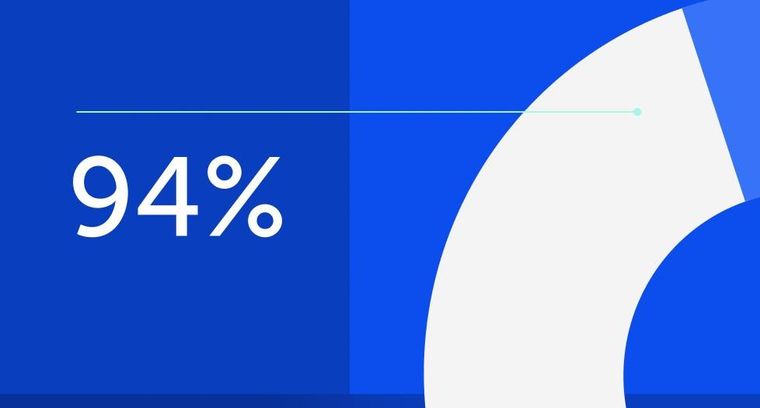
94% of researchers rate our articles as excellent or good
Learn more about the work of our research integrity team to safeguard the quality of each article we publish.
Find out more
REVIEW article
Front. Immunol., 16 March 2020
Sec. Mucosal Immunity
Volume 11 - 2020 | https://doi.org/10.3389/fimmu.2020.00378
This article is part of the Research TopicImmunological Role of the Maternal Microbiome in PregnancyView all 11 articles
In the last decade, the microbiota, i.e., combined populations of microorganisms living inside and on the surface of the human body, has increasingly attracted attention of researchers in the medical field. Indeed, since the completion of the Human Microbiome Project, insight and interest in the role of microbiota in health and disease, also through study of its combined genomes, the microbiome, has been steadily expanding. One less explored field of microbiome research has been the female reproductive tract. Research mainly from the past decade suggests that microbial communities residing in the reproductive tract represent a large proportion of the female microbial network and appear to be involved in reproductive failure and pregnancy complications. Microbiome research is facing technological and methodological challenges, as detection techniques and analysis methods are far from being standardized. A further hurdle is understanding the complex host-microbiota interaction and the confounding effect of a multitude of constitutional and environmental factors. A key regulator of this interaction is the maternal immune system that, during the peri-conceptional stage and even more so during pregnancy, undergoes considerable modulation. This review aims to summarize the current literature on reproductive tract microbiota describing the composition of microbiota in different anatomical locations (vagina, cervix, endometrium, and placenta). We also discuss putative mechanisms of interaction between such microbial communities and various aspects of the immune system, with a focus on the characteristic immunological changes during normal pregnancy. Furthermore, we discuss how abnormal microbiota composition, “dysbiosis,” is linked to a spectrum of clinical disorders related to the female reproductive system and how the maternal immune system is involved. Finally, based on the data presented in this review, the future perspectives in diagnostic approaches, research directions and therapeutic opportunities are explored.
The human microbiome represents the collection of microbes living inside and on the surface of human body. Research efforts by the scientific community worldwide are increasingly focused on understanding the role of microbiota in health and disease. Since the completion of the Human Microbiome Project (HMP), and publication of the characteristics and functions of human microbiota located in different body habitats, this field of science has gained momentum (1). Current microbiome research tries to fill in the missing details in the pathophysiology and explain the seemingly random variation in disease severity and phenotype of confounding factors such as ethnicity, geographical location and societal habits. Due to advances in microbiome research, scientists have obtained valuable insight in many complex disorders such as obesity, cancer and inflammatory bowel disease, and a similar trend is observed in female reproductive tract in both physiological and pathological states (2).
Because of the invasive nature of sampling methods, microorganisms populating the female reproductive tract remain less explored compared to microbiota populating the intestines; nonetheless, they represent an appreciable proportion (around 9%) of the female microbial network (3). Furthermore, disrupted female reproductive tract microbial communities have been implicated in reproductive and pregnancy complications, as reviewed in (4, 5). Reproductive and pregnancy complications are of global health interest, and comprise diverse health problems that occur prior to conception and during gestation. Notably, these involve the mother’s health, the baby’s health or both. Such problems may entail difficulties to conceive, or arise throughout gestation and span from the inability to maintain pregnancy in the first weeks of gestation, to its early termination in the third trimester. An increasing body of evidence associates microorganisms (including mutualistic) to the onset of reproductive health and maternal-fetal conditions, and to some of the major obstetrical syndromes, including premature birth, premature rupture of the membranes, premature labor, intrauterine growth restriction, and stillbirth (6).
The inability to conceive is an often neglected health concern affecting individuals around the globe. In 2010, an estimated 48.5 million couples worldwide were infertile, with little changes over the previous two decades (7). Early pregnancy loss (frequently before 13 weeks of gestation) is estimated to occur in 15–20% of recognized pregnancies, without major geographical differences (8–10). Of note, morbidity and pregnancy complications at more advanced stages of gestation (e.g., preeclampsia, preterm labor and stillbirth) often have a higher burden in low-resources settings, especially in south Asia and sub-Saharan Africa (11). Among such late complications, preterm birth remains the leading cause of worldwide neonatal morbidity and mortality: approximately 10.6% of all live births in 2014 were preterm, 80% of which occurred in Asian and sub-Saharan African countries (12).
Over recent years technological advancements, in particular sequencing-based methods for bacterial detection (metagenomics and 16S rRNA gene amplicon sequencing), have greatly expanded the literature on diverse microbiota colonizing the female reproductive tract both in healthy and disease states (5, 13). However, the wide spread application of sequencing-based methods incorporates a potential for false-positive results (i.e., low specificity) due to contamination as in case of the placenta (14). Our current knowledge is limited on how these microbiota interact with host cells, including local immune mediators, and whether this interaction is causally involved in the pathogenesis of pregnancy complications. It is well known that the mechanisms pivotal in regulating the establishment and maintenance of pregnancy, including epigenetic regulation and immune adaptation, are directly affected by local microorganisms (5, 13). Furthermore, the impact of maternal factors such as BMI, pre-existing disorders or life style habits (e.g., diet and nutrition) on this host-microbiota interaction need to be integrated and thoroughly studied in future studies (15). Understanding the interplay at the fetomaternal interface is essential for developing predictive human biomarkers for implantation and placentation and is a key step toward designing novel therapeutic approaches.
This review aims to present an account of currently available literature on reproductive tract microbiota, describing the composition and function of microbiota and their links to pregnancy complications. The potential mechanisms of interaction between microbes and the immune system are discussed with respect to specific locations, focusing on the unique changes that characterize physiological pregnancy. Furthermore, the clinical impact of abnormal microbiota composition, i.e., “dysbiosis,” on female reproductive biology, from the pre-conceptional stage throughout early and late pregnancy. Common methodological challenges confronting research in the field related to study design and technical issues of sample collection and assay standardization are highlighted. Finally, we present options with respect to translation of various aspects and insights to future therapeutic approaches in reproductive medicine.
The community of microbes in the female lower genital tract plays a fundamental role in the promotion of homeostasis and in the prevention of colonization by pathogenic microorganisms. Compared to other sites, the vagina appears to harbor particularly simple microbial communities of low diversity (1). Although relatively simple at the genus-level, the diversity of Lactobacilli in the vaginal space is nonetheless higher than at other body sites (1). Many studies among non-pregnant women of reproductive age report that Lactobacillus spp. is the predominant species in the vagina, although the possibility of normal vaginal microbiota dominated by bacteria other than Lactobacilli seems to be plausible [as reviewed in (16)]. Current evidence suggests that the vaginal bacterial community is in a state of dynamic equilibrium (17). The composition of the vaginal microbiota correlates with the most dominant bacterial community composition (i.e., community state type; CST) across time (17). This notion appears to be in line with the concept of community resilience. Community resilience suggests that the ability of a microbial ecosystem to mitigate composition changes depends on the presence of beneficial species with stabilizing roles. For instance, Lactobacillus crispatus-dominated communities are less likely to transition to non-beneficial CST, than communities dominated by other Lactobacillus spp., like L. iners (17, 18).
In non-pregnant women of reproductive age, transient variations in the vaginal microbiota’s dynamic equilibrium are the results of physiological changes in response to hormones during the menstrual cycle, or human activities (e.g., sexual intercourse and hygienic practices) (18). In addition, other constitutional and environmental factors, including age, ethnicity, geographical variation and sexual habits influence the bacterial communities detected in the lower urogenital tract (19–21). For example, Lactobacilli-dominated vaginal microbiota has been shown to be less prevalent among non-Caucasian women in several studies (22–24), though, when present, their beneficial role seems maintained. A well-known study performed in North America characterized the vaginal microbiota of women of reproductive age from four ethnic groups (Caucasian, African-American, Hispanic and Asian): five groups of microbial communities, called Community State Types (CSTs) were identified. Whereas CST-IV was the most diverse CST and was also associated with a higher local pH, the remaining four (CST-I, CST-II, CST-III, and CST-V) were dominated by Lactobacilli (20). Lactobacilli thrive in anaerobic environments and produce lactic acid, therefore contributing to the acidic vaginal environment. Several studies have reported how depletion of these microorganisms often leads to vaginal dysbiosis, which is occasionally symptomatic, and at times associated with several important reproductive complications (25, 26).
The microbiota residing in the cervix have been sparsely studied as an independent entity. Current evidence suggests a strong similarity between bacterial communities in the cervix and in the vaginal area, suggesting ascending bacterial colonization from the vagina to the cervix (27). Such microbial communities are sometimes jointly referred to as cervicovaginal microbiota (28).
While in non-pregnant reproductive age women the vaginal microbiota is relatively dynamic, in healthy pregnancies it is characterized by an increase in stability (29). This is one of the physiological changes taking place in response to gestation. Other changes in this microbial community located in the vaginal area are an overall decrease in richness (number of different species present) and diversity (of the microbial ecosystem, i.e., the relative abundance of species). Overall, abundance of Lactobacillus spp. appears to be higher in healthy pregnant women, than in women with complicated pregnancies; whereas Mycoplasma and Ureaplasma appear to be lower (30). Most of the studies in the field seem to agree that Lactobacilli, in particular L. crispatus, L. iners, L. jenseii, and L. gasseri are the dominant bacteria detected in the vaginal microbiota of pregnant women (29, 31, 32).
Some of the factors influencing vaginal microbiota’s diversity during pregnancy are: gestational age (32), previous pregnancy history (33) and ethnicity (31, 34). In particular, L. crispatus was the most dominant species in a Caucasian cohort (35), L. iners in an African American one (29) and, surprisingly, L. acidophilus in a mostly Hispanic population where L. crispatus was not detected, even though tested for (36). Although ethnic and geographical differences in vaginal microbiota are not yet understood, nor consistently observed (32), a combination of colonization by gut microbes, hygienic and sexual practices, and host genetics may contribute to underlying mechanisms (37).
As pregnancy progresses, the vaginal microbiota changes with an increase in the relative abundance of Lactobacilli and decrease in anaerobe or strict-anaerobe species, until around 36 weeks of gestation, when the number of species increases significantly (31). Such composition has been reported to resemble that of the vaginal microbiota in the non-pregnant state (34). Throughout pregnancy, some species of Lactobacilli associate with “normal” (i.e., healthy) vaginal microbiota, whereas the presence of other species reflect an abnormal, less beneficial microbial community. The former has been reported for L. crispatus, whereas the latter for L. gasseri and L. iners (35). Finally, reported changes in the vaginal microbiota after delivery include a decrease in Lactobacillus species and an increase in anaerobe ones (38), irrespective of the vaginal communities during pregnancy and independent of ethnicity (39).
It is becoming increasingly apparent from studies in the field that, besides investigation of the vaginal microbiota as an ecological community (defined by its most abundant species), individual (even low abundant) species with less beneficial roles should be considered, as their mere presence might be sufficiently detrimental to a healthy microbiotal equilibrium.
Unlike the vagina, the endometrium has not been extensively studied as a site of commensal bacterial colonization, in part likely due to the relatively limited access to uncontaminated samples. Historically, the uterus has been considered sterile (40), and presence of bacteria in endometrial, placental or amniotic fluid samples was viewed as pathological. However, with the advent of culture-independent techniques, recently compiled data support both the prevalence and variation of bacterial communities in the endometrium and their possible role in reproductive health (41) but is still under debate (14). Together, much of our knowledge comes from the interpretation and comparison of the role of microbiome in anatomically related sites.
In healthy non-pregnant women, the endometrium appears to harbor a unique, low-biomass microbiota, dominated by a few bacterial species including Bacteroidetes (Flavobacterium spp.) and Firmicutes (Lactobacillus spp.) (5, 41, 42). Compared to the vaginal microbiome, the endometrium harbors a significantly lower quantity of microbes, suggesting that the cervix and/or uterine environment serve as a barrier for ascending microorganisms (42). Since Lactobacilli and Streptococci represent the most dominant bacteria in the vagina and in the cervix, respectively, the occurrence of these species in the uterine cavity may indicate contamination during sample collection. Therefore, the use of paired samples from vagina, cervix and endometrial fluid has been proposed in order to facilitate interpretation (43).
A recent review reported that, in studies using culture-dependent techniques, no bacterial family was reported more than once in uterine or endometrial samples, indicating one of the challenges in determining the complexity of “normal” reference microbiota of the uterine cavity in this manner. However, in culture-independent analyses, Lactobacilli, Bifidobacteriaceae, Comamonadaceae, and Streptococcaceae were reported more than once in the uterine cavity, suggesting that the culture-independent technology approximate microbiotal complexity more truthfully (41).
