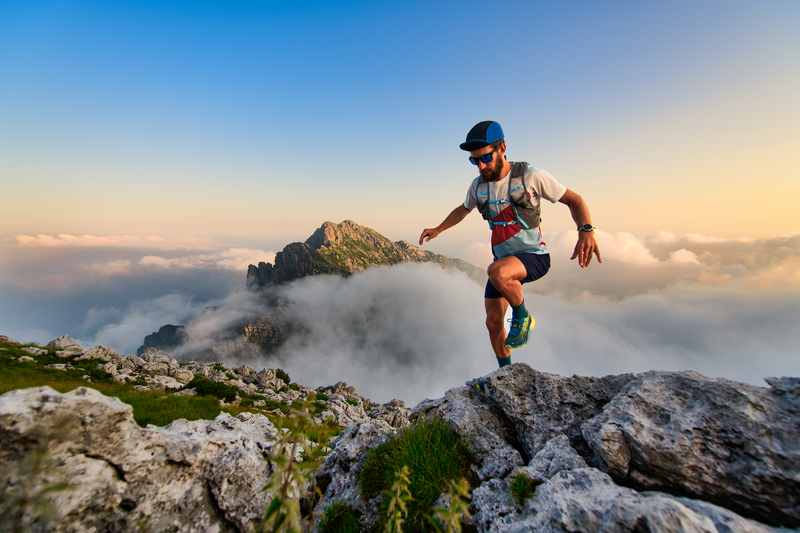
95% of researchers rate our articles as excellent or good
Learn more about the work of our research integrity team to safeguard the quality of each article we publish.
Find out more
REVIEW article
Front. Immunol. , 08 January 2020
Sec. Nutritional Immunology
Volume 10 - 2019 | https://doi.org/10.3389/fimmu.2019.02981
This article is part of the Research Topic Dietary Polyphenols for Improving Gut Health: Volume 1 View all 23 articles
Polyphenols are essential antioxidants in our regular diet, and have shown potential antibacterial effects. Other important biological effects, such as anticancer or antibacterial activities, have been demonstrated by some polyphenols. In recent years, the benefits of polyphenols to human health have attracted increasing attention from the scientific community. Recent studies have shown that polyphenols such as anthocyanin, catechin, chlorogenic acid, and resveratrol can inhibit pathogenic bacteria such as Escherichia coli and Salmonella to help regulate intestinal microflora. An imbalance of intestinal microflora and the destruction of intestinal barrier function have been found to have a potential relationship with the occurrence of chronic kidney disease (CKD). Specifically, they can aberrantly trigger the immune system to cause inflammation, increase the production of uremic toxins, and further worsen the condition of CKD. Therefore, the maintenance of intestinal microflora and the intestinal tract in a stable and healthy state may be able to “immunize” patients against CKD, and treat pre-existing disease. The use of common antibiotics may lead to drug resistance in pathogens, and thus beneficial polyphenols may be suitable natural substitutes for antibiotics. Herein we review the ability of different polyphenols, such as anthocyanin, catechin, chlorogenic acid, and resveratrol, to regulate intestinal microorganisms, inhibit pathogenic bacteria, and improve inflammation. In addition, we review the ability of different polyphenols to reduce kidney injury, as described in recent studies.
Chronic kidney disease (CKD) affects over 10% of the population and is increasingly pervasive in several nations, largely due to aging populations and changing lifestyles (1). Disease presence and occurrence vary due to differences in people's pre-existing underlying conditions and access to government-subsidized care. In many countries, the frequency of CKD is as high as 200 cases per million per year (2).
CKD is characterized by chronic renal damage and/or functional impairment, and it is often associated with high rates of morbid obesity and mortality throughout its progression, from early to advanced stages, at which point renal replacement therapy (i.e., dialysis or transplant) is required. Although, significant progress has been achieved in the prevention, detection, and treatment of CKD, it remains a significant public health problem (3), and there is therefore an immediate need to develop new approaches to treat patients with CKD (4).
The human intestinal tract is the location of a diverse bacterial community denoted the intestinal microbiota. It has a key role in preserving the well-being of humans, but it is vulnerable to external factors such as dietary patterns and the use of medications (5). The microbiota retains a codependent relationship with its human host in normal conditions, but its dysfunction has been linked with a number of diseases. For example, intestinal microbial dysbiosis occurs in CKD, manifesting as an increase in pathogenic flora relative to symbiotic flora (5). CKD has also been observed to increase the permeability of the intestinal barrier, leading to the increased passage of endotoxins and other bacterial substances into the blood (6).
In a specific example, an accumulation of uremic contaminants is usually associated with an increased risk of CKD development. Some uremic toxins or their precursors are generated by the processing of nutrients by intestinal microbiota, where these precursor are typically trimethylamine N-oxide (TMAO), indole-3-acetic acid, p-cresyl sulfate, and indoxyl sulfate. A higher intake of certain nutrients may also affect the intestinal microbiota, and lead to the increased bacterial production of uremic toxins or precursors. Thus, circulating concentrations of nutrient-derived uremic contaminants are associated with an increased risk of CKD development (7).
Dietary polyphenols found in a wide range of plant foods have been shown to be correlated with beneficial effects in the hosts (8). Polyphenols can also help to prevent chronic diseases such as cardiovascular disease, hypertension, obesity, and neurodegenerative diseases (1). Studies have shown that dietary polyphenols can help to maintain bowel health by promoting the development of beneficial bacteria (i.e., Lactobacilli and Bifidobacteria), thereby maintaining microbial gut equilibria, and by inhibiting pathogenic bacteria, thus demonstrating polyphenols' positive prebiotic effect (8).
In this article we review the evidence in the literature of a link between intestinal health and CKD, with a special focus on the effects of polyphenols on the regulation of intestinal microbial communities and immune responses for the prevention and treatment of CKD.
Polyphenols are a class of phytochemicals with the strongest presence in plants. They are the products of secondary plant metabolism via two fundamental metabolic pathways: the shikimate pathway and the acetate pathway. Polyphenols are categorized as flavonoids or non-flavonoids, and are present in two main structural forms: bound to sugars to form glycosides, which have enhanced solubility, or as free, non-sugar-bound species, known as aglycones (9). Polyphenol aglycones are a heterogeneous molecular group, classified into four main classes according to their chemical structure: flavonoids (e.g., flavonols, flavanols, flavanones, anthocyanidin, chalcone, dihydroflavonoles, and isoflavone), lignans, stilbenes, and tannins [e.g., phenolic acids, such as hydroxybenzoic, hydroxygenylpropanoic, and hydroxybenzoic acids (5)].
The potential health benefits of polyphenols and their possible ability to help prevent cancer and cardiovascular diseases have made them a subject of intensive research. Polyphenols have demonstrated health-enhancing effects in anticarcinogenic, antimicrobial, vasodilatory, and analgesic processes (10). Mechanistically, the in situ intestinal processing of polyphenols by microbes in the human intestine yields potentially bioactive low-molecular-weight metabolites, which are the putative health-enhancing species (11).
There are different ways to define bioavailability, and the commonly accepted conceptualization is that it represents the proportion of nutrients that are digested, absorbed and metabolized by normal biochemical pathways. It is therefore important to know not only how many foods or dietary supplements contain a given nutrient, but also how much of this nutrient is bioavailable (12). Various polyphenols, such as flavonoids and phenolic acids, have been found to reduce the absorption of minerals such as iron, zinc and copper species, and other trace components, most likely due to chelation, e.g., by galloyl and catechol hydroxyl groups. For example, polyphenols like epigallocatechin gallate (EGCG) and other gallates appear to negatively affect iron and zinc absorption in Caco-2 (colon cancer) cells (13). The effect of the intake of minerals on the bioavailability of polyphenols has been thoroughly evaluated, except for the more controversial effect on zinc (14).
In the past, the concept of a prebiotic applied only to carbohydrates, but recent evidence has led to a new definition: “a prebiotic is a non-ingestible compound that modulates the structure or function of gut microbiota through its metabolization by microorganisms in the intestines, thereby giving the hosts a physiologically beneficial effect.” Crucially, this new definition includes polyphenols as relevant microbiota modulators (15). A human study found that red wine polyphenol consumption increased the levels of Enterococcus, Prevotella, Bacteroides, Bifidobacterium, Bacteroides uniformis, Eggerthella lenta, and Blautiacoccoides in the rectale group in the intestine. Meanwhile, the quantity of Lactobacillus spp. was unchanged. Moreover, the development of pathogenic bacteria such as Clostridium difficile, Clostridium perfringens, and Bacteroides spp. was significantly constrained by the treatment of cultures with different tea phenols (16). In addition, while anaerobes such as Bifidobacterium and Lactobacillus are less affected, Vendrame et al. observed a significant increase in the quantity of Bifidobacterium after the consumption of beverages containing wild blueberries, indicating that wild blueberry-derived polyphenols play a key role in the regulation of intestinal microbiota composition (16).
Anthocyanins are water-soluble plant pigments that exhibit intensely vibrant violet, red, or blue colors. The comprise a primary subclass of polyphenol flavonoids, and epidemiological studies have shown that they can improve a diverse range of health indicators, such as vision, blood pressure, and cognition, as well as protect against risk factors for heart disease (17).
