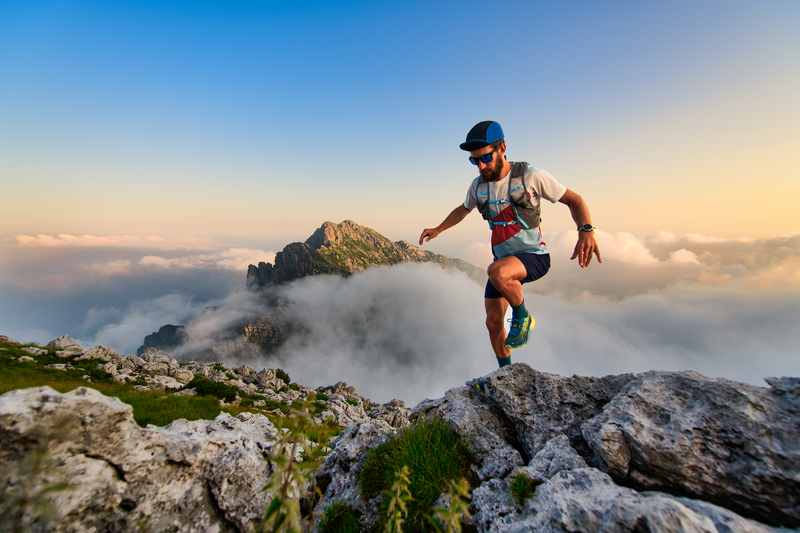
95% of researchers rate our articles as excellent or good
Learn more about the work of our research integrity team to safeguard the quality of each article we publish.
Find out more
MINI REVIEW article
Front. Immunol. , 11 December 2019
Sec. Antigen Presenting Cell Biology
Volume 10 - 2019 | https://doi.org/10.3389/fimmu.2019.02880
This article is part of the Research Topic Sentinel CLECs at Immunological Decision Nodes View all 9 articles
Phagocytic cells [dendritic cells (DCs), macrophages, monocytes, neutrophils, and mast cells] utilize C-type (Ca2+-dependent) lectin-like (CLEC) receptors to identify and internalize pathogens or danger signals. As monitors of environmental imbalances, CLEC receptors are particularly important in the function of DCs. Activation of the immune system requires, in sequence, presentation of antigen to the T cell receptor (TCR) by DCs, interaction of co-stimulatory factors such as CD40/80/86 on DCs with CD40L and CD28 on T cells, and production of IL-12 and/or IFN-α/β to amplify T cell differentiation and expansion. Without this sequence of events within an inflammatory environment, or in a different order, antigen-specific T cells become unresponsive, are deleted or become regulatory T cells. Thus, the mode by which CLEC receptors on DCs are engaged can either elicit activation of T cells to achieve an immune response or induce tolerance. This minireview illustrates these aspects with Dectin-1, DEC205, the mannose receptor and CLEC10A as examples.
Carbohydrate-recognition domains (CRDs), or more specifically C-type lectin-like domains (CTLDs), have evolved to generate a myriad of surface proteins that allow cells to monitor their environment and sense danger. The repertoire of glycan and non-glycan ligands that these receptors recognize is vast and internalization of the ligand begins the process of presentation of antigen to T cells and generation of an immune response. A striking feature of DCs is their 3- to 4-fold greater surface area to volume ratio than that of macrophages or lymphocytes, which provides a greater “sweep volume” for DCs to scan their environment (1). The larger surface area allows contact with ~5,000 T cells per hour, with an average contact duration of about 3 min. The minimum dwell time for TCR triggering is 2 s, and the half-life of contact between antigen-presenting cells (APCs) and T cells is 120 s, which is well within the 3-min window (2). The number of different CLEC receptors expressed by myeloid cells mark them as truly sentinels of the immune system (3–5). But these receptors can also cause DCs to become “tolerogenic” or induce unresponsiveness (anergy) in T cells (6, 7).
CLEC receptors, by forming clusters, distinguish between ligands of different sizes. Whereas, a single sugar generally binds with relatively low affinity (millimolar to micromolar range), multivalent ligands on the surface of pathogens bind with several orders of magnitude greater avidity (nanomolar to picomolar). Thus, the combination of CLEC receptors, which are often multimeric (8), and multivalent ligands provide a highly sensitive detection system. A revealing example of the role of these factors is recognition and discrimination of ß-glucans by Dectin-1 (CLEC7A, CD369) (9). Large ß-glucan polymers such as yeast cell walls induce formation of extensive clusters of Dectin-1 along the particle surface that exclude the cell membrane phosphatases, CD45 and CD148. Consequently, the hemi-immunoreceptor tyrosine-based activation motif (hemITAM, i.e., a single YxxL/F motif with an upstream triacidic sequence) in the cytoplasmic domain of Dectin-1 initiates signals through the kinases Src and Syk (9). This motif is also present in the cytoplasmic domain of other C-type lectin receptors (3, 4, 10) and is in contrast to the two immunoreceptor tyrosine-based activation motifs in tandem that describe an ITAM (YxxI/Lx6−12YxxI/L) (3, 4). The phagocytic process involves massive reorganization of cellular membranes, coordinated by the actin cytoskeleton (11), which also generates reactive oxygen species by activation of NADPH oxidase in the plasma membrane (12) to kill the pathogen. Digestive enzymes are inserted into an acidified endolysosomal system to degrade the invader, and degradation products are then presented in MHC class I or class II complexes on the cell surface. The extensive structural changes of the cytoskeleton and plasma membrane required for phagocytosis are dependent on an elevated cytosolic concentration of Ca2+ (13). In contrast, small, soluble oligosaccharides of ß-glucans such as laminarin bind only a few Dectin-1 molecules and result in a receptor cluster too small to exclude the phosphatases. Thus, phosphorylation of tyrosine in the hemITAMs is not sustained and signal transduction is minimized (9).
Phagocytic cells also recognize polymers such as mannan, a polysaccharide of mannose and glucose, that is present in the yeast cell wall and recognized by the mannose receptor C-type 1 (MRC1, MR, CLEC13D, CD206) (14, 15). MRC1 is a type I membrane protein that contains 8 CRDs in its external domain (15) and is expressed on macrophages and immature DCs (16–18). DCs that emerge under inflammatory conditions from monocytes (mo-DCs or infDCs) highly express MRC1 (19, 20). Glycoproteins that contain mannose, fucose, glucose, or N-acetyl glucosamine bind MRC1 (15) and are found in early endosomes after endocytosis (21). Whereas, MRC1 binds preferentially to single mannose residues, the type II protein receptor DC-SIGN [dendritic cell-specific intercellular adhesion molecule (ICAM)-3 grabbing non-integrin, CLEC4L, CD209] has a single CRD but the multimeric receptor preferentially binds high-mannose oligosaccharides (22). DC-SIGN is expressed by murine and human monocyte-derived immature and mature DCs (23–26) but not by DCs that differentiate from monocytes under the highly inflammatory conditions of ovarian ascites in humans (20). Other C-type, mannose-binding receptors are the type II receptors Dectin-2 (CLEC6A) and langerin (CLEC4K, CD207) (27), and C-type lectin DC immunoreceptor (DCIR, CLEC4A, CD367), which belongs to the Dectin-2 family and contains an immunoreceptor tyrosine-based inhibitory motif (ITIM) and sustains type I IFN (IFN-α/β) signaling through the transcriptional factor STAT3 (28).