The notion that the uterus represents a sterile environment during pregnancy was first challenged with the advent of the Human Microbiome Project (HMP) (1) and other studies (44). The detection of unique bacterial DNA profiles in human placenta supported the idea that a rich microbial community normally exists in utero. Surprisingly, these early data suggested that the placental microbiome resembles the oral cavity microbiome rather than that of adjacent sites, e.g., the vagina. Since these early reports, several reservations on this notion and its implications on pregnancy complications have been voiced, noting that the low-biomass that bacterial DNA in the placenta represents, is sensitive to capturing background contamination (from DNA extraction kits, polymerase chain reaction reagents, and laboratory environments) (45, 46). Recent meticulous analyses of metagenomic DNA, consistently found no significant differences in the abundance and/or presence of a microbiota between placental tissue (term women without labor) and background technical controls (14, 47). The proposed link between oral dysbiosis and pregnancy complications puts the debate about placental microbiota in the focus: clinical studies on the association between gingivitis and PTB have reported bacteria in the very old structures of the placenta (48). In the context of placental microbiome analysis, the pitfalls associated with technology of choice, in addition to the methodological problems, needs careful consideration (see section “Discussion,” diagnostic challenges).
During normal pregnancy, the immune system of the female reproductive tract is uniquely challenged by the fact that it has to protect against invading pathogens while simultaneously tolerating and supporting implantation and growth of the semi-allogenic fetus in a tightly regulated process (49). The implantation phase is characterized by low grade pro-inflammatory immune reactivity, including the production of major cytokines IL-6, IL-8, and tumor necrosis factor (TNF)-α. This response is believed to support local repair of endometrial injury and removal of cellular debris during trophoblast invasion and implantation. The placentation phase is predominantly an anti-inflammatory state, which is needed to ascertain tolerance of maternal immune cells to paternal antigens expressed by placental trophoblasts for the fetus and, at later stages, vice versa. The final parturition phase again requires controlled pro-inflammatory immune reactivation to trigger labor, delivery and placental rejection (50). Dysregulation of this tight immunological balance, based either in the (immuno)genetic constitution of the parents or the (local) uterine environment, may underlie several pregnancy complications. The homeostasis of the female reproductive tract during pregnancy depends on the interactive protective roles of epithelial defenses and immune cells. A broad variety of innate-(predominantly macrophages, dendritic cells and innate lymphoid cells) and adaptive immune cells (especially T cells) can be found each in different anatomic compartments with their own unique and specialized role. This section summarizes key immune players involved in immuno-modulatory events that support normal pregnancy, both the genetic and environmental aspects will be discussed in successive paragraphs. The maternal immune regulation in pregnancy has been extensively described (51) and is beyond the scope of this review.
Like in many other mucosal tissues, the female reproductive tract’s first line of defense against pathogens is a physical barrier that, among other things, consists of a mucous layer, IgA antibodies and commensal bacteria limiting colonization by pathogenic bacteria. In addition, epithelial cells lining the female reproductive tract physically block pathogen invasion and produce protective molecules like antimicrobial peptides (AMPs). AMPs are multifunctional molecules with important roles in direct microbial killing, protection against proteolytic enzymes from various pathogens including bacteria, fungi, and some viruses, and modulation of both innate and adaptive immune responses (52, 53). AMPs are produced either constitutively or after induction by inflammatory stimuli, and are present on the mucosal surface, in decidual stroma, in endometrial fluid and even in amniotic fluid (54). In humans, AMPs have been linked to key regulatory processes in implantation and are implicated in the pathogenesis of various pregnancy complications (52, 55). An example of an AMP found in the uterus is the secretory leukocyte protease inhibitor (SLPI), which has antiviral and antifungal properties, and acts as a bactericidal against gram negative as well as gram-positive bacteria such as Escherichia coli and Staphylococcus aureus (56).
Natural Killer (NK) cells are a critical component of the innate immune system and comprise up to 70% of all endometrial leukocytes during the secretory phase of the menstrual cycle and in early pregnancy, but decline in numbers by mid-gestation (49). The main function NK cells in the vagina is to provide protection against a broad variety of viruses. Like their blood counterparts, vaginal NK cells recognize virus- or stress-associated molecules and they destroy infected cells through the release of toxic granules containing granzymes and perforins or though interaction with death receptors (57). The exact function of uterine NK cells (uNK) is not completely clear as they clearly differ from NK cells in peripheral blood in surface markers and cytokine repertoire. uNK cells are poorly cytotoxic, but are a potent source of cytokines such as Interferon (IFN)γ, TNF-α, Granulocyte-macrophage colony-stimulating factor (GM-CSF) and Interleukin (IL)-10 as well as proangiogenic factors like vascular endothelial growth factor (VEGF) (58–60). Studies using genetic mouse models lacking uNK cells provided histological evidence of failed trophoblast invasion and defective spiral artery remodeling and highlighted the critical role for uNK in for normal placentation (61, 62).
Myeloid cells (macrophages and dendritic cells) represent the second most abundant immune cell subset in the endometrium and account for 10–20% of the decidual leukocyte population (49). They are responsible for the surveillance and scavenging of bacteria present on mucosal surfaces and act as antigen presenting cells (APCs) (58, 63, 64). APCs are equipped with pattern recognition receptors (PRR), such as Toll-like receptors (TLRs), allowing them to recognize the so-called pathogen-associated molecular patterns (PAMPs). PAMPs are species-specific and include molecules from the microbial cell wall (e.g., peptidoglycan) and cell membranes (e.g., LPS) or virus-derived single stranded DNA or double stranded RNA. PAMPs promote the production of cytokines and cell adhesion molecules that lead to the recruitment other immune cells such as neutrophils and NK cells. In addition, APCs have a major function in instructing the adaptive immune response by virtue of expressing major histocompatibility complex (MHC) class II receptors. In contrast to uNK cells, the numbers of decidual macrophages remain relatively constant throughout gestation, however, the diverse repertoire of macrophages in cytokines production, in regulation of T cell responses and in tissue repair suggest an important role in decidualization (49, 59). Together with the uNK cells, uterine macrophages are also postulated to facilitate angiogenesis during placentation and more importantly, spiral artery remodeling by production of growth factors and clearance of cell debris (60, 65).
Uterine dendritic cells (uDC) have a tolerogenic phenotype and both uDC and uterine macrophages produce IL-10, TGF-β, and indolomine2,3 (IDO) contributing to a tolerogenic and receptive micro-environment (66). In addition, uDC have been shown to interact in a bidirectional manner with uNK cells in mouse models, as well as in vitro human studies (67, 68). As a subclass of APC, uDC promote uNK differentiation and activation in a contact-dependent manner and via the production of IL-15 (69).
Adaptive immune cells (B- and T lymphocytes) provide highly specific and long-lasting cellular and humoral immunity against pathogens. While B cells are relatively infrequent in the female reproductive tract, T cells can be consistently found in both the vaginal compartment as well as in the uterus, albeit, in the uterus, their number and phenotype highly vary depending on stage of the menstrual cycle and pregnancy. CD8+ (cytotoxic) T cells represent the most abundant adaptive lymphocyte subset in the pregnant uterus (70). CD4+ (helper) T cells are less abundant, however, important in production of cytokines and interaction with other immune cells upon activation by APC (71). Traditionally, pregnancy was considered an immune privileged state by the different cytokines produced from a dominant T-cell phenotype (Th2) involved in immune tolerance, over another phenotype (Th1) involved in immune rejection. The dominance of Th1 polarized T cells was considered detrimental to embryo implantation and was associated with obstetric complications mainly preeclampsia (72). More recent studies show that the Th1/Th2 paradigm is not inclusive enough and that fetal tolerance is a complex process involving more specialized T cell subtypes, such as Th17 and regulatory T (Treg) cells (51). In humans, Treg cells have been shown to migrate from peripheral blood to the decidua (73), and their levels peak during the second trimester of pregnancy (74). They produce IL-10, leukemia inhibitory factor (LIF), transforming growth factor (TGF)-β, and heme oxygenase 1 (HO-1) contributing to fetal-maternal immune tolerance (75). It is clear that tight regulation of T cell activation and polarization is essential to balance the protection from pathogens, mediated by Th1/Th17 CD4+ T cells and CD8+ (cytotoxic) T cells versus tolerance to paternal antigens expressed by fetal cells, mediated by Th2 and Treg cells (76).
The induction of regulatory T cells (suppressor T cells/Treg) is favored over pro-inflammatory Th17 cells through interaction of uDC and uNK cells (77), corroborating the importance of intricate immunological instruction in acquisition of tolerance during implantation. Both macrophages and uDCs can be activated by encountering pathogens in the endometrium and start the process of phagocytosis, internalization, and degradation of the components of the antigen. They subsequently present these bacterial peptides to T-cells via MHC receptors, which activate the T-cells to initiate a cell-mediated and/or humoral immune response via MHC class II molecules (78).
Even though the intricate role of the immune system in pregnancy is not completely understood, collaborative action of innate- and adaptive immune cells appear to be critical for orchestration of the immunological changes required for successful fertilization, implantation and pregnancy.
The interaction between microbiota and the immune system is a complex process that is crucial for maintaining normal homeostasis in organs, albeit under the influence of several constitutional and environmental factors. We hypothesize that under normal circumstances, a healthy lifestyle (including diet, physical and psychological aspects) would result in a normal reproductive tract microbiota “eubiosis,” kept in check by a well-balanced immune regulation (Figure 1). Disturbance of this delicate balance could lead to either to inappropriate immune response and an exaggerated inflammatory reaction, or to downregulated immune response and dominance of pathogenic bacteria over normal commensals “dysbiosis”. Evidence on the existence of such balance during in pregnancy is limited and hence, the data on host-microbiota interaction discussed in this section are largely derived from the non-pregnant population.
Figure 1. The interaction between the endometrial microbiome and local immune mediators. (A) In healthy women, commensal bacterial communities interact with immune cells with at the feto-maternal interface through three potential mechanisms: (1) Commensal bacteria (green) maintain a healthy physical barrier by stimulating the production of different antimicrobial peptides (AMP) from endometrial cells and preserving epithelial tight junctions and stable mucus production. (2) Once encountered by immune cells in the endometrium, e.g., antigen presenting cells (APC), commensal bacteria trigger signal transduction via pattern recognition receptors (PRR) through their pathogen-associated molecular patterns (PAMPs). (3) Commensal bacteria can also produce metabolites, such as polysaccharides and short-chain fatty acids (SCFAs), that potentially affect immune responses in endometrial epithelial cells and T-cells, or alter the endometrial fluid pH to produce a competitive niche microenvironment against pathogenic bacteria. These mechanisms result in activation of uterine NK (uNK) cells and the development of specific subsets of T-cells, characterized by high number of regulatory T-cells (Treg), low number of Th-17, and a switch from the Th1 to Th2 cytokine production (4). The interaction of activated uNK cells (KIR receptors) with HLA-C and -G from extravillous trophoblasts (EVT) from the implanting embryo will also promote EVT invasion, stromal matrix degradation, angiogenesis and ultimately the remodeling of maternal spiral arteries (5). These adaptive changes ensure an immunotolerant milieu for the semi-allogenic fetus and are essential steps essential step in normal placentation. (B) Disturbance of the normal endometrial microbiome can negatively impact the implantation process: (1) First, the dominance of non-commensal bacterial communities could weaken the integrity of the endometrial mucosal barrier by affecting the epithelial tight junctions and reducing AMP and mucin secretion. (2) This in turn will further weaken host defense mechanisms and allow pathogens to enter the endometrial stroma and elicit a profound immune reaction from APC and other immune cells harboring pattern recognition receptors (PRR). (3) Aberrant stimulation of T-cells, either directly from invading pathogens breaching the mucosal barrier or indirectly from absorbed bacterial products results in disbalance in cytokine production in favor of the pro-inflammatory Th-1 types, predominated by TNF-a, IFN, IL-2, and IL-10 (4). Aberration in uNK cell maturation, either primary or secondary to shallow EVT invasion, is a possible link between disturbed endometrial microbiome and incomplete remodeling of maternal spiral arteries, characteristic of the great obstetric syndromes (5).
The vaginal mucosa is a barrier that provides protection against invading pathogens, as a result of the interaction between its epithelial cells, the immune system, and symbiotic microorganisms (79). The microbiota residing in the vaginal space are an active critical component in such defense system against infections. In particular, Lactobacillus spp. are thought to protect the upper genital tract from ascending infection, such as sexually transmitted ones (80). The main mechanism associated with the protective effect Lactobacillus spp. is the ability to produce lactic acid, thus maintaining a local pH of <4.5, deleterious to pathogens (81). Another defense mechanism is the Lactobacilli’s production of bacteriocins, which directly inhibit or kill bacterial and viral pathogens (81). The ability to form micro-colonies that adhere to epithelial cells and prevent adhesion of pathogens is additional means of defense by vaginal microbiota, as is their ability to trigger the host’s defense (81). In vitro studies have shown that certain Lactobacillus species are able to temper inflammation by, for example, a reduction of IL-6, IL-8, and TNF-α secretion after bacterial stimulation of toll-like receptors (TLRs) (82). The association between Lactobacilli-poor vaginal ecosystems and an increased risk of sexually transmitted infection is strong [as reviewed in (80, 83)]. Nonetheless, non-Lactobacilli-dominated vaginal microbiota occur in 25% of asymptomatic women, which challenges the notion of Lactobacilli as the sole microbial defense mediator (17, 84). Possible other explanations may be that maintenance of low pH in non-Lactobacilli-dominated vaginal microbiota is achieved in a different manner (18), or that not all suboptimal microbiota are manifested as symptomatic, whereas such CSTs may nevertheless correlate with increased risk for adverse reproductive health outcomes (85). Furthermore, as discussed before, distinct Lactobacillus species appear to differentially affect microbiota, e.g., with L. iners being more conductive to pathogen invasion than L. crispatus (25).
