ in intestinal epithelial cells through IL-22 induction and protected against VRE infections (156). A main source of IL-22 is Th17 cells that regulate RegIIIγ in human and murine colonic epithelial cells (154, 157). Therefore, upregulation of intestinal RegIII can be mediated through cytokines, such as IL-22, or potentially through recognition of pathogen associated molecular patterns, e.g., LPS or flagellin. Collectively, these functions could work to drive pathogen clearance and restore homeostasis.
Antimicrobial functions of RegIII in colonic defense were recently demonstrated (Table 2). In vitro, HIP/PAP kills Gram-positive bacteria (L. monocytogenes and E. faecalis) through formation of an oligomeric pore in the bacterial membrane, although HIP/PAP failed to kill Gram-negative bacteria (83, 84). Unlike other C-type lectins, HIP/PAP binds to bacterial peptidoglycan in a calcium-independent fashion, and bacterial killing requires a conserved EPN motif (140). Accordingly, RegIIIγ−/− mice have increased abundance of Gram-positive mucosa-associated commensal bacteria (158). Interestingly, whereas RegIIIγ disrupts Gram-positive bacterial membranes, RegIIIβ preferentially kills Gram-negative bacteria (81, 82, 159). The ability of RegIIIβ to specifically target Gram-negative bacteria is due to its ability to bind to carbohydrate motifs of lipid A, a main component of LPS (159). The antibacterial function of RegIIIγ and RegIIIβ to preferentially target Gram-positive and Gram-negative bacteria, respectively, offers an interesting mechanism to for these two related HDPs to jointly protect against different types of bacterial pathogens. The antibacterial activity of RegIIIγ and HIP/PAP is activated through proteolytic removal of an N-terminal pro-segment by trypsin; the protein is inactive until it is secreted into the intestinal lumen and proteolytically processed to generate an active peptide (160). This fine control of RegIIIγ activity is similar to other regulatory mechanisms described for small intestinal α-defensins that require activation by the metalloproteinase matrilysin or trypsin (161, 162).
Roles of RegIII proteins in gut homeostasis may extend beyond direct bactericidal functions (Table 1). In the skin of psoriasis patients, HIP/PAP is highly expressed via IL-17 and promotes keratinocyte proliferation through engagement of exostosin-like 3 (EXTL3) and activation of the PI3K-AKT signaling pathway (33). Some of these immunomodulatory roles for RegIII proteins could apply to the gut, including preventing apoptosis. Treatment of HT-29 cells with recombinant HIP/PAP protected against apoptosis (34). Moreover, IL-22 protected mice from intestinal stem cell apoptosis during graft-vs.-host disease (GVDH) through upregulation of RegIIIγ (34).
Intestinal microbiota is an important regulator of RegIII. RegIIIγ genes were upregulated in the colon of germ-free mice upon bacterial colonization (83), and specific commensal bacteria induce RegIIIγ genes in the colon. Monocolonization of germ-free mice with Gram-positive Bifidobacterium breve, but not Gram-negative E. coli JM83, increased RegIIIγ production (149). Similarly, specific pathogen free (SPF) Nod1−/−/Nod2−/− mice showed decreased RegIIIγ expression, which was restored with altered Schaedler flora (ASF) (in gnotobiotic Nod1−/−/Nod2−/− mice) or B. breve (in SPF Nod1−/−/Nod2−/− mice) (152). In addition, monocolonization of germ-free SCID mice with segmented filamentous bacteria (SFB), strong inducers of Th17 cells and mucosal IL-22, increased RegIIIγ expression in intestinal epithelial cells (163). Likewise, monocolonization of germ-free mice with Gram-negative Bacteroides thetaiotaomicron resulted in a modest upregulation of RegIIIγ (2.5-fold), whereas monocolonization with Gram-positive Listeria innocua had no effect on RegIIIγ expression (83). Interestingly, monocolonization of Rag-1−/− germ-free mice with B. thetaiotaomicron and L. innocua resulted in a large (40 to 50-fold) increase in RegIIIγ expression, respectively (83). This phenomenon is hypothesized to be due to the absence of luminal IgA, which normally sequesters commensal bacteria away from intestinal epithelial cells, suggesting contact between bacteria and intestinal epithelial cells is a major driver of RegIIIγ expression (83).
It has been proposed that RegIIIγ antimicrobial activity is critical to separate commensal bacteria from underlying intestinal epithelial cells (158), or modulate the intestinal microbiota population when stimulated by intestinal infection or inflammation. In fact, RegIII-mediated alteration of the intestinal microbiota can have implications on outcomes of infectious colitis. RegIIIβ expression resulted in prolonged and worsened disease in a streptomycin murine model of Salmonella-induced colitis, corresponding with decreased presence and re-establishment of commensal Bacteroides spp. (81). Similarly, the regulation of RegIII by the microbiota has implication on infections, as depletion of the intestinal microbiota by antibiotic treatment decreased intestinal RegIIIγ expression and increased VRE burden (151). Production of RegIIIγ varies across the gut, with lower RegIIIγ expression in colon compared to small intestine (146); this difference may account for differential microbiome-host interactions. Whereas, RegIIIγ−/− mice did not display increased commensal bacteria/epithelial cell contact in the colon, they had more intimate bacterial contact in the terminal ileum associated with increased production of inflammatory IL-22 and myeloperoxidase (164). The antibacterial function of RegIIIγ is thought to be of particular importance due to its presence within the mucus layer, preventing penetration of both commensal and pathogenic bacteria. The mucus layer contains RegIIIγ (164) and its larger size compared with other HDPs (e.g., defensins or cathelicidins) could favor closer interactions with mucus glycoproteins thereby preventing RegIIIγ diffusion from the mucus layer into the lumen (158). Thus, RegIII may contribute to gut homeostasis via direct antibacterial functions against intestinal bacterial pathogens, microbiome regulation and immunomodulation (Figure 1).
Resistin-Like Molecules (RELM)
Resistin-like molecules (RELMs) are a family of secreted proteins characterized by a conserved cysteine-rich C-terminus (165). Previously named Found in Inflammatory Zone (FIZZ) and Hypoxia-Induced Mitogenic Factor (HIMF) proteins (166), the RELM protein family has been studied in a wide range of diseases, including asthma, diabetes, and bacterial and parasitic infections. RELM proteins range in size from 105 to 114 amino acids, with 3 conserved domains: a signal sequence, a variable middle domain, and a C-terminal domain (165). The latter is particularly rich in cysteine residues, invariantly spaced following the sequence C-X11-C-X8-C-X-C-X3-C-X10-C-X-C-X-C-X9-CC-X3−6 (165). Mice have 4 Relm proteins (RELMα, -β, -γ, and resistin) encoded by Retlna, Retlnb, Retlng, and Retn genes, respectively, whereas humans possess only RELMβ and Resistin, encoded by RETLNB and RETN genes (165, 167). Murine RELMα is mostly restricted to airway epithelial cells (168) and immune cells (i.e., macrophages and dendritic cells) (169, 170), while murine RELMγ is expressed in bone marrow, spleen, and lungs (171). Human Resistin is expressed in immune cells (172), whereas murine Resistin is restricted to adipocytes (173). Resistin and RELMβ form large hexamers consisting of 2 trimers, linked by disulphide bonds present in their N-terminal coiled-coiled domains (174), although RELMβ may also exist as secreted dimers (175).
RELMβ is the most abundantly expressed RELM in the large intestine of both humans and mice (176), mostly in cecum and distal colon (176). The peptide is produced as a 111-amino acid protein with an N-terminal 11-amino acid signal sequence, and is predominantly restricted to secretory granules of mucus-secreting goblet cells (176). Unsurprisingly, RELMβ expression is regulated by factors that influence goblet cell functions, e.g., IL-18 and IL-22 (177). Expression of RELMβ is dependent on the microbiota, being transcriptionally induced in germ-free mice upon colonization with a conventional microbiota (176). Moreover, oral antibiotic treatment of mice decreased Firmicutes, with persistence of Bacteroidetes and Proteobateria, and decreased RELMβ production, along with decreased IFNγ and IL-17 production from CD4+ T-cells (178).
RELMβ is particularly reactive to helminth intestinal infections, including Trichinella muris, Trichuris spiralis, and Nippostrongylus brasiliensis as a part of a TH2-driven immune response, mainly mediated through IL-4 and IL-13 (179). Accordingly, RELMβ-deficient mice were more susceptible to Heligmosomoides polygyrus and Nippostrongylus brasiliensis infections (180). Detection of RELMβ in feces of mice with gastrointestinal nematode infections has also been demonstrated as a non-invasive tool to assess intestinal changes in response to intestinal infections (181). Mechanistically, RELMβ is necessary for IL-4-mediated intestinal worm expulsion, impairing the ability of worms to feed on host tissues and generate ATP (180). In addition, RELMβ can directly bind chemosensory components of parasitic nematodes to block their sensory functions (179).