Carcinoma cells richly express the Tn antigen (N-acetylgalactosamine [GalNAc]-OSer/Thr) (29, 30), which binds to the type II GalNAc-specific, C-type lectin domain family 10 member A (CLEC10A, CD301, also designated the human macrophage galactose-type lectin, hMGL, or simply MGL) on DCs and macrophages (31–33). Two forms of MGL were identified in the mouse, mMGL1 (CD301a), which preferentially binds ligands containing terminal galactose, and mMGL2 (CD301b), which is specific for GalNAc (34). Subcutaneous injection of the Tn antigen into patients bearing a carcinoma tumor causes a delayed hypersensitive response, indicative of the presence of Tn-specific T cells (30). However, the single sugar ligand, as the Tn antigen, binds with relatively low affinity (KD = 8–12 μM) to CLEC10A (33, 35). Linear glycopeptides (33, 35) or dendrimers built on a tri-lysine core (36–38), which contain 4–12 GalNAc residues, have KD values of 50–100 nM, an indication of the effect of multivalency. These larger structures induced a strong Tn-specific TH2 and B-cell anti-tumoral antibody response with secretion of IL-4, IL-5, IL-13, and IL-10 (38). Kurze et al. (39) showed that expression of the Tn antigen is induced by tamoxifen, oxidative stress, and DNA damage in breast cancer tissues, which enhanced binding of CLEC10A (40), and was associated with improved outcome and survival.
Ligand constructs that include the Tn antigen take advantage of the sugar as the specific natural ligand for CLEC10A (38). However, a short peptide sequence has been found that mimics GalNAc and binds to CLEC10A with much higher avidity (41). This peptide is effective in suppressing ovarian ascites, which is an inflammatory environment that recruits monocytes that differentiate into DCs that express CLEC10A (41–43). The response to treatment of ovarian ascites in mice with this peptide demonstrated that not only the properties of the ligand but also the tumor environment and frequency of dosing determine whether co-stimulatory factors continue to maintain activity of T cells or DCs initiate tolerance (41).
CLECs often function in combination with toll-like receptor-2 (TLR-2) for detection of pathogens (36, 44, 45), which forms heterodimers with TLR1 or TLR6 to bind lipoteichoic acids and lipoproteins in the pathogen cell wall. The cytoplasmic domain of TLR2 binds the adapter proteins TIRAP and MyD88 and activates a signal transduction pathway that leads to NF-κB and AP-1 and eventual phagocytosis (46–48). van Vliet et al. (36) and Heger et al. (49) found that antibodies against MGL (CLEC10A) on human DCs did not induce release of IL-10 or IL-12 but stimulated release of IL-10 and TNF-α upon addition of TLR ligands (Pam3CysK4 for TLR2, LPS for TLR4, or R848 for TLR7/8).
Subsets of human DCs express distinct patterns of CLEC receptors. Dectin-1, DEC205 (CLEC13B, LY75, CD205), and DCIR are highly expressed on CD141+, CD1c+, and CD16+ subtypes (50). As analyzed by single-cell RNA sequencing, CD141+ DCs (DC1) express, among other distinctive genes, the unique marker CLEC9A, whereas CD1c+ DCs (DC2A and DC2B) express the unique marker CLEC10A (49, 51, 52). Mo-DCs in the inflammatory ascites environment are DC2-type in which CLEC10A but not CLEC9A is expressed (42).
Immature DCs actively internalize surrounding material by “non-specific” macropinocytosis and “specific” receptor-mediated endocytosis and phagocytosis (53, 54). A specific “endocytosis” motif, the tyrosine-containing sequence YENFY in MGL (CLEC10A), YKSL in DC-SIGN, FENTLY in MRC1, and FSSVRY in DEC205 were identified in the cytoplasmic domain of several CLEC receptors that are similar to the hemITAM (15, 24, 55). As with other regulatory motifs, phosphorylation of the tyrosine residue in the motif is required for activity. Valladeau et al. (56) described an asialoglycoprotein receptor, homologous to the hepatic ASGPR-1 (57), that is expressed by DCs and designated DC-ASGPR. Similarly to CLEC10A, DC-ASGPR undergoes ligand-induced endocytosis with a t½ of 5–8 min at 37°C and delivers bound antibodies to early endosomes (55, 56, 58). The short isoform of DC-ASGPR is identical to the sequence of human CLEC10A, with the exception of a three-amino acid deletion near the base of the CRD (56). The long isoform has an insertion of 27 amino acids in the membrane proximal region of the extracellular CRD. Higashi et al. (25) described a total of seven isoforms of this receptor in humans, which are splicing variants from a single gene, and all contain the YENF endocytosis motif in the cytoplasmic domain. Overall endocytic activity decreases as DCs mature in response to inflammatory conditions (53, 54), including expression of MRC1 (54) and CLEC10A (49). However, expression of DEC205 was upregulated in the mouse and continued to capture antigens as DCs matured (54).
Within the cell, a second level of discrimination occurs. Internalization, degradation of the pathogen within the endosomal/lysosomal vacuolar pathway, and antigen presentation on the cell surface may require an extended period of time, depending on the ligand (21, 58–60). Large glycoproteins can be trapped in early endosomes and progress slowly through the vacuolar system for digestion and presentation on MHC class II complexes. In contrast, small glycopeptides enter the vacuolar system rapidly but also enter the cytosol and are cross-presented via MHC class I complexes to CD8+ T cells. Mo-DCs in inflamed tissues efficiently cross-present small antigens (10- and 26-mer peptides) to CD8+ T cells and stimulate CD8+ T cell proliferation and secretion of IFN-γ with the help of CD4+ T cells (42). A modified MUC1-derived glycopeptide bearing multiple Tn antigens was internalized by CLEC10A expressed by DCs and processed through MHC class I and II pathways but was still detectable in these compartments 24 h later (58). Similar to endocytosis by MRC1, a larger MUC1 glycoprotein was confined to the endosomal compartments and was not processed through the MHC pathways.