Most evidence and knowledge on the interactions between vaginal microbiota and host immune system comes from studies in non-pregnant women and should be extrapolated with caution to infer host-vaginal microbiota interactions in pregnancy. Key regulators of this interaction are sex hormones, which regulate the release of pro-inflammatory cytokines, chemokines and antimicrobial peptides, and contribute to the selection of vaginal microbial species [as reviewed in (86)]. In particular, estradiol has been implicated in the shift from a Lactobacillus-poor to a Lactobacillus-rich vaginal microbiota during puberty, as well as a reverse shift after menopause (86). Estrogen-induced glycogen synthesis in epithelial cells and production of glycogen-metabolites (maltose, maltotriose, α-dextrines) provides substrates for conversion to lactic acid by Lactobacilli (87–89). At reproductive age, a healthy vaginal microbiota was found to amplify the fluctuation in local immune responses in synchrony with hormonal changes during the menstrual cycle (90). In particular, women with a Lactobacillus-poor vaginal microbiota altered hormone-associated immune change may correlate with an increase susceptibility to infections (90). The close interplay between immune status and vaginal microbiota composition is further supported by the correlation of a less beneficial vaginal immune signature with ongoing HIV infection status in post-menopausal women (91).
As an integral part of the defense mechanisms of the vaginal space, the local microbiota is required to interact with the host immune system. The ability of the host to protect against pathogenic microorganisms, but not react against the symbiotic microbes residing in the vagina, relies on the bi-directional relationship between immune system and microbiota (92). Such interplay helps maintain an immune-tolerant environment, more so during pregnancy. As a result of this symbiotic tolerance, bacterial communities thrive in the vaginal environment, contribute to local immune defense. Conversely, dysbiosis of the vaginal communities has been implicated in the disruption of the mucosal layer, decreasing the ability of the mucus and vaginal secretions to trap and inactivate pathogens. This might also facilitate the formation of epithelial entry portals for the same pathogens (93).
A possible mechanism for vaginal dysbiosis is the increased production of pro-inflammatory cytokines and chemokines, associated with the increase in pathogenic microbial diversity, which contributes to further recruitment of immune cells and amplification of the inflammatory response [reviewed in (86)]. Clinical studies performed on vaginal samples from sub-Saharan Africa, have shown a correlation between the presence of a non-Lactobacillus dominant microbiota and a rise in inflammatory cytokines and chemokines in the vagina (94, 95). Selected non-beneficial bacteria found in the vaginal tract induce pro-inflammatory cytokines and chemokines in in vitro co-cultures with vaginal epithelial cells. Relevantly, L. crispatus, the most well-known beneficial vaginal microorganism, does not induce inflammatory cytokine release in such settings [reviewed in (82, 96)]. In accordance with this, another study had shown how the possible contribution of vaginal dysbiosis to infections of the urinary tract was mediated by defects of the immune response (97).
Healthy vaginal microbiota was associated, both in vitro and in vivo, with increased expression (mRNA and protein) of defensins, specific types of vaginal antimicrobial peptides (AMP) that prevent binding of pathogen-specific proteins to human cells. AMP levels were significantly lower in bacterial vaginosis conditions, in vitro and in vivo (98). The expression of other types of antimicrobial peptides, the secretory leukocyte protease inhibitor and the human epididymis protein 4, correlates with the presence of less beneficial vaginal microbes (96). The complement system was proposed as a key player in adverse pregnancy outcome, as female microbiota composition regulates complement function in the maternal vasculature (99). Complement dysregulation in the intrauterine space, promotes inflammation and triggers a cascade of physiological changes (cervical changes, degradation of collagen, uterine decidua activation and uterine contractility), which in turn increases risk for preterm delivery (97).
Such combined evidence suggests that the vaginal microbiota modulates the local immune system and inflammatory response at least in part through interaction with epithelial cells, thereby influencing the susceptibility to infection. Although interactions between the maternal immune system and vaginal microbiota appear to be complex and far from completely understood, based on the above outlined observations it was suggested that the overall microbiota composition, rather than any individual microbial population, underlies adverse interactions with the host-immune system in the female reproductive system (100).
Successful implantation and subsequent formation of the placenta (placentation) encompass several steps ensuring tissue adhesion between fetal trophoblasts and maternal tissues and the adaptation of their blood vessels and to facilitate nutrient supply (101). These steps involve mechanisms such as angiogenesis (101), decidualization (102) and immune response adaptation (60). This immune adaptation is essential in pregnancy and is required in order to avoid a graft vs. host disease between the semi-allogenic trophoblast and maternal tissues, including immune cells, decidual microbiota and other decidual components such as epithelial and stromal cells and blood vessels. Not surprisingly, this complex interaction is postulated to affect subsequent stages of pregnancy, and is implicated in many pregnancy complications (103).
Published literature points to the existence of a diverse and metabolically active endometrial microbiota and predicts an important physiologically modulatory role of the main function of its host tissue: harboring and nurturing the developing embryo. In healthy women, the presence of commensal bacterial communities in the cycling endometrium mediate physiological responses from various cells at the feto-maternal interface, including epithelial and stromal cells in the endometrium, immune cells and trophoblasts from the implanting embryo. Although the exact molecular nature and extent of these interactions are not fully understood, evidence from in vitro experiments and animal models have provided important insights (13, 55, 104, 105). Based on currently available knowledge, we postulate the following mechanisms:
1) Commensal bacteria interact with endometrial epithelial cells to maintain a healthy physical and antimicrobial barrier against pathogens. The binding of commensal bacteria to epithelial cells triggers the release of various antimicrobial peptides (AMPs) into the uterine cavity, which constitute part of key defense mechanisms of epithelial tissues against a proteolytic enzymes from various pathogens including bacteria, fungi, and some viruses (52, 53). In addition to the production of AMPs, commensal bacteria induce a biochemically neutral and biophysically stable mucus production by endometrial cells and stabilize the adherens junctions and tight junctions (55, 106). The maintenance of an intact and stable epithelial barrier is an integral part of the natural defense strategies in preventing the colonization and penetration protecting the endometrium from opportunistic microbial infections.
2) Commensal bacteria can alter the immune response at the cellular level through numerous components of the innate and adaptive immune system in the endometrium. The key sensors of bacterial presence in tissues are antigen presenting cells (APCs), in the endometrium they are represented by macrophages and dendritic cells (uDCs) (see section “Maternal Immune Response in Pregnancy”). Both cell types play an important role in maintaining tolerance against the commensal microbiota by modulating the immune response of other components of the innate and adaptive systems (63, 107). Macrophage-derived IL-10 is critical for Foxp3 + Treg cell development, maintenance, and expansion (77). uDCs also are a major source of IL-23 which, in combination with other cytokines, influences the differentiation of Th17 (77). Although both macrophages and DCs can process and present bacterial antigens, differences in their physiology and function suggest they have complementary roles in the immune response against bacteria.
3) The downstream effects of triggering the immune system at the feto-maternal interface by bacteria is the activation of uterine NK (uNK) cells and the development of specific subsets of T-cells, characterized by high number of regulatory T (Treg) cells, low number of Th-17, and a switch from the Th1 to Th2 cytokine production. These adaptive changes ensure an immunotolerant milieu for the semi-allogenic fetus and are essential steps in normal placentation. The interaction of activated uNK cells via specific receptors (KIR) with HLA-C and -G from extravillous trophoblasts (EVT) from the implanting embryo will also promote EVT invasion, stromal matrix degradation, angiogenesis and ultimately the remodeling of maternal spiral arteries.
How the host-microbiota interactions affect the maternal immune response during implantation is not clearly understood. One of the factors, which govern immune modulation and maternal tolerance is the Pre-Implantation Factor (PIF) (108). PIF is a 9–15 amino acid peptide secreted by viable placenta with high concentrations in the maternal circulation in the first trimester (108, 109). PIF shows local effects on the endometrium and trophoblast promoting implantation and invasion of the trophoblast and has a direct impact on immune cell function and targets neutrophils and macrophages, as well as CD4+ and CD8+ T-cells (109). PIF reduces the activation of the NALP3 inflammasome complex (mainly TLR-4 mediated) resulting in reduction of pro-inflammatory cytokines such as IL-1β, IL-18, and IL-33 (110, 111). In addition, PIF creates an anti-inflammatory milieu by reducing IFNγ and stimulating IL-10 secretion, as well as enhancing Th2 cytokines. In context of the macrophages responding to bacterial stimuli, PIF blocks the release of nitric oxide induced by lipopolysaccharides (LPS) (108). This effect is present in case of excessive stimulus only. Thus, PIF operates as an immune modulator, rather than an immune suppressor with minimal impact on the innate, but firm effect on the adaptive immune response (108). Additional protective effects on the embryo protection and development include targeting the protein-disulfide isomerase (PDI) and heat shock proteins (HSP), which impact oxidative stress and protein misfolding (112, 113). Overall PIF is an example of embryonal factor shaping maternal immune response and therefore promoting successful implantation by generating an anti-inflammatory milieu and facilitates immune tolerance. The interaction of microbiome and PIF on pregnancy complications are currently under investigation.
Abnormal composition and/or function of the of reproductive tract dysbiosis is implicated in various gynecological disorders and pregnancy complications (4, 114, 115) (Figure 2). Although many gynecological disorders have been linked to dysbiosis and can indirectly affect reproductive outcomes [e.g., endometriosis and ectopic pregnancy (55)], discussion of these specific complications is outside the scope of this review. Depending on the anatomical site within the female reproductive tract, dysbiosis is associated with various clinical disorders The clinical implications of dysbiosis of the female reproductive tract in relation to pregnancy ranging from infections: BV, bacterial vaginosis; PID, pelvic inflammatory disease; STI’s, sexually transmitted infections; early pregnancy complications: RM, recurrent miscarriage; RIF, recurrent implantation failure; and late pregnancy complications: pPROM, premature pre-labor rupture of membranes and placental dysfunction.
Figure 2. The conceptual relationship of reproductive tract microbiome and immune response. A multitude of environmental and constitutional factors affect the balance between tolerance to the fetus and commensal bacteria on one hand, and the immune response to pathogens and abnormal cells on the other hand. Loss of balance between the microbiome and immune responses would lead to either abnormal commensal microbiome and pathogenic infection “dysbiosis” or excessive immune reaction and sterile inflammation “dysregulated immunity.”
As outlined in preceding sections, the immune system and microbiota play an interactive and collaborative role in maintaining a physiological healthy state in the reproductive tract. Disturbance of this physiological interaction has been implicated in the onset of diverse complications related to female reproductive health.
One of the largest longitudinal cohort studies that assessed vaginal microbiota vs. risk of STI is the Longitudinal Study of Vaginal Flora (LSVF) based in the United States (116). Women diagnosed with BV (by Nugent score assessment) had a nearly 2-fold increased risk of STI, like trichomonal, gonococcal, and/or chlamydial infection (of note: microbiota-testing preceded detection of STI by 3 months). This evidence is in agreement with previous reports that define vaginal dysbiosis as a predictor for gonorrhea and chlamydial infection (117). More recently, individuals with chlamydial infection were found more likely to have a cervicovaginal microbiota dominated by L. iners, or non-L. crispatus anaerobic bacteria (118), although not all associations were statistically significant and may depend on ethnicity (119, 120).
Human Papilloma Virus (HPV) and the Human Immunodeficiency Virus (HIV) represent sexually transmitted viral infections increasingly studied in association with reproductive tract microbiota. Women with detected or persistent HPV infections showed a more diverse vaginal microbiota, in studies conducted among African/Caribbean and Italian women and suggested an association with Atopobium spp. and G. vaginalis (121, 122). Among Nigerian women, the prevalent high-risk HPV (hrHPV) infection was associated with a decrease in Lactobacilli and abundance of anaerobes, particularly of the general Prevotella and Leptotrichia (123). In Asia an association between increased vaginal bacterial diversity and presence of HPV were reported, with a Korean study suggesting Fusobacteria, in particular Sneathia spp. to be particularly implicated (124). A Chinese study identified several microbial genera in hrHPV-infected women (Bifidobacterium, Bacillus, Megasphaera, Sneathia, Prevotella, Gardnerella, Fastidiosipila, and Dialister), while another set (Bifidobacterium, Megasphaera, Bacillus, Acidovorax, Oceanobacillus, and Lactococcus) in hrHPV-infected pregnant women. In pregnancy, this study showed an association between a more diverse cervical microbiota and HPV (125). In addition, several genera and species were associated to HPV positivity (Ureaplasma parvum), HPV negativity (Brochothrix, Diplorickettsia, Ezakiella, Faecalibacterium, and Fusobacterium), likelihood of reinfection (Actinomyces) or persistence (Prevotella, Dialister, and Lachnospiraceae) (126). Recent data also suggested altered microbiota in placenta, cervix and mouth in the presence of HPV infection (119).
Two decades ago, the absence of Lactobacilli was already associated with an increased risk of acquiring HIV infection in a cohort of Kenyan women (120). Similarly, vaginal dysbiosis was suggested as a contributor to the acquisition of HIV in Ugandan and Zimbabwean women (127). Conversely, Rwandan women with a Lactobacillus-dominated (particularly L. crispatus) cervicovaginal microbiota were less likely to be infected with HIV, hrHPV, as well as Herpes Simplex Virus 2 (HRV 2) (128). Low pH, due to lactic acid production by Lactobacilli, was suggested as a main strategy to prevent HIV infection, either by means of inactivating the virus, or inactivating T lymphocytes, thus decreasing their susceptibility to HIV infection (129). Other possible defense mechanisms of vaginal microbiota to HIV include the production of peroxide or bacteriocins by Lactobacilli, although their effect on viral biology is not fully understood (129). These findings are seemingly congruous with the protective role of Lactobacilli in urinary tract infections [as reviewed in (130)].
The above mentioned sexually transmitted infections, among others, have been extensively implicated in diverse reproductive tract complications, from infertility, to adverse pregnancy outcomes. A growing body of evidence collectively supports the association between vaginal dysbiosis and different genital tract infections and corroborates an important physiological role for microbiota in protection from, or susceptibility to pathogenic infections. Interaction and cross-regulation between the vaginal microbiota and the immune responses in the lower genital tract are therefore crucial in creating a protective environment against external pathogens, thus ensuring the right condition for the initiation of pregnancy.