Colonic RELMβ expression is increased during bacterial infection (e.g., C. rodentium), with secreted RELMβ present in feces (35). Indeed, RELMβ deficient mice are more susceptible to C. rodentium infection, with decreased survival and increased bacterial colonization deep within colonic crypts (35). Direct microbial killing may be a key role of RELMβ in this gut defense. RELMβ causes pore formation and bacterial death in both Gram-positive and Gram-negative bacteria, including L. monocytogenes, E. faecails, C. rodentium, and P. aeruginosa (80) (Table 2). Although it is broad-spectrum bactericidal, RELMβ preferentially kills Gram-negative bacteria, with antibacterial functions observed for both monomeric and dimeric forms of the protein (80). Additionally, colonic RELMβ may protect against C. rodentium indirectly, by promoting intestinal epithelial cell (IEC) proliferation and chemoattracting T-cells. RELMβ−/− mice infected with C. rodentium had increased mortality, with reduced CD4+ T-cell infiltration, reduced IL-22 production, and impaired IEC proliferation in colons (35). Interestingly, RELMβ−/− mice displayed decreased expression of RegIII C-type lectins (36, 37). Thus, decreased CD4+ T-cell infiltration and IL-22 production in the colons of RELMβ−/− mice may be major drivers of decreased RegIIIγ gene expression, as IL-22 is a strong inducer of RegIIIγ production (153, 154).
RELMβ expression is induced in mice during DSS colitis, with increased expression requiring IL-13, as IL-13−/− mice were unable to induce RELMβ (36). Some pro-inflammatory roles for RELMβ are postulated based on decreased colitis in RELMβ−/− mice exposed to DSS (e.g., decreased weight loss, colonic shortening, and mortality) (36). However, in the same study, RELMβ−/− mice were more susceptible to TNBS-induced colitis (36). These apparent conflicting results are likely due to the underlying inflammatory mechanisms behind these 2 models of colitis. While DSS colitis causes inflammation driven by direct erosion of the epithelial barrier, TNBS-induced colitis is mediated by potent TH1 immune responses (182). In addition, RELMβ−/− mice responses to DSS and TNBS-induced colitis could be regulated by RegIIIβ expression, which showed to reduce TNF-α induced immune responses in monocytes and epithelial cells (36, 183). Thus, RELMβ could undertake either pro- or anti-inflammatory roles depending on the inflammatory stimuli or surrounding immune activation.
RELMs may enhance gut mucosal barrier defenses against pathogenic bacteria by promoting colonic mucin. RELMβ upregulated MUC2 and increased secretion of MUC5AC in mucin-producing colonic epithelial (HT29-Cl.16E) cells, signaling through calcium and PKC (38). Moreover, mice pre-treated with synthetic RELMβ experienced increased mucus production and attenuated TNBS colitis (38). However, RELMβ could still exert pro-inflammatory roles beyond a mucin secretagogue effect. Mice genetically deficient in Muc2 (Muc2−/−) developed spontaneous colitis, an effect that was diminished in mice double knock out for Muc2 and RELMβ (Muc2−/−/RELMβ−/−) (37). Interestingly, RELMβ expression in Muc2−/− mice induced expression of both RegIIIγ and RegIIIβ, which corresponded to a decrease in Lactobacilli spp. (37). It is likely that spontaneous colitis in Muc2−/− mice responds to replenishment of Lactobacilli spp. and a pro inflammatory role of RELMβ affecting healthy intestinal microbiota. Furthermore, the presence of RELMβ in Muc2−/− mice could contribute to increased RegIII expression, resulting in microbial dysbiosis and more severe colitis in comparison to Muc2−/−/RELMβ−/− mice (37). Effects of RELMβ on gut permeability could also impact intestinal homeostasis. RELMβ−/− mice have decreased trans-epithelial electrical resistance (TEER) and increased permeability to (4-kDa) dextran in whole intestinal mounts (36). RELMβ stimulation of rat jejunal tissue promoted glucose transport mediated by alteration of glucose transporter proteins (diminished SGLT-1 and increased GLUT2 expression) and activation of PKC and AMPK signaling (184). Thus, the function of RELMβ in colitis is, at least in part, due to its ability to induce expression of RegIII proteins, modulate the intestinal microbiota, and influence epithelial permeability.
Other RELMs, including RELMα, usually restricted to immune cells (e.g., macrophages and dendritic cells) (169, 170), are expressed by epithelial cells, macrophages, and eosinophils during C. rodentium infection (185). However, RELMα could promote gut inflammation without microbicidal activities. RELMα−/− mice infected with C. rodentium did not show higher pathogen burden, but decreased colitis with decreased CD4+ T cell expression of pro-inflammatory IL-17A (185). Additionally, intraperitoneal injection of mice with recombinant RELMα increased C. rodentium-induced colitis, including increased IL-17, whereas IL-17A−/− mice did not display increased colitis in response to RELMα treatment (185). These data demonstrate a pro-inflammatory role of RELMα during C. rodentium infection, working mainly through the induction of IL-17. Similarly, RELMα−/− mice have decreased inflammation during DSS colitis (186, 187). Thus, influence of RELMs on intestinal inflammation and infection is complex and involves direct antimicrobial activity, regulation of intestinal RegIII C-type lectins, modulation of the microbiota, and potential direct immunomodulatory effects.
Conclusions
Aspects of major HDPs (i.e., cathelicidins, β-defensins, and members of RegIII and RELM families) in the colon and their relevance in pathogenesis of infectious colitis reviewed herein aid to uncover roles of these peptides in promoting epithelial defenses beyond direct microbial elimination (Figure 1). The presence of HDPs abundantly secreted into the intestinal lumen by epithelial cells and leukocytes during inflammation must be critical components of the innate immune response against enteropathogenic bacteria. They are bactericidal against enteric pathogens as well as the microbiota, while simultaneously modulating numerous cellular responses including leukocyte chemotaxis and migration, wound healing, cytokine production and pathogen sensing (Tables 1, 2). In fact, crude colonic mucus isolates from uninfected mice had no direct antibacterial activity against C. rodentium (96). Thus, there is increasing interest to decipher major mechanisms of HDPs in innate defense, which seem to be largely attributed to immunomodulatory functions.
To date, our understanding of HDP function in the colon is mostly limited to studies using mice genetically deficient in a single HDP, or via stimulation of mice with a specific exogenous peptide (commonly a synthetic peptide derived from an endogenous HDP). We propose a theoretical framework of how these peptides may work together in the gut. Host defense peptides would form an interactive peptide network capable of preventing colonization of enteropathogens by: (1) direct bacterial killing and (2) fine-tuning of the host immune response in the colon (i.e., modulation of epithelial cell responses and migration of leukocyte populations to the site of infection). In these activities, HPDs likely have overlapping and potentially complementary roles. Cathelicidins from different species displayed synergistic antibacterial activity against P. aeruginosa, E. coli, E. faecalis, and MRSA (188). Additionally, cathelicidins demonstrated synergistic antibacterial ability with human lysozyme (188). Thus, in the real scenario of numerous HDPs co-existing with other innate factors (e.g., MUC2 mucin, lysozyme), antibacterial activity against specific pathogenic species is likely enhanced.
The synergistic effects of multiple HDPs could apply to cytokine and chemokine signaling in the gut. Combined hBD-3 and LL-37 showed a synergistic reduction in secretion of proinflammatory factors (GRO-α, G-CSF, MCP-1, and IL-6) in gingival fibroblast-epithelial cells exposed to LPS, although they displayed only additive effects reducing IL-8 secretion (189). Other synergies can be predicted to occur during infectious colitis based on the effects of single HDPs. RELMβ regulates T-cell migration, IL-22 production and RegIIIγ in the colon (35–37), while cathelicidins, β-defensins, and RELMβ have all been demonstrated to regulate both mucus production and epithelial permeability (9, 11, 14, 15, 36, 38, 137, 138). This HDP network may decode how the intestinal innate immune system functions to quickly restore gut homeostasis and avoid damaging inflammation associated with pathogen colonization. Exploring how these peptides act synergistically in innate immune defenses and the complex signaling networks they activate during infectious colitis should lead to identification of therapeutic anti-infectious targets, or development of synthetic HDPs that work in combination to resolve intestinal infections. Such synthetic HDPs could be used either alone or in combination with reduced doses of antibiotics. These strategies of infectious disease control would be greatly beneficial, as emergence of antimicrobial resistance is rendering conventional antibiotics use unsustainable.
Author Contributions
All authors listed have made a substantial, direct and intellectual contribution to the work, and approved it for publication.
Funding
This work was supported by NSERC Discovery Grant (RGPAS-2017-507827) and Alberta Agriculture and Forestry (2018F050R and 2019F041R).
Conflict of Interest
The authors declare that the research was conducted in the absence of any commercial or financial relationships that could be construed as a potential conflict of interest.
Acknowledgments
Authors thank Dr. John Kastelic (Veterinary Medicine, University of Calgary) for editing the manuscript.