The growth of Bifidobacterium spp. and Lactobacillus-Enterococcus spp. have been found to be widely and significantly enhanced by anthocyanins, indicating that anthocyanin metabolites support intestinal bacterial community members (11). Studies have shown that dietary anthocyanins obtained from fruits and vegetables can protect against bowel inflammation and offer other colon health benefits. Thus, anthocyanins are active in maintaining intestinal mucosity, restoring the epithelial barrier structure, immuno-modulating and controlling the microbiota, which combine to provide anti-inflammatory benefits (18, 19). In a model of DSS-induced murine colitis, a 2-week diet of cooked black bean (20% consumption) dramatically blocked colon shortening and spleen enlargement (20). Bibi et al. found that red raspberry anthocyanins exhibited protective barrier activity in the intestine; specifically, they significantly inhibited the elevation of claudin-2 protein and increased the expression of claudin-3 and ZO-1 under dextran sulfate sodium (DSS) treatment (21).
These results show that anthocyanins are able to protect the intestinal barrier by modulating tight junction-positive and -negative protein ratios, exemplifying the anti-colonic inflammation effects of anthocyanins from various fruits and vegetables (22, 23). In addition, anthocyanins have high antioxidant abilities, attributable to the ability of their phenolic groups to donate electrons, or transfer electrons from hydrogen atoms, to quench free radicals. Moreover, anthocyanins have shown anti-estrogenic, anti-inflammatory, cell proliferation-inhibiting abilities, and the capacity to reduce lipid accumulation during adipocyte differentiation, further demonstrating their ability to decrease disease risk (24).
Catechins are volatile and can be easily degraded and metabolized by interactions with hydroxyl groups on phenol rings under physiological conditions. Even when administered intravenously, catechins are partly degraded before they reach the target tissues (25). Catechins also undergo metabolic breakdown in the liver, small intestine, and colon (26). Several recent studies have described the anti-infective characteristics of the primary catechin of green tea leaves, epigallocatechin-3-gallate (EGCG). Several authors have observed that EGCG blocks the entry of the hepatitis C virus by impairing viral attachment to the cell surface (27). EGCG has also been found to block cell invasion by multiple viruses and exhibit anti-HIV-1 viral effects at several points in the viral lifecycle (28). It has also been shown that catechins and tea epigallocatechins have a protective effect in gastrointestinal ailments such colitis and colon cancer (5). The antimicrobial activity of green tea catechins (GTCs) has been widely researched, and green tea has been shown to function synergistically with various antibiotics in many ways, directly and indirectly, against these pathogens. Antimicrobial activity can also be derived from the anti-inflammatory and antioxidant properties of green tea (26).
During carcinogenesis, the diversity and count of microbiota are enriched, Bacteroides spp. thrive and the abundance of butyrate-producing bacteria, such as Clostridiaceae and Ruminococcus, decreases continuously (29). In comparison, EGCG treatment during colon carcinogenesis maintains a relatively stable intestinal microbiota composition (29). This tumor-suppressive effects of EGCG may be attributable to its enhancement of the accumulation of beneficial microbes such as Bifidobacterium (29). Thus, EGCG and other dietary components affects the intestinal microbiota. In addition, EGCG exhibits antioxidant behavior and epigenetic effects, such as inhibiting DNMT1 to block CpG methylation, thus re-activating silenced genes, such as tumor suppressors (30). These results jointly indicate the protective effects of epicatechin against high-fat diet (HFD)-related increased intestinal permeability and endotoxemia, and against cancer. This also partly explains epicatechin's ability to prevent steatosis and insulin resistance in people who consume an HFD (31). Further studies are needed to investigate the molecular mechanisms of bioactive catechins (32).
Resveratrol is an antifungal and antibacterial stilbenoid derived from plants and can be obtained from diets consisting of various fruits such as grapes and their juice, oranges and cranberries, red currants and peanut skins (33). In both in vitro and in vivo studies, resveratrol has proved to be an effective antioxidant, antibacterial, anti-obesity, anti-inflammatory, and anti-cancer agent (34–36). Resveratrol is generally thought to be an efficient scavenger of reactive oxygen species (ROS) and free radicals. Moreover, Manach et al. suggested that the main nutrient antioxidants in the colon are polyphenols because the small intestine utilizes other antioxidants such as vitamins E and C. Khader et al. also reported novel resveratrol analogs that may alleviate ischemia-reperfusion-induced renal damage by the regulation of IL-1, IL-6, and TNF-α levels, and the modulation of the host energy metabolism (37). In contrast, Bienholz et al. reported that resveratrol itself did not ameliorate ischemia-reperfusion-induced acute kidney injury in rats (38).
Although the effect of resveratrol on host metabolism has been shown to be positive, analyses of the plasma concentrations have indicated that resveratrol has low bioavailability, as it is too rapidly metabolized to be an effective orally administered medication. Hepatic and gut microbial enzymes can metabolize resveratrol (34), and, surprisingly, numerous studies have suggested that the composition of intestinal microbiota is modulated by resveratrol, and that this may account for its anti-obesity activity and metabolic benefits. Resveratrol intake (200 mg/kg/day) effects were first demonstrated in an overweight HFD mouse model, where a marked improvement in gut microbial dysbiosis and reduced fat/body weigh were observed. Impressively, the counts of Lactobacillus and Bifidobacterium and the levels of Bacteroidetes and Firmicutes–which usually decrease in obese animals—significantly increased, while the concentration of Enterococcus faecalis decreased (36).
Other research has revealed that resveratrol can enhance the integrity of cell junctions by upregulating the expression of intestinal tight-junction proteins such as tight-junction protein 2 (39) and occludin. In addition, resveratrol has been shown to facilitate the assembly of close junction protein claudine 4, which may reverse the increase in intestinal permeability caused by the widespread mycotoxin deoxynivaenol. Given the role of resveratrol in maintaining an intact intestinal barrier and the “leaky gut” pathophysiology present in several mechanisms of disease, this research avenue warrants further investigation (33).
Finally, the dietary use of resveratrol is partly able to ease the adverse effects of heat stress in broilers by restoring the damaged structure of the villus-crypt in the intestinal barrier, altering intestinal microflora profiles and increasing the mRNA expression of genes related to tight- and adherence-junctions of the gut (35).
Chlorogenic acid (3-O-caffeoyl-quinic acid, C-QA) is the caffeic ester of quinic acid, and one of the most abundant phenolic acids in the Western diet. It was found that some C-QA bypasses absorption in the small intestine and travels to the colon, where it is converted into several metabolites by the resident microbiota (40). C-QA administration (150 mg per kg daily) significantly increased body fat depletion, decreased lipid levels in plasma and altered levels of mRNA of lipogenesis and lipolysis related-genes in adipose tissues. In addition, C-QA reversed gut microbiota dysbiosis caused by an HFD, substantially inhibiting the growth of Desulfovibrionaceae, Ruminococcaceae, Lachnospiraceae, Erysipelotrichaceae, and boosting the growth of Bacteroidaceae and Lactobacillaceae.
The C-QA-generated amelioration of HFD-induced dysbiosis in gut microbiota may partly explain its beneficial effects on HFD-induced obesity (41). Moreover, C-QA therapy has been shown to reverse the deleterious effect of lead ion (Pb2+)-induced intestinal microbiota compositional changes. For example, the proportion of Helicobacter increased from 2.95% (Pb2+) to 11.24 % (Pb2 + + C-QA) and the proportion of Lachnospiraceae NK4A136 group decreased from 7.09% (Pb2+) to 2.68% (Pb2+ + C-QA) (42). This suggests that C-QA is a natural product capable of reversing Pb2+-induced nephrotoxicity and hepatotoxicity.
In a DSS-induced colitis mouse model, C-QA acted on the bacterial population to modify intestinal structure, leading to lower intestinal and systemic inflammation and increased healthy progression, related to a proportional increase in Akkermansia (43). Moreover, C-QA reduced diversity loss in the composition of fecal microbiota in ACTH-treated rats (44). C-QA enhanced microbiota diversity at the genus and order level: pre-treatment with C-QA increased the relative abundance of certain primary bacteria in the gut, such as Desulfovibrionales, Desulfovibrio, and Burkholderiales, as well as Klebsiella and Bifidobacterium.
The body's gastrointestinal system is colonized by an extremely large number of microorganisms, primarily bacteria. It has been calculated that the human adult microbiota comprises ~1014 bacterial cells, equivalent to ~10 times the total average number of human cells. Meanwhile, the metabolic capacity of the intestinal microbiome is ~10 times greater than the liver's metabolic abilities (45). The host organism and the intestinal microbiota change and develop together, executing a wide range of immunogenic, and metabolic interactions (46–48). Commensal bacteria promote cell generation, growth, and maturity within the intestine, improve immune functions and provide a defense against enteric pathogens (49). Indeed, the intestinal microbiota plays a crucial role in preventing and protecting the host organism from infection, as it inhibits the spread and colonization of pathogens. Moreover, intestinal microbiota assist in the process of native immune-cell differentiation and development (46).