Although Dectin-1 is an exception (61), CLEC receptors generally require 2–4 Ca2+ ions in the CRD to bind a ligand (22, 33, 57). Endocytosis of these receptors therefore transfers bound Ca2+ into the endosome along with Ca2+ in extracellular fluid. The large concentration gradient of Ca2+ from ~1 to 2 mM outside the cell to 100 nM in the cytosol drives a flux across the endosomal membrane (32). As a “second messenger,” Ca2+ activates metabolic pathways through regulatory proteins (62–64). Activation of protein kinase C and calmodulin-regulated networks of kinases and phosphatases such as calmodulin-dependent kinase II and calcineurin occurs within minutes. The initial increase in Ca2+ may be augmented by activation of phospholipase C, which generates inositol trisphosphate, the signal for release of Ca2+ from the endoplasmic reticulum (65, 66).
In 1987 Jenkins and Schwartz presented evidence that antigen-specific T cells cultured in vitro with splenocytes, which were coupled to antigen through a carbodiimide derivative, became unresponsive within several hours (67). The effect appeared within 2 h, was essentially complete by 16 h and lasted more than a week. These results were confirmed with in vivo studies and indicated that the carbodiimide derivative inactivated a factor on the antigen-presenting cells that was required to sustain T cell activity. Some years later, Steinman and colleagues observed that when the C-type lectin receptor DEC205 on DCs in mice was engaged by subcutaneous injection of an anti-DEC205 antibody-antigen conjugate and T cells were isolated 2 days later and challenged with the antigen, activation of antigen-specific T cells was demonstrated by the release of IFN-γ and IL-2 and a proliferative response. However, when challenged 1 week later, T cells were unresponsive (17). Injection of an antibody agonist of CD40, a co-stimulatory protein expressed by DCs, sustained activation of T cells. Thus, in the absence of co-stimulation, presentation of antigen by “steady-state” or immature DCs led to transient activation of antigen-specific T cells followed by T cell deletion and anergy in surviving cells (17, 68).
The discovery of tolerance by delivery of antigens through DEC205 to immature DCs led to an extensive line of research into treatments for autoimmune diseases (69–71). In particular, when the myelin oligodendrocyte glycoprotein (MOG) was coupled to an antibody specific for DEC205 and injected intravenously into mice, the symptoms of experimental allergic encephalomyelitis (EAE), a model system for muscular dystrophy, were drastically suppressed (69). However, intravenous injection of MOG35−55, a major autoimmune epitope of the glycoprotein, alone also suppressed the symptoms of EAE (72). Definitive evidence for the role of DEC205 in EAE tolerance were experiments in which anti-DEC205/MOG was injected subcutaneously (17, 70) or intraperitoneally (71). This treatment also elevated the number of IL-10-secreting Treg cells, which was dependent on the transcription factor Hopx (71). Moreover, antigen-loaded DCs that migrate to draining lymph nodes have a superior ability to generate Treg cells to support the tolerogenic state (70, 73). Similar results were obtained in a mouse model of rheumatoid arthritis with a proteoglycan conjugated to an antibody against DEC205 (74).
DEC205 is a type 1 protein receptor that contains 10 CRDs (15) and has a short cytoplasmic tail with an endocytic motif similar to MRC1 (15, 24, 75). DEC205 does not bind a sugar but is a receptor for CpG-rich oligonucleotides (76, 77) The filamentous bacteriophage fd, whose single-stranded DNA is rich in CpG, binds to DEC205 and effectively delivers antigens to late endosomes or lysosomes (77). As with MRC1 (21), DEC205 recycles back to the cell surface within 1 h (75). In humans, mature DCs down-regulate MRC1 and DEC205-mediated endocytosis (54, 78). DEC205 is over-expressed in high-grade serous ovarian tumors as compared with low malignant potential tumors or normal tissues (79). A fully humanized monoclonal antibody against DEC205, when cross-linked to a cleavable maytansinoid derivative that disrupts microtubule function, targeted the receptor on tumor cells and was an effective anticancer agent (80).
An antibody against MRC1 on immature mo-DCs induced maturation of the cells as indicated by upregulation of CD80/83/86 but also increased secretion of IL-10 and decreased secretion of IL-12 (18). As with DEC205, T cells cultured with these DCs initially had a proliferative response but then became unresponsive to challenge. An aggregate of lipoarabinomannans from Mycobacterium bovis or M. tuberculosis, capped with mannose, bound to MRC1 and inhibited release of IL-12, a key factor in development of the TH1 response by T cells (81). MRC1 brings the cancer-related, highly glycosylated protein MUC1 into early endosomes when taken up by DCs but is recycled to the cell surface with some of the ligand still attached (21). MUC1 that dissociated from the receptor remained undegraded for more than 24 h within early endosomes (58). The inertness or slow processing of MUCI results when the sites of cleavage by lysosomal cathepsin L are blocked by glycosylation, and thus presentation to CD4+ T cells lags far behind the endocytic event (82). In apparent contrast, MUC1 coupled to mannan and then oxidized with sodium periodate to introduce aldehyde groups rapidly entered macrophages through MRC1, was processed in the cytosol and activated CD8+ T cells through the MHC class I pathway (83, 84).
Targeting DC-ASGPR (CLEC10A) on mo-DCs with antigen-conjugated antibodies induced antigen-specific CD4+ T cells to produce the cytokine, IL-10 (85). Intradermal injection of these conjugates into non-human primates also elevated IL-10 and reduced IFN-γ levels in blood. Further studies showed that binding of an antibody to DC-ASGPR induced maturation of DCs, indicated by expression of CD86, and activated a Src/Syk signal transduction pathway that includes PLCγ2, ERK1/2, p90RSK, and CREB, which led to expression of IL-10 (86). The increased secretion of IL-10 but decreased secretion of IFN-γ and IL-12 are indicative of a tolerogenic profile.
Within the reciprocal relationship between the anti-inflammatory IL-10 and pro-inflammatory IL-12 (87), IL-10 is produced by APCs that are activated by an increase in intracellular Ca2+ (88–92). Endocytosis of pathogens by CLECs expressed by these cells increased intracellular Ca2+, which can lead to expression of the IL10 gene via the signal transduction pathway described above by Gu et al. (86). Phosphorylation of the transcriptional factor CREB by calmodulin-dependent protein kinases mediates the response to Ca2+ and production of IL-10 (93). In contrast, IL12 expression is promoted by low intracellular Ca2+ concentrations and regulated by NF-κB (89, 94, 95).
Extensive evidence has been obtained that presentation by DCs of extrinsic antigen alone to T cells is insufficient to maintain T cell activity and leads to tolerance. Activation of T cells is induced by mature DCs, but either absence of a co-stimulatory signal or inability to process an antigen allows an inhibitory system in T cells to become dominant, which leads to a state of anergy (6, 7, 96). Heissmeyer et al. (97) showed that a sustained elevated Ca2+ signaling induces a state of unresponsiveness in T cells by calcineurin-mediated degradation of PLC γ1 and PKCθ. PLC γ1 activity leads to activation of NFAT, whereas PKCθ activity is required for activation of AP-1, which in combination with NFAT induces expression of activation genes (98). Without AP-1, NFAT locks in an inhibitory pattern of gene transcription (96–98). A more lengthy process, described as “exhaustion,” is associated with loss of effector T cell function and altered transcriptional patterns (99). Whereas, anergy is achieved within a few days, development of exhaustion requires weeks. The exhausted state can be overcome by checkpoint blockade (100).