References
1. DuPont HL. Persistent diarrhea: a clinical review. JAMA. (2016) 315:2712–23. doi: 10.1001/jama.2016.7833
2. GBD 2016 Diarrhoeal Disease Collaborators. Estimates of the global, regional, and national morbidity, mortality, and aetiologies of diarrhoea in 195 countries: a systematic analysis for the global burden of disease Study 2016. Lancet Infect Dis. (2018) 18:1211–28. doi: 10.1016/S1473-3099(18)30362-1
3. Kirk MD, Pires SM, Black RE, Caipo M, Crump JA, Devleesschauwer B, et al. World Health Organization estimates of the global and regional disease burden of 22 foodborne bacterial, protozoal, and viral diseases, 2010: a data synthesis. PLoS Med. (2015) 12:e1001921. doi: 10.1371/journal.pmed.1001921
4. Freedman DO, Weld LH, Kozarsky PE, Fisk T, Robins R, von Sonnenburg F, et al. GeoSentinel surveillance network. spectrum of disease and relation to place of exposure among ill returned travelers. N Engl J Med. (2006) 354:119–30. doi: 10.1056/NEJMoa051331
5. Mir RA, Kudva IT. Antibiotic-resistant shiga toxin-producing Escherichia coli: an overview of prevalence and intervention strategies. Zoonoses Public Health. (2019) 66:1–13. doi: 10.1111/zph.12533
6. Gut AM, Vasiljevic T, Yeager T, Donkor ON. Salmonella infection - prevention and treatment by antibiotics and probiotic yeasts: a review. Microbiol Read Engl. (2018) 164:1327–44. doi: 10.1099/mic.0.000709
7. Ruppé E, Andremont A, Armand-Lefèvre L. Digestive tract colonization by multidrug-resistant Enterobacteriaceae in travellers: an update. Travel Med Infect Dis. (2018) 21:28–35. doi: 10.1016/j.tmaid.2017.11.007
8. Shallcross LJ, Howard SJ, Fowler T, Davies SC. Tackling the threat of antimicrobial resistance: from policy to sustainable action. Philos Trans R Soc Lond B Biol Sci. (2015) 370:20140082. doi: 10.1098/rstb.2014.0082
9. Otte J-M, Zdebik A-E, Brand S, Chromik AM, Strauss S, Schmitz F, et al. Effects of the cathelicidin LL-37 on intestinal epithelial barrier integrity. Regul Pept. (2009) 156:104–17. doi: 10.1016/j.regpep.2009.03.009
10. Mookherjee N, Brown KL, Bowdish DME, Doria S, Falsafi R, Hokamp K, et al. Modulation of the TLR-mediated inflammatory response by the endogenous human host defense peptide LL-37. J Immunol. (2006) 176:2455–64. doi: 10.4049/jimmunol.176.4.2455
11. Chromek M, Arvidsson I, Karpman D. The antimicrobial peptide cathelicidin protects mice from Escherichia coli O157:H7-mediated disease. PLoS ONE. (2012) 7:e46476. doi: 10.1371/journal.pone.0046476
12. Coorens M, Scheenstra MR, Veldhuizen EJA, Haagsman HP. Interspecies cathelicidin comparison reveals divergence in antimicrobial activity, TLR modulation, chemokine induction and regulation of phagocytosis. Sci Rep. (2017) 7:40874. doi: 10.1038/srep40874
13. Marin M, Holani R, Shah CB, Odeón A, Cobo ER. Cathelicidin modulates synthesis of Toll-like receptors (TLRs) 4 and 9 in colonic epithelium. Mol Immunol. (2017) 91:249–58. doi: 10.1016/j.molimm.2017.09.011
14. Marin M, Holani R, Blyth GAD, Drouin D, Odeón A, Cobo ER. Human cathelicidin improves colonic epithelial defenses against Salmonella typhimurium by modulating bacterial invasion, TLR4 and pro-inflammatory cytokines. Cell Tissue Res. (2019) 376:433–42. doi: 10.1007/s00441-018-02984-7
15. Tai EKK, Wong HPS, Lam EKY, Wu WKK, Yu L, Koo MWL, et al. Cathelicidin stimulates colonic mucus synthesis by up-regulating MUC1 and MUC2 expression through a mitogen-activated protein kinase pathway. J Cell Biochem. (2008) 104:251–8. doi: 10.1002/jcb.21615
16. De Yang, Chen Q, Schmidt AP, Anderson GM, Wang JM, Wooters J, et al. LL-37, the neutrophil granule- and epithelial cell-derived cathelicidin, utilizes formyl peptide receptor-like 1 (FPRL1) as a receptor to chemoattract human peripheral blood neutrophils, monocytes, and T cells. J Exp Med. (2000) 192:1069–74. doi: 10.1084/jem.192.7.1069
17. Neumann A, Berends ETM, Nerlich A, Molhoek EM, Gallo RL, Meerloo T, et al. The antimicrobial peptide LL-37 facilitates the formation of neutrophil extracellular traps. Biochem J. (2014) 464:3–11. doi: 10.1042/BJ20140778
18. Cao Y, Chen F, Sun Y, Hong H, Wen Y, Lai Y, et al. LL-37 promotes neutrophil extracellular trap formation in chronic rhinosinusitis with nasal polyps. Clin Exp Allergy J Br Soc Allergy Clin Immunol. (2019) 49:990–9. doi: 10.1111/cea.13408
19. Nagaoka I, Hirota S, Niyonsaba F, Hirata M, Adachi Y, Tamura H, et al. Cathelicidin family of antibacterial peptides CAP18 and CAP11 inhibit the expression of TNF-alpha by blocking the binding of LPS to CD14+ cells. J Immunol. (2001) 167:3329–38. doi: 10.4049/jimmunol.167.6.3329
20. Hu Z, Murakami T, Suzuki K, Tamura H, Kuwahara-Arai K, Iba T, et al. Antimicrobial cathelicidin peptide LL-37 inhibits the LPS/ATP-induced pyroptosis of macrophages by dual mechanism. PLoS ONE. (2014) 9:e85765. doi: 10.1371/journal.pone.0085765
21. Di Nardo A, Braff MH, Taylor KR, Na C, Granstein RD, McInturff JE, et al. Cathelicidin antimicrobial peptides block dendritic cell TLR4 activation and allergic contact sensitization. J Immunol. (2007) 178:1829–34. doi: 10.4049/jimmunol.178.3.1829
22. Kandler K, Shaykhiev R, Kleemann P, Klescz F, Lohoff M, Vogelmeier C, et al. The anti-microbial peptide LL-37 inhibits the activation of dendritic cells by TLR ligands. Int Immunol. (2006) 18:1729–36. doi: 10.1093/intimm/dxl107
23. Kurosaka K, Chen Q, Yarovinsky F, Oppenheim JJ, Yang D. Mouse cathelin-related antimicrobial peptide chemoattracts leukocytes using formyl peptide receptor-like 1/mouse formyl peptide receptor-like 2 as the receptor and acts as an immune adjuvant. J Immunol. (2005) 174:6257–65. doi: 10.4049/jimmunol.174.10.6257
24. Mansour SC, de la Fuente-Núñez C, Hancock REW. Peptide IDR-1018: modulating the immune system and targeting bacterial biofilms to treat antibiotic-resistant bacterial infections. J Pept Sci. (2015) 21:323–9. doi: 10.1002/psc.2708
25. Röhrl J, Yang D, Oppenheim JJ, Hehlgans T. Human beta-defensin 2 and 3 and their mouse orthologs induce chemotaxis through interaction with CCR2. J Immunol. (2010) 184:6688–94. doi: 10.4049/jimmunol.0903984
26. O'Neil DA, Porter EM, Elewaut D, Anderson GM, Eckmann L, Ganz T, et al. Expression and regulation of the human beta-defensins hBD-1 and hBD-2 in intestinal epithelium. J Immunol. (1999) 163:6718–24.
