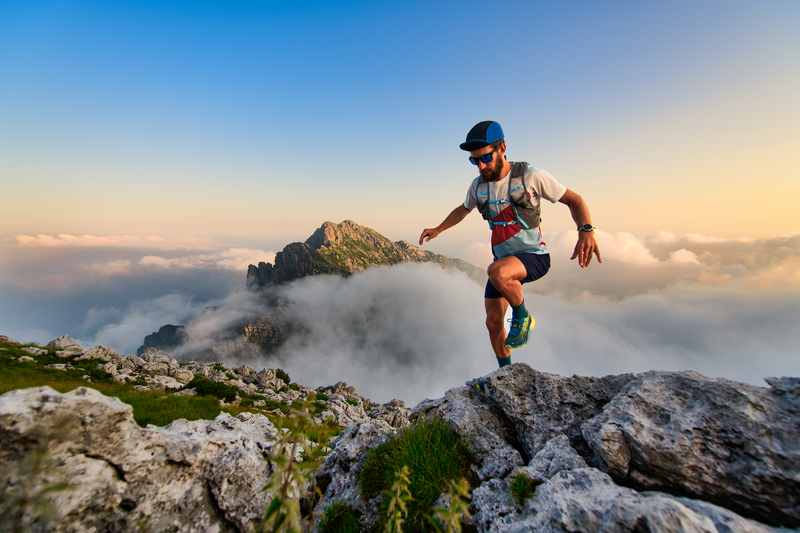
94% of researchers rate our articles as excellent or good
Learn more about the work of our research integrity team to safeguard the quality of each article we publish.
Find out more
REVIEW article
Front. Immunol. , 23 October 2019
Sec. Cancer Immunity and Immunotherapy
Volume 10 - 2019 | https://doi.org/10.3389/fimmu.2019.02457
Head and neck squamous cell carcinomas (HNSCCs) are closely linked with immunosuppression, accompanied by complex immune cell functional activities. The abnormal competition between costimulatory and coinhibitory signal molecules plays an important role in the malignant progression of HNSCC. This review will summarize the features of costimulatory molecules (including CD137, OX40 as well as CD40) and coinhibitory molecules (including CTLA-4, PD-1, LAG3, and TIM3), analyze the underlying mechanism behind these molecules' regulation of the progression of HNSCC, and introduce the clinic application. Vaccines, such as those targeting STING while working synergistically with monoclonal antibodies, are also discussed. A deep understanding of the tumor immune landscape will help find new and improved tumor immunotherapy for HNSCC.
The head and neck squamous cell carcinoma (HNSCC) is the sixth most common systemic malignant tumor (1, 2). Its occurrence is closely related to exposure to tobacco, alcohol (3) and HPV infection (4). The clinical outcomes of HNSCC are always frustrating and the 5-year survival rate of early HNSCC is only 40–60%. For patients with local recurrence or/and distant metastasis, the median survival time after palliative chemotherapy is 6–9 months, while only 3–6 months for chemotherapy tolerance (2). Thus, the question of how to improve the poor prognosis of HNSCC has attracted great attention, and there is a need for further study into the molecular mechanisms of tumor growth and metastasis.
Previous studies have demonstrated that almost all types of tumors, including HNSCC, have the ability to evade the immune surveillance and clearance, resulting in tumor growth and metastasis. In an immunosuppressive tumor microenvironment (TME), there are several suppressive cytokines and chemokines, such as IL-10, TGF-β, VEGF, and PGE2, as well as negative regulatory cells, including myeloid-derived suppressor cell (MDSC), regulatory T cell (Treg) and so on. In this negative TME, the function of some effector immune cells, such as T cells and NK cells, are suppressed in various direct or indirect ways, resulting in tumor growth and metastasis (5). One such way is aimed at effector cell activation and involves the costimulatory and coinhibitory signal molecules on the surface of the immune cells.
Costimulatory molecules as a second signal for T cell activation could promote the secretion of many cytokines and the expression of their corresponding receptor molecules, which participate in the activation and proliferation of T cells as well as the induction of T cells to differentiate them into different functional subgroups (6–8). For example, CD28 on T cells binding with B7 on an antigen-presenting cell (APC) surface exert their immune function. On the other hand, coinhibitory molecules, such as CTLA-4 and PD-1, expressed on the surface of activated T cells could play the role of the inhibitor of T cells function via a suppression signal. Therefore, the interaction between the costimulatory and coinhibitory signal molecules directly affects the function of immune cells (9, 10). And the same signal molecules on the surface of different immune cells or tumor cells play different functions, leading to a more complex TME. This review will describe the common costimulatory and coinhibitory signal molecules in HNSCC and analyze the underlying mechanism of these molecules in regulating the malignant progression of HNSCC, and it will also introduce the clinic application of mAbs for costimulatory and coinhibitory signal molecules (Figure 1).