Functional presentation of digestion products to T cells requires three signals. Sckisel et al. (101) stated that “primary T cell activation is tightly regulated and requires three signals in sequence: signal 1, where TCR recognition of cognate antigen in the context of major histocompatibility complex (MHC) restriction occurs; signal 2, involving binding of costimulatory molecules; and signal 3, where cytokine ‘instructions' direct and amplify T cell differentiation and expansion.” Low density (i.e., low concentrations or poor affinity) of an antigen can lead to anergy; higher concentrations of the antigen can maintain T cell activation (102). The inflammatory stimulus (signal 3) occurs via secretion by DCs of cytokines such as IL-12, the principal cytokine for a TH1 response, or type I interferon (IFN-α/β) (43, 103). Secretion of the type I interferons is an expression of danger signals emitted by stressed cells that provide the essential context for an immune response, without which T cells receive a tolerogenic signal from DCs (104–106). Tolerance that results from clonal deletion of T cells to which antigens are presented by “steady state” DCs is a major factor in prevention of autoimmunity (104).
A major characteristic of immature DCs is their capacity as sentinels to scan their environment, perform receptor-mediated endocytosis and phagocytosis, with subsequent maturation and interaction with T cells. CLEC receptors are intimately involved with instructions, described above, given to T cells that determine their course of action, whether destruction of tumor cells or, by different mechanisms, anergy or tolerance. Along with understanding conformational changes within the CRD induced by ligand binding (107), the signals that lead to these functional consequences are becoming clear.
Taylor and Drickamer (108) provided an up-to-date review of mammalian sugar-binding receptors. A complete catalog of CLEC receptors is at the web site for the Imperial College London1.
This manuscript was written by JH with editorial and literature assistance from LE and RC. All authors approved the version for submission.
This work was supported by Susavion Biosciences, Inc. The funder was not involved in the study design, collection, analysis, interpretation of data, the writing of this article or the decision to submit it for publication.
LE and JH are co-founders of Susavion Biosciences, Inc., and inventors of intellectual property that has been assigned to Susavion Biosciences, in which these authors hold shares. RC is an independent consultant for Susavion Biosciences.
1. ^http://www.imperial.ac.uk/research/animallectins/ctld/default.html
http://www.imperial.ac.uk/research/animallectins/ctld/mammals/domain.html
http://www.imperial.ac.uk/research/animallectins/ctld/mammals/mammals.html
1. Miller MJ, Hejazi AS, Wei SH, Cahalan MD, Parker I. T cell repertoire is promoted by dynamic dendritic cell behavior and random T cell motility in the lymph node. Proc Natl Acad Sci USA. (2004) 101:998–1003. doi: 10.1073/pnas.0306407101
2. Fernandes RA, Ganzinger KA, Tzou JC, Jönsson P, Lee SF, Palayret M, et al. A cell topography-based mechanism for ligand discrimination by the T cell receptor. Proc Natl Acad Sci USA. (2019) 116:14002–10. doi: 10.1073/pnas.1817255116
3. Sancho D, Reis e Sousa C. Signaling by myeloid C-type lectin receptors in immunity and homeostasis. Annu Rev Immunol. (2012) 30:491–529. doi: 10.1146/annurev-immunol-031210-101352
4. del Fresno C, Iborra S, Saz-Leal P, Martinez-López M, Sancho D. Flexible signaling of myeloid C-type lectin receptors in immunity and inflammation. Front Immunol. (2018) 9:804. doi: 10.3389/fimmu.2018.00804
5. Brown GD, Willment JA, Whitehead L. C-type lectins in immunity and homeostasis. Nat Rev Immunol. (2018) 18:374–389. doi: 10.1038/s41577-018-0004-8
6. Schwartz RH. T cell anergy. Annu Rev Immunol 21. (2003) 21:305–34. doi: 10.1146/annurev.immunol.21.120601.141110
7. Hasegawa H, Matsumoto T. Mechanisms of tolerance induction by dendritic cells in vivo. Front Immunol. (2018) 9:350. doi: 10.3389/fimmu.2018.00350
8. Jégouzo SAF, Quintero-Martinez A, Ouyang X, dos Santos Á, Taylor ME, Drickamer K. Organization of the extracellular portion of the macrophage galactose receptor: a trimeric cluster of simple binding sites for N-acetylgalactosamine. Glycobiology. (2013) 23:853–64. doi: 10.1093/glycob/cwt022
9. Goodridge HS, Reyes CN, Becker CA, Katsumoto TR, Ma J, Wolf AJ, et al. Activation of the innate immune receptor Dectin-1 upon formation of a ‘phagocytic synapse'. Nature. (2011) 472:471–6. doi: 10.1038/nature10071
10. Bauer B, Steinle A. HemITAM: a single tyrosine motif that packs a punch. Sci Signal. (2017) 10:eaan3676 doi: 10.1126/scisignal.aan3676
11. Tsai FC, Kuo GH, Chang SW, Tsai PJ. Ca2+ signaling in cytoskeletal reorganization, cell migration, and cancer metastasis. BioMed Res Int. (2015) 2015:409245. doi: 10.1155/2015/409245
12. Thomas DC. The phagocyte respiratory burst: historical perspectives and recent advances. Immunol Lett. (2017) 192:88–96. doi: 10.1016/j.imlet.2017.08.016
13. Nunes P, Demaurex N. The role of calcium signaling in phagocytosis. J Leukoc Biol. (2010) 88:57–68. doi: 10.1189/jlb.0110028
14. Hoving JC, Wilson GJ, Brown GD. Signaling C-type lectin receptors, microbial recognition and immunity. Cell Microbiol. (2014) 16:185–94. doi: 10.1111/cmi.12249
15. East L, Isacke CM. The mannose receptor family. Biochim Biophys Acta. (2002) 1572:364–86. doi: 10.1016/S0304-4165(02)00319-7