In recent years, the availability of culture-independent sequence techniques has led to a rise in the number of studies investigating the association of disturbed vaginal and endometrial microbiome composition and reproductive failure, focusing mainly on implantation failure. In women undergoing in vitro fertilization (IVF), the percentage of vaginal and endometrial Lactobacilli were significantly lower than non-IVF patients and healthy volunteers (131). In addition, studies have shown that presence of various bacterial contaminants, such as Enterobacteriaceae, Streptococcus, Staphylococcus, and Gram-negative bacteria, from catheter tips at the time of embryo transfer had a negative impact on pregnancy outcome, as reviewed in (13). Using 16S ribosomal RNA sequencing of paired endometrial and vaginal samples from 13 fertile women and 35 infertile patients undergoing IVF, Moreno et al. showed that the presence of a non-Lactobacillus-dominated microbiota (defined as <90% Lactobacillus spp.) was associated with significant decreases in implantation (60% vs. 23%), pregnancy (70% vs. 33%), ongoing pregnancy (59% vs. 13%) and live birth (59% vs. 7%) rates (43). Using a similar approach in a cohort of 31 women, Bernabeu et al. showed that women achieving pregnancy after IVF (cryotransfer of a single embryo) showed a greater presence of Lactobacillus spp., while a trend toward higher alpha diversity in vaginal samples was found in patients who did not achieve pregnancy and no difference in beta diversity (132). In a recent large prospective cohort study, Koedooder et al., used a new technique “IS-PRO” (see section “Diagnostic Challenges”) to examine microbial profiles of vaginal microbiota in 192 women undergoing IVF. Women with a low percentage of Lactobacillus in their vaginal sample were less likely to have a successful embryo implantation (133). This failure was correctly predicted in 32 out of 34 women based on the vaginal microbiota composition, resulting in a predictive accuracy of 94% (sensitivity, 26%; specificity, 97%). Additionally, the degree of dominance of Lactobacillus crispatus was an important factor in predicting pregnancy: none of the women who had a negative prediction (low chance of pregnancy) became pregnant. Taken together, these data suggest that a balanced, less diverse vaginal microbiota, dominated by Lactobacillus species increased the chances of a successful outcome.
In women with recurrent reproductive failure, ascribing a causative or correlative connection to aberrant microbiota is controversial. This group is composed of women with recurrent miscarriage (RM) (defined as loss of two or more clinically or biochemically established pregnancies) (134) and women with recurrent implantation failure (RIF), defined as loss of two or more pregnancy losses after transfer of good-quality embryos (135). RM and RIF both have heterogeneous etiology, with diverse risk factors being implicated covering genetic, metabolic, hormonal, immune maternal aspects. Although several groups have studied microbiota disturbances in women with recurrent reproductive failure, the complex pathogenesis has hampered any meaningful conclusion on the role of reproductive tract dysbiosis in this early pregnancy disorder. Analysis of endometrial samples from women investigated for recurrent reproductive failure showed that the uterine microbiota was dominated by Bacteroides species in >90% of the women (35). However, dissimilarities in dominance of Prevotella spp. or L. crispatus due to possible contamination from the vagina limits the interpretation of these data.
Chronic endometritis (CE) is an inflammatory condition typified by dysregulated interactions between endometrial pathogens and the endometrium. Chronic endometritis is a persistent inflammation of the endometrium, characterized by the presence of plasma cells syndecan-1 (CD138) on immunohistochemical staining of endometrial biopsies [reviewed in (136)]. Although various pathogens have been implicated in causing CE, the most commonly reported species were common bacteria (Escherichia coli, Enterococcus faecalis, and Streptococcus agalactiae) in 77.5%, followed by Mycoplasma/Ureaplasma (25%) and Chlamydia (13%) (137).
Recently, research has focused on the role of CE in reproductive failure, with various studies reporting a wide range prevalence depending on the clinical characteristics of the studied group and reflecting heterogeneity of diagnostic methodology and definitions. Studies have found an increased prevalence of CE in women with recurrent pregnancy loss (13%) (138) and RIF (30%) (139), while the rate of CE in the general infertility population was suggested to be much lower, with a prevalence of 2.8% among 606 infertility patients (140). A recent meta-analysis of five studies (total of 796 patients) concluded that women receiving antibiotic therapy for CE did not show any reproductive advantage in comparison with untreated controls (141). However, patients with cured CE, confirmed by a repeat biopsy, showed higher clinical pregnancy rate (OR 4), ongoing pregnancy rate/live birth rate (OR 6.8) and implantation rate (OR 3.2) (141). The exact mechanism of how CE affects implantation is yet unknown, but a negative effect on endometrial receptivity by abnormal infiltration of plasma cells (B lymphocytes) and antibody production is suggested (142). Similarly, as the causal connection between CE and dysbiosis is unknown, it is unclear whether a status of dysregulated immune response in the endometrium is responsible for the higher prevalence of dysbiosis, or whether dysbiosis is the cause of CE.
How the disturbance of the endometrial microbial ecosystem can have a negative impact implantation process is not fully understood. Parallel to the proposed mechanism of interaction between the microbiota and endometrial cells, a disturbed balance can act upon the following mechanisms (Figure 3). (1) First, the dominance of non-commensal bacterial communities could weaken the integrity of the endometrial mucosal barrier by affecting the epithelial tight junctions and reducing AMP and mucin secretion; (2) This in turn could further weaken host defense mechanisms and allow pathogens to enter the endometrial stroma and elicit a profound immune reaction from APC and other immune cells harboring pattern recognition receptors (PRR); (3) Aberrant stimulation of T-cells, either directly from invading pathogens breaching the mucosal barrier, or indirectly from absorbed bacterial products, results in disbalance in cytokine production in favor of the pro-inflammatory Th-1 types, predominated by TNF-a, IFN, IL-2, and IL-10. Abnormal uNK cell maturation, either primary or secondary to shallow extravillous trophoblast (EVT) invasion, is a possible link between a disturbed endometrial microbiome and incomplete remodeling of maternal spiral arteries, characteristic of the great obstetric syndromes (143).
Figure 3. The clinical implications of dysbiosis (abnormal microbiota) of the female reproductive tract in relation to pregnancy. Some disorders, e.g., endometriosis are indirectly related to pregnancy disorders through their negative impact on fertility. BV, bacterial vaginosis; PID, pelvic inflammatory disease; PPROM, premature prelabor rupture of membranes; RM, recurrent miscarriage; RIF, recurrent implantation failure; STI’s, sexually transmitted infections.
Alteration of the ecology of the female reproductive tract has been linked to maternal and fetal health, and to adverse pregnancy outcomes (27, 79, 144, 145). The most widely studied pregnancy complication in relation to the vaginal microbiota is preterm birth (PTB) (146–148). The prevalence of a Lactobacillus-poor microbiota was inversely correlated with gestational age at delivery in some studies (38), not in others (149). Distinctive species of Lactobacilli were reported to be associated with differential pregnancy outcomes. Indeed, in two ethnically distinct cohorts, L. crispatus was associated with a low PTB risk, whereas L. iners was not (42). Also, Gardnerella was also associated with PTB and coexisted with L. iners, but not with L. crispatus (42). Based on these findings, a model was suggested to help describe the interplay between key vaginal bacterial species: the presence of G. vaginalis correlates with adverse outcomes, such as PTB and symptomatic Bacterial Vaginosis (BV); G. vaginalis and L. crispatus are strongly mutually exclusive, while this is not the case for L. iners and Gardnerella (150). Comparison of vaginal samples of 90 women who delivered at term and 45 women with preterm birth suggested that a specific signature: the presence of BV-associated bacterium (BVAB) 1, Prevotella cluster 2, Sneathia amnii and BVAB-TM7 in early pregnancy, may be useful for prediction of PTB risk, particularly in high-risk populations of African ancestry (147).
Abnormal vaginal colonization in the second trimester was also associated with an increased PTB risk (151). Similarly, in a predominantly African-American population, an increased vaginal microbial community richness and diversity between the first and second trimester was associated with PTB (152). In addition to decreased Lactobacillus spp., specific pathogens have been linked to PTB in different populations, such as Klebsiella pneumonia, Gardnerella, Ureaplasma and other genera, including Prevotella, Atopobium, Sneathia, Gemella, Megasphaera, Dorea, Streptococcus, and Escherichia/Shigella (38).
At this point, although one out of four preterm births appears to be associated with intra-amniotic infection, and some associations between microbial states or abundance of individual species with PTB have been reported, it does not appear clear whether changes in the bacterial communities of the lower genital tract allow for a clear identification of women at risk (144). A recent overview summarizing studies on the association between vaginal microbiota and PTB emphasized the methodological heterogeneity, and scarcity of, studies in the field (146). Overall, research on the topic has produced conflicting outcome, often related to ethnical background of the women included and the associated risk degree of PTB. Nonetheless, more recent studies more consistently report an association between vaginal dysbiosis and PTB, possibly resulting from the improved understanding of the contribution of L. iners to this association (146).
Multiple studies provide evidence of placental and amniotic fluid microorganisms affecting miscarriage, chorioamnionitis, premature rupture of membranes (PROM), stillbirth, preeclampsia (PE), and intra uterine growth restriction (IUGR) rates (143, 153). In some cases of spontaneous preterm delivery, microorganisms have been found to invade the amniotic cavity, leading to increased maternal/neonatal morbidity and mortality (154). Infectious bacteria gain access, typically via ascending route and/or perturbations of the vaginal microbiota and can have an adverse impact on pregnancy outcomes (146). Chorioamnionitis is an inflammatory disease of the extraembryonic membranes, placenta and amniotic fluid due to microbial invasion mostly commonly Ureaplasma and Mycoplasma spp. infections (155). Culture-dependent studies identified members of the genera Prevotella, Bacteroides, Peptostreptococcus, Gardnerella, Mobiluncus and genital mycoplasmas in the placentae of women delivering preterm with or without PE, suggestion the involvement of multiple bacterial strains (15). In line with this, antibiotic treatments have not reduced the rates of preterm birth, suggesting that a single inflammatory/infectious pathway may not fully explain the problem (144). In contrast, DNA-based investigations of the placental microbiota in PTB showed increased enrichment of Burkholderia spp. and an increased relative abundance of Alphaprotoebacteria and Actinomycetales and mixed non-cultivable anaerobes (15). However, in case of chorioamnionitis a higher abundance of Streptococcus agalactiae, Fusobacterium nucleatum and Ureaplasma parvum was reported (15). These results fuel the debate whether a prenatal bacterial microbiota really exist (45). In physiological pregnancies or in the presence and absence of active labor, many “causal” microorganisms or their DNA are detectable in the placenta and amniotic fluid (156, 157). Since microbiotal ratios of specific microbial species change throughout gestation, the placental response to such environmental cues possibly does as well (158). This is supported by the observation that PIF is differently expressed in the placenta throughout gestation or in response to an inflammatory insult (109). Since, as of yet, the amniotic fluid and the membranes are not available for non-invasive sampling, the time of onset of chorioamnionitis is not available for clinical stratification and decision making. Besides placental inflammation, systemic inflammation in concert with oxidative stress and endothelial dysfunction plays a role in pregnancy complications such as IUGR or PE (159).
The interaction of vaginal and endometrial microbiota with local maternal components modulates the maternal immune system. Although the contribution of the fetal immune system to this interplay is currently unknown, it is conceivable that when the fetus initiates an inflammatory response, premature labor may impose a risk to fetal wellbeing. Conversely, there is an inherent risk that the fetus may be injured by a longer stay in utero either directly through microbial toxins or through proinflammatory cytokines. This delicate balance between maternal and fetal needs possibly dictates the course and outcome of pregnancy and may contribute to long-term health of the offspring. This may be another example how future health is primed by the intrauterine environment according to the principles of the DOHAD (Developmental Origins of Health and Disease) hypothesis (160).
As discussed throughout this review, one of the major limitations in microbiome research is the diversity of the techniques used and the databases linked to those techniques to identify individual species and determine the composition of microbiota. Such differences potentially introduce significant variations in analysis and constitute a major source of difference in interpretation of data. Although currently no compelling evidence points to the existence of a universal mammalian placental or fetal microbiota, consensus on the importance of uniform technological and analytical approaches warrants further investigation into standardization of microbiota research and incorporating geographical, ethnic and societal data (45) (Table 1). These recommendations are general for all microbial community profiles, including the female reproductive tract microbiota, and are expected to reduce variation and inconsistency between studies on microbiomes.
Moreover, most of the techniques described have not undergone rigorous validation steps and certification by regulatory organs, such as the European commission in vitro diagnostic (CE-IVD) label or the American Food and Drug Administration (FDA) approval, limiting their commercial availability. The availability of CE-IVD-certified microbiome analysis tools would meet part of the recommendations (see: Table 1) and allow more reliable comparisons between studies in different countries and settings. Besides the widespread Next-Generation Sequencing (NGS) approaches, our group developed another detection technique called IS-pro, first described in 2009 (161). The IS-pro test is already CE-IVD certified and is currently being used in many different disease profiling activities and applications including analyses of the vaginal microbiome as a predictor for outcome of in vitro fertilization (133). A wider application of this technique to study the role of reproductive tract microbiome in various pregnancy complications is expected to be available in the near future.
The general concept of interactions between commensal microbiota and human cells has recently been embraced as a principal of human physiology. The realization that a dysbalanced and/or harmful microbiotal composition frequently correlates with specific clinical conditions, has led to a sharp rise in studies on host-microbiota interaction over the last decade (25, 55, 114, 162, 163). Although it is becoming increasingly clear that the interplay between host and microbiota also affects human reproductive biology, the exact molecular mechanisms underpinning these interactions are far from understood.