Advanced metagenome research, such as DNA sequencing-based studies used for microbial ecology analysis, has identified strong correlations between intestinal microbiota and human disease. Knowledge of the intestinal microbiome and various microbial functionalities has accumulated in recent decades, primarily generated from large-scale research projects such as the Human Microbiome Project (HMP), the European Metagenomics of the Human Intestinal Tract (MetaHIT), integrative HMP (iHMP), and MetaGenoPolis. As technology and clinic-based care become more interconnected, it will become possible to monitor and assess an individual's health on the basis of his/her intestinal microbiome data (50).
In addition, intestinal microbes are involved in the physiology of the gastrointestinal tract, and changes in their relative population concentrations can damage the beneficial relationship between microbiota and host organism, and have a direct effect on health (51). Over the last decade, researchers have emphasized several key dimensions of the mammalian host–gut microbial interaction. The microbiota plays a very significant role in human metabolism, and is now regarded as a metabolizing “organ” with effects on endo- and xenobiotic catalysis, carbohydrate metabolism and on other key chemical and immunological processes (52).
The effect of the intestinal microbiota on human health merits more attention given that significant differences in the composition of intestinal bacteria or the gut itself are seen in subjects with inflammatory bowel disease, autoimmune diseases, allergies, and lifestyle-related illnesses (53). Notably, the dietary-influenced composition of microbiota is not consistent throughout a person's life; for example, it is normal for the diet of elderly people to change due to decreasing taste and smell perception, tooth loss and chewing problems, or because illnesses necessitate the removal of certain components from their diets (45).
In the last 50 years, many sociocultural developments have brought new, greater disturbances to the human gut microbial ecology, as the modernization of the Western industrial lifestyle has led to the increased intake of antibiotics and components from the production of meat-based and hygiene goods (e.g., emulsifiers and sweetening agents). Complex habitats such as the bacterial intestinal ecosystems can maintain only a few balanced equilibrium states (54), and when these delicate equilibria are strongly perturbed, host immune cells target resident bacteria. In people with certain genetic defects in immune response control, this can create chronic bowel inflammation (53). In another example, research comparing human host insulin sensitivity, the fasting serum metabolome, and the intestinal microbiome with findings from mice studies has indicated that specific bacteria can cause resistance to insulin (55).
Additionally, the early composition of microbiota in preterm infants has been shown to be an indicator of the future occurrence of necrotizing enterocolitis (56). Animal studies have also demonstrated that the early microbiota composition is a key driver of the correct subsequent development of homeostasis, and of the host as a whole (5). Inflammatory bowel disorders (IBDs), inflammatory skin diseases such as psoriasis and atopic dermatitis, auto-immune arthritis, type 2 diabetes, obesity, and atherosclerosis have been found to be associated aberrant structure and function of the intestinal microbiota (57).
Some phenolic compounds with bacteriostatic and bactericidal activity have been considered as potential antimicrobial agents. It has been shown that these can be used to prevent bacterial infections in the stomach and urinary epithelium (11). The effects of common polyphenols on the growth of human intestinal bacteria and their adherence to enterocytes were investigated by Parkar et al. (58). The in vitro viability of representative gut microflora was found to be affected by doses of polyphenols equivalent to concentrations likely to be present in the gastrointestinal tract, but these effects depended on the type of polyphenols analyzed, and did not include rutin (45).
Polyphenol-rich cocoa decreased the Firmicutes/ Bacteriodetes ratio and increased Faecalibacterium prausnitzii levels in pigs (59). The countering of intestinal microbiota dysbiosis in rats fed with an HFD and sucrose diet inhibited body weight gain, decreased serum insulin, reduced the Firmicutes/Bacteriodetes ratio, and restricted bacterium production associated with lifestyle-associated obesity (60, 61). A relative increase in the abundance of bactericides in subjects drinking red wine polyphenols has also been reported (62).
Bifidobacterium is a widely used probiotic strain with documented health benefits in areas such as immuno-modulation, cancer prevention, and IBD control (57). Vendrame et al. found a significant rise in Bifidobacteria in subjects who drank a wild blueberry-based beverage, showing the important role of polyphenols within wild blueberries in the regulation of the intestinal microbiota structure and environment (16). Table 1 provides a summary of recent studies on the effects of various polyphenols on intestinal health.
CKD is characterized by an unusual renal structure or activity with health consequences spanning more than 3 months. Indicators include albuminuria, irregular urinary debris, abnormal vision, serum electrolyte and pH changes and a glomerular filtration rate (GFR) <60 ml.min−1 relative to a normal body surface area of 1.73 m2 (78).
CKD is a major risk factor for cardiovascular disease (CVD). Uremic conditions such as CKD are treated as a consequence of aggregation of so-called uremic retention solutes (URSs), usually released from the kidneys as organic waste materials. URSs derived from phenols and indoles exist along the gastrointestinal tract (GIT), and intestinal microbiota have been shown to have be crucial in preventing detrimental effects of USRs on the cardiovascular system (79).
Hypertension, leading to renal arteriolar sclerosis and nephrosclerosis, is another important risk factor for CKD. Increasing evidence has shown that the intestinal microbiota can affect arterial blood pressure. In addition, experiments involving the transplantation of fecal material from hypertensive rats to standard-stretched mice and rats have revealed a causal relationship between gut dysbiosis and hypertension in normotensive animals. Hypertension may therefore be another gut dysbiosis mediated cause of CKD (80).
Chronic kidney failure is generally associated with aging, diabetes, asthma, obesity, and cardiovascular diseases in the developed world, with diabetic glomerulosclerosis and hypertensive nephrosclerosis being the suspected pathological entities. However, precise diagnosis is often difficult (2). Renal hypoplasia/dysplasia, posterior urethral valve problems, and other congenital kidney-or urinary tract abnormalities are the most severe etiologies. Other etiologies include polycystic kidney disease, cortical necrosis, and renal vascular thrombosis (81). CKD is usually detected in many patients during diagnostic screening procedures for other diseases. Declines in kidney function cause central and peripheral neural activity changes, due to synergistic pathway defects such as vascular calcification and urinary toxin increases both in humans and in rodents, leading to many neurological complications (82).
Current evidence suggests that the host–microbiome relationship is bidirectional in people and animals with CKDs. Changes in microbiosis and function lead to excessive levels of uremic contaminants (e.g., indoxyl sulfate, p-cresyl sulfate, and TMAO) and decreases in levels of protective metabolites, and these changes are implicated in oxidative stress, uremia, inflammation, and deterioration of kidney function, and increased incidence of cardiovascular risk and mortality (83).
A variety of proteinuric kidney diseases such as IgA nephropathy and lupus nephritis are associated with microbial dysbiosis. By regulating the activity of regulatory T cells (Treg), intestinal microbiota can influence the development of idiopathic nephrotic syndrome, which has been shown to be alleviated by increased Treg activity. In addition, the development of renal amyloidosis may be facilitated by the chronic inflammation caused by intestinal dysbiosis, as this contributes to the production of chronically regulated serum amyloids A (SAA) (80). Levels of claudin-1, occluding, and ZO-1 protein are excessively reduced in the colonic mucosa of animals with CKD, as shown by Vaziri et al. (84). This suggests that the disruption of the colonic epithelial tight-junction could lead to bacterial and endotoxin translocation across the intestinal wall, generating the systemic inflammation typical of CKD. A damaged bowel barrier structure has been shown to enable the transmission of uremic toxins, contributing to an accumulation of these toxins, due to the compromised excretory process (85).
In addition, as proteobacteria may cause inflammation by damaging intestinal barrier functions and generating pro-inflammatory compounds such as uremic metabolites and serotonin, an increased Proteobacteria count may contribute to the pathogenesis of a number of chronic inflammatory diseases, and thus lead to proteinuric nephropathy (80).
When analyzing the causes of CKD and the factors affecting its progression—such as type 2 mellitus (T2DM), overweightness and hypertension—it can be concluded that lifestyle changes can directly and positively affect the natural course of CKD (1). Post-natal bacterial bowel colonization stimulates the immune system and decreases sensitivity to food and toxins. Human immune regulation of the microbiota is best demonstrated by a gut microbial human-immune maturation induction experiment. In this manner, different species of microbiota have been shown to cause levels of forkhead protein 3-expressing Tregs to increase, or to differentiate. Some of these Tregs are active in microbial antigen detection, and in germfree mice with a number of specified benign commensals, levels of colonic Tregs also increase (85). Cumulative analyses and experiments have shown that some microbial species of human intestinal microbiota are correlated with multiple physiological disorders, including those with effects on human health. The Bifidobacteria in breast-fed children are a typical example of this association.
Thus, the protective effect of nutritious feeding and numerous studies on immune disorders and diseases have been demonstrated (51). Host genes and immune function also influence gut microbiota composition. To date, the human genes believed to affect the structure of the intestinal microbiota have been mostly those coding for proteins of the immune system (86).