16. Allavena P, Chieppa M, Bianchi G, Solinas G, Fabbri M, Laskarin G, Montovani A. Engagement of the mannose receptor by tumoral mucins activates an immune suppressive phenotype in human tumor-associated macrophages. Clin Devel Immunol. (2010) 2010:547179. doi: 10.1155/2010/547179
17. Hawiger D, Inaba K, Dorsett Y, Guo M, Mahnke K, Rivera M, et al. Dendritic cells induce peripheral T cell unresponsiveness under steady state conditions in vivo. J Exp Med. (2001) 194:769–79. doi: 10.1084/jem.194.6.769
18. Chieppa M, Bianchi G, Doni A, Del Prete A, Sironi M, Laskarin G, et al. Cross-linking of the mannose receptor on monocyte-derived dendritic cells activates an anti-infammatory immunosuppressive program. J Immunol. (2003) 171:4552–60. doi: 10.4049/jimmunol.171.9.4552
19. Goudot C, Coillard A, Villani A-C, Gueguen P, Cros A, Sarkizova S, et al. Aryl hydrocarbon receptor controls monocyte differentiation into dendritic cells versus marophages. Immunity. (2017) 47:582–96. doi: 10.1016/j.immuni.2017.08.016
20. Segura E, Touzot M, Bohineust A, Cappuccio A, Chiocchia G, Hosmalin A, et al. Human inflammatory dendritic cells induce Th17 cell differentiation. Immunity. (2013) 38:336–48. doi: 10.1016/j.immuni.2012.10.018
21. Hiltbold EM, Vlad AM, Ciborowski P, Watkins SC, Finn OJ. The mechanism of unresponsiveness to circulating tumor antigen MUC1 is a block in intracellular sorting and processing by dendritic cells. J Immunol. (2000) 165:3730–41. doi: 10.4049/jimmunol.165.7.3730
22. Guo Y, Feinberg H, Conroy E, Mitchell DA, Alvarez R, Blixt O, et al. Structural basis for distinct ligand-binding and targeting properties of the receptors DC-SIGN and DC-SIGNR. Nat Struct Mol Biol. (2004) 11:591–8. doi: 10.1038/nsmb784
23. Geijtenbeek TBH, Torensma R, van Vliet SJ, van Duijnhoven GCF, Adema GJ, van Kooyk Y, et al. Identification of DC-SIGN, a novel dendritic cell-specific ICAM-3 receptor that supports primary immune responses. Cell. (2000) 100:575–85. doi: 10.1016/S0092-8674(00)80693-5
24. Engering A, Geijtenbeek TBH, van Vliet SJ, Wijers M, van Liempt E, Demaurex N, et al. The dendritic cell-specific adhesion receptor DC-SIGN internalizes antigen for presentation to T cells. J Immunol. (2002) 168:2118–26. doi: 10.4049/jimmunol.168.5.2118
25. Higashi N, Fujioka K, Denda-Nagai K, Hashimoto S, Nagai S, Sato T, et al. The macrophage C-type lectin specific for galactose/N-acetylgalactosamine is an endocytic receptor expressed on monocyte-derived immature dendritic cells. J Biol Chem. (2002) 277:20686–93. doi: 10.1074/jbc.M202104200
26. Cheong C, Matos I, Choi JH, Dandamudi DB, Shrestha E, Longhi MP, et al. Microbial stimulation fully differentiates monocytes to DC-SIGN/CD209+ dendritic cells for immune T cell areas. Cell. (2010) 143:418–29. doi: 10.1016/j.cell.2010.09.039
27. Hanske J, Aleksić S, Ballaschk M, Jurk M, Shanina E, Beerbaum M, et al. Intradomain allosteric network modulates calcium affinity of the C-type lectin receptor langerin. J Am Chem Soc. (2016) 138:12176–85. doi: 10.1021/jacs.6b05458
28. Troegeler A, Mercier I, Cougoule C, Pietretti D, Colom A, Duval C, et al. C-type lectin receptor DCIR modulates immunity to tuberculosis by sustaining type I interferon signaling in dendritic cells. Proc Natl Acad Sci USA. (2017) 114:E540–9. doi: 10.1073/pnas.1613254114
29. Springer GF. T and Tn, general carcinoma autoantigens. Science. (1984) 224:1198–206. doi: 10.1126/science.6729450
30. Springer GF. Immunoreactive T and Tn epitopes in cancer diagnosis, prognosis, and immunotherapy. J Mol Med. (1997) 75:594–602. doi: 10.1007/s001090050144
31. Suzuki N, Yamamoto K, Toyoshima S, Osawa T, Irimura T. Molecular cloning and expression of cDNA encoding human macrophage C-type lectin. J Immunol. (1996) 156:128–35.
32. Cote R, Eggink LL, Hoober JK. CLEC receptors, endocytosis and calcium signaling. AIMS Allerg Immunol. (2017) 1:207–31. doi: 10.3934/Allergy.2017.4.207
33. Iida S-i, Yamamoto K, Irimra T. Interaction of human macrophage C-type lectin with O-linked N-acetylgalactosamine residues on mucin glyopeptides. J Biol Chem. (1999) 274:10697–705. doi: 10.1074/jbc.274.16.10697
34. Tsuiji M, Fujimori M, Ohashi Y, Higashi N, Onami TM, Hedrick SM, Irimura T. Molecular cloning and characterization of a novel mouse macrophage C-type lectin, mMGL2, which has a distinct carbohydrate specificity from mMGL1. J Biol Chem. (2002) 277:28892–901. doi: 10.1074/jbc.M203774200
35. Marcelo F, Garcia-Martin F, Matsushita T, Sardinha J, Coelho H, Oude-Vrielink A, et al. Delineating binding modes of Gal/GalNAc and structural elements of the molecular recognition of tumor-associated mucin glycopeptides by the human macrophage galactose-type lectin. Chem Eur J. (2014) 20:16147–55. doi: 10.1002/chem.201404566
36. van Vliet CJ, Bay S, Vuist IM, Kalay H, Garcia-Vallejo JJ, Leclerc C, et al. MGL signaling augments TLR2-mediated responses for enhanced IL-10 and TNF-α secretion. J Leukoc Biol. (2013) 94:315–23. doi: 10.1189/jlb.1012520
37. Lo-Man R, Vichier-Guerre S, Bay S, Dériaud E, Cantacuzène D, Leclerc C. Anti-tumor immunity provided by a synthetic multiple antigenic glycopeptide displaying a tri-Tn glycotope. J Immunol. (2001) 166:2849–54. doi: 10.4049/jimmunol.166.4.2849
38. Freire T, Zhang X, Dériaud E, Ganneau C, Vichier-Guerre S, Azria E, et al. Glycosidic Tn-based vaccines targeting dermal dendritic cells favor germinal center B-cell development and potent antiibody response in the absence of adjuvant. Blood. (2010) 116:3526–36. doi: 10.1182/blood-2010-04-279133