27. Wehkamp J, Harder J, Weichenthal M, Mueller O, Herrlinger KR, Fellermann K, et al. Inducible and constitutive beta-defensins are differentially expressed in Crohn's disease and ulcerative colitis. Inflamm Bowel Dis. (2003) 9:215–23. doi: 10.1097/00054725-200307000-00001
28. Fahlgren A, Hammarstrom S, Danielsson A, Hammarstrom M-L. beta-Defensin-3 and−4 in intestinal epithelial cells display increased mRNA expression in ulcerative colitis. Clin Exp Immunol. (2004) 137:379–85. doi: 10.1111/j.1365-2249.2004.02543.x
29. Schroeder BO, Wu Z, Nuding S, Groscurth S, Marcinowski M, Beisner J, et al. Reduction of disulphide bonds unmasks potent antimicrobial activity of human β-defensin 1. Nature. (2011) 469:419–23. doi: 10.1038/nature09674
30. Vora P, Youdim A, Thomas LS, Fukata M, Tesfay SY, Lukasek K, et al. Beta-defensin-2 expression is regulated by TLR signaling in intestinal epithelial cells. J Immunol. (2004) 173:5398–405. doi: 10.4049/jimmunol.173.9.5398
31. Voss E, Wehkamp J, Wehkamp K, Stange EF, Schröder JM, Harder J. NOD2/CARD15 mediates induction of the antimicrobial peptide human beta-defensin-2. J Biol Chem. (2006) 281:2005–11. doi: 10.1074/jbc.M511044200
32. Witthöft T, Pilz CS, Fellermann K, Nitschke M, Stange EF, Ludwig D. Enhanced human beta-defensin-2 (hBD-2) expression by corticosteroids is independent of NF-kappaB in colonic epithelial cells (CaCo2). Dig Dis Sci. (2005) 50:1252–9. doi: 10.1007/s10620-005-2768-5
33. Lai Y, Li D, Li C, Muehleisen B, Radek KA, Park HJ, et al. The antimicrobial protein REG3A regulates keratinocyte proliferation and differentiation after skin injury. Immunity. (2012) 37:74–84. doi: 10.1016/j.immuni.2012.04.010
34. Zhao D, Kim Y-H, Jeong S, Greenson JK, Chaudhry MS, Hoepting M, et al. Survival signal REG3α prevents crypt apoptosis to control acute gastrointestinal graft-versus-host disease. J Clin Invest. (2018) 128:4970–79. doi: 10.1172/JCI99261
35. Bergstrom KSB, Morampudi V, Chan JM, Bhinder G, Lau J, Yang H, et al. Goblet cell derived RELM-β recruits CD4+ T cells during infectious colitis to promote protective intestinal epithelial cell proliferation. PLoS Pathog. (2015) 11:e1005108. doi: 10.1371/journal.ppat.1005108
36. Hogan SP, Seidu L, Blanchard C, Groschwitz K, Mishra A, Karow ML, et al. Resistin-like molecule beta regulates innate colonic function: barrier integrity and inflammation susceptibility. J Allergy Clin Immunol. (2006) 118:257–68. doi: 10.1016/j.jaci.2006.04.039
37. Morampudi V, Dalwadi U, Bhinder G, Sham HP, Gill SK, Chan J, et al. The goblet cell-derived mediator RELM-β drives spontaneous colitis in Muc2-deficient mice by promoting commensal microbial dysbiosis. Mucosal Immunol. (2016) 9:1218–33. doi: 10.1038/mi.2015.140
38. Krimi RB, Kotelevets L, Dubuquoy L, Plaisancié P, Walker F, Lehy T, et al. Resistin-like molecule beta regulates intestinal mucous secretion and curtails TNBS-induced colitis in mice. Inflamm Bowel Dis. (2008) 14:931–41. doi: 10.1002/ibd.20420
39. Angelini DJ, Su Q, Yamaji-Kegan K, Fan C, Teng X, Hassoun PM, et al. Resistin-like molecule-beta in scleroderma-associated pulmonary hypertension. Am J Respir Cell Mol Biol. (2009) 41:553–61. doi: 10.1165/rcmb.2008-0271OC
40. Liu T, Baek HA, Yu H, Lee HJ, Park B-H, Ullenbruch M, et al. FIZZ2/RELM-β induction and role in pulmonary fibrosis. J Immunol. (2011) 187:450–61. doi: 10.4049/jimmunol.1000964
41. Fang CL, Yin LJ, Sharma S, Kierstein S, Wu HF, Eid G, et al. Resistin-like molecule-β (RELM-β) targets airways fibroblasts to effect remodelling in asthma: from mouse to man. Clin Exp Allergy J Br Soc Allergy Clin Immunol. (2015) 45:940–52. doi: 10.1111/cea.12481
42. Iimura M, Gallo RL, Hase K, Miyamoto Y, Eckmann L, Kagnoff MF. Cathelicidin mediates innate intestinal defense against colonization with epithelial adherent bacterial pathogens. J Immunol. (2005) 174:4901–7. doi: 10.4049/jimmunol.174.8.4901
43. Koon HW, Shih DQ, Chen J, Bakirtzi K, Hing TC, Law I, et al. Cathelicidin signaling via the Toll-like receptor protects against colitis in mice. Gastroenterology. (2011) 141:1852–63.e1–3. doi: 10.1053/j.gastro.2011.06.079
44. Hancock REW, Haney EF, Gill EE. The immunology of host defence peptides: beyond antimicrobial activity. Nat Rev Immunol. (2016) 16:321–34. doi: 10.1038/nri.2016.29
45. Kościuczuk EM, Lisowski P, Jarczak J, Strzałkowska N, Józwik A, Horbanczuk J, et al. Cathelicidins: family of antimicrobial peptides. A review. Mol Biol Rep. (2012) 39:10957–70. doi: 10.1007/s11033-012-1997-x
46. Reinholz M, Ruzicka T, Schauber J. Cathelicidin LL-37: an antimicrobial peptide with a role in inflammatory skin disease. Ann Dermatol. (2012) 24:126–35. doi: 10.5021/ad.2012.24.2.126
47. Nizet V, Ohtake T, Lauth X, Trowbridge J, Rudisill J, Dorschner RA, et al. Innate antimicrobial peptide protects the skin from invasive bacterial infection. Nature. (2001) 414:454–7. doi: 10.1038/35106587
48. Hase K, Eckmann L, Leopard JD, Varki N, Kagnoff MF. Cell differentiation is a key determinant of cathelicidin LL-37/human cationic antimicrobial protein 18 expression by human colon epithelium. Infect Immun. (2002) 70:953–63. doi: 10.1128/iai.70.2.953-963.2002
49. Schauber J, Svanholm C, Termén S, Iffland K, Menzel T, Scheppach W, et al. Expression of the cathelicidin LL-37 is modulated by short chain fatty acids in colonocytes: relevance of signalling pathways. Gut. (2003) 52:735–41. doi: 10.1136/gut.52.5.735
50. Antoni L, Nuding S, Weller D, Gersemann M, Ott G, Wehkamp J, et al. Human colonic mucus is a reservoir for antimicrobial peptides. J Crohns Colitis. (2013) 7:e652–64. doi: 10.1016/j.crohns.2013.05.006
51. Hing TC, Ho S, Shih DQ, Ichikawa R, Cheng M, Chen J, et al. The antimicrobial peptide cathelicidin modulates Clostridium difficile-associated colitis and toxin A-mediated enteritis in mice. Gut. (2013) 62:1295–305. doi: 10.1136/gutjnl-2012-302180
52. Al-Mamun A, Mily A, Sarker P, Tiash S, Navarro A, Akter M, et al. Treatment with phenylbutyrate in a pre-clinical trial reduces diarrhea due to enteropathogenic Escherichia coli: link to cathelicidin induction. Microbes Infect. (2013) 15:939–50. doi: 10.1016/j.micinf.2013.08.007
53. Zanetti M. Cathelicidins, multifunctional peptides of the innate immunity. J Leukoc Biol. (2004) 75:39–48. doi: 10.1189/jlb.0403147
54. Schauber J, Iffland K, Frisch S, Kudlich T, Schmausser B, Eck M, et al. Histone-deacetylase inhibitors induce the cathelicidin LL-37 in gastrointestinal cells. Mol Immunol. (2004) 41:847–54. doi: 10.1016/j.molimm.2004.05.005
55. Ta A, Thakur BK, Dutta P, Sinha R, Koley H, Das S. Double-stranded RNA induces cathelicidin expression in the intestinal epithelial cells through phosphatidylinositol 3-kinase-protein kinase Cζ-Sp1 pathway and ameliorates shigellosis in mice. Cell Signal. (2017) 35:140–53. doi: 10.1016/j.cellsig.2017.03.016
56. Cobo ER, Kissoon-Singh V, Moreau F, Holani R, Chadee K. MUC2 mucin and butyrate contribute to the synthesis of the antimicrobial peptide cathelicidin in response to Entamoeba histolytica- and dextran sodium sulfate-induced colitis. Infect Immun. (2017) 85:e00905–16. doi: 10.1128/IAI.00905-16
57. Larrick JW, Hirata M, Shimomoura Y, Yoshida M, Zheng H, Zhong J, et al. Antimicrobial activity of rabbit CAP18-derived peptides. Antimicrob Agents Chemother. (1993) 37:2534–9. doi: 10.1128/aac.37.12.2534
58. Oren Z, Lerman JC, Gudmundsson GH, Agerberth B, Shai Y. Structure and organization of the human antimicrobial peptide LL-37 in phospholipid membranes: relevance to the molecular basis for its non-cell-selective activity. Biochem J. (1999) 341(Pt 3):501–13.
59. Henzler Wildman KA, Lee D-K, Ramamoorthy A. Mechanism of lipid bilayer disruption by the human antimicrobial peptide, LL-37. Biochemistry. (2003) 42:6545–58. doi: 10.1021/bi0273563
60. Lee C-C, Sun Y, Qian S, Huang HW. Transmembrane pores formed by human antimicrobial peptide LL-37. Biophys J. (2011) 100:1688–96. doi: 10.1016/j.bpj.2011.02.018
61. Xhindoli D, Pacor S, Benincasa M, Scocchi M, Gennaro R, Tossi A. The human cathelicidin LL-37-A pore-forming antibacterial peptide and host-cell modulator. Biochim Biophys Acta. (2016) 1858:546–66. doi: 10.1016/j.bbamem.2015.11.003
62. Sochacki KA, Barns KJ, Bucki R, Weisshaar JC. Real-time attack on single Escherichia coli cells by the human antimicrobial peptide LL-37. Proc Natl Acad Sci USA. (2011) 108:E77–81. doi: 10.1073/pnas.1101130108
63. Zhu Y, Mohapatra S, Weisshaar JC. Rigidification of the Escherichia coli cytoplasm by the human antimicrobial peptide LL-37 revealed by superresolution fluorescence microscopy. Proc Natl Acad Sci USA. (2019) 116:1017–26. doi: 10.1073/pnas.1814924116
64. Snoussi M, Talledo JP, Del Rosario N-A, Mohammadi S, Ha B-Y, Košmrlj A, et al. Heterogeneous absorption of antimicrobial peptide LL37 in Escherichia coli cells enhances population survivability. Elife. (2018) 7:e38174. doi: 10.7554/eLife.38174
65. Boman HG, Agerberth B, Boman A. Mechanisms of action on Escherichia coli of cecropin P1 and PR-39, two antibacterial peptides from pig intestine. Infect Immun. (1993) 61:2978–84.