Intestinal dysbiosis, typical in CKD subjects, results from qualitative and quantitative modifications of the host microbiome profile and resultant effects on the intestinal barrier. Increased CKD, increased cardiovascular risk, uremic toxicity, and inflammation are associated with modifications to the microbiota and the subsequent diverse host responses (85). Experimental and clinical data from genomic and non-genomic studies have suggested that an unusual composition of microbiota exists in CKD (7), and preliminary results have suggested that the composition of the microbiota in chronic renal failure patients and early stages of CKD may be altered (87). More recently, the accumulation of uremic toxins was shown to be due to a number of microbiota strains. For example, the breakdown of tyrosine and phenylalanine by variety of obligate or non-obligate intestinal anaerobics such as the genera Bacteroides, Lactobacillus, Enterobacter, Bifidobacterium, and in particular Clostridio leads to the generation of p-cresyl from its conjugate p-cresyl sulfate (PCS) (88).
In 1965, Einheber and Carter found that anephric mice with an unaltered intestinal microbiome survived longer than anephric mice (85). There is now extensive evidence that the intestinal barrier is damaged in CKD, leading to the translocation of uremic toxins originating from bacteria and other noxious luminal products into the bloodstream, resulting in inflammation, and the activation of leukocytes. Gas chromatography studies in CKD rats and end-stage kidney disease (ESKD) patients showed significant changes in exhaled breath gases compared with those of healthy controls, further evidencing the altered gut microbe composition in these patients (89).
The gut microbiome may also secrete useful kidney-preserving metabolites such as short-chain fatty acids (SCFAs) (86), which can reduce CKD injuries. For example, in research by Wang et al., butyrate levels were approximately three times higher in the healthy controls than in CKD patients. They also showed that mice transplanted with fecal microbiota obtained from CKD subjects showed accelerated CKD progression, in the form of higher levels of TMAO, and that this could be reversed by additional butyrate supplementation (90). Thus, delayed CKD progression may be mediated SCFA produced by intestinal microbiota.
The accumulation of uremic toxins is related to the increased risk of CKD development. Many uremic toxins are derived from the absorption of nutrients by gut microbiota, as these nutrients enhance bacterial growth, which in turn produce more uremic toxins. Thus, possible treatments can involve diet modification, changes in microbiota, reducing the production of uremic toxins by microbiota, increasing the excretion of toxins or targeting the removal of certain uremic toxins (7). Of these, diet is the most powerful and easily controlled factor in intestinal microbiota modulation. To date, however, no research on the impact on the gut microbiota profile of CKD has been undertaken, and most related questions remain unanswered.
The bioactive compounds in foods like polyphenols, and their potential to modulate intestinal microbiota, have been given more recent attention. These compounds, which are powerful antioxidants and natural anti-inflammatory agents, are widely used to prevent inflammation and oxidative stress in chronic “burden of lifestyle” illnesses (91). Several studies have confirmed the capacity of probiotics to control gastrointestinal disease, and the success of treating CKD patients with Clostridium difficile infection should be paid particular attention in this regard (79). The findings of various studies, which have been based on a number of laboratory models, including in vitro experiments, in vivo experiments, and clinical studies, have reinforced the support for the health-promoting properties of polyphenols. Table 2 summarizes various types of polyphenol effects on CKD found in recent years.
Resveratrol treatments of the CRL-2573 rat kidney cell line and mesangial primary cells prevented the fibronectin expression and the proliferation of high glucose-induced mesangial cells, respectively (109). Resveratrol decreased atrophy in skeletal muscles in chronic muscle disease via the muscle-specific ring-finger protein 1 (MURF1) signaling pathway (92). Also, it significantly reduced creatinine-mediated interstitial damage in murine CKD models (93), with resveratrol-treated mice showing better renal function and increased albuminuria, and good histological test results.
In another example, the angiotensin II (Ang II)-angiotensin II type 1 receptor (AT1R) axis was suppressed and the function of the angiotensin II type 2 receptor (AT2R)–Ang 1-7-Mas receptor (MasR) axis was enhanced by resveratrol. Additionally, the expression of nicotinamide adenine dinucleotide phosphate oxidase 4, 8-hydroxy-2′-deoxyguanosine, 3-nitrotyrosine, collagen IV, and fibronectin were decreased, while the expression of endothelial nitric oxide synthase and superoxide dismutase 2 were increased following resveratrol treatment. Resveratrol can thus have protective effects on aging kidneys, via Ang II inhibition, and MasR activation leading to decreased oxidative stress, inflammation, and fibrosis (94).
Research also showed that resveratrol decreased in vitro LPS-induced inflammatory effects in kidney cells, and lead to the activation of nuclear factor E2-related factor 2 (Nrf2) signaling, resulting in nuclear Nrf2 accumulation, and increased expression of Nrf2 target genes heme oxygenase (HO)-1 and NAD(P)H dehydrogenase (quinone) 1 (NQO1). This was corroborated by the induction of the expression of HO-1 and NQO1 being observed to occur subsequent to in vitro and in vivo resveratrol treatment. It is worth noting that the knockdown of Nrf2 effectively abrogated the downregulation of TNF-α, IL-1β, and kidney injury molecule-1 (KIM-1) expression resulting from in vitro resveratrol treatment. These findings also indicated that resveratrol improved acute kidney damage from sepsis in a pediatric acute kidney injury (AKI) model via the Nrf2 signaling pathway (95). In vitro studies have also demonstrated that resveratrol increased cell viability, reduced NF-κB phosphorylation, and the production of inflammatory conditions in LPS and tunicamycin-induced HK-2 cells via the restriction of inositol-requiring enzyme 1 (IRE1) activation. Resveratrol administration also immediately protected against septic AKI by inhibiting IRE1-NF-κB pathway-triggered inflammatory reactions in the kidney. Resveratrol could be thus be a rapid and elegant treatment for septic disease (96).
In cultured human glomerular endothelial cells, anthocyanins prevented high glucose-induced oxidative stress, and apoptosis through activation of AMPK. These findings suggest that anthocyanins reduce diabetic nephropathy via the phosphorylation of AMPK, the major energy-sensing enzyme, with subsequent effects on its target molecules, which seem to inhibit lipotoxicity-related apoptosis and oxidative stress in the kidney (98).
When either H. sabdariffa aqueous extract or the anthocyanins isolated from it were used with adenine, the detrimental impact of adenine-induced CKD was remarkably reduced, and this was mostly in a dose-dependent manner. The beneficial effects were akin to those obtained by treatment with the ACE inhibitor lisinopril (99). PCE (anthocyanin-rich purple corn extract) reduced the mesangial and endothelial induction of angiopoietin (Angpt) proteins under hyperglycemic conditions (100), and the induction and activation of VEGF receptor 2 (VEGFR2) was inhibited by treating db/db mice with PCE. These results showed that PCE antagonized glomerular angiogenesis by disturbing the Angpt-Tie-2 ligand-receptor system connected to the renal VEGFR2 signaling pathway, due to chronic hyperglycemia and diabetes. PCE could therefore be a strong, abnormal angiogenesis therapeutic agent for diabetic nephropathy that causes renal disease (100).
Reductions in levels of antioxidant thiols, superoxide dismutase, and catalase have also been found in the renal tissues of cadmium-intoxicated rats. These alterations have often been associated with mitochondrial dysfunction, supported by an increase in the production of mitochondrial ROS and a decrease in mitochondrial membrane potential. These results indicated that, probably by means of its antioxidant, anti-inflammatory, and mitochondrial defense abilities, catechin effectively protected the kidney against cadmium toxicity (101). In another example, histopathology showed degenerative changes in glomeruli and tubules 2 weeks after administration of gentamicin to rats. These renal structural and functional defects have been correlated with renal oxidative stress in such rat models, as demonstrated by the substantial, measured reductions in renal glutathione (GSH).
Catechin hydrate therapy showed significant nephroprotective action in the prevention of renal structural and functional abnormalities, and oxidative stress, in rats with gentamicin-induced nephrotoxicity (102). Oxidation was much less pronounced in ammonium metavanadate (AMV)-treated animals who received EGCG, and antioxidant enzyme activity kept close to control values. There have been other, less noticeable histopathological shifts, and taken together with those detailed above, the findings confirmed that green tea and other sources of flavonoids could provide significant defense against oxidative stress caused by ammonium metavanadate (103). Pre-treatment with epicatechin ultimately inhibited upregulation and NOX activation of toll-like receptor 4 (TLR4) and subsequent downstream events, preventing the adverse effects of LPS, e.g., activation of the NF-κB pathway.
C-QA protects liver and kidney injury in mice caused by D-galactose, due to C-QA's antioxidation and anti-inflammation effects (110). C-QA also attenuates AKI caused by LPS by inhibiting the TLR4/NF-κB signaling pathway (107). The results of this study indicated that C-QA decreased LPS-induced kidney damage by suppressing oxidative stress, inflammation, apoptosis and autophagy, enhancing kidney regeneration (111). M2/M1 macrophage polarization modulation (112) increased the ability of quercetin to reduce kidney damage and fibrosis, and treatment with quercetin improved kidney function, reduced oxidation stress factors, serum fibroblast growth factor 23 (FGF23) levels, and renal inflammation in a rat model of adenine-induced chronic kidney disease (113). Quercetin also reduced kidney damage from doxorubicin (114). Histological findings showed that rutin administration significantly reduced the interstitial lesions of renal cells and removed the deposits of UUO collagen. However, in rats with obstructive nephropathy, rutin increased interstitial kidney fibrosis (108).