39. Kurze AK, Buhs S, Eggert D, Oliveira-Ferrer L, Müller V, Niendorf A, et al. Immature O-glycans recognized by the macrophage glycoreceptor CLEC10A (MGL) are induced by 4-hydroxy-tamoxifen, oxidative stress and DNA-damage in breast cancer cells. Cell Commun Signal. (2019) 17:107. doi: 10.1186/s12964-019-0420-9
40. van Vliet S, Vuist IM, Lenos K, Tefsen B, Kalay H, Garcia-Vallejo JJ, van Kooyk Y. Human T Cell activation results in extracellular signal-regulated kinase (ERK)-calcineurin-dependent exposure of Tn antigen on the cell surface and binding of the macrophage galactose-type lectin (MGL). J Biol Chem. (2013) 288:27519–32. doi: 10.1074/jbc.M113.471045
41. Eggink LL, Roby KF, Cote R, Hoober JK. An innovative immunotherapeutic strategy for ovarian cancer: CLEC10A and glycomimetic peptides. J ImmunoTher Cancer. (2018) 6:28. doi: 10.1186/s40425-018-0339-5
42. Tang-Huau TL, Gueguen P, Goudot C, Durand M, Bohec M, Baulande S, et al. Human in vivo-generated monocyte-derived dendritic cells and macrophages cross-present antigens through a vacuolar pathway. Nat Commun. (2018) 9:2570. doi: 10.1038/s41467-018-04985-0
43. Tang-Huau TL, Segura E. Human in vivo-differentiated monocyte-derived dendritic cells. Sem Cell Devel Biol. (2019) 86:44–9. doi: 10.1016/j.semcdb.2018.02.018
44. Dimitriev BA, Toukach FV, Holst O, Rietschel ET, Ehlers S. Tertiary structure of Staphylococcus aureus cell wall murein. J Bacteriol. (2004) 186:7141–8. doi: 10.1128/JB.186.21.7141-7148.2004
45. Mnich M, van Dalen R, Gerlach D, Hendriks A, Xia G, Peschel A, et al. The C-type lectin receptor MGL senses N-acetylgalactosamine on the unique Staphylococcus aureus ST395 wall teichoic acid. Cell Microbiol. (2019) 21:e13072. doi: 10.1111/cmi.13072
46. Oliveira-Nascimento L, Massan P, Wetzler LM. The role of TLR2 in infection and immunity. Front Immunol. (2012) 3:79. doi: 10.3389/fimmu.2012.00079
47. Brown V, Brown RA, Ozinsky A, Hesselberth JR, Fields S. Binding specificity of Toll-like receptor cytoplasmic domains. Eur J Immunol. (2006) 36:742–53. doi: 10.1002/eji.200535158
48. Vidya MK, Kumar VG, Sejian V, Bagath M, Krishnan G, Bhatta R. Toll-like receptors: significance, ligands, signaling pathways, and functions in mammals. Int Rev Immunol. (2018) 37:20–36. doi: 10.1080/08830185.2017.1380200
49. Heger L, Balk S, Lühr JJ, Heidkamp GF, Lehmann CHK, Hatscher L, et al. CLEC10A is a specific marker for human CD1c+ dendritic cells and enhances their toll-like receptor 7/8-induced cytokine secretion. Front Immunol. (2018) 9:744. doi: 10.3389/fimmu.2018.00744
50. Lundberg K, Rydnert F, Greiff L, Lindstedt M. Human blood dendritic cell subsets exhibit discriminative pattern recognition receptor profiles. Immunology. (2014) 142:279–88. doi: 10.1111/imm.12252
51. Minoda Y, Virshup I, Rojas IL, Haigh O, Wong Y, Miles JJ, et al. Human CD141+ dendritic cell and CD1c+ dendritic cell undergo concordant early genetic programming after activation in humanized mice in vivo. Front Immunol. (2017) 8:1419. doi: 10.3389/fimmu.2017.01419
52. Villani A-C, Satija R, Reynolds G, Sarkisova S, Shekhar K, Fletcher J, et al. Single-cell RNA-seq reveals new types of human blood dendritic cells, monocytes, and progenitors. Science. (2017) 356:eaah4573. doi: 10.1126/science.aah4573
53. Garrett WS, Chen LM, Kroschewski R, Ebrsold M, Turley S, Trombetta S, et al. Developmental control of endocytosis in dendritic cells by Cdc42. Cell. (2000) 102:325–34. doi: 10.1016/S0092-8674(00)00038-6
54. Platt CD, Ma JK, Chalouni C, Ebersold M, Bou-Reslan H, Carano RAD, et al. Mature dendritic cells use endocytic receptors to capture and present antigens. Proc Natl Acad Sci USA. (2010) 107:4287–92. doi: 10.1073/pnas.0910609107
55. van Vliet SJ, Aarnoudse CA, Broks-van den Berg VCM, Boks M, Geijtenbeek TBH, van Kooyk Y. MGL-mediated internalization and antigen presentation by dendritic cells: a role for tyrosine-5. Eur J Immunol. (2007) 37:2075–81. doi: 10.1002/eji.200636838
56. Valladeau J, Duvert-Frances V, Pin JJ, Kleijmeer MJ, Ait-Yahia S, Ravel O, et al. Immature human dendritic cells express asialoglycoprotein receptor isoforms for efficient receptor-mediated endocytosis. J Immunol. (2001) 167:5767–74. doi: 10.4049/jimmunol.167.10.5767
57. Meier M, Bider MD, Malashkevich VN, Spiess M, Burkhard P. Crystal structure of the carbohydrate recognition domain of the H1 subunit of the asialoglycoprotein reeptor. J Mol Biol. (2000) 300:857–65. doi: 10.1006/jmbi.2000.3853
58. Napoletano C, Rughettu A, Tarp MPA, Coleman J, Bennett EP, Picco G, et al. Tumor-associated Tn-MUC1 glycoform is internalized through the macrophage galactose-type C-type lectin and delivered to the HLA class I and II compartments in dendritic cells. Cancer Res. (2007) 67:8358–67. doi: 10.1158/0008-5472.CAN-07-1035
59. Napoletano C, Zizzari IG, Rughetti A, Rahimi H, Irimura T, Clausen H, et al. Targeting of macrophage galactose-type C-type lectin (MGL) induces DC signaling and activation. Eur J Immunol. (2012) 42:936–45. doi: 10.1002/eji.201142086
60. Zizzari IG, Napoletano C, Battisti F, Rahimi H, Caponnetto S, Pierelli L, et al. MGL receptor and immunity: when the ligand can make a difference. J Immunol Res. (2015) 2015:450695. doi: 10.1155/2015/450695
61. Brown J, O'Callaghan CA, Marshall ASJ, Gilbert RJC, Siebold C, Gordon S, et al. Structure of the fungal β-glucan-binding immune receptor dectin-1: implications for function. Prot Sci. (2017) 16:1042–52. doi: 10.1110/ps.072791207
62. Linse S, Helmersson A, Forsén S. Calcium binding to calmodulin and its globular domains. J Biol Chem. (1991) 266:8050–4.