66. Islam D, Bandholtz L, Nilsson J, Wigzell H, Christensson B, Agerberth B, et al. Downregulation of bactericidal peptides in enteric infections: a novel immune escape mechanism with bacterial DNA as a potential regulator. Nat Med. (2001) 7:180–5. doi: 10.1038/84627
67. Chakraborty K, Ghosh S, Koley H, Mukhopadhyay AK, Ramamurthy T, Saha DR, et al. Bacterial exotoxins downregulate cathelicidin (hCAP-18/LL-37) and human beta-defensin 1 (HBD-1) expression in the intestinal epithelial cells. Cell Microbiol. (2008) 10:2520–37. doi: 10.1111/j.1462-5822.2008.01227.x
68. Turner J, Cho Y, Dinh NN, Waring AJ, Lehrer RI. Activities of LL-37, a cathelin-associated antimicrobial peptide of human neutrophils. Antimicrob Agents Chemother. (1998) 42:2206–14.
69. Dalebroux ZD, Miller SI. Salmonellae PhoPQ regulation of the outer membrane to resist innate immunity. Curr Opin Microbiol. (2014) 17:106–13. doi: 10.1016/j.mib.2013.12.005
70. Bommineni YR, Dai H, Gong Y-X, Soulages JL, Fernando SC, Desilva U, et al. Fowlicidin-3 is an alpha-helical cationic host defense peptide with potent antibacterial and lipopolysaccharide-neutralizing activities. FEBS J. (2007) 274:418–28. doi: 10.1111/j.1742-4658.2006.05589.x
71. Kao C, Lin X, Yi G, Zhang Y, Rowe-Magnus DA, Bush K. Cathelicidin antimicrobial peptides with reduced activation of Toll-like receptor signaling have potent bactericidal activity against colistin-resistant bacteria. mBio. (2016) 7:e01418–16. doi: 10.1128/mBio.01418-16
72. Rowe-Magnus DA, Kao AY, Prieto AC, Pu M, Kao C. Cathelicidin peptides restrict bacterial growth via membrane perturbation and induction of reactive oxygen species. mBio. (2019) 10:e02021–19. doi: 10.1128/mBio.02021-19
73. Scheenstra MR, van den Belt M, Tjeerdsma-van Bokhoven JLM, Schneider VAF, Ordonez SR, van Dijk A, et al. Cathelicidins PMAP-36, LL-37 and CATH-2 are similar peptides with different modes of action. Sci Rep. (2019) 9:4780. doi: 10.1038/s41598-019-41246-6
74. Raschig J, Mailänder-Sánchez D, Berscheid A, Berger J, Strömstedt AA, Courth LF, et al. Ubiquitously expressed Human beta defensin 1 (hBD1) forms bacteria-entrapping nets in a redox dependent mode of action. PLoS Pathog. (2017) 13:e1006261. doi: 10.1371/journal.ppat.1006261
75. Cobo ER, Kissoon-Singh V, Moreau F, Chadee K. Colonic MUC2 mucin regulates the expression and antimicrobial activity of β-defensin 2. Mucosal Immunol. (2015) 8:1360–72. doi: 10.1038/mi.2015.27
76. Harder J, Bartels J, Christophers E, Schröder JM. A peptide antibiotic from human skin. Nature. (1997) 387:861. doi: 10.1038/43088
77. Hamanaka Y, Nakashima M, Wada A, Ito M, Kurazono H, Hojo H, et al. Expression of human beta-defensin 2 (hBD-2) in Helicobacter pylori induced gastritis: antibacterial effect of hBD-2 against Helicobacter pylori. Gut. (2001) 49:481–487. doi: 10.1136/gut.49.4.481
78. Harder J, Bartels J, Christophers E, Schroder JM. Isolation and characterization of human beta -defensin-3, a novel human inducible peptide antibiotic. J Biol Chem. (2001) 276:5707–13. doi: 10.1074/jbc.M008557200
79. Sharma H, Nagaraj R. Human β-defensin 4 with non-native disulfide bridges exhibit antimicrobial activity. PLoS ONE. (2015) 10:e0119525. doi: 10.1371/journal.pone.0119525
80. Propheter DC, Chara AL, Harris TA, Ruhn KA, Hooper LV. Resistin-like molecule β is a bactericidal protein that promotes spatial segregation of the microbiota and the colonic epithelium. Proc Natl Acad Sci USA. (2017) 114:11027–33. doi: 10.1073/pnas.1711395114
81. Miki T, Goto R, Fujimoto M, Okada N, Hardt W-D. The bactericidal lectin RegIIIβ prolongs gut colonization and enteropathy in the streptomycin mouse model for Salmonella diarrhea. Cell Host Microbe. (2017) 21:195–207. doi: 10.1016/j.chom.2016.12.008
82. Stelter C, Käppeli R, König C, Krah A, Hardt W-D, Stecher B, et al. Salmonella-induced mucosal lectin RegIIIβ kills competing gut microbiota. PLoS ONE. (2011) 6:e20749. doi: 10.1371/journal.pone.0020749
83. Cash HL, Whitham CV, Behrendt CL, Hooper LV. Symbiotic bacteria direct expression of an intestinal bactericidal lectin. Science. (2006) 313:1126–30. doi: 10.1126/science.1127119
84. Mukherjee S, Zheng H, Derebe MG, Callenberg KM, Partch CL, Rollins D, et al. Antibacterial membrane attack by a pore-forming intestinal C-type lectin. Nature. (2014) 505:103–7. doi: 10.1038/nature12729
85. Smith JJ, Travis SM, Greenberg EP, Welsh MJ. Cystic fibrosis airway epithelia fail to kill bacteria because of abnormal airway surface fluid. Cell. (1996) 85:229–36. doi: 10.1016/s0092-8674(00)81099-5
86. Johansson J, Gudmundsson GH, Rottenberg ME, Berndt KD, Agerberth B. Conformation-dependent antibacterial activity of the naturally occurring human peptide LL-37. J Biol Chem. (1998) 273:3718–24. doi: 10.1074/jbc.273.6.3718
87. Wang Y, Agerberth B, Löthgren A, Almstedt A, Johansson J. Apolipoprotein A-I binds and inhibits the human antibacterial/cytotoxic peptide LL-37. J Biol Chem. (1998) 273:33115–8. doi: 10.1074/jbc.273.50.33115
88. Scott A, Weldon S, Buchanan PJ, Schock B, Ernst RK, McAuley DF, et al. Evaluation of the ability of LL-37 to neutralise LPS in vitro and ex vivo. PLoS ONE. (2011) 6:e26525. doi: 10.1371/journal.pone.0026525
89. Guo L, Lim KB, Poduje CM, Daniel M, Gunn JS, Hackett M, et al. Lipid A acylation and bacterial resistance against vertebrate antimicrobial peptides. Cell. (1998) 95:189–98. doi: 10.1016/s0092-8674(00)81750-x
90. Böcker U, Yezerskyy O, Feick P, Manigold T, Panja A, Kalina U, et al. Responsiveness of intestinal epithelial cell lines to lipopolysaccharide is correlated with Toll-like receptor 4 but not Toll-like receptor 2 or CD14 expression. Int J Colorectal Dis. (2003) 18:25–32. doi: 10.1007/s00384-002-0415-6
91. McHugh BJ, Wang R, Li H-N, Beaumont PE, Kells R, Stevens H, et al. Cathelicidin is a “fire alarm”, generating protective NLRP3-dependent airway epithelial cell inflammatory responses during infection with Pseudomonas aeruginosa. PLoS Pathog. (2019) 15:e1007694. doi: 10.1371/journal.ppat.1007694
92. Jung K, Eyerly B, Annamalai T, Lu Z, Saif LJ. Structural alteration of tight and adherens junctions in villous and crypt epithelium of the small and large intestine of conventional nursing piglets infected with porcine epidemic diarrhea virus. Vet Microbiol. (2015) 177:373–8. doi: 10.1016/j.vetmic.2015.03.022
93. Yi H, Zhang L, Gan Z, Xiong H, Yu C, Du H, et al. High therapeutic efficacy of cathelicidin-WA against postweaning diarrhea via inhibiting inflammation and enhancing epithelial barrier in the intestine. Sci Rep. (2016) 6:25679. doi: 10.1038/srep25679
94. Akiyama T, Niyonsaba F, Kiatsurayanon C, Nguyen TT, Ushio H, Fujimura T, et al. The human cathelicidin LL-37 host defense peptide upregulates tight junction-related proteins and increases human epidermal keratinocyte barrier function. J Innate Immun. (2014) 6:739–53. doi: 10.1159/000362789
95. Mack DR, Michail S, Wei S, McDougall L, Hollingsworth MA. Probiotics inhibit enteropathogenic E. coli adherence in vitro by inducing intestinal mucin gene expression. Am J Physiol. (1999) 276:G941–50. doi: 10.1152/ajpgi.1999.276.4.G941
96. Bergstrom KSB, Kissoon-Singh V, Gibson DL, Ma C, Montero M, Sham HP, et al. Muc2 protects against lethal infectious colitis by disassociating pathogenic and commensal bacteria from the colonic mucosa. PLoS Pathog. (2010) 6:e1000902. doi: 10.1371/journal.ppat.1000902
97. Johansson MEV, Phillipson M, Petersson J, Velcich A, Holm L, Hansson GC. The inner of the two Muc2 mucin-dependent mucus layers in colon is devoid of bacteria. Proc Natl Acad Sci USA. (2008) 105:15064–9. doi: 10.1073/pnas.0803124105