All long-lasting physiological adaptations of cells and tissues in response to altered environmental conditions have their basis in altered epigenomic programming. Environmental changes are detected by a myriad of cellular sensing mechanisms and, via signal transduction routes, ultimately reach the nucleus where environmental cues are translated to epigenetic regulation and chromatin remodeling. This epigenetic regulation of genetic input and potential environmental cues underlies maternal physiological plasticity and embryonal development during gestation. Programming of immune cells during immunological responses is mediated by cellular stress responses and is typically accompanied by metabolic changes in the cell. Prolonged disturbance of these interactions is associated with the development of immunological disorders, such as chronic inflammatory conditions (164). The close link between cellular metabolism and epigenetic responses has its origin in early evolution, has enabled multicellularity and is closely connected to organismal survival (165). Hence, fine-tuning of epigenetic control occurs in conjunction with cellular metabolic status, as available energy ultimately directs and limits cell responses. Recent advances in this field have identified oxygen, numerous metabolic intermediates, cellular reduction (NADH, FADH2) and energy equivalents (ATP, GTP), as direct molecular effectors of epigenetic regulatory activity (165). Conversely, cellular metabolic adaptation is controlled by epigenetic regulation, substantiating the reciprocal nature of the physiological interaction between metabolism and epigenetics (166). Sex hormones, metabolic profiles, nutrition, maternal stress, drugs, smoking and air pollution represent obvious examples of environmental cues that, via active modulation of epigenetic regulatory mechanism.
Interestingly, microbial metabolites, among which Short-Chain Fatty Acids (SCFA), like acetate, butyrate and propionate, are known to harbor the ability to alter epigenetic status of numerous cell types; studied examples thereof include the effect of such compounds on immune cells (78). In the context of human reproduction, it is conceivable that microbial metabolites, directly (e.g., via SCFA) or indirectly (e.g., acidification, alkalization, inflammatory responses), can either support (healthy microbiota) or upset (unbalanced/harmful microbiota) local cell-cell communication and tissue physiology and adaptation. As such, all relevant reproductive processes, including fertilization, implantation, placentation, immune tolerance, embryonal development, infant and adult health and may be harmfully altered by dysbiosis (167). Such gene-environment interactions are yet to be examined in detail in the context of reproductive health and disease.
Given the association between pregnancy complications and microbiota, the question of modulation strategies is valid. Postnatal dietary strategies, including human colostrum/milk or prebiotics/probiotics, reduce morbidities in preterm infants (163). Evidence of prenatal strategies to support reproductive success and fetal health is slowly emerging: modulation of early microbiota in pregnancy shows promising effects (168). Maternal bacteria were shown to enter the gastrointestinal tract of the fetus (169) and microbiota alteration in the neonate and placenta is detectable in pregnant women receiving probiotics (170) but still under debate (14).
Recently our group published a meta-analysis on the relation between vaginal microbiota and early pregnancy development after IVF and the effect of probiotics thereon (71). It provides an overview of published studies describing long term modulation of the vaginal microbiome using Lactobacillus-based probiotics. Patients were often treated by Metronidazole, and followed up with a Lactobacillus-based probiotic. Besides lactobacillus-based probiotics, mixtures of Lactobacillus, Bifidobacterium and Streptococcus strains were also used in different studies (71). Further studies assessing the potential to modulate pregnancy outcomes are needed.
The notion that the neonatal immune system can be shaped by early fetal microbial colonization by inference implies that reciprocal interactions between the host and microbiota exist (171). This hypothesis is in line with the evidence that factors like PIF mediate maternal immune tolerance during pregnancy (108). Fine tuning of immune modulation is not only relevant for embryo implantation but also for defense against potentially harmful microbes. An exaggerated maternal immune response is putatively linked to preterm birth and fetal loss (172). Therefore, a novel strategy could be the maternal immune system modulation by synthetic PIF (110). Inflammatory challenge during pregnancy results in endogenous PIF expression and additional administration of synthetic PIF could prevent fetal loss.
The neonatal microbiota, mainly that of the gut, skin and oral cavity, has long been postulated to be acquired postnatally known as “postnatal microbiota seeding”. Alternatively, the hypothesis of perinatal microbiota transfer and its potential relevance to infant and adult general health is gaining attention. Given the importance of transfer of maternal microbiota to the child via colonization, the significance of birth mode is of high interest (173). Vaginal birth exposes the baby to maternal vaginal and intestinal microbiota, whereas cesarean section limits exposure of the newborn to parental dermal microbiota and any microbes present in the surgical theater. The “prophylactic” antibiotic treatment of the mother during labor or cesarean section may aggravate any effect of non-natural birth on colonization and may also negatively affect intestinal microbiota of the offspring (174). Hence, birth mode may have long-lasting effects on the composition of the newborn gut microbiome, and predispose for adverse health outcomes (174). The notion that cesarean delivery deprives the infant of exposure to vaginal microbiota and consequently leads to neonatal dysbiosis has led to the popularized, yet unsubstantiated and potentially hazardous practice of “vaginal seeding”. In a pilot study of 18 mother-infant pairs, there was partial restoration of microbiome (mainly of the skin and oral cavity and less so of the gut) in infants exposed to vaginal fluids from a vaginally placed gauze after cesarean delivery (n = 4), compared to non-exposed infants (n = 7), and resembling the microbiome of vaginally delivered infants (n = 7) (175). This widely cited trial was criticized for the small sample size and the potential bias from confounders such as intrapartum antibiotic prophylaxis and maternal BMI (173, 174). Although postnatal vaginal seeding altered newborn intestinal microbiota composition over several months, this intervention is believed to introduce an inherent risk of transferring pathogenic microbes (e.g., HPV, GBS) onto the newborn (176). For this reason, perinatal seeding is currently not encouraged as standard practice by the American College of Gynecology and Obstetrics (177). Whether and how vaginal seeding has any long-term beneficial health effects for the offspring requires large properly conducted studies with robust microbiome analysis.
As microbiota represents an environmental factor which affects host-microbe interactions at the epigenetic level, detailed understanding of the molecular workings of the close functional interplay between host cell systems and microbiota holds the promise that therapeutic intervention strategies can be designed for the benefit of general and reproductive health. These include the use of probiotics, beneficial microbial metabolites, rational diets and/or (ant)agonists of specific biological response pathways (162). Combined the research cited in his review define opportunities for modulation of the female reproductive tract microbiota, while harnessing its protective and immuno-regulating role during pregnancy. Such opportunities should take into consideration individual differences in microbial communities, and tailor therapeutics to different anatomical and gestational factors in an attempt to provide precision tools for reproductive health.
The composition and interaction of the female reproductive tract microbiome with the host not only shape the mothers’ physiology and health during pregnancy, but also that of the fetus in accordance with the developmental origins of health and disease principles. Scientific knowledge on and insight into the properties and workings of human microbiota has increased over the last decade. However, the definition and possible implications of beneficial versus harmful microbes in physiological and pathological pregnancies is just beginning to emerge. A growing body of evidence associates stability of reproductive tract microbiota to reproductive health and maternal-fetal status during gestation, in which the interplay between microbiota and the maternal immune response takes up a prominent position. The important question of whether and how reproductive tract microbiota can be modified during and beyond pregnancy is under debate and awaits solid confirmation. The challenge for future research is to deliver standardized and validated reference methods for comparative analysis and interpretation of reproductive tract microbiomes, in order to understand their role in various clinical disorders and test the implementation of individualized therapies in large prospective trials.
SA-N and EA designed and wrote the manuscript and illustrations. LW, MSc, MSp, and JV wrote the sections of the manuscript and contributed to the interpretation of the results. SM, MM, and BK conceived the original idea, supervised the project, and edited the manuscript. All authors provided the critical feedback and contributed to the final version of the manuscript.
The authors declare that the research was conducted in the absence of any commercial or financial relationships that could be construed as a potential conflict of interest.
The reviewer, SS, declared a past co-authorship, with one of the authors, SM, to the handling editor.
The authors would like to thank Mrs. Laila Alghifary for making the illustrations used in this manuscript.
1. Human Microbiome Project Consortium.Structure, function and diversity of the healthy human microbiome. Nature. (2012) 486:207–14. doi: 10.1038/nature11234
2. NIH Human Microbiome Portfolio Analysis Team. A review of 10 years of human microbiome research activities at the US national institutes of health, fiscal years 2007-2016. Microbiome. (2019) 7:31. doi: 10.1186/s40168-019-0620-y
3. Sirota I, Zarek S, Segars J. Potential influence of the microbiome on infertility and assisted reproductive technology. Semin Reprod Med. (2014) 32:35–42. doi: 10.1055/s-0033-1361821
4. Power ML, Quaglieri C, Schulkin J. Reproductive microbiomes: a new thread in the microbial network. Reproduct Sci. (2017) 24:1482–92. doi: 10.1177/1933719117698577
5. Younes JA, Lievens E, Hummelen R, van der Westen R, Reid G, Petrova MI. Women and their microbes: the unexpected friendship. Trends Microbiol. (2018) 26:16–32. doi: 10.1016/j.tim.2017.07.008
6. Romero R. Prenatal medicine: the child is the father of the man∗. J Matern Fetal Neonatal Med. (2009) 22:636–9. doi: 10.1080/14767050902784171
7. Mascarenhas MN, Flaxman SR, Boerma T, Vanderpoel S, Stevens GA. National, regional, and global trends in infertility prevalence since 1990: a systematic analysis of 277 health surveys. PLoS Med. (2012) 9:e1001356. doi: 10.1371/journal.pmed.1001356
8. Rossen LM, Ahrens KA, Branum AM. Trends in risk of pregnancy loss among US women, 1990-2011. Paediatr Perina Epidemiol. (2017) 32:19–29. doi: 10.1111/ppe.12417
9. Dhaded SM, Somannavar MS, Jacob JP, McClure EM, Vernekar SS, Yogesh Kumar S, et al. Early pregnancy loss in Belagavi, Karnataka, India 2014–2017: a prospective population-based observational study in a low-resource setting. Reprod Health. (2018) 15:2683–8. doi: 10.1186/s12978-018-0525-4
10. Dellicour S, Aol G, Ouma P, Yan N, Bigogo G, Hamel MJ, et al. Weekly miscarriage rates in a community-based prospective cohort study in rural western Kenya. BMJ Open. (2016) 6:e011088. doi: 10.1136/bmjopen-2016-011088
11. Lozano R, Wang H, Foreman KJ, Rajaratnam JK, Naghavi M, Marcus JR, et al. Progress towards millennium development goals 4 and 5 on maternal and child mortality: an updated systematic analysis. Lancet. (2011) 378:1139–65. doi: 10.1016/S0140-6736(11)61337-8
12. Chawanpaiboon S, Vogel JP, Moller A-B Lumbiganon P, Petzold M, Hogan D, et al. Global, regional, and national estimates of levels of preterm birth in 2014: a systematic review and modelling analysis. Lancet Glob Health. (2019) 7:e37–46. doi: 10.1016/S2214-109X(18)30451-0
13. Franasiak JM, Scott RT. Endometrial microbiome. Curr Opin Obstet Gynecol. (2017) 29:146–52. doi: 10.1097/GCO.0000000000000357
14. de Goffau MC, Lager S, Sovio U, Gaccioli F, Cook E, Peacock SJ, et al. Human placenta has no microbiome but can contain potential pathogens. Nature. (2019) 572:1–21. doi: 10.1038/s41586-019-1451-5
15. Pelzer E, Gomez-Arango LF, Barrett HL, Nitert MD. Review: maternal health and the placental microbiome. Placenta. (2017) 54:30–7. doi: 10.1016/j.placenta.2016.12.003
16. Redelinghuys MJ, Ehlers MM, Dreyer AW, Kock MM. Normal flora and bacterial vaginosis in pregnancy: an overview. Critic Rev Microbiol. (2016) 1:1–12. doi: 10.3109/1040841X.2014.954522
17. Gajer P, Brotman RM, Bai G, Sakamoto J, Schütte UME, Zhong X, et al. Temporal dynamics of the human vaginal microbiota. Sci Transl Med. (2012) 4:ra52–132. doi: 10.1126/scitranslmed.3003605
18. Hickey RJ, Zhou X, Pierson JD, Ravel J, Forney LJ. Understanding vaginal microbiome complexity from an ecological perspective. Trans Res. (2012) 160:267–82. doi: 10.1016/j.trsl.2012.02.008
19. Gupta VK, Paul S, Dutta C. Geography, ethnicity or subsistence-specific variations in human microbiome composition and diversity. Front Microbiol. (2017) 8:1162. doi: 10.3389/fmicb.2017.01162
20. Noyes N, Cho K-C, Ravel J, Forney LJ, Abdo Z. Associations between sexual habits, menstrual hygiene, practices demographics and the vaginal microbiome as revealed by Bayesian network analysis. PLoS One (2018) 13:e0191625. doi: 10.1371/journal.pone.0191625
21. Jespers V, van de Wijgert J, Cools P, Verhelst R, Verstraelen H, Delany-Moretlwe, S, et al. The significance of Lactobacillus crispatus and L. vaginalis for vaginal health and the negative effect of recent sex: a cross-sectional descriptive study across groups of African women. BMC Infect Dis. (2015) 15:115. doi: 10.1186/s12879-015-0825-z
22. Zhou X, Hansmann MA, Davis CC, Suzuki H, Brown CJ, Schütte U, et al. The vaginal bacterial communities of Japanese women resemble those of women in other racial groups. FEMS Immunol Med Microbiol. (2010) 58:169–81. doi: 10.1111/j.1574-695X.2009.00618.x
23. Bayigga L, Kateete DP, Anderson DJ, Sekikubo M, Nakanjako D. Diversity of vaginal microbiota in sub-Saharan Africa and its effects on HIV transmission and prevention. Am J Obstet Gynecol. (2019) 220:155–66. doi: 10.1016/j.ajog.2018.10.014
24. Borgdorff H, van der Veer C, van Houdt R Alberts CJ, de Vries HJ, Bruisten SM, et al. The association between ethnicity and vaginal microbiota composition in Amsterdam, the Netherlands. PLoS One (2017) 12:e0181135. doi: 10.1371/journal.pone.0181135
25. van de Wijgert JHHM, Jespers V. The global health impact of vaginal dysbiosis. Res Microbiol. (2017) 168:859–64. doi: 10.1016/j.resmic.2017.02.003
26. Kroon SJ, Ravel J, Huston WM. Cervicovaginal microbiota, women’s health, and reproductive outcomes. Fertil Steril. (2018) 110:327–36. doi: 10.1016/j.fertnstert.2018.06.036
27. Parnell LA, Briggs CM, Mysorekar IU. Maternal microbiomes in preterm birth: recent progress and analytical pipelines. Semin Perinatol. (2017) 41:392–400. doi: 10.1053/j.semperi.2017.07.010
28. Anahtar MN, Gootenberg DB, Mitchell CM, Kwon DS. Cervicovaginal microbiota and reproductive health: the virtue of simplicity. Cell Host and Microbe. (2018) 23:159–68. doi: 10.1016/j.chom.2018.01.013
29. Romero R, Hassan S, Gajer P, Tarca AL, Fadrosh DW, Nikita L, et al. The composition and stability of the vaginal microbiota of normal pregnant women is different from that of non-pregnant women. Microbiome (2014) 2:4. doi: 10.1186/2049-2618-2-4
30. Freitas AC, Chaban B, Bocking A, Rocco M, Yang S, Hill JE, et al. The vaginal microbiome of pregnant women is less rich and diverse, with lower prevalence of mollicutes, compared to non-pregnant women. Sci Rep. (2017) 7:9212. doi: 10.1038/s41598-017-07790-9
31. Avershina E, Slangsvold S, Simpson MR, Storrø, O Johnsen, R Øien, T, et al. Diversity of vaginal microbiota increases by the time of labor onset. Sci Rep. (2017) 7:17558. doi: 10.1038/s41598-017-17972-0
32. Walther-António MRS, Jeraldo P, Berg Miller ME Yeoman CJ, Nelson KE, Wilson BA, et al. Pregnancy’s stronghold on the vaginal microbiome. PLoS One (2014) 9:e98514. doi: 10.1371/journal.pone.0098514
33. Nasioudis D, Forney LJ, Schneider GM, Gliniewicz K, France M, Boester A, et al. Influence of pregnancy history on the vaginal microbiome of pregnant women in their first trimester. Sci Rep. (2017) 7:10201. doi: 10.1038/s41598-017-09857-z
34. Aagaard K, Riehle K, Ma J, Segata N, Mistretta T-A, Coarfa C, et al. A metagenomic approach to characterization of the vaginal microbiome signature in pregnancy. PLoS One (2012) 7:e36466. doi: 10.1371/journal.pone.0036466
35. Verstraelen H, Verhelst R, Claeys G, De Backer E, Temmerman M, Vaneechoutte M. Longitudinal analysis of the vaginal microflora in pregnancy suggests that L. crispatus promotes the stability of the normal vaginal microflora and that L. gasseri and/or L. iners are more conducive to the occurrence of abnormal vaginal microflora. BMC Microbiol. (2009) 9:116. doi: 10.1186/1471-2180-9-116
36. Hernández-Rodríguez, C, Romero-González, R, Albani-Campanario M, Figueroa-Damián R, Meraz-Cruz N, and Hernández-Guerrero C. Vaginal microbiota of healthy pregnant mexican women is constituted by four Lactobacillus species and several vaginosis-associated bacteria. Infect Dis Obstetr Gynecol. (2011) 2011:851485. doi: 10.1155/2011/851485
37. Muzny CA, Schwebke JR. Pathogenesis of bacterial vaginosis: discussion of current hypotheses. J Infect Dis. (2016) 214(Suppl. 1):S1–5. doi: 10.1093/infdis/jiw121
38. DiGiulio DB, Callahan BJ, McMurdie PJ, Costello EK, Lyell DJ, Robaczewska A, et al. Temporal and spatial variation of the human microbiota during pregnancy. Proc Natl Acad Sci USA. (2015) 112:11060–5. doi: 10.1073/pnas.1502875112
39. MacIntyre DA, Chandiramani M, Lee YS, Kindinger L, Smith A, Angelopoulos N, et al. The vaginal microbiome during pregnancy and the postpartum period in a European population. Sci Rep. (2015) 5:8988. doi: 10.1038/srep08988
40. Harris JW, Brown JHLR. The bacterial content of the uterus at cesarean section. II. Am J Obstet Gynecol. (1927) 13:133–43.