63. Carafoli E, Krebs J. Why calcium? How calcium became the best communicator. J Biol Chem. (2016) 291:20849–57. doi: 10.1074/jbc.R116.735894
64. Shumilina E, Huber SM, Lang F. Ca2+ signaling in the regulation of dendritic cell functions. Am J Physiol Cell Physiol. (2011) 300:C1205–14. doi: 10.1152/ajpcell.00039.2011
65. Putney JW, Tomita T. Phospholipase C signaling and calcium influx. Adv Enz Reg. (2012) 52:152–64. doi: 10.1016/j.advenzreg.2011.09.005
66. Krebs J, Agellon LB, Michalak M. Ca2+ homeostasis and endoplasmic reticulum. (ER) stress: an integrated view of calcium signaling. Biochem Biophys Res Commun. (2015) 460:114–21. doi: 10.1016/j.bbrc.2015.02.004
67. Jenkins MK, Schwartz RH. Antigen presentation by chemically modified splenocytes induces antigen-specific T cell unresponsiveness in vitro and in vivo. J Exp Med. (1987) 165:302–19. doi: 10.1084/jem.165.2.302
68. Liu K, Iyoda T, Saternus M, Kimura Y, Inaba K, Steinman RM. Immune tolerance after delivery of dying cells to dendritic cells in situ. J Exp Med. (2002) 196:1091–7. doi: 10.1084/jem.20021215
69. Ring S, Maas M, Nettelbek DM, Enk AH, Mahnke K. Targeting of autoantigens to DEC205+ dendritic cells in vivo suppress experimental allergic encephalomyelitis in mice. J Immunol. (2013) 191:2938–47. doi: 10.4049/jimmunol.1202592
70. Idoyaga J, Fiorese C, Zbytnulk L, Lubkin A, Miller J, Malissen B, et al. Specialized role of migratory dendritic cells in peripheral tolerance induction. J Clin Invest. (2013) 123:844–54. doi: 10.1172/JCI65260
71. Jones A, Opejin A, Henderson JG, Gross C, Jain R, Epstein JA, et al. Peripherally induced tolerance depends on peripheral regulatory T cells that require Hopx to inhibit intrinsic IL-2 expression. J Immunol. (2015) 195:1489–97. doi: 10.4049/jimmunol.1500174
72. Li H, Zhang G-X, Chen Y, Xu H, Fizgerald DC, Zhao Z, Rostami A. CD11c+CD11b+ dendritic cells play an important role in intravenous tolerance and the suppression of experimental autoimmune encephalomyelitis. J Immunol. (2008) 181:2483–93. doi: 10.4049/jimmunol.181.4.2483
73. Mahnke K, Ring S, Enk AH. Antibody targeting of “steady-state” dendritic cells induces tolerance mediated by regulatory T cells. Front Immunol. (2016) 7:63. doi: 10.3389/fimmu.2016.00063
74. Spierling R, Margry B, Keijzer C, Petzold C, Hoek A, Wagenaar-Hilbers J, et al. DEC205+ dendritic cell-targeted tolerogenic vaccination promotes immune tolerance in experimental autoimmune arthritis. J Immunol. (2015) 194:4804–13. doi: 10.4049/jimmunol.1400986
75. Mahnke K, Guo M, Lee S, Sepulveda H, Swain SL, Nussenzweig M, et al. The dendritic cell receptor for endocytosis, DEC-205, can recycle and enhance antigen presenation via major histocompatibility complex class II-positive lysosomal compartments. J Cell Biol. (2000) 151:673–83. doi: 10.1083/jcb.151.3.673
76. Lahoud MH, Ahmet F, Zhang JG, Meuter S, Policheni AN, Kitsoulis S, et al. DEC-205 is a cell surface receptor for CpG oligonucleotides. Proc Natl Acad Sci USA. (2012) 109:16270–5. doi: 10.1073/pnas.1208796109
77. Sartorius R, D'Apice L, Trovato M, Cuccaro F, Costa V, De Leo MG, et al. Antigen delivery by filamentous bacteriophage fd displaying an anti-DEC-205 single-chain variable fragment confers adjuvanticity by triggering an TLR9-mediated immune response. EMBO Mol Med. (2015) 7:973–88. doi: 10.15252/emmm.201404525
78. Butler M, Morel AS, Jordan WJ, Eren E, Hue S, Shrimpton RE, et al. Altered expression and endocytic function of CD205 in human dendritic cells, and detection of a CD205—DCL-1 fusion protein upon dendritic cell maturation. Immunology. (2006) 120:362–71. doi: 10.1111/j.1365-2567.2006.02512.x
79. Faddaoui A, Bachvarova M, Plante M, Gregoire J, Renaud M-C, Sebastianelli A, et al. The mannose receptor LY75 (DEC205/CD205) modulates cellular phenotype and metastatic potential of ovarian cancer cells. Oncotarget. (2016) 7:14125–42. doi: 10.18632/oncotarget.7288
80. Merlino G, Fiascarelli A, Bigioni M, Bressan A, Carrisi C, Bellarosa D, et al. MEN1309/OBT076, a first-in-class antibody-drug conjugate targeting CD205 in solid tumors. Mol Cancer Ther. (2019) 18:1533–43. doi: 10.1158/1535-7163.MCT-18-0624
81. Nigou J, Zelle-Rieser C, Gilleron M, Thurnher M, Puzo G. Mannosylated lipoarabinomannans inhibit IL-12 production by human dendritic cells: evidence for a negative signal delivered through the mannose receptor. J Immunol. (2001) 166:7477–85. doi: 10.4049/jimmunol.166.12.7477
82. Hanisch FG, Schwientek T, von Bergwelt-Baildon, Schultze JL, Finn O. O-Linked glycans control glycoprotein processing by antigen-presenting cells: a biochemical approach to the molecular aspects of MUC1 processing by dendritic cells. Eur J Immunol. (2003) 33:3242–54. doi: 10.1002/eji.200324189
83. Apostolopoulos V, Pietersz GA, Gordon S, Martinez-Pomares L, McKenzie IFC. Aldehyde-mannan antigen comlexes target the MHC class I antigen-presentation pathway. Eur J Immunol. (2000) 30:1714–23 https://onlinelibrary.wiley.com/doi/abs/10.1002/1521-4141%28200006%2930%3A6%3C1714%3A%3AAID-IMMU1714%3E3.0.CO%3B2-C
84. Apostolopoulos V, Thalhammer T, Tzakos AG, Stojanovska L. Targeting antigens to dendritic cell receptors for vaccine development. J Drug Deliv. (2013) 2013:869718. doi: 10.1155/2013/869718
85. Li D, Romain G, Flamar AL, Duluc D, Dullaers M, Li XH, et al. Targeting self- and foreign antigens to dendritic cells via DC-ASGPR generates IL-10—producing suppressive CD4+ T cells. J Exp Med. (2012) 209:109–21. doi: 10.1084/jem.20110399
86. Gu C, Wang L, Zurawski S, Oh SK. Signaling cascade through DC-ASGPR induces transcriptionally active CREB for IL-10 induction and immune regulation. J Immunol. (2019) 203:389–99. doi: 10.4049/jimmunol.1900289
87. Ma X, Yan W, Zheng H, Du Q, Zhang L, Ban Y, et al. Regulation of IL-10 and IL-12 production and function in macrophages and dendritic cells [version 1; peer review: 3 approved]. F1000Research. (2015) 4:F1000 Faculty Rev-1465. doi: 10.12688/f1000research.7010.1