98. Zhang Y, Zhu M, Yang Z, Pan X, Jiang Y, Sun C, et al. The human cathelicidin LL-37 induces MUC5AC mucin production by airway epithelial cells via TACE-TGF-α-EGFR pathway. Exp Lung Res. (2014) 40:333–42. doi: 10.3109/01902148.2014.926434
99. Yoshimura T, McLean MH, Dzutsev AK, Yao X, Chen K, Huang J, et al. The antimicrobial peptide CRAMP is essential for colon homeostasis by maintaining microbiota balance. J Immunol. (2018) 200:2174–85. doi: 10.4049/jimmunol.1602073
100. Lebeis SL, Bommarius B, Parkos CA, Sherman MA, Kalman D. TLR signaling mediated by MyD88 is required for a protective innate immune response by neutrophils to Citrobacter rodentium. J Immunol. (2007) 179:566–77. doi: 10.4049/jimmunol.179.1.566
101. McDermott AJ, Falkowski NR, McDonald RA, Pandit CR, Young VB, Huffnagle GB. Interleukin-23 (IL-23), independent of IL-17 and IL-22, drives neutrophil recruitment and innate inflammation during Clostridium difficile colitis in mice. Immunology. (2016) 147:114–24. doi: 10.1111/imm.12545
102. Barlow PG, Li Y, Wilkinson TS, Bowdish DME, Lau YE, Cosseau C, et al. The human cationic host defense peptide LL-37 mediates contrasting effects on apoptotic pathways in different primary cells of the innate immune system. J Leukoc Biol. (2006) 80:509–20. doi: 10.1189/jlb.1005560
103. Podolnikova NP, Podolnikov AV, Haas TA, Lishko VK, Ugarova TP. Ligand recognition specificity of leukocyte integrin αMβ2 (Mac-1, CD11b/CD18) and its functional consequences. Biochemistry. (2015) 54:1408–20. doi: 10.1021/bi5013782
104. Lishko VK, Moreno B, Podolnikova NP, Ugarova TP. Identification of human cathelicidin peptide LL-37 as a ligand for macrophage integrin αMβ2 (Mac-1, CD11b/CD18) that promotes phagocytosis by opsonizing bacteria. Res Rep Biochem. (2016) 2016:39–55. doi: 10.2147/rrbc.s107070
105. Wantha S, Alard J-E, Megens RTA, van der Does AM, Döring Y, Drechsler M, et al. Neutrophil-derived cathelicidin promotes adhesion of classical monocytes. Circ Res. (2013) 112:792–801. doi: 10.1161/CIRCRESAHA.112.300666
106. Suzuki K, Murakami T, Kuwahara-Arai K, Tamura H, Hiramatsu K, Nagaoka I. Human anti-microbial cathelicidin peptide LL-37 suppresses the LPS-induced apoptosis of endothelial cells. Int Immunol. (2011) 23:185–93. doi: 10.1093/intimm/dxq471
107. Shahid M, Cavalcante PA, Knight CG, Barkema HW, Han B, Gao J, et al. Murine and human cathelicidins contribute differently to hallmarks of mastitis induced by pathogenic Prototheca bovis algae. Front Cell Infect Microbiol. (2020) 10:31. doi: 10.3389/fcimb.2020.00031
108. Zanetti M. The role of cathelicidins in the innate host defenses of mammals. Curr Issues Mol Biol. (2005) 7:179–96.
109. Barlow PG, Svoboda P, Mackellar A, Nash AA, York IA, Pohl J, et al. Antiviral activity and increased host defense against influenza infection elicited by the human cathelicidin LL-37. PLoS ONE. (2011) 6:e25333. doi: 10.1371/journal.pone.0025333
110. Alt JA, Qin X, Pulsipher A, Orb Q, Orlandi RR, Zhang J, et al. Topical cathelicidin (LL-37) an innate immune peptide induces acute olfactory epithelium inflammation in a mouse model. Int Forum Allergy Rhinol. (2015) 5:1141–50. doi: 10.1002/alr.21634
111. Xia X, Zhang L, Wang Y. The antimicrobial peptide cathelicidin-BF could be a potential therapeutic for Salmonella typhimurium infection. Microbiol Res. (2015) 171:45–51. doi: 10.1016/j.micres.2014.12.009
112. Haiwen Z, Rui H, Bingxi Z, Qingfeng G, Beibei W, Jifeng Z, et al. Cathelicidin- derived PR39 protects enterohemorrhagic Escherichia coli O157:H7 challenged mice by improving epithelial function and balancing the microbiota in the intestine. Sci Rep. (2019) 9:9456. doi: 10.1038/s41598-019-45913-6
113. Yi H, Hu W, Chen S, Lu Z, Wang Y. Cathelicidin-WA improves intestinal epithelial barrier function and enhances host defense against enterohemorrhagic Escherichia coli O157:H7 infection. J Immunol. (2017) 198:1696–705. doi: 10.4049/jimmunol.1601221
114. Mishra NM, Briers Y, Lamberigts C, Steenackers H, Robijns S, Landuyt B, et al. Evaluation of the antibacterial and antibiofilm activities of novel CRAMP-vancomycin conjugates with diverse linkers. Org Biomol Chem. (2015) 13:7477–86. doi: 10.1039/c5ob00830a
115. Scott MG, Dullaghan E, Mookherjee N, Glavas N, Waldbrook M, Thompson A, et al. An anti-infective peptide that selectively modulates the innate immune response. Nat Biotechnol. (2007) 25:465–72. doi: 10.1038/nbt1288
116. Niyonsaba F, Madera L, Afacan N, Okumura K, Ogawa H, Hancock REW. The innate defense regulator peptides IDR-HH2, IDR-1002, and IDR-1018 modulate human neutrophil functions. J Leukoc Biol. (2013) 94:159–70. doi: 10.1189/jlb.1012497
117. Wu BC, Lee AH-Y, Hancock REW. Mechanisms of the innate defense regulator peptide-1002 anti-inflammatory activity in a sterile inflammation mouse model. J Immunol. (2017) 199:3592–603. doi: 10.4049/jimmunol.1700985
118. Wuerth K, Lee AHY, Falsafi R, Gill EE, Hancock REW. Characterization of host responses during Pseudomonas aeruginosa acute infection in the lungs and blood and after treatment with the synthetic immunomodulatory peptide IDR-1002. Infect Immun. (2019) 87:e00661–18. doi: 10.1128/IAI.00661-18
119. Huante-Mendoza A, Silva-García O, Oviedo-Boyso J, Hancock REW, Baizabal-Aguirre VM. Peptide IDR-1002 inhibits NF-κB nuclear translocation by inhibition of IκBα degradation and activates p38/ERK1/2-MSK1-dependent CREB phosphorylation in macrophages stimulated with lipopolysaccharide. Front Immunol. (2016) 7:533. doi: 10.3389/fimmu.2016.00533
120. Meade KG, O'Farrelly C. β-Defensins: farming the microbiome for homeostasis and health. Front Immunol. (2018) 9:3072. doi: 10.3389/fimmu.2018.03072
121. Morrison GM, Davidson DJ, Kilanowski FM, Borthwick DW, Crook K, Maxwell AI, et al. Mouse beta defensin-1 is a functional homolog of human beta defensin-1. Mamm Genome. (1998) 9:453–7. doi: 10.1007/s003359900795
122. Sechet E, Telford E, Bonamy C, Sansonetti PJ, Sperandio B. Natural molecules induce and synergize to boost expression of the human antimicrobial peptide β-defensin-3. Proc Natl Acad Sci USA. (2018) 115:E9869–78. doi: 10.1073/pnas.1805298115
123. Nguyen GC, Kaplan GG, Harris ML, Brant SR. A national survey of the prevalence and impact of Clostridium difficile infection among hospitalized inflammatory bowel disease patients. Am J Gastroenterol. (2008) 103:1443–50. doi: 10.1111/j.1572-0241.2007.01780.x
124. Rodemann JF, Dubberke ER, Reske KA, Seo DH, Stone CD. Incidence of Clostridium difficile infection in inflammatory bowel disease. Clin Gastroenterol Hepatol. (2007) 5:339–44. doi: 10.1016/j.cgh.2006.12.027
125. Yeaman MR, Yount NY. Mechanisms of antimicrobial peptide action and resistance. Pharmacol Rev. (2003) 55:27–55. doi: 10.1124/pr.55.1.2
126. Yang D, Chertov O, Bykovskaia SN, Chen Q, Buffo MJ, Shogan J, et al. Beta-defensins: linking innate and adaptive immunity through dendritic and T cell CCR6. Science. (1999) 286:525–8. doi: 10.1126/science.286.5439.525
127. Wu Z, Hoover DM, Yang D, Boulègue C, Santamaria F, Oppenheim JJ, et al. Engineering disulfide bridges to dissect antimicrobial and chemotactic activities of human beta-defensin 3. Proc Natl Acad Sci USA. (2003) 100:8880–85. doi: 10.1073/pnas.1533186100
128. Biragyn A, Surenhu M, Yang D, Ruffini PA, Haines BA, Klyushnenkova E, et al. Mediators of innate immunity that target immature, but not mature, dendritic cells induce antitumor immunity when genetically fused with nonimmunogenic tumor antigens. J Immunol. (2001) 167:6644–53. doi: 10.4049/jimmunol.167.11.6644
129. Röhrl J, Yang D, Oppenheim JJ, Hehlgans T. Identification and biological characterization of mouse beta-defensin 14, the orthologue of human beta-defensin 3. J Biol Chem. (2008) 283:5414–19. doi: 10.1074/jbc.M709103200