41. Koedooder R, Mackens S, Budding A, Fares D, Blockeel C, Laven J, et al. Identification and evaluation of the microbiome in the female and male reproductive tracts. Hum Reprod Update. (2019) 25:298–325. doi: 10.1093/humupd/dmy048
42. Mitchell CM, Haick A, Nkwopara E, Garcia R, Rendi M, Agnew K, et al. Colonization of the upper genital tract by vaginal bacterial species in nonpregnant women. Am J Obstet Gynecol. (2015) 212:611.e1–9. doi: 10.1016/j.ajog.2014.11.043
43. Moreno I, Codoñer FM, Vilella F, Valbuena D, Martinez-Blanch JF, Jimenez-Almazán, J, et al. Evidence that the endometrial microbiota has an effect on implantation success or failure. Am J Obstet Gynecol. (2016) 215:684–703. doi: 10.1016/j.ajog.2016.09.075
44. Aagaard K, Ma J, Antony KM, Ganu R, Petrosino J, Versalovic J. The placenta harbors a unique microbiome. Sci Transl Med. (2014) 6:ra65–237. doi: 10.1126/scitranslmed.3008599
45. Hornef M, Penders J. Does a prenatal bacterial microbiota exist? Mucosal Immunol. (2017) 10:598–601. doi: 10.1038/mi.2016.141
46. Bushman FD. De-discovery of the placenta microbiome. Am J Obstet Gynecol. (2019) 220:213–4. doi: 10.1016/j.ajog.2018.11.1093
47. Theis KR, Romero R, Winters AD, Greenberg JM, Gomez-Lopez N, Alhousseini A, et al. Does the human placenta delivered at term have a microbiota? Results of cultivation, quantitative real-time PCR, 16S rRNA gene sequencing, and metagenomics. Am J Obstet Gynecol. (2019) 220:267.e1–39. doi: 10.1016/j.ajog.2018.10.018
48. Vanterpool SF, Been JV, Houben ML, Nikkels PGJ, De Krijger, RR, Zimmermann LJI, et al. Porphyromonas gingivalis within placental villous mesenchyme and umbilical cord stroma is associated with adverse pregnancy outcome. PLoS One. (2016) 11:e0146157. doi: 10.1371/journal.pone.0146157
49. Bulmer JN, Williams PJ, Lash GE. Immune cells in the placental bed. Int J Dev Biol. (2010) 54:281–94. doi: 10.1387/ijdb.082763jb
50. Mor G, Cardenas I, Abrahams V, Guller S. Inflammation and pregnancy: the role of the immune system at the implantation site. Ann N Y Acad Sci. (2011) 1221:80–7. doi: 10.1111/j.1749-6632.2010.05938.x
51. Bonney EA. Immune regulation in pregnancy. Obst Gynecol Clin North Am. (2016) 43:679–98. doi: 10.1016/j.ogc.2016.07.004
52. Frew L, Stock SJ. Antimicrobial peptides and pregnancy. Reproduction. (2011) 141:725–35. doi: 10.1530/REP-10-0537
53. Hancock REW, Rozek A. Role of membranes in the activities of antimicrobial cationic peptides. FEMS Microbiol Lett. (2002) 206:143–9. doi: 10.1111/j.1574-6968.2002.tb11000.x
54. Underwood MA, Gilbert WM, Sherman MP. Amniotic fluid: not just fetal urine anymore. J Perinatol. (2005) 25:341–8. doi: 10.1038/sj.jp.7211290
55. Baker JM, Chase DM, and Herbst-Kralovetz MM. Uterine microbiota: residents tourists, or invaders? Front Immunol. (2018) 9:208. doi: 10.3389/fimmu.2018.00208
56. King AE, Critchley HO, Kelly RW. Presence of secretory leukocyte protease inhibitor in human endometrium and first trimester decidua suggests an antibacterial protective role. Mol Hum Reprod. (2000) 6:191–6. doi: 10.1093/molehr/6.2.191
57. Trundley A, Moffett A. Human uterine leukocytes and pregnancy. Tissue Antigens. (2004) 63:1–12. doi: 10.1111/j.1399-0039.2004.00170.x
58. McIntire RH, Hunt JS. Antigen presenting cells and HLA-G – a review. Placenta. (2005) 26:S104–9. doi: 10.1016/j.placenta.2005.01.006
59. Racicot K, Kwon J-Y, Aldo P, Silasi M, Mor G. Understanding the complexity of the immune system during pregnancy. Am J Reprod Immunol. (2014) 72:107–16. doi: 10.1111/aji.12289
60. Faas MM, de Vos, P. Uterine NK cells and macrophages in pregnancy. Placenta. (2017) 56:44–52. doi: 10.1016/j.placenta.2017.03.001
61. Ashkar AA, Di Santo JP, Croy BA. Interferon gamma contributes to initiation of uterine vascular modification, decidual integrity, and uterine natural killer cell maturation during normal murine pregnancy. J Exp Med. (2000) 192:259–70. doi: 10.1084/jem.192.2.259
62. Boulenouar S, Doisne J-M, Sferruzzi-Perri A, Gaynor LM, Kieckbusch J, Balmas E, et al. The residual innate lymphoid cells in NFIL3-deficient mice support suboptimal maternal adaptations to pregnancy. Front Immunol. (2016) 7:43. doi: 10.3389/fimmu.2016.00043
63. Nagamatsu T, Schust DJ. Review: the immunomodulatory roles of macrophages at the maternal—-fetal interface. Reprod Sci. (2010) 17:209–18. doi: 10.1177/1933719109349962
64. Mor G, Abrahams VM. Reproductive biology and endocrinology. Reprod Biol Endocrinol. (2003) 1:119–8. doi: 10.1186/1477-7827-1-119
65. Faas MM, Spaans F, and De Vos P. Monocytes and macrophages in pregnancy and pre-eclampsia. Front Immunol. (2014) 5:298. doi: 10.3389/fimmu.2014.00298/abstract
66. Swartwout B, Luo XM. Implications of probiotics on the maternal-neonatal interface: gut microbiota, immunomodulation, and autoimmunity. Front Immunol. (2018) 9:2840. doi: 10.3389/fimmu.2018.02840
67. Blois SM, Barrientos G, Garcia MG, Orsal AS, Tometten M, Cordo-Russo RI, et al. Interaction between dendritic cells and natural killer cells during pregnancy in mice. J Mol Med. (2008) 86:837–52. doi: 10.1007/s00109-008-0342-2
68. Kämmerer U, Eggert AO, Kapp M, McLellan AD, Geijtenbeek TBH, Dietl J, et al. Unique appearance of proliferating antigen-presenting cells expressing DC-SIGN (CD209) in the decidua of early human pregnancy. Am J Pathol. (2003) 162:887–96. doi: 10.1016/S0002-9440(10)63884-9
69. Krey G, Frank P, Shaikly V, Barrientos G, Cordo-Russo R, Ringel F, et al. In vivo dendritic cell depletion reduces breeding efficiency, affecting implantation and early placental development in mice. J Mol Med. (2008) 86:999–1011. doi: 10.1007/s00109-008-0379-2
70. Williams PJ, Searle RF, Robson SC, Innes BA, Bulmer JN. Decidual leucocyte populations in early to late gestation normal human pregnancy. J Reprod Immunol. (2009) 82:24–31. doi: 10.1016/j.jri.2009.08.001
71. Singer M, Borg M, Ouburg S, Morré SA. The relation of the vaginal microbiota to early pregnancy development during in vitro fertilization treatment-A meta-analysis. J Gynecol Obstet Hum Reprod. (2019) 48:223–9. doi: 10.1016/j.jogoh.2019.01.007
72. Chaouat G. The Th1/Th2 paradigm: still important in pregnancy? Semin Immunopathol. (2007) 29:95–113. doi: 10.1007/s00281-007-0069-0
73. Tilburgs T, Roelen DL, van der Mast BJ, de Groot-Swings GM, Kleijburg C, Scherjon SA, et al. Evidence for a selective migration of fetus-specific CD4+CD25bright regulatory T cells from the peripheral blood to the decidua in human pregnancy. J Immunol. (2008) 180:5737–45. doi: 10.4049/jimmunol.180.8.5737
74. Saito S, Nakashima A, Shima T, Ito M. Th1/Th2/Th17 and regulatory T-cell paradigm in pregnancy. Am J Reprod Immunol. (2010) 63:601–10. doi: 10.1111/j.1600-0897.2010.00852.x
75. Zenclussen AC, Gerlof K, Zenclussen ML, Ritschel S, Zambon Bertoja, A Fest S, et al. Regulatory T cells induce a privileged tolerant microenvironment at the fetal-maternal interface. Eur J Immunol. (2006) 36:82–94. doi: 10.1002/eji.200535428
76. Polese B, Gridelet V, Araklioti E, Martens H, Perrier d’Hauterive S, Geenen V. The endocrine milieu and CD4 T-lymphocyte polarization during pregnancy. Front Endocrinol. (2014) 5:106. doi: 10.3389/fendo.2014.00106
77. Vacca P, Cantoni C, Vitale M, Prato C, Canegallo F, Fenoglio D, et al. Crosstalk between decidual NK and CD14+ myelomonocytic cells results in induction of Tregs and immunosuppression. Proc Natl Acad Sci USA. (2010) 107:11918–23. doi: 10.1073/pnas.1001749107
78. Gaudino SJ, Kumar P. Cross-talk between antigen presenting cells and T cells impacts intestinal homeostasis, bacterial infections, and tumorigenesis. Front Immunol. (2019) 10:360. doi: 10.3389/fimmu.2019.00360
79. Taddei CR, Cortez RV, Mattar R, Torloni MR, Daher S. Microbiome in normal and pathological pregnancies: a literature overview. Am J Reprod Immunol. (2018) 80:e12993. doi: 10.1111/aji.12993
80. Brotman RM. Vaginal microbiome and sexually transmitted infections: an epidemiologic perspective. J Clin Invest. (2011) 121:4610–7. doi: 10.1172/JCI57172
81. Petrova MI, Lievens E, Malik S, Imholz N, Lebeer S. Lactobacillus species as biomarkers and agents that can promote various aspects of vaginal health. Front Physiol. (2015) 6:81. doi: 10.3389/fphys.2015.00081
82. Rose WA, McGowin CL, Spagnuolo RA, Eaves-Pyles TD, Popov VL, Pyles RB. Commensal bacteria modulate innate immune responses of vaginal epithelial cell multilayer cultures. PLoS One. (2012) 7:e32728. doi: 10.1371/journal.pone.0032728
83. van de Wijgert JHHM. The vaginal microbiome and sexually transmitted infections are interlinked: consequences for treatment and prevention. PLoS Med. (2017) 14: e1002478. doi: 10.1371/journal.pmed.1002478
84. Ravel J, Gajer P, Abdo Z, Schneider GM, Koenig SSK, McCulle SL, et al. Vaginal microbiome of reproductive-age women. Proc Natl Acad Sci USA. (2011) 108(Suppl 1):4680–7. doi: 10.1073/pnas.1002611107
85. McKinnon LR, Achilles SL, Bradshaw CS, Burgener A, Crucitti T, Fredricks DN, et al. The evolving facets of bacterial vaginosis: implications for HIV transmission. AIDS Res Hum Retroviruses. (2019) 35:219–28. doi: 10.1089/aid.2018.0304
86. Torcia M. Interplay among vaginal microbiome, immune response and sexually transmitted viral infections. Int J Mol Sci. (2019) 20:266–213. doi: 10.3390/ijms20020266
87. Amabebe E, Anumba DOC. The vaginal microenvironment: the physiologic role of Lactobacilli. Front Med. (2018) 5:181. doi: 10.3389/fmed.2018.00181
88. Wessels JM, Felker AM, Dupont HA, Kaushic C. The relationship between sex hormones, the vaginal microbiome and immunity in HIV-1 susceptibility in women. Dis Model Mech. (2018) 11:dmm035147. doi: 10.1242/dmm.035147
89. Vitali D, Wessels JM, Kaushic C. Role of sex hormones and the vaginal microbiome in susceptibility and mucosal immunity to HIV-1 in the female genital tract. AIDS Res Ther. (2017) 14:39. doi: 10.1186/s12981-017-0169-4
90. Bradley F, Birse K, Hasselrot K, Noël-Romas L, Introini A, Wefer H, et al. The vaginal microbiome amplifies sex hormone-associated cyclic changes in cervicovaginal inflammation and epithelial barrier disruption. Am J Reprod Immunol. (2018) 80:e12863. doi: 10.1111/aji.12863
91. Murphy K, Keller MJ, Anastos K, Sinclair S, Devlin JC, Shi Q, et al. Impact of reproductive aging on the vaginal microbiome and soluble immune mediators in women living with and at-risk for HIV infection. PLoS One (2019) 14:e0216049. doi: 10.1371/journal.pone.0216049
92. Sansonetti PJ. To be or not to be a pathogen: that is the mucosally relevant question. Mucosal Immunol. (2010) 4:8–14. doi: 10.1038/mi.2010.77