Antioxidant functions in lupus nephritis (LN) was evaluated in the context of CKD. In this study, a routine dose of EGCG enhanced renal function and decreased proteinuria in a mouse model of LN (115). Furthermore, serum, urine, and kidney oxidative stress parameters in the EGCG-treated mice were considerably reduced. In a mouse model of crescentic glomerulonephritis, the therapeutic potential of EGCG was also demonstrated. This research has shown that EGCG treatment can improve renal function can be and histopathology in kidney function-impaired mice (116). The treatment also regenerated Nrf2 and its downstream products, including a catalytic subset of glutamate-cysteine ligase, a subunit modification of glutamate-cysteine ligase, and glutathione peroxidase (GPx) (116). Funamoto et al. also reported that EGCG may have a renoprotective effect after cardiopulmonary bypass in diabetic rats (117). In related work, green tea supplementation prevented the growth of rat kidney-side crystals, decreased oxalate excretion, and had inhibitory effects on the activities of N-acetyl-β-D glucosaminidase (118).
The prevention of the adverse effects of high fructosis (10% w/v of fructose in drinking water) in male Sprague Dawley rats in rat chow diets in 8 weeks was also observed. Dietary supplementation with epicatechin ameliorated the following ill effects in fructose-fed rats: proteinuria, decreased nephrine, synaptopodin, and tumor transcription factor (TF1) of Wilmes levels in the renal cortex, and all podocyte dysfunction indicators associated with increased oxidative stress markers, changes in activity, and expression of non-synthase (NOS) and an increased inflammatory effect (119).
The intestinal microbiota has emerged as an important factor in the learning and control of the immune system, and has previously unknown functions in affecting the manifestations of many non-communicable diseases. Therefore, raising similar questions about the effects of the intestinal microbiota on CKD (120) seems logical and reasonable. In comparison, the effects of food and nutraceuticals on the uremic phenotype and its contribution to intestinal dysbiosis, given the enormous capacity of food to be used as medicine for CKD, warrant much greater attention (91).
As there is mounting evidence of the therapeutic effects of dietary polyphenols, an understanding of the in vivo absorption and metabolism of these compounds is increasingly important (12). In future years, meticulous analysis of these topics will be needed to exploit the potential of these research avenues.
NB finished the first draft of the manuscript. FC and DD critically revised the manuscript. All the authors approved the submission of the manuscript.
This work was supported by Science and Technology Research Project of Liaoning Provincial Education Department (LK201651).
The authors declare that the research was conducted in the absence of any commercial or financial relationships that could be construed as a potential conflict of interest.
1. Camara NO, Iseki K, Kramer H, Liu ZH, Sharma K. Kidney disease and obesity: epidemiology, mechanisms and treatment. Nat Rev Nephrol. (2017) 13:181–90. doi: 10.1038/nrneph.2016.191
2. Levey AS, Coresh J. Chronic kidney disease. Lancet. (2012) 379:165–80. doi: 10.1016/S0140-6736(11)60178-5
3. Delles C, Vanholder R. Chronic kidney disease. Clin Sci. (2017) 131:225–6. doi: 10.1042/CS20160624
4. Bao H, Peng A. The green tea polyphenol(-)-epigallocatechin-3-gallate and its beneficial roles in chronic kidney disease. J Transl Int Med. (2016) 4:99–103. doi: 10.1515/jtim-2016-0031
5. Valdes L, Cuervo A, Salazar N, Ruas-Madiedo P, Gueimonde M, Gonzalez S. The relationship between phenolic compounds from diet and microbiota: impact on human health. Food Funct. (2015) 6:2424–39. doi: 10.1039/C5FO00322A
6. Cigarran Guldris S, Gonzalez Parra E, Cases Amenos A. Gut microbiota in chronic kidney disease. Nefrologia. (2017) 37:9–19. doi: 10.1016/j.nefro.2016.05.008
7. Castillo-Rodriguez E, Fernandez-Prado R, Esteras R, Perez-Gomez MV, Gracia-Iguacel C, Fernandez-Fernandez B, et al. Impact of altered intestinal microbiota on chronic kidney disease progression. Toxins. (2018) 10:300. doi: 10.3390/toxins10070300
8. Duenas M, Munoz-Gonzalez I, Cueva C, Jimenez-Giron A, Sanchez-Patan F, Santos-Buelga C, et al. A survey of modulation of gut microbiota by dietary polyphenols. Biomed Res Int. (2015) 2015:850902. doi: 10.1155/2015/850902
9. Santhakumar AB, Battino M, Alvarez-Suarez JM. Dietary polyphenols: structures, bioavailability and protective effects against atherosclerosis. Food Chem Toxicol. (2018) 113:49–65. doi: 10.1016/j.fct.2018.01.022
10. Ding S, Jiang H, Fang J. Regulation of immune function by polyphenols. J Immunol Res. (2018) 2018:1264074. doi: 10.1155/2018/1264074
11. Ozdal T, Sela DA, Xiao J, Boyacioglu D, Chen F, Capanoglu E. The reciprocal interactions between polyphenols and gut microbiota and effects on bioaccessibility. Nutrients. (2016) 8:78. doi: 10.3390/nu8020078
12. D'Archivio M, Filesi C, Di Benedetto R, Gargiulo R, Giovannini C, Masella R. Polyphenols, dietary sources and bioavailability. Ann Ist Super Sanita. (2007) 43:348–61. Available online at: http://old.iss.it/publ/anna/2007/4/434348.pdf
13. Suresh D, Udayabhanu, Nethravathi PC, Lingaraju K, Rajanaika H, Sharma SC, et al. EGCG assisted green synthesis of ZnO nanopowders: Photodegradative, antimicrobial and antioxidant activities. Spectrochim Acta A Mol Biomol Spectrosc. (2015) 136(Pt C):1467–74. doi: 10.1016/j.saa.2014.10.038
14. Bohn T. Dietary factors affecting polyphenol bioavailability. Nutr Rev. (2014) 72:429–52. doi: 10.1111/nure.12114
15. Tomas-Barberan FA, Selma MV, Espin JC. Interactions of gut microbiota with dietary polyphenols and consequences to human health. Curr Opin Clin Nutr Metab Care. (2016) 19:471–6. doi: 10.1097/MCO.0000000000000314
16. Cardona F, Andres-Lacueva C, Tulipani S, Tinahones FJ, Queipo-Ortuno MI. Benefits of polyphenols on gut microbiota and implications in human health. J Nutr Biochem. (2013) 24:1415–22. doi: 10.1016/j.jnutbio.2013.05.001
17. Igwe EO, Charlton KE, Probst YC, Kent K, Netzel ME. A systematic literature review of the effect of anthocyanins on gut microbiota populations. J Hum Nutr Diet. (2019) 32:53–62. doi: 10.1111/jhn.12582
18. Medda R, Lyros O, Schmidt JL, Jovanovic N, Nie L, Link BJ, et al. Anti inflammatory and anti angiogenic effect of black raspberry extract on human esophageal and intestinal microvascular endothelial cells. Microvasc Res. (2015) 97:167–80. doi: 10.1016/j.mvr.2014.10.008
19. Biedermann L, Mwinyi J, Scharl M, Frei P, Zeitz J, Kullak-Ublick GA, et al. Bilberry ingestion improves disease activity in mild to moderate ulcerative colitis - an open pilot study. J Crohns Colitis. (2013) 7:271–9. doi: 10.1016/j.crohns.2012.07.010
20. Zhang C, Monk JM, Lu JT, Zarepoor L, Wu W, Liu R, et al. Cooked navy and black bean diets improve biomarkers of colon health and reduce inflammation during colitis. Br J Nutr. (2014) 111:1549–63. doi: 10.1017/S0007114513004352
21. Bibi S, Kang Y, Du M, Zhu MJ. Dietary red raspberries attenuate dextran sulfate sodium-induced acute colitis. J Nutr Biochem. (2018) 51:40–6. doi: 10.1016/j.jnutbio.2017.08.017
22. Shan Q, Zheng Y, Lu J, Zhang Z, Wu D, Fan S, et al. Purple sweet potato color ameliorates kidney damage via inhibiting oxidative stress mediated NLRP3 inflammasome activation in high fat diet mice. Food Chem Toxicol. (2014) 69:339–46. doi: 10.1016/j.fct.2014.04.033
23. Li S, Wu B, Fu W, Reddivari L. The anti-inflammatory effects of dietary anthocyanins against ulcerative colitis. Int J Mol Sci. (2019) 20:E2588. doi: 10.3390/ijms20102588