130. Biragyn A, Ruffini PA, Leifer CA, Klyushnenkova E, Shakhov A, Chertov O, et al. Toll-like receptor 4-dependent activation of dendritic cells by beta-defensin 2. Science. (2002) 298:1025–9. doi: 10.1126/science.1075565
131. Funderburg N, Lederman MM, Feng Z, Drage MG, Jadlowsky J, Harding CV, et al. Human -defensin-3 activates professional antigen-presenting cells via Toll-like receptors 1 and 2. Proc Natl Acad Sci USA. (2007) 104:18631–5. doi: 10.1073/pnas.0702130104
132. Wanke D, Mauch-Mücke K, Holler E, Hehlgans T. Human beta-defensin-2 and−3 enhance pro-inflammatory cytokine expression induced by TLR ligands via ATP-release in a P2X7R dependent manner. Immunobiology. (2016) 221:1259–65. doi: 10.1016/j.imbio.2016.06.006
133. Boniotto M, Jordan WJ, Eskdale J, Tossi A, Antcheva N, Crovella S, et al. Human beta-defensin 2 induces a vigorous cytokine response in peripheral blood mononuclear cells. Antimicrob Agents Chemother. (2006) 50:1433–41. doi: 10.1128/AAC.50.4.1433-1441.2006
134. Bensch KW, Raida M, Mägert HJ, Schulz-Knappe P, Forssmann WG. hBD-1: a novel beta-defensin from human plasma. FEBS Lett. (1995) 368:331–5. doi: 10.1016/0014-5793(95)00687-5
135. Duits LA, Ravensbergen B, Rademaker M, Hiemstra PS, Nibbering PH. Expression of beta-defensin 1 and 2 mRNA by human monocytes, macrophages and dendritic cells. Immunology. (2002) 106:517–25. doi: 10.1046/j.1365-2567.2002.01430.x
136. Vongsa RA, Zimmerman NP, Dwinell MB. CCR6 regulation of the actin cytoskeleton orchestrates human beta defensin-2- and CCL20-mediated restitution of colonic epithelial cells. J Biol Chem. (2009) 284:10034–45. doi: 10.1074/jbc.M805289200
137. Otte J-M, Werner I, Brand S, Chromik AM, Schmitz F, Kleine M, et al. Human beta defensin 2 promotes intestinal wound healing in vitro. J Cell Biochem. (2008) 104:2286–97. doi: 10.1002/jcb.21787
138. Kiatsurayanon C, Niyonsaba F, Smithrithee R, Akiyama T, Ushio H, Hara M, et al. Host defense (antimicrobial) peptide, human β-defensin-3, improves the function of the epithelial tight-junction barrier in human keratinocytes. J Invest Dermatol. (2014) 134:2163–73. doi: 10.1038/jid.2014.143
139. Narushima Y, Unno M, Nakagawara K, Mori M, Miyashita H, Suzuki Y, et al. Structure, chromosomal localization and expression of mouse genes encoding type III Reg, RegIII alpha, RegIII beta, RegIII gamma. Gene. (1997) 185:159–68. doi: 10.1016/s0378-1119(96)00589-6
140. Lehotzky RE, Partch CL, Mukherjee S, Cash HL, Goldman WE, Gardner KH, et al. Molecular basis for peptidoglycan recognition by a bactericidal lectin. Proc Natl Acad Sci USA. (2010) 107:7722–7. doi: 10.1073/pnas.0909449107
141. Terazono K, Yamamoto H, Takasawa S, Shiga K, Yonemura Y, Tochino Y, et al. A novel gene activated in regenerating islets. J Biol Chem. (1988) 263:2111–14.
142. Shin JH, Seeley RJ. Reg3 proteins as gut hormones? Endocrinology. (2019) 160:1506–14. doi: 10.1210/en.2019-00073
143. Takasawa S. Regenerating gene (REG) product and its potential clinical usage. Expert Opin Ther Targets. (2016) 20:541–50. doi: 10.1517/14728222.2016.1123691
144. Abe M, Nata K, Akiyama T, Shervani NJ, Kobayashi S, Tomioka-Kumagai T, et al. Identification of a novel Reg family gene, Reg IIIdelta, and mapping of all three types of Reg family gene in a 75 kilobase mouse genomic region. Gene. (2000) 246:111–22. doi: 10.1016/s0378-1119(00)00059-7
145. Ogawa H, Fukushima K, Naito H, Funayama Y, Unno M, Takahashi K, et al. Increased expression of HIP/PAP and regenerating gene III in human inflammatory bowel disease and a murine bacterial reconstitution model. Inflamm Bowel Dis. (2003) 9:162–70. doi: 10.1097/00054725-200305000-00003
146. Burger-van Paassen N, Loonen LMP, Witte-Bouma J, Korteland-van Male AM, de Bruijn ACJM, van der Sluis M, et al. Mucin Muc2 deficiency and weaning influences the expression of the innate defense genes Reg3β, Reg3γ and angiogenin-4. PLoS ONE. (2012) 7:e38798. doi: 10.1371/journal.pone.0038798
147. Iovanna J, Orelle B, Keim V, Dagorn JC. Messenger RNA sequence and expression of rat pancreatitis-associated protein, a lectin-related protein overexpressed during acute experimental pancreatitis. J Biol Chem. (1991) 266:24664–9.
148. Okamoto H. The Reg gene family and Reg proteins: with special attention to the regeneration of pancreatic beta-cells. J Hepatobiliary Pancreat Surg. (1999) 6:254–62.
149. Natividad JMM, Hayes CL, Motta J-P, Jury J, Galipeau HJ, Philip V, et al. Differential induction of antimicrobial REGIII by the intestinal microbiota and Bifidobacterium breve NCC2950. Appl Environ Microbiol. (2013) 79:7745–54. doi: 10.1128/AEM.02470-13
150. Brandl K, Plitas G, Schnabl B, DeMatteo RP, Pamer EG. MyD88-mediated signals induce the bactericidal lectin RegIII gamma and protect mice against intestinal Listeria monocytogenes infection. J Exp Med. (2007) 204:1891–900. doi: 10.1084/jem.20070563
151. Brandl K, Plitas G, Mihu CN, Ubeda C, Jia T, Fleisher M, et al. Vancomycin-resistant enterococci exploit antibiotic-induced innate immune deficits. Nature. (2008) 455:804–7. doi: 10.1038/nature07250
152. Natividad JMM, Petit V, Huang X, de Palma G, Jury J, Sanz Y, et al. Commensal and probiotic bacteria influence intestinal barrier function and susceptibility to colitis in Nod1−/−; Nod2−/− mice. Inflamm Bowel Dis. (2012) 18:1434–46. doi: 10.1002/ibd.22848
153. Liang SC, Tan X-Y, Luxenberg DP, Karim R, Dunussi-Joannopoulos K, Collins M, et al. Interleukin (IL)-22 and IL-17 are coexpressed by Th17 cells and cooperatively enhance expression of antimicrobial peptides. J Exp Med. (2006) 203:2271–9. doi: 10.1084/jem.20061308
154. Zheng Y, Valdez PA, Danilenko DM, Hu Y, Sa SM, Gong Q, et al. Interleukin-22 mediates early host defense against attaching and effacing bacterial pathogens. Nat Med. (2008) 14:282–9. doi: 10.1038/nm1720
155. Pickert G, Neufert C, Leppkes M, Zheng Y, Wittkopf N, Warntjen M, et al. STAT3 links IL-22 signaling in intestinal epithelial cells to mucosal wound healing. J Exp Med. (2009) 206:1465–72. doi: 10.1084/jem.20082683
156. Kinnebrew MA, Ubeda C, Zenewicz LA, Smith N, Flavell RA, Pamer EG. Bacterial flagellin stimulates Toll-like receptor 5-dependent defense against vancomycin-resistant Enterococcus infection. J Infect Dis. (2010) 201:534–43. doi: 10.1086/650203
157. Geddes K, Rubino SJ, Magalhaes JG, Streutker C, Le Bourhis L, Cho JH, et al. Identification of an innate T helper type 17 response to intestinal bacterial pathogens. Nat Med. (2011) 17:837–44. doi: 10.1038/nm.2391
158. Vaishnava S, Yamamoto M, Severson KM, Ruhn KA, Yu X, Koren O, et al. The antibacterial lectin RegIIIgamma promotes the spatial segregation of microbiota and host in the intestine. Science. (2011) 334:255–8. doi: 10.1126/science.1209791
159. Miki T, Holst O, Hardt W-D. The bactericidal activity of the C-type lectin RegIIIβ against Gram-negative bacteria involves binding to lipid A. J Biol Chem. (2012) 287:34844–55. doi: 10.1074/jbc.M112.399998
160. Mukherjee S, Partch CL, Lehotzky RE, Whitham CV, Chu H, Bevins CL, et al. Regulation of C-type lectin antimicrobial activity by a flexible N-terminal prosegment. J Biol Chem. (2009) 284:4881–8. doi: 10.1074/jbc.M808077200
161. Wilson CL, Ouellette AJ, Satchell DP, Ayabe T, López-Boado YS, Stratman JL, et al. Regulation of intestinal alpha-defensin activation by the metalloproteinase matrilysin in innate host defense. Science. (1999) 286:113–17. doi: 10.1126/science.286.5437.113
162. Ghosh D, Porter E, Shen B, Lee SK, Wilk D, Drazba J, et al. Paneth cell trypsin is the processing enzyme for human defensin-5. Nat Immunol. (2002) 3:583–90. doi: 10.1038/ni797