93. Borgdorff H, Gautam R, Armstrong SD, Xia D, Ndayisaba GF, van Teijlingen NH, et al. Cervicovaginal microbiome dysbiosis is associated with proteome changes related to alterations of the cervicovaginal mucosal barrier. Mucosal Immunol. (2015) 9:621–33. doi: 10.1038/mi.2015.86
94. Anahtar MN, Byrne EH, Doherty KE, Bowman BA, Yamamoto HS, Soumillon M, et al. Cervicovaginal bacteria are a major modulator of host inflammatory responses in the female genital tract. Immunity. (2015) 42:965–76. doi: 10.1016/j.immuni.2015.04.019
95. Jespers V, Kyongo J, Joseph S, Hardy L, Cools P, Crucitti T, et al. A longitudinal analysis of the vaginal microbiota and vaginal immune mediators in women from sub-Saharan Africa. Sci Rep. (2017) 7:11974. doi: 10.1038/s41598-017-12198-6
96. Smith SB, Ravel J. The vaginal microbiota, host defence and reproductive physiology. J Physiol. (2016) 595:451–63. doi: 10.1113/JP271694
97. Kirjavainen PV, Pautler S, Baroja ML, Anukam K, Crowley K, Carter K, et al. Abnormal immunological profile and vaginal microbiota in women prone to urinary tract infections. Clin Vaccine Immunol. (2009) 16:29–36. doi: 10.1128/CVI.00323-08
98. Valore EV, Wiley DJ, Ganz T. Reversible deficiency of antimicrobial polypeptides in bacterial vaginosis. Infect Immun. (2006) 74:5693–702. doi: 10.1128/IAI.00524-06
99. Dunn AB, Dunlop AL, Hogue CJ, Miller A, Corwin EJ. The microbiome and complement activation: a mechanistic model for preterm birth. Biol Res Nurs. (2017) 19:295–307. doi: 10.1177/1099800416687648
100. Chu DM, Seferovic M, Pace RM, Microbiome autoimmunity, Aagaard KM. The microbiome in preterm birth. Best Pract Res Clin Obstet Gynaecol. (2018) 52:103–13. doi: 10.1016/j.bpobgyn.2018.03.006
101. Chen X, Liu S, Tan Q, Shoenfeld Y, Zeng Y. Microbiome, autoimmunity allergy, and helminth infection: the importance of the pregnancy period. Am J Reprod Immunol. (2017) 78:e12654. doi: 10.1111/aji.12654
102. Mori M, Bogdan A, Balassa T, Csabai T, and Szekeres-Bartho J. The decidua-the maternal bed embracing the embryo-maintains the pregnancy. Semin Immunopathol. (2016) 38:635–49. doi: 10.1007/s00281-016-0574-0
103. PrabhuDas M, Bonney E, Caron K, Dey S, Erlebacher A, Fazleabas A, et al. Immune mechanisms at the maternal-fetal interface: perspectives and challenges. Nat Immunol. (2015) 16:328–34. doi: 10.1038/ni.3131
104. D’Ippolito, S, Di Nicuolo F, Pontecorvi A, Gratta M, Scambia G, Di Simone N. Endometrial microbes and microbiome: recent insights on the inflammatory and immune “players” of the human endometrium. Am J Reprod Immunol. (2018) 80:e13065. doi: 10.1111/aji.13065
105. Benner M, Ferwerda G, Joosten I, van der Molen RG. How uterine microbiota might be responsible for a receptive, fertile endometrium. Hum Reprod Update. (2018) 24:393–415. doi: 10.1093/humupd/dmy012
106. Radtke AL, Quayle AJ, and Herbst-Kralovetz MM. Microbial products alter the expression of membrane-associated mucin and antimicrobial peptides in a three-dimensional human endocervical epithelial cell model. Biol Reprod. (2012) 87:132. doi: 10.1095/biolreprod.112.103366
107. Harding CV, Ramachandra L, Wick MJ. Interaction of bacteria with antigen presenting cells: influences on antigen presentation and antibacterial immunity. Curr Opin Immunol. (2003) 15:112–9. doi: 10.1016/S0952-7915(02)00008-0
108. Barnea ER, Kirk D, Todorova K, McElhinney J, Hayrabedyan S, and Fernández N. PIF direct immune regulation: blocks mitogen-activated PBMCs proliferation, promotes TH2/TH1 bias, independent of Ca(2+). Immunobiology. (2015) 220:865–75. doi: 10.1016/j.imbio.2015.01.010
109. Barnea ER, Vialard F, Moindjie H, Ornaghi S, Dieudonne MN, Paidas MJ. PreImplantation Factor (PIF∗) endogenously prevents preeclampsia: promotes trophoblast invasion and reduces oxidative stress. J Reprod Immunol. (2016) 114:58–64. doi: 10.1016/j.jri.2015.06.002
110. Di, Simone N, Di Nicuolo F, Marana R, Castellani R, Ria F, Veglia M, et al. Synthetic preimplantation factor (PIF) prevents fetal loss by modulating LPS induced inflammatory response. PLoS One. (2017) 12:e0180642. doi: 10.1371/journal.pone.0180642
111. Mueller M, Zhou J, Yang L, Gao Y, Wu F, Schoeberlein A, et al. Preimplantation factor promotes neuroprotection by targeting microRNA let-7. Proc Natl Acad Sci USA. (2014) 111:13882–7. doi: 10.1073/pnas.1411674111
112. Goodale LF, Hayrabedyan S, Todorova K, Roussev K, Ramu S, Stamatkin C, et al. Preimplantation factor (PIF) protects cultured embryos against oxidative stress: relevance for recurrent pregnancy loss (RPL) therapy. Oncotarget. (2017) 20:32419–32. doi: 10.18632/oncotarget.16028
113. Barnea ER, Lubman DM, Liu YH, Absalon-Medina V, Hayrabedyan S, Todorova K, et al. Insight into preimplantation factor (PIF∗) mechanism for embryo protection and development: target oxidative stress and protein misfolding (PDI and HSP) through essential RIKP [corrected] binding site. PloS One (2014) 7:e100263. doi: 10.1371/journal.pone.0100263
114. Schoenmakers S, Steegers-Theunissen R, Faas M. The matter of the reproductive microbiome. Obstet Med. (2019) 12:107–15. doi: 10.1177/1753495X18775899
115. Franasiak JM, Scott RT. Introduction: microbiome in human reproduction. Fertil Steril. (2015) 104:1341–3. doi: 10.1016/j.fertnstert.2015.10.021
116. Brotman RM, Klebanoff MA, Nansel TR, Yu KF, Andrews WW, Zhang J, et al. Bacterial vaginosis assessed by gram stain and diminished colonization resistance to incident gonococcal, chlamydial, and trichomonal genital infection. J Infect Dis. (2010) 202:1907–15. doi: 10.1086/657320
117. Wiesenfeld HC, Hillier SL, Krohn MA, Landers DV, Sweet RL. Bacterial vaginosis is a strong predictor of Neisseria gonorrhoeae and Chlamydia trachomatis infection. Clin Infect Dis. (2003) 36:663–8. doi: 10.1086/367658
118. van Houdt R, Ma B, Bruisten SM, Speksnijder AGCL, Ravel J, and de Vries HJC. Lactobacillus iners-dominated vaginal microbiota is associated with increased susceptibility to Chlamydia trachomatis infection in dutch women: a case–control study. Sex Transm Infect. (2018) 94:117–23. doi: 10.1136/sextrans-2017-053133
119. Tuominen H, Rautava S, Nen SSX, Collado MC, Rautava J. HPV infection and bacterial microbiota in the placenta, uterine cervix and oral mucosa. Sci Rep. (2018) 8:9787. doi: 10.1038/s41598-018-27980-3
120. Martin HL, Richardson BA, Nyange PM, Lavreys L, Hillier SL, Chohan B, et al. Vaginal lactobacilli, microbial flora, and risk of human immunodeficiency virus type 1 and sexually transmitted disease acquisition. J Infect Dis. (1999) 180:1863–8. doi: 10.1086/315127
121. Di Paola M, Sani C, Clemente AM, Iossa A, Perissi E, Castronovo G, et al. Characterization of cervico-vaginal microbiota in women developing persistent high-risk human papillomavirus infection. Sci Rep. (2017) 7:10200. doi: 10.1038/s41598-017-09842-6
122. Shannon B, Yi TJ, Perusini S, Gajer P, Ma B, Humphrys MS, et al. Association of HPV infection and clearance with cervicovaginal immunology and the vaginal microbiota. Mucosal Immunol. (2017) 10:1310–9. doi: 10.1038/mi.2016.129
123. Dareng EO, Ma B, Famooto AO, Adebamowo SN, Offiong RA, Olaniyan O, et al. Prevalent high-risk HPV infection and vaginal microbiota in Nigerian women. Epidemiol Infect. (2016) 144:123–37. doi: 10.1017/S0950268815000965
124. Lee JE, Lee S, Lee H, Song, Y-M, Lee K, Han MJ, et al. Association of the vaginal microbiota with human papillomavirus infection in a Korean twin cohort. PLoS One. (2013) 8:e63514. doi: 10.1371/journal.pone.0063514
125. Chen Y, Hong Z, Wang W, Gu L, Gao H, Qiu L, et al. Association between the vaginal microbiome and high-risk human papillomavirus infection in pregnant Chinese women. BMC Infect Dis. (2019) 19:677. doi: 10.1186/s12879-019-4279-6
126. Ritu W, Enqi W, Zheng S, Wang J, Ling Y, Wang Y. Evaluation of the associations between cervical microbiota and HPV infection, clearance, and persistence in cytologically normal women. Cancer Prev Res (Phila). (2019) 12:43–56. doi: 10.1158/1940-6207.CAPR-18-0233
127. van de Wijgert JHHM, Morrison CS, Cornelisse PGA, Munjoma M, Moncada J, Awio P, et al. Bacterial vaginosis and vaginal yeast, but not vaginal cleansing, increase HIV-1 acquisition in African women. J Acquir Immune Defic Syndr. (2008) 48:203–10. doi: 10.1097/QAI.0b013e3181743936
128. Borgdorff H, Tsivtsivadze E, Verhelst R, Marzorati M, Jurriaans S, Ndayisaba GF, et al. Lactobacillus-dominated cervicovaginal microbiota associated with reduced HIV/STI prevalence and genital HIV viral load in African women. ISME J. (2014) 8:1781–93. doi: 10.1038/ismej.2014.26
129. Petrova MI, van den Broek M, Balzarini J, Vanderleyden J, Lebeer S. Vaginal microbiota and its role in HIV transmission and infection. FEMS Microbiol Rev. (2013) 37:762–92. doi: 10.1111/1574-6976.12029
130. Stapleton AE. The vaginal microbiota and urinary tract infection. Microbiol Spectr. (2016) 4:1–6. doi: 10.1128/microbiolspec.UTI-0025-2016
131. Kyono K, Hashimoto T, Nagai Y, Sakuraba Y. Analysis of endometrial microbiota by 16S ribosomal RNA gene sequencing among infertile patients: a single-center pilot study. Reprod Med Biol. (2018) 17:297–306. doi: 10.1002/rmb2.12105
132. Bernabeu A, Lledo B, Díaz MC, Lozano FM, Ruiz V, Fuentes A, et al. Effect of the vaginal microbiome on the pregnancy rate in women receiving assisted reproductive treatment. J Assist Reprod Genet. (2019) 36:2111–9. doi: 10.1007/s10815-019-01564-0
133. Koedooder R, Singer M, Schoenmakers S, Savelkoul PHM, Morré, SA, de Jonge JD, et al. The vaginal microbiome as a predictor for outcome of in vitro fertilization with or without intracytoplasmic sperm injection: a prospective study. Hum Reprod. (2019) 34:1042–54. doi: 10.1093/humrep/dez065
134. Practice Committee of American Society for Reproductive Medicine. Definitions of infertility and recurrent pregnancy loss: a committee opinion. Fertil Steril. (2013) 99:63. doi: 10.1016/j.fertnstert.2012.09.023
135. Practice Committee of the American Society for Reproductive. Evaluation and treatment of recurrent pregnancy loss: a committee opinion. Fertil Steril. (2012) 5:1103–11. doi: 10.1016/j.fertnstert.2012.06.048
136. Kitaya K, Matsubayashi H, Yamaguchi K, Nishiyama R, Takaya Y, Ishikawa T, et al. Chronic endometritis: potential cause of infertility and obstetric and neonatal complications. Am J Reprod Immunol. (2015) 75:13–22. doi: 10.1111/aji.12438