24. Morais CA, de Rosso VV, Estadella D, Pisani LP. Anthocyanins as inflammatory modulators and the role of the gut microbiota. J Nutr Biochem. (2016) 33:1–7. doi: 10.1016/j.jnutbio.2015.11.008
25. Cai ZY, Li XM, Liang JP, Xiang LP, Wang KR, Shi YL, et al. Bioavailability of tea catechins and its improvement. Molecules. (2018) 23:E2346. doi: 10.3390/molecules23092346
26. Reygaert WC. Green tea catechins: their use in treating and preventing infectious diseases. Biomed Res Int. (2018) 2018:9105261. doi: 10.1155/2018/9105261
27. Calland N, Sahuc ME, Belouzard S, Pene V, Bonnafous P, Mesalam AA, et al. Polyphenols inhibit hepatitis C virus entry by a new mechanism of action. J Virol. (2015) 89:10053–63. doi: 10.1128/JVI.01473-15
28. Marin L, Miguelez EM, Villar CJ, Lombo F. Bioavailability of dietary polyphenols and gut microbiota metabolism: antimicrobial properties. Biomed Res Int. (2015) 2015:905215. doi: 10.1155/2015/905215
29. Wang X, Ye T, Chen WJ, Lv Y, Hao Z, Chen J, et al. Structural shift of gut microbiota during chemo-preventive effects of epigallocatechin gallate on colorectal carcinogenesis in mice. World J Gastroenterol. (2017) 23:8128–39. doi: 10.3748/wjg.v23.i46.8128
30. Remely M, Ferk F, Sterneder S, Setayesh T, Roth S, Kepcija T, et al. EGCG prevents high fat diet-induced changes in gut microbiota, decreases of DNA strand breaks, and changes in expression and DNA methylation of Dnmt1 and MLH1 in C57BL/6J male mice. Oxid Med Cell Longev. (2017) 2017:3079148. doi: 10.1155/2017/3079148
31. Cremonini E, Wang Z, Bettaieb A, Adamo AM, Daveri E, Mills DA, et al. (-)-Epicatechin protects the intestinal barrier from high fat diet-induced permeabilization: implications for steatosis and insulin resistance. Redox Biol. (2018) 14:588–99. doi: 10.1016/j.redox.2017.11.002
32. Fan FY, Sang LX, Jiang M. Catechins and their therapeutic benefits to inflammatory bowel disease. Molecules. (2017) 22:E484. doi: 10.3390/molecules22030484
33. Bird JK, Raederstorff D, Weber P, Steinert RE. Cardiovascular and antiobesity effects of resveratrol mediated through the gut microbiota. Adv Nutr. (2017) 8:839–49. doi: 10.3945/an.117.016568
34. Hu Y, Chen D, Zheng P, Yu J, He J, Mao X, et al. The bidirectional interactions between resveratrol and gut microbiota: an insight into oxidative stress and inflammatory bowel disease therapy. Biomed Res Int. (2019) 2019:5403761. doi: 10.1155/2019/5403761
35. Zhang C, Zhao XH, Yang L, Chen XY, Jiang RS, Jin SH, et al. Resveratrol alleviates heat stress-induced impairment of intestinal morphology, microflora, and barrier integrity in broilers. Poult Sci. (2017) 96:4325–32. doi: 10.3382/ps/pex266
36. Zhou L, Xiao X, Zhang Q, Zheng J, Deng M. Deciphering the anti-obesity benefits of resveratrol: the “gut microbiota-adipose tissue” axis. Front Endocrinol. (2019) 10:413. doi: 10.3389/fendo.2019.00413
37. Fan Y, Chen H, Peng H, Huang F, Zhong J, Zhou J. Molecular mechanisms of curcumin renoprotection in experimental acute renal injury. Front Pharmacol. (2017) 8:912. doi: 10.3389/fphar.2017.00912
38. Bienholz A, Mae Pang R, Guberina H, Rauen U, Witzke O, Wilde B, et al. Resveratrol does not protect from ischemia-induced acute kidney injury in an in vivo rat model. Kidney Blood Press Res. (2017) 42:1090–103. doi: 10.1159/000485606
39. Etxeberria U, Arias N, Boque N, Macarulla MT, Portillo MP, Martinez JA, et al. Reshaping faecal gut microbiota composition by the intake of trans-resveratrol and quercetin in high-fat sucrose diet-fed rats. J Nutr Biochem. (2015) 26:651–60. doi: 10.1016/j.jnutbio.2015.01.002
40. Tomas-Barberan F, Garcia-Villalba R, Quartieri A, Raimondi S, Amaretti A, Leonardi A, et al. In vitro transformation of chlorogenic acid by human gut microbiota. Mol Nutr Food Res. (2014) 58:1122–31. doi: 10.1002/mnfr.201300441
41. Wang Z, Lam KL, Hu J, Ge S, Zhou A, Zheng B, et al. Chlorogenic acid alleviates obesity and modulates gut microbiota in high-fat-fed mice. Food Sci Nutr. (2019) 7:579–88. doi: 10.1002/fsn3.868
42. Cheng D, Li H, Zhou J, Wang S. Chlorogenic acid relieves lead-induced cognitive impairments and hepato-renal damage via regulating the dysbiosis of the gut microbiota in mice. Food Funct. (2019) 10:681–90. doi: 10.1039/C8FO01755G
43. Zhang Z, Wu X, Cao S, Cromie M, Shen Y, Feng Y, et al. Chlorogenic acid ameliorates experimental colitis by promoting growth of akkermansia in mice. Nutrients. (2017) 9:677. doi: 10.3390/nu9070677
44. Song J, Zhou N, Ma W, Gu X, Chen B, Zeng Y, et al. Modulation of gut microbiota by chlorogenic acid pretreatment on rats with adrenocorticotropic hormone induced depression-like behavior. Food Funct. (2019) 10:2947–57. doi: 10.1039/C8FO02599A
45. Duda-Chodak A, Tarko T, Satora P, Sroka P. Interaction of dietary compounds, especially polyphenols, with the intestinal microbiota: a review. Eur J Nutr. (2015) 54:325–41. doi: 10.1007/s00394-015-0852-y
46. Nagao-Kitamoto H, Kitamoto S, Kuffa P, Kamada N. Pathogenic role of the gut microbiota in gastrointestinal diseases. Intest Res. (2016) 14:127–38. doi: 10.5217/ir.2016.14.2.127
47. Wang K, Wan Z, Ou A, Liang X, Guo X, Zhang Z, et al. Monofloral honey from a medical plant, Prunella Vulgaris, protected against dextran sulfate sodium-induced ulcerative colitis via modulating gut microbial populations in rats. Food Funct. (2019) 10:3828–38. doi: 10.1039/C9FO00460B
48. Wang K, Jin X, Li Q, Sawaya A, Le Leu RK, Conlon MA, et al. Propolis from different geographic origins decreases intestinal inflammation and Bacteroides spp. populations in a model of DSS-induced colitis. Mol Nutr Food Res. (2018) 62:e1800080. doi: 10.1002/mnfr.201800080
49. Cockburn DW, Koropatkin NM. Polysaccharide degradation by the intestinal microbiota and its influence on human health and disease. J Mol Biol. (2016) 428:3230–52. doi: 10.1016/j.jmb.2016.06.021
50. Lee ES, Song EJ, Nam YD, Lee SY. Probiotics in human health and disease: from nutribiotics to pharmabiotics. J Microbiol. (2018) 56:773–82. doi: 10.1007/s12275-018-8293-y
51. Sanchez B, Delgado S, Blanco-Miguez A, Lourenco A, Gueimonde M, Margolles A. Probiotics, gut microbiota, and their influence on host health and disease. Mol Nutr Food Res. (2017) 61:1600240. doi: 10.1002/mnfr.201600240
52. Faria A, Fernandes I, Norberto S, Mateus N, Calhau C. Interplay between anthocyanins and gut microbiota. J Agric Food Chem. (2014) 62:6898–902. doi: 10.1021/jf501808a
53. Kataoka K. The intestinal microbiota and its role in human health and disease. J Med Invest. (2016) 63:27–37. doi: 10.2152/jmi.63.27
54. Sommer F, Anderson JM, Bharti R, Raes J, Rosenstiel P. The resilience of the intestinal microbiota influences health and disease. Nat Rev Microbiol. (2017) 15:630–8. doi: 10.1038/nrmicro.2017.58
55. Lynch SV, Pedersen O. The human intestinal microbiome in health and disease. N Engl J Med. (2016) 375:2369–79. doi: 10.1056/NEJMra1600266
56. Niemarkt HJ, De Meij TG, van Ganzewinkel CJ, de Boer NKH, Andriessen P, Hutten MC, et al. Necrotizing enterocolitis, gut microbiota, and brain development: role of the brain-gut axis. Neonatology. (2019) 115:423–31. doi: 10.1159/000497420
57. Singh RK, Chang HW, Yan D, Lee KM, Ucmak D, Wong K, et al. Influence of diet on the gut microbiome and implications for human health. J Transl Med. (2017) 15:73. doi: 10.1186/s12967-017-1175-y
58. Parkar SG, Trower TM, Stevenson DE. Fecal microbial metabolism of polyphenols and its effects on human gut microbiota. Anaerobe. (2013) 23:12–9. doi: 10.1016/j.anaerobe.2013.07.009