163. Keilbaugh SA, Shin ME, Banchereau RF, McVay LD, Boyko N, Artis D, et al. Activation of RegIIIbeta/gamma and interferon gamma expression in the intestinal tract of SCID mice: an innate response to bacterial colonisation of the gut. Gut. (2005) 54:623–9. doi: 10.1136/gut.2004.056028
164. Loonen LMP, Stolte EH, Jaklofsky MTJ, Meijerink M, Dekker J, van Baarlen P, et al. REG3γ-deficient mice have altered mucus distribution and increased mucosal inflammatory responses to the microbiota and enteric pathogens in the ileum. Mucosal Immunol. (2014) 7:939–47. doi: 10.1038/mi.2013.109
165. Steppan CM, Brown EJ, Wright CM, Bhat S, Banerjee RR, Dai CY, et al. A family of tissue-specific resistin-like molecules. Proc Natl Acad Sci USA. (2001) 98:502–6. doi: 10.1073/pnas.98.2.502
166. Pine GM, Batugedara HM, Nair MG. Here, there and everywhere: resistin-like molecules in infection, inflammation, and metabolic disorders. Cytokine. (2018) 110:442–51. doi: 10.1016/j.cyto.2018.05.014
167. Gerstmayer B, Küsters D, Gebel S, Müller T, Van Miert E, Hofmann K, et al. Identification of RELMgamma, a novel resistin-like molecule with a distinct expression pattern. Genomics. (2003) 81:588–95. doi: 10.1016/s0888-7543(03)00070-3
168. Holcomb IN, Kabakoff RC, Chan B, Baker TW, Gurney A, Henzel W, et al. FIZZ1, a novel cysteine-rich secreted protein associated with pulmonary inflammation, defines a new gene family. EMBO J. (2000) 19:4046–55. doi: 10.1093/emboj/19.15.4046
169. Loke P, Nair MG, Parkinson J, Guiliano D, Blaxter M, Allen JE. IL-4 dependent alternatively-activated macrophages have a distinctive in vivo gene expression phenotype. BMC Immunol. (2002) 3:7. doi: 10.1186/1471-2172-3-7
170. Nair MG, Gallagher IJ, Taylor MD, Loke P, Coulson PS, Wilson RA, et al. Chitinase and Fizz family members are a generalized feature of nematode infection with selective upregulation of Ym1 and Fizz1 by antigen-presenting cells. Infect Immun. (2005) 73:385–94. doi: 10.1128/IAI.73.1.385-394.2005
171. Schinke T, Haberland M, Jamshidi A, Nollau P, Rueger JM, Amling M. Cloning and functional characterization of resistin-like molecule gamma. Biochem Biophys Res Commun. (2004) 314:356–62. doi: 10.1016/j.bbrc.2003.12.100
172. Jang JC, Chen G, Wang SH, Barnes MA, Chung JI, Camberis M, et al. Macrophage-derived human resistin is induced in multiple helminth infections and promotes inflammatory monocytes and increased parasite burden. PLoS Pathog. (2015) 11:e1004579. doi: 10.1371/journal.ppat.1004579
173. Kim K-H, Zhao L, Moon Y, Kang C, Sul HS. Dominant inhibitory adipocyte-specific secretory factor (ADSF)/resistin enhances adipogenesis and improves insulin sensitivity. Proc Natl Acad Sci USA. (2004) 101:6780–85. doi: 10.1073/pnas.0305905101
174. Patel SD, Rajala MW, Rossetti L, Scherer PE, Shapiro L. Disulfide-dependent multimeric assembly of resistin family hormones. Science. (2004) 304:1154–8. doi: 10.1126/science.1093466
175. Banerjee RR, Lazar MA. Dimerization of resistin and resistin-like molecules is determined by a single cysteine. J Biol Chem. (2001) 276:25970–3. doi: 10.1074/jbc.M103109200
176. He W, Wang M-L, Jiang H-Q, Steppan CM, Shin ME, Thurnheer MC, et al. Bacterial colonization leads to the colonic secretion of RELMbeta/FIZZ2, a novel goblet cell-specific protein. Gastroenterology. (2003) 125:1388–97. doi: 10.1016/j.gastro.2003.07.009
177. Pu Z, Che Y, Zhang W, Sun H, Meng T, Xie H, et al. Dual roles of IL-18 in colitis through regulation of the function and quantity of goblet cells. Int J Mol Med. (2019) 43:2291–302. doi: 10.3892/ijmm.2019.4156
178. Hill DA, Hoffmann C, Abt MC, Du Y, Kobuley D, Kirn TJ, et al. Metagenomic analyses reveal antibiotic-induced temporal and spatial changes in intestinal microbiota with associated alterations in immune cell homeostasis. Mucosal Immunol. (2010) 3:148–58. doi: 10.1038/mi.2009.132
179. Artis D, Wang ML, Keilbaugh SA, He W, Brenes M, Swain GP, et al. RELMbeta/FIZZ2 is a goblet cell-specific immune-effector molecule in the gastrointestinal tract. Proc Natl Acad Sci USA. (2004) 101:13596–600. doi: 10.1073/pnas.0404034101
180. Herbert DR, Yang J-Q, Hogan SP, Groschwitz K, Khodoun M, Munitz A, et al. Intestinal epithelial cell secretion of RELM-beta protects against gastrointestinal worm infection. J Exp Med. (2009) 206:2947–57. doi: 10.1084/jem.20091268
181. Ahmed N, Heitlinger E, Affinass N, Kühl AA, Xenophontos N, Jarquin VH, et al. A novel non-invasive method to detect RELM beta transcript in gut barrier related changes during a gastrointestinal nematode infection. Front Immunol. (2019) 10:445. doi: 10.3389/fimmu.2019.00445
182. Strober W, Fuss IJ, Blumberg RS. The immunology of mucosal models of inflammation. Annu Rev Immunol. (2002) 20:495–49. doi: 10.1146/annurev.immunol.20.100301.064816
183. Gironella M, Iovanna JL, Sans M, Gil F, Peñalva M, Closa D, et al. Anti-inflammatory effects of pancreatitis associated protein in inflammatory bowel disease. Gut. (2005) 54:1244–53. doi: 10.1136/gut.2004.056309
184. Krimi RB, Letteron P, Chedid P, Nazaret C, Ducroc R, Marie J-C. Resistin-like molecule-beta inhibits SGLT-1 activity and enhances GLUT2-dependent jejunal glucose transport. Diabetes. (2009) 58:2032–8. doi: 10.2337/db08-1786
185. Osborne LC, Joyce KL, Alenghat T, Sonnenberg GF, Giacomin PR, Du Y, et al. Resistin-like molecule α promotes pathogenic Th17 cell responses and bacterial-induced intestinal inflammation. J Immunol. (2013) 190:2292–2300. doi: 10.4049/jimmunol.1200706
186. Munitz A, Waddell A, Seidu L, Cole ET, Ahrens R, Hogan SP, et al. Resistin-like molecule alpha enhances myeloid cell activation and promotes colitis. J Allergy Clin Immunol. (2008) 122:1200–1207.e1. doi: 10.1016/j.jaci.2008.10.017
187. Munitz A, Seidu L, Cole ET, Ahrens R, Hogan SP, Rothenberg ME. Resistin-like molecule alpha decreases glucose tolerance during intestinal inflammation. J Immunol. (2009) 182:2357–63. doi: 10.4049/jimmunol.0803130
188. Yan H, Hancock RE. Synergistic interactions between mammalian antimicrobial defense peptides. Antimicrob Agents Chemother. (2001) 45:1558–60. doi: 10.1128/AAC.45.5.1558-1560.2001
189. Bedran TBL, Mayer MPA, Spolidorio DP, Grenier D. Synergistic anti-inflammatory activity of the antimicrobial peptides human beta-defensin-3 (hBD-3) and cathelicidin (LL-37) in a three-dimensional co-culture model of gingival epithelial cells and fibroblasts. PLoS ONE. (2014) 9:e106766. doi: 10.1371/journal.pone.0106766
Keywords: colonic epithelium, host defense peptides, cathelicidin, defensin, colitis
Citation: Blyth GAD, Connors L, Fodor C and Cobo ER (2020) The Network of Colonic Host Defense Peptides as an Innate Immune Defense Against Enteropathogenic Bacteria. Front. Immunol. 11:965. doi: 10.3389/fimmu.2020.00965
Received: 27 February 2020; Accepted: 24 April 2020;
Published: 20 May 2020.
Edited by:
Charles Lee Bevins, University of California, Davis, United StatesReviewed by:
Felix Ngosa Toka, Ross University School of Veterinary Medicine, Saint Kitts and NevisAmy Mackos, The Ohio State University, United States
Copyright © 2020 Blyth, Connors, Fodor and Cobo. This is an open-access article distributed under the terms of the Creative Commons Attribution License (CC BY). The use, distribution or reproduction in other forums is permitted, provided the original author(s) and the copyright owner(s) are credited and that the original publication in this journal is cited, in accordance with accepted academic practice. No use, distribution or reproduction is permitted which does not comply with these terms.
*Correspondence: Eduardo R. Cobo, ZWNvYm9AdWNhbGdhcnkuY2E=