137. Cicinelli E, Matteo M, Tinelli R, Pinto V, Marinaccio M, Indraccolo U, et al. Chronic endometritis due to common bacteria is prevalent in women with recurrent miscarriage as confirmed by improved pregnancy outcome after antibiotic treatment. Reprod Sci. (2014) 21:640–7. doi: 10.1177/1933719113508817
138. Kitaya K. Prevalence of chronic endometritis in recurrent miscarriages. Fertil Steril. (2011) 95:1156–8. doi: 10.1016/j.fertnstert.2010.09.061
139. Johnston-MacAnanny EB, Hartnett J, Engmann LL, Nulsen JC, Sanders MM, Benadiva CA. Chronic endometritis is a frequent finding in women with recurrent implantation failure after in vitro fertilization. Fertil Steril. (2010) 93:437–41. doi: 10.1016/j.fertnstert.2008.12.131
140. Kasius JC, Broekmans FJM, Sie-Go DMDS, Bourgain C, Eijkemans MJC, Fauser BC, et al. The reliability of the histological diagnosis of endometritis in asymptomatic IVF cases: a multicenter observer study. Hum Reprod. (2011) 27:153–8. doi: 10.1093/humrep/der341
141. Vitagliano A, Saccardi C, Noventa M, Di Spiezio Sardo A, Saccone G, Cicinelli E, et al. Effects of chronic endometritis therapy on in vitro fertilization outcome in women with repeated implantation failure: a systematic review and meta-analysis. Fertil Steril. (2018) 110:103–12.e1. doi: 10.1016/j.fertnstert.2018.03.017
142. Kitaya K, Tada Y, Hayashi T, Taguchi S, Funabiki M, Nakamura Y. Comprehensive endometrial immunoglobulin subclass analysis in infertile women suffering from repeated implantation failure with or without chronic endometritis. Am J Reprod Immunol. (2014) 72:386–91. doi: 10.1111/aji.12277
143. Solt I. The human microbiome and the great obstetrical syndromes: a new frontier in maternal-fetal medicine. Best Pract Res Clin Obstet Gynaecol. (2015) 29:165–75. doi: 10.1016/j.bpobgyn.2014.04.024
144. Vinturache AE, Gyamfi-Bannerman C, Hwang J, Mysorekar IU, Jacobsson B, Prebic TPBIC. Maternal microbiome – a pathway to preterm birth. Semin Fetal Neonatal Med. (2016) 21:94–9. doi: 10.1016/j.siny.2016.02.004
145. Brown RG, Marchesi JR, Lee YS, Smith A, Lehne B, Kindinger LM, et al. Vaginal dysbiosis increases risk of preterm fetal membrane rupture, neonatal sepsis and is exacerbated by erythromycin. BMC Med. (2018) 16:9. doi: 10.1186/s12916-017-0999-x
146. Peelen MJ, Luef BM, Lamont RF, de Milliano I, Jensen JS, Limpens J, et al. The influence of the vaginal microbiota on preterm birth: a systematic review and recommendations for a minimum dataset for future research. Placenta. (2019) 79:30–9. doi: 10.1016/j.placenta.2019.03.011
147. Fettweis JM, Serrano MG, Brooks JP, Edwards DJ, Girerd PH, Parikh HI, et al. The vaginal microbiome and preterm birth. Nat Med. (2019) 25:1–28. doi: 10.1038/s41591-019-0450-2
148. Dunlop AL, Mulle JG, Ferranti EP, Edwards S, Dunn AB, Corwin EJ. Maternal microbiome and pregnancy outcomes that impact infant health. Adv Neonat Care. (2015) 15:377–85. doi: 10.1097/ANC.0000000000000218
149. Romero R, Hassan S, Gajer P, Tarca AL, Fadrosh DW, Bieda J, et al. The vaginal microbiota of pregnant women who subsequently have spontaneous preterm labor and delivery and those with a normal delivery at term. Microbiome. (2014) 2:31–15. doi: 10.1186/2049-2618-2-18
150. Callahan BJ, DiGiulio DB, Goltsman DSA, Sun CL, Costello EK, Jeganathan P, et al. Replication and refinement of a vaginal microbial signature of preterm birth in two racially distinct cohorts of US women. Proc Natl Acad Sci USA. (2017) 114:9966–71. doi: 10.1073/pnas.1705899114
151. Son K-A, Kim M, Kim YM, Kim SH, Choi S-J, Oh S-Y, et al. Prevalence of vaginal microorganisms among pregnant women according to trimester and association with preterm birth. Obstet Gynecol Sci. (2018) 61:38–10. doi: 10.5468/ogs.2018.61.1.38
152. Stout MJ, Zhou Y, Wylie KM, Tarr PI, Macones GA, Tuuli MG. Early pregnancy vaginal microbiome trends and preterm birth. Am J Obstet Gynecol. (2017) 217:356.e1–e8. doi: 10.1016/j.ajog.2017.05.030
153. Solt I, Cohavy O. The great obstetrical syndromes and the human microbiome – a new frontier. Rambam Maimonides Med J. (2012) 3:e0009. doi: 10.5041/RMMJ.10076
154. Lawn JE, Cousens S, Zupan J. Lancet neonatal survival steering team. 4 million neonatal deaths: when? where? why? Lancet. (2005) 365:891–900. doi: 10.1016/S0140-6736(05)71048-5
155. Kim CJ, Romero R, Chaemsaithong P, Chaiyasit N, Yoon BH, Kim YM. Acute chorioamnionitis and funisitis: definition, pathologic features, and clinical significance. Am J Obstet Gynecol. (2015) 213:S29–52. doi: 10.1016/j.ajog.2015.08.040
156. Fortner KB, Grotegut CA, Ransom CE, Bentley RC, Feng L, Lan L, et al. Bacteria localization and chorion thinning among preterm premature rupture of membranes. PLoS One (2014) 9:e83338. doi: 10.1371/journal.pone.0083338
157. Steel JH, Malatos S, Kennea N, Edwards AD, Miles L, Duggan P, et al. Bacteria and inflammatory cells in fetal membranes do not always cause preterm labor. Pediatr Res. (2005) 57:404–11. doi: 10.1203/01.PDR.0000153869.96337.90
158. Watts DH, Krohn MA, Hillier SL, Eschenbach DA. The association of occult amniotic fluid infection with gestational age and neonatal outcome among women in preterm labor. Obstet Gynecol. (1992) 79:351–7. doi: 10.1097/00006250-199203000-00005
159. Ilekis JV, Tsilou E, Fisher S, Abrahams VM, Soares MJ, Cross JC, et al. Placental origins of adverse pregnancy outcomes: potential molecular targets: an executive workshop summary of the eunice kennedy shriver national institute of child health and human development. Am J Obstet Gynecol. (2016) 15(Suppl. 1):S1–46. doi: 10.1016/j.ajog.2016.03.001
160. Barker DJ. Adult consequences of fetal growth restriction. Clin Obstet Gynecol. (2006) 49:270–83. doi: 10.1097/00003081-200606000-00009
161. Budding AE, Grasman ME, Lin F, Bogaards JA, Soeltan-Kaersenhout DJ, Vandenbroucke-Grauls, CMJE, et al. IS-pro: high-throughput molecular fingerprinting of the intestinal microbiota. FASEB J. (2010) 24:4556–64. doi: 10.1096/fj.10-156190
162. Riscuta G, Xi D, Pierre-Victor D, Starke-Reed P, Khalsa J, Duffy L. Diet, microbiome, and epigenetics in the era of precision medicine. Methods Mol Biol. (2018) 1856:141–56. doi: 10.1007/978-1-4939-8751-1_8
163. Ruiz L, Moles L, Gueimonde M, Rodríguez JM. Perinatal microbiomes “Influence on Preterm Birth and Preterms” health: influencing factors and modulation strategies. J Pediatr Gastroenterol Nutr. (2016) 63:e193–203. doi: 10.1097/MPG.0000000000001196
164. Sharma H, Tal R, Clark N, Segars J. Microbiota and pelvic inflammatory disease. Semin Reprod Med. (2014) 32:43–9. doi: 10.1055/s-0033-1361822
165. Wallace DC. Bioenergetics and the epigenome: interface between the environment and genes in common diseases. Dev Disabil Res Rev. (2010) 16:114–9. doi: 10.1002/ddrr.113
166. Jasiulionis MG. Abnormal epigenetic regulation of immune system during aging. Front Immunol. (2018) 9:197. doi: 10.3389/fimmu.2018.00197
167. Niemarkt, HJ De Meij TG, van Ganzewinkel, C-J, de Boer NKH, Andriessen P, Hütten, MC, et al. Necrotizing enterocolitis, gut microbiota, and brain development: role of the brain-gut axis. Neonatology. (2019) 115:423–31. doi: 10.1159/000497420
168. Baldassarre ME, DiMauro A, Capozza M, Rizzo V, Schettini F, Panza R, et al. Dysbiosis and prematurity: is there a role for probiotics? Nutrients. (2019) 11:1273–1212. doi: 10.3390/nu11061273
169. Jiménez E, Marín ML, Martín R, Odriozola JM, Olivares M, Xaus J, et al. Is meconium from healthy newborns actually sterile? Res Microbiol. (2008) 159:187–93. doi: 10.1016/j.resmic.2007.12.007
170. Rautava S, Kainonen E, Salminen S, Isolauri E. Maternal probiotic supplementation during pregnancy and breast-feeding reduces the risk of eczema in the infant. J Allergy Clin Immunol. (2012) 130:1355–60. doi: 10.1016/j.jaci.2012.09.003
171. Gomez de Agüero M, Ganal-Vonarburg SC, Fuhrer T, Rupp S, Uchimura Y, Li H, et al. The maternal microbiota drives early postnatal innate immune development. Science. (2016) 351:1296–302. doi: 10.1126/science.aad2571
172. Goldenberg RL, McClure EM, Saleem S, Reddy UM. Infection-related stillbirths. Lancet. (2010) 375:1482–90. doi: 10.1016/S0140-6736(09)61712-8
173. Willyard C. Could baby’s first bacteria take root before birth? Nature. (2018) 553:264–6. doi: 10.1038/d41586-018-00664-8
174. Stinson LF, Payne MS, Keelan JAA. Critical review of the bacterial baptism hypothesis and the impact of cesarean delivery on the infant microbiome. Front Med. (2018) 5:135. doi: 10.3389/fmed.2018.00135
175. Dominguez-Bello MG, De Jesus-Laboy KM, Shen N, Cox LM, Amir A, Gonzalez A, et al. Partial restoration of the microbiota of cesarean-born infants via vaginal microbial transfer. Nat Med. (2016) 22:250–3. doi: 10.1038/nm.4039
176. Reardon S. Do C-section babies need mum’s microbes? Trials tackle controversial idea. Nature. (2019) 572:423–4. doi: 10.1038/d41586-019-02348-3
Keywords: microbiota, immunology, female reproductive tract, vaginal, endometrial, placental, preterm birth, pregnancy complications
Citation: Al-Nasiry S, Ambrosino E, Schlaepfer M, Morré SA, Wieten L, Voncken JW, Spinelli M, Mueller M and Kramer BW (2020) The Interplay Between Reproductive Tract Microbiota and Immunological System in Human Reproduction. Front. Immunol. 11:378. doi: 10.3389/fimmu.2020.00378
Received: 23 August 2019; Accepted: 17 February 2020;
Published: 16 March 2020.
Edited by:
Mats Bemark, University of Gothenburg, SwedenReviewed by:
Laura Ensign, Johns Hopkins University, United StatesCopyright © 2020 Al-Nasiry Ambrosino, Schlaepfer Morré, Wieten Voncken, Spinelli Mueller and Kramer. This is an open-access article distributed under the terms of the Creative Commons Attribution License (CC BY). The use, distribution or reproduction in other forums is permitted, provided the original author(s) and the copyright owner(s) are credited and that the original publication in this journal is cited, in accordance with accepted academic practice. No use, distribution or reproduction is permitted which does not comply with these terms.
*Correspondence: Salwan Al-Nasiry, c2Fsd2FuLmFsbmFzaXJ5QG11bWMubmw=
†These authors have contributed equally to this work
Disclaimer: All claims expressed in this article are solely those of the authors and do not necessarily represent those of their affiliated organizations, or those of the publisher, the editors and the reviewers. Any product that may be evaluated in this article or claim that may be made by its manufacturer is not guaranteed or endorsed by the publisher.
Research integrity at Frontiers
Learn more about the work of our research integrity team to safeguard the quality of each article we publish.