59. Magistrelli D, Zanchi R, Malagutti L, Galassi G, Canzi E, Rosi F. Effects of cocoa husk feeding on the composition of swine intestinal microbiota. J Agric Food Chem. (2016) 64:2046–52. doi: 10.1021/acs.jafc.5b05732
60. Hills RD Jr, Pontefract BA, Mishcon HR, Black CA, Sutton SC, Theberge CR. Gut microbiome: profound implications for diet and disease. Nutrients. (2019) 11:E1613. doi: 10.3390/nu11071613
61. Porras D, Nistal E, Martinez-Florez S, Gonzalez-Gallego J, Garcia-Mediavilla MV, Sanchez-Campos S. Intestinal microbiota modulation in obesity-related non-alcoholic fatty liver disease. Front Physiol. (2018) 9:1813. doi: 10.3389/fphys.2018.01813
62. Queipo-Ortuno MI, Boto-Ordonez M, Murri M, Gomez-Zumaquero JM, Clemente-Postigo M, Estruch R, et al. Influence of red wine polyphenols and ethanol on the gut microbiota ecology and biochemical biomarkers. Am J Clin Nutr. (2012) 95:1323–34. doi: 10.3945/ajcn.111.027847
63. Yayintas OT, Alpaslan D, Karagul Yuceer Y, Yilmaz S, Sahiner N. Chemical composition, antimicrobial, antioxidant and anthocyanin activities of mosses (Cinclidotus fontinaloides (Hedw.) P. Beauv. and Palustriella commutata (Hedw.) Ochyra) gathered from Turkey. Nat Prod Res. (2017) 31:2169–73. doi: 10.1080/14786419.2016.1277355
64. Abutheraa R, Hettiarachchy N, Kumar-Phillips G, Horax R, Chen P, Morawicki R, et al. Antimicrobial activities of phenolic extracts derived from seed coats of selected soybean varieties. J Food Sci. (2017) 82:731–7. doi: 10.1111/1750-3841.13644
65. Zhu Y, Sun H, He S, Lou Q, Yu M, Tang M, et al. Metabolism and prebiotics activity of anthocyanins from black rice (Oryza sativa L.) in vitro. PLoS ONE. (2018) 13:e0195754. doi: 10.1371/journal.pone.0195754
66. Sun H, Zhang P, Zhu Y, Lou Q, He S. Antioxidant and prebiotic activity of five peonidin-based anthocyanins extracted from purple sweet potato (Ipomoea batatas (L.) Lam.). Sci Rep. (2018) 8:5018. doi: 10.1038/s41598-018-23397-0
67. Chen L, Jiang B, Zhong C, Guo J, Zhang L, Mu T, et al. Chemoprevention of colorectal cancer by black raspberry anthocyanins involved the modulation of gut microbiota and SFRP2 demethylation. Carcinogenesis. (2018) 39:471–81. doi: 10.1093/carcin/bgy009
68. Paturi G, Butts CA, Monro JA, Hedderley D. Effects of blackcurrant and dietary fibers on large intestinal health biomarkers in rats. Plant Foods Hum Nutr. (2018) 73:54–60. doi: 10.1007/s11130-018-0652-7
69. Lee S, Keirsey KI, Kirkland R, Grunewald ZI, Fischer JG, de La Serre CB. Blueberry supplementation influences the gut microbiota, inflammation, and insulin resistance in high-fat-diet-fed rats. J Nutr. (2018) 148:209–19. doi: 10.1093/jn/nxx027
70. Pan P, Lam V, Salzman N, Huang YW, Yu J, Zhang J, et al. Black raspberries and their anthocyanin and fiber fractions alter the composition and diversity of gut microbiota in F-344 rats. Nutr Cancer. (2017) 69:943–51. doi: 10.1080/01635581.2017.1340491
71. Abe O, Ono T, Sato H, Muller F, Ogata H, Miura I, et al. Role of (-)-epigallocatechin gallate in the pharmacokinetic interaction between nadolol and green tea in healthy volunteers. Eur J Clin Pharmacol. (2018) 74:775–83. doi: 10.1007/s00228-018-2436-2
72. Luo J, Han L, Liu L, Gao L, Xue B, Wang Y, et al. Catechin supplemented in a FOS diet induces weight loss by altering cecal microbiota and gene expression of colonic epithelial cells. Food Funct. (2018) 9:2962–9. doi: 10.1039/C8FO00035B
73. Zhang Y, Zhao T, Deng J, Zhou X, Wu Z, Su Q, et al. Positive effects of the tea catechin (-)-epigallocatechin-3-gallate on gut bacteria and fitness of Ectropis obliqua Prout (Lepidoptera: Geometridae). Sci Rep. (2019) 9:5021. doi: 10.1038/s41598-019-41637-9
74. Calzada F, Juarez T, Garcia-Hernandez N, Valdes M, Avila O, Mulia LY, et al. Antiprotozoal, antibacterial and antidiarrheal properties from the flowers of chiranthodendron pentadactylon and isolated flavonoids. Pharmacogn Mag. (2017) 13:240–4. doi: 10.4103/0973-1296.204564
75. Abdul Qadir M, Shahzadi SK, Bashir A, Munir A, Shahzad S. Evaluation of phenolic compounds and antioxidant and antimicrobial activities of some common herbs. Int J Anal Chem. (2017) 2017:3475738. doi: 10.1155/2017/3475738
76. Gan Z, Wei W, Li Y, Wu J, Zhao Y, Zhang L, et al. Curcumin and resveratrol regulate intestinal bacteria and alleviate intestinal inflammation in weaned piglets. Molecules. (2019) 24:E1220. doi: 10.3390/molecules24071220
77. Kim BC, Kim JH. Role of rac GTPase in the nuclear signaling by EGF. FEBS Lett. (1997) 407:7–12. doi: 10.1016/S0014-5793(97)00289-5
78. Gaitonde DY, Cook DL, Rivera IM. Chronic kidney disease: detection and evaluation. Am Fam Physician. (2017) 96:776–83. Available online at: https://www.aafp.org/afp/2017/1215/p776.html
79. Koppe L, Mafra D, Fouque D. Probiotics and chronic kidney disease. Kidney Int. (2015) 88:958–66. doi: 10.1038/ki.2015.255
80. Kanbay M, Onal EM, Afsar B, Dagel T, Yerlikaya A, Covic A, et al. The crosstalk of gut microbiota and chronic kidney disease: role of inflammation, proteinuria, hypertension, and diabetes mellitus. Int Urol Nephrol. (2018) 50:1453–66. doi: 10.1007/s11255-018-1873-2
81. Misurac J. Chronic kidney disease in the neonate: etiologies, management, and outcomes. Semin Fetal Neonatal Med. (2017) 22:98–103. doi: 10.1016/j.siny.2016.09.003
82. Chelluboina B, Vemuganti R. Chronic kidney disease in the pathogenesis of acute ischemic stroke. J Cereb Blood Flow Metab. (2019) 39:1893–905. doi: 10.1177/0271678X19866733
83. Mahmoodpoor F, Rahbar Saadat Y, Barzegari A, Ardalan M, Zununi Vahed S. The impact of gut microbiota on kidney function and pathogenesis. Biomed Pharmacother. (2017) 93:412–9. doi: 10.1016/j.biopha.2017.06.066
84. Vaziri ND, Zhao YY, Pahl MV. Altered intestinal microbial flora and impaired epithelial barrier structure and function in CKD: the nature, mechanisms, consequences and potential treatment. Nephrol Dial Transplant. (2016) 31:737–46. doi: 10.1093/ndt/gfv095
85. Nallu A, Sharma S, Ramezani A, Muralidharan J, Raj D. Gut microbiome in chronic kidney disease: challenges and opportunities. Transl Res. (2017) 179:24–37. doi: 10.1016/j.trsl.2016.04.007
86. Wing MR, Patel SS, Ramezani A, Raj DS. Gut microbiome in chronic kidney disease. Exp Physiol. (2016) 101:471–7. doi: 10.1113/EP085283
87. Ramezani A, Massy ZA, Meijers B, Evenepoel P, Vanholder R, Raj DS. Role of the gut microbiome in uremia: a potential therapeutic target. Am J Kidney Dis. (2016) 67:483–98. doi: 10.1053/j.ajkd.2015.09.027
88. Briskey D, Tucker P, Johnson DW, Coombes JS. The role of the gastrointestinal tract and microbiota on uremic toxins and chronic kidney disease development. Clin Exp Nephrol. (2017) 21:7–15. doi: 10.1007/s10157-016-1255-y
89. Lau WL, Savoj J, Nakata MB, Vaziri ND. Altered microbiome in chronic kidney disease: systemic effects of gut-derived uremic toxins. Clin Sci. (2018) 132:509–22. doi: 10.1042/CS20171107
90. Wang S, Lv D, Jiang S, Jiang J, Liang M, Hou F, et al. Quantitative reduction in short-chain fatty acids, especially butyrate, contributes to the progression of chronic kidney disease. Clin Sci. (2019) 133:1857–70. doi: 10.1042/CS20190171
91. Mafra D, Borges N, Alvarenga L, Esgalhado M, Cardozo L, Lindholm B, et al. Dietary components that may influence the disturbed gut microbiota in chronic kidney disease. Nutrients. (2019) 11:496. doi: 10.3390/nu11030496
92. Sun LJ, Sun YN, Chen SJ, Liu S, Jiang GR. Resveratrol attenuates skeletal muscle atrophy induced by chronic kidney disease via MuRF1 signaling pathway. Biochem Biophys Res Commun. (2017) 487:83–9. doi: 10.1016/j.bbrc.2017.04.022