88. Lehmann MH, Berg H. Interleukin-10 expression is induced by increase of intracellular calcium levels in the monocytic cell line U937. Pflügers Archiv. (1998) 435:868–70. doi: 10.1007/s004240050596
89. Liu X, Wang N, Zhu Y, Yang Y, Chen X, Fan S, et al. Inhibition of extracellular calcium influx results in enhanced IL-12 production in LPS-treated murine macrophages by downregulation of the CaMKKβ-AMPK-SIRT1 signaling pathway. Med Inflamm. (2016) 2016: 6152713. doi: 10.1155/2016/6152713
90. Rafiq K, Charitidou L, Bullens DMA, Kasran A, Lorré K, Ceuppens JL, et al. Regulation of the IL-10 production by human T cells. Scand J Immunol. (2001) 53:139–47. doi: 10.1046/j.1365-3083.2001.00851.x
91. Kelly EK, Wang L, Ivashkiv LB. Calcium-activated pathways and oxidative burst mediate zymosan-induced signaling and IL-10 production in human macrophages. J Immunol. (2010) 184:5545–52. doi: 10.4049/jimmunol.0901293
92. Boubali S, Liopeta K, Virgillo L, Thyphronitis G, Mavrothallassitis G, Dimitracopoulos G, et al. Calcium/calmodulin-dependent protein kinase II regulates IL-10 production by human T lymphocytes: a distinct target in the calcium dependent pathway. Mol Immunol. (2012) 52:51–60. doi: 10.1016/j.molimm.2012.04.008
93. Sheng M, Thompson MA, Greenberg ME. CREB: a Ca2+-regulated transcription factor phosphorylated by calmodulin-dependent kinases. Science. (1991) 252:1427–30. doi: 10.1126/science.1646483
94. Moeller T, Wolfheimer S, Goretzki A, Scheurer S, Schülke S. NF-κB- and MAP-kinase signaling contribute to the activation of murine myeloid dendritic cells by a flagellin A:allergen fusion protein. Cells. (2019) 8:E355. doi: 10.3390/cells8040355
95. Schülke S. Induction of interleukin-10 producing dendritic cells as a tool to suppress allergen-specific T helper 2 responses. Front Immunol. (2018) 9:455. doi: 10.3389/fimmu.2018.00455
96. Hogan PG. Calcium-NFAT transcriptional signalling in T cell activation and T cell exhaustion. Cell Calcium. (2017) 63:66–69. doi: 10.1016/j.ceca.2017.01.014
97. Heissmeyer V, Macián F, Im SH, Varma R, Feske S, Venuprasad K, et al. Calcineurin imposes T cell unresponsiveness through targeted proteolysis of signaling proteins. Nat Immunol. (2004) 5:255–65. doi: 10.1038/ni1047
98. Powell JD. The induction and maintenance of T cell anergy. Clin Immunol. (2006) 120:239–46. doi: 10.1016/j.clim.2006.02.004
99. Wherry EJ, Kurachi M. Molecular and cellular insights into T cell exhaustion. Nat Rev Immunol. (2015) 15:486–99. doi: 10.1038/nri3862
100. Saeidi A, Zandi K, Cheok YY, Saeidi H, Wong WF, Lee CYQ, et al. T-cell exhaustion in chronic infections: reversing the state of exhaustion and reinvigorating optimal protective immune responses. Front Immunol. (2018) 9:2569. doi: 10.3389/fimmu.2018.02569
101. Sckisel GD, Bouchlaka MN, Monjazeb AM, Crittenden M, Curti BD, Wilkins DEC, et al. Out-of-sequence signal 3 paralyzes primary CD4+ T-cell-dependent immunity. Immunity. (2015) 43:240–50. doi: 10.1016/j.immuni.2015.06.023
102. Mirshahidi S, Huang CT, Sadegh-Nasser S. Anergy in peripheral memory CD4+ T cells induced by low avidity engagement of T cell receptor. J Exp Med. (2001) 194:719–31. doi: 10.1084/jem.194.6.719
103. Keppler SJ, Rosenits K, Koegl T, Vucikuja S, Aichele P. Signal 3 cytokines as modulators of primary immune responses during infectionss: the interplay of type I IFN and IL-12 in CD8 T cell responses. PLoS ONE. (2012) 7:e40865. doi: 10.1371/journal.pone.0040865
104. Steinman RM, Hawiger D, Liu K, Bonifaz L, Bonnyay D, Mahnke K, et al. Dendritic cell function in vivo during the steady state: a role in peripheral tolerance. Ann NY Acad Sci. (2003) 987:15–25. doi: 10.1111/j.1749-6632.2003.tb06029.x
105. Pradeu T, Cooper EL. The danger theory: 20 years later. Front Immunol. (2012) 3:287. doi: 10.3389/fimmu.2012.00287
106. Ramadan A, Land WG, Paczesny S. Editorial: danger signals triggering immune response and inflammation. Front Immunol. (2017) 8:979. doi: 10.3389/fimmu.2017.00979
107. Diniz A, Coelho H, Dias JS, van Vliet SJ, Jiménez-Barbero J, Corzana F, et al. The plasticity of carbohydrate recognition domain dictates the exquisite mechanism of binding of human macrophage galactose-type lectin. Chemistry. (2019) 25:13945–55. doi: 10.1002/chem.201902780
Keywords: CLEC10A, DEC205, dectin-1, mannose receptor, endocytosis, calcium, immunosuppression, anergy
Citation: Hoober JK, Eggink LL and Cote R (2019) Stories From the Dendritic Cell Guardhouse. Front. Immunol. 10:2880. doi: 10.3389/fimmu.2019.02880
Received: 24 September 2019; Accepted: 25 November 2019;
Published: 11 December 2019.
Edited by:
Irina Caminschi, Monash University, AustraliaReviewed by:
Ken Shortman, Walter and Eliza Hall Institute of Medical Research, AustraliaCopyright © 2019 Hoober, Eggink and Cote. This is an open-access article distributed under the terms of the Creative Commons Attribution License (CC BY). The use, distribution or reproduction in other forums is permitted, provided the original author(s) and the copyright owner(s) are credited and that the original publication in this journal is cited, in accordance with accepted academic practice. No use, distribution or reproduction is permitted which does not comply with these terms.
*Correspondence: J. Kenneth Hoober, amtob29iZXJAc3VzYXZpb24uY29t
Disclaimer: All claims expressed in this article are solely those of the authors and do not necessarily represent those of their affiliated organizations, or those of the publisher, the editors and the reviewers. Any product that may be evaluated in this article or claim that may be made by its manufacturer is not guaranteed or endorsed by the publisher.
Research integrity at Frontiers
Learn more about the work of our research integrity team to safeguard the quality of each article we publish.