93. Lin YF, Lee YH, Hsu YH, Chen YJ, Lin YF, Cheng FY, et al. Resveratrol-loaded nanoparticles conjugated with kidney injury molecule-1 as a drug delivery system for potential use in chronic kidney disease. Nanomedicine. (2017) 12:2741–56. doi: 10.2217/nnm-2017-0256
94. Jang IA, Kim EN, Lim JH, Kim MY, Ban TH, Yoon HE, et al. Effects of resveratrol on the renin-angiotensin system in the aging kidney. Nutrients. (2018) 10:E1741. doi: 10.3390/nu10111741
95. Wang Y, Feng F, Liu M, Xue J, Huang H. Resveratrol ameliorates sepsis-induced acute kidney injury in a pediatric rat model via Nrf2 signaling pathway. Exp Ther Med. (2018) 16:3233–40. doi: 10.3892/etm.2018.6533
96. Wang N, Mao L, Yang L, Zou J, Liu K, Liu M, et al. Resveratrol protects against early polymicrobial sepsis-induced acute kidney injury through inhibiting endoplasmic reticulum stress-activated NF-kappaB pathway. Oncotarget. (2017) 8:36449–61. doi: 10.18632/oncotarget.16860
97. Turki K, Charradi K, Boukhalfa H, Belhaj M, Limam F, Aouani E. Grape seed powder improves renal failure of chronic kidney disease patients. EXCLI J. (2016) 15:424–33. doi: 10.17179/excli2016-363
98. Koh ES, Lim JH, Kim MY, Chung S, Shin SJ, Choi BS, et al. Anthocyanin-rich Seoritae extract ameliorates renal lipotoxicity via activation of AMP-activated protein kinase in diabetic mice. J Transl Med. (2015) 13:203. doi: 10.1186/s12967-015-0563-4
99. Ali BH, Cahlikova L, Opletal L, Karaca T, Manoj P, Ramkumar A, et al. Effect of aqueous extract and anthocyanins of calyces of Hibiscus sabdariffa (Malvaceae) in rats with adenine-induced chronic kidney disease. J Pharm Pharmacol. (2017) 69:1219–29. doi: 10.1111/jphp.12748
100. Kang MK, Lim SS, Lee JY, Yeo KM, Kang YH. Anthocyanin-rich purple corn extract inhibit diabetes-associated glomerular angiogenesis. PLoS ONE. (2013) 8:e79823. doi: 10.1371/journal.pone.0079823
101. Wongmekiat O, Peerapanyasut W, Kobroob A. Catechin supplementation prevents kidney damage in rats repeatedly exposed to cadmium through mitochondrial protection. Naunyn Schmiedebergs Arch Pharmacol. (2018) 391:385–94. doi: 10.1007/s00210-018-1468-6
102. Sardana A, Kalra S, Khanna D, Balakumar P. Nephroprotective effect of catechin on gentamicin-induced experimental nephrotoxicity. Clin Exp Nephrol. (2015) 19:178–84. doi: 10.1007/s10157-014-0980-3
103. Soussi A, Abdennabi R, Ghorbel F, Murat JC, El Feki AF. Ameliorated effects of (-)-epigallocatechin gallate against toxicity induced by vanadium in the kidneys of wistar rats. Biol Trace Elem Res. (2017) 180:239–45. doi: 10.1007/s12011-017-1004-4
104. Prince PD, Fischerman L, Toblli JE, Fraga CG, Galleano M. LPS-induced renal inflammation is prevented by (-)-epicatechin in rats. Redox Biol. (2017) 11:342–9. doi: 10.1016/j.redox.2016.12.023
105. Prince PD, Rodriguez Lanzi C, Fraga CG, Galleano M. Dietary (-)-epicatechin affects NF-kappaB activation and NADPH oxidases in the kidney cortex of high-fructose-fed rats. Food Funct. (2019) 10:26–32. doi: 10.1039/C8FO02230E
106. Sun W, Liu X, Zhang H, Song Y, Li T, Liu X, et al. Epigallocatechin gallate upregulates NRF2 to prevent diabetic nephropathy via disabling KEAP1. Free Radic Biol Med. (2017) 108:840–57. doi: 10.1016/j.freeradbiomed.2017.04.365
107. Ye HY, Jin J, Jin LW, Chen Y, Zhou ZH, Li ZY. Chlorogenic acid attenuates lipopolysaccharide-induced acute kidney injury by inhibiting TLR4/NF-kappaB signal pathway. Inflammation. (2017) 40:523–9. doi: 10.1007/s10753-016-0498-9
108. Wang B, Liu D, Zhu QH, Li M, Chen H, Guo Y, et al. Rutin ameliorates kidney interstitial fibrosis in rats with obstructive nephropathy. Int Immunopharmacol. (2016) 35:77–84. doi: 10.1016/j.intimp.2016.03.029
109. Den Hartogh DJ, Tsiani E. Health benefits of resveratrol in kidney disease: evidence from In vitro and in vivo studies. Nutrients. (2019) 11:E1624. doi: 10.3390/nu11071624
110. Feng Y, Yu YH, Wang ST, Ren J, Camer D, Hua YZ, et al. Chlorogenic acid protects D-galactose-induced liver and kidney injury via antioxidation and anti-inflammation effects in mice. Pharm Biol. (2016) 54:1027–34. doi: 10.3109/13880209.2015.1093510
111. Domitrovic R, Cvijanovic O, Susnic V, Katalinic N. Renoprotective mechanisms of chlorogenic acid in cisplatin-induced kidney injury. Toxicology. (2014) 324:98–107. doi: 10.1016/j.tox.2014.07.004
112. Lu H, Wu L, Liu L, Ruan Q, Zhang X, Hong W, et al. Quercetin ameliorates kidney injury and fibrosis by modulating M1/M2 macrophage polarization. Biochem Pharmacol. (2018) 154:203–12. doi: 10.1016/j.bcp.2018.05.007
113. Yang H, Song Y, Liang YN, Li R. Quercetin treatment improves renal function and protects the kidney in a rat model of adenine-induced chronic kidney disease. Med Sci Monit. (2018) 24:4760–6. doi: 10.12659/MSM.909259
114. Yagmurca M, Yasar Z, Bas O. Effects of quercetin on kidney injury induced by doxorubicin. Bratisl Lek Listy. (2015) 116:486–9. doi: 10.4149/BLL_2015_092
115. Tsai PY, Ka SM, Chang JM, Chen HC, Shui HA, Li CY, et al. Epigallocatechin-3-gallate prevents lupus nephritis development in mice via enhancing the Nrf2 antioxidant pathway and inhibiting NLRP3 inflammasome activation. Free Radic Biol Med. (2011) 51:744–54. doi: 10.1016/j.freeradbiomed.2011.05.016
116. Kanlaya R, Thongboonkerd V. Molecular mechanisms of epigallocatechin-3-gallate for prevention of chronic kidney disease and renal fibrosis: preclinical evidence. Curr Dev Nutr. (2019) 3:nzz101. doi: 10.1093/cdn/nzz101
117. Funamoto M, Masumoto H, Takaori K, Taki T, Setozaki S, Yamazaki K, et al. Green tea polyphenol prevents diabetic rats from acute kidney injury after cardiopulmonary bypass. Ann Thorac Surg. (2016) 101:1507–13. doi: 10.1016/j.athoracsur.2015.09.080
118. Nirumand MC, Hajialyani M, Rahimi R, Farzaei MH, Zingue S, Nabavi SM, et al. Dietary plants for the prevention and management of kidney stones: preclinical and clinical evidence and molecular mechanisms. Int J Mol Sci. (2018) 19:E765. doi: 10.3390/ijms19030765
119. Vargas F, Romecin P, Garcia-Guillen AI, Wangesteen R, Vargas-Tendero P, Paredes MD, et al. Flavonoids in kidney health and disease. Front Physiol. (2018) 9:394. doi: 10.3389/fphys.2018.00394
Keywords: polyphenols, gut health, chronic kidney disease, inflammation, intestinal microbiota
Citation: Bao N, Chen F and Dai D (2020) The Regulation of Host Intestinal Microbiota by Polyphenols in the Development and Prevention of Chronic Kidney Disease. Front. Immunol. 10:2981. doi: 10.3389/fimmu.2019.02981
Received: 28 October 2019; Accepted: 04 December 2019;
Published: 08 January 2020.
Edited by:
Kai Wang, Chinese Academy of Agricultural Sciences, ChinaReviewed by:
Lei Sun, Moffitt Cancer Center, United StatesCopyright © 2020 Bao, Chen and Dai. This is an open-access article distributed under the terms of the Creative Commons Attribution License (CC BY). The use, distribution or reproduction in other forums is permitted, provided the original author(s) and the copyright owner(s) are credited and that the original publication in this journal is cited, in accordance with accepted academic practice. No use, distribution or reproduction is permitted which does not comply with these terms.
*Correspondence: Di Dai, ZGlhbmFfZGRAMTI2LmNvbQ==
Disclaimer: All claims expressed in this article are solely those of the authors and do not necessarily represent those of their affiliated organizations, or those of the publisher, the editors and the reviewers. Any product that may be evaluated in this article or claim that may be made by its manufacturer is not guaranteed or endorsed by the publisher.
Research integrity at Frontiers
Learn more about the work of our research integrity team to safeguard the quality of each article we publish.