Figure 1. Normal activation of T cells requires costimulatory and coinhibitory signal molecules. The activation of T cells means firstly that T cells recognize the antigen presented by APC, and secondly that the costimulatory molecule B7 on the APC surface binds to the CD28 presenting on the surface of T cells to provide the second signal for the activation of T cells, which makes T cells secrete IL-2 and express high levels of IL-2R, thus promoting the proliferation and activation of T cells. Meanwhile, a variety of costimulatory and coinhibitory molecules are expressed successively on the surface of T cells: costimulatory signals further promote T cell proliferation and coinhibitory signals limit T cell activation and proliferation, which regulate T cell moderate activation accurately, effectively start the immune response, and prevent excessive immune damage to the tissue.
Costimulatory signals offer the requisite second signal for immune cell activation and proliferation. Most activated T cells could play an anti-tumor role in inhibiting tumor growth (11). However, the activation of regulatory T cell (Tregs) could negatively regulate T cell function and promote tumor growth in most types of cancer. Many costimulatory signaling molecules on the surface of immune cells, including CD137, OX40 and CD40, have now been found to play a vital role in HNSCC development (12–14).
CD137 is a member of the tumor necrosis factor receptor (TNFR) families and has been regarded as a costimulatory signal receptor (12). CD137 is expressed on the surface of activated T cells, DCs and NK cells (15–17). CD137, activated by its ligand CD137L, conveys polyubiquitination-mediated signals via TNF receptor associated factor 2 and activates the NF-κB pathway that inhibits apoptosis, as well as enhances proliferation and effector functions of T cell and NK cell (18, 19). Activated CD137 could enhance the antibody dependent cell-mediated cytotoxicity (ADCC) effect through the use of NK cells (20), promote the differentiation of effector T cells, inhibit the function of Tregs and facilitate T cells to play an anti-tumor function (21). When NK cells encounter tumor cells, the expression of CD137 on NK cells increases significantly (22), helping NK cell clean tumor cells by ADCC. Besides, DC cells activated with CD137 could not only secrete IL-2 and IL-6 to promote the proliferation of T cells (23) but also activate cytotoxic lymphocytes (CTLs) and promote the secretion of IFN, hereby enhancing the anti-tumor effect (24). However, previous experiments indicated that soluble CD137 (sCD137), secreted by tumor cells in a low oxygen environment, blocked its membrane-CD137 costimulatory function, resulting in tumor escape (25). How tumor cells increase sCD137 secretion in hypoxic conditions remains unclear.
Based on the physiological effects of CD137 against tumors, Lucido et al. found that agonists for CD137 in the HPV (+) HNSCC mouse model had a synergistic effect on inhibiting tumor growth compared to traditional single radiotherapy and chemotherapy. Meanwhile, CD137L on the surface of tumor cells could also improve the efficacy of chemotherapy/radiation through the CD137/CD137L signal axis (26). Srivastava et al. showed that the use of CD137 agonists in HNSCC patients promoted the maturation of DC cells induced by cetuximab, and it also enhanced the cross-presentation function of NK cells and DC cells to HNSCC antigens. Besides, urelumab, agonistic mAbs (monoclonal antibodies) of CD137, helped inhibit the apoptosis of NK cells, playing an anti-tumor effect (27).
Two agonistic mAbs of CD137, urelumab (BMS-663513) and PF-05082566, have been developed for clinical use. Urelumab alone has a very severe hepatotoxicity and its exact mechanism is still unclear (28, 29). On the other hand, CD137 agonist mAbs could enhance other mAbs' efficacy in HNSCC patients, such as cetuximab (27). Thus, it may have an enhanced anti-tumor effect and could weaken hepatotoxicity through reducing anti-CD137 mAbs' dosage when combined with other mAbs.
OX40, one of the tumor necrosis factor receptors, is mainly expressed on the surface of activated T cells, especially on CD4+ T cells (30), while the expression on CD8+T cells is low (31). What's more, OX40 is highly expressed on intratumoral T cells, particularly the FoxP3+ regulatory T-cell (Treg) lineage (32, 33). In general, the ligand of OX40 (OX40L) on the surface of DCs or MCs binds directly to OX40. Besides, bone marrow mononuclear cells (BMMCs) and some DCs could secrete exosomes containing OX40L, which remotely regulate T cell differentiation (34, 35).
OX40 activation could augment the downstream signaling of TCR mainly through the PI3-K/PKB pathway, accounting for T cell division, survival and cytokine production. Meanwhile, OX40 activated in conjunction with TCR signaling could increase calcium influx, promote nuclear factor of activated T cells (NFAT) activation and enhance several cytokines secretion, such as IL-2, IL-4, IL-5, and IFN-γ. These cytokines help promote the proliferation and differentiation of immune cells and exert anti-tumor effects. Therefore, the signaling downstream of OX40 can augment proliferation, suppress apoptosis and induce greater cytokine responses from T cells (36).
An experiment on skin squamous carcinomas in vitro observed that there were more OX40 + Tregs in tumor tissues than in peripheral tissues, which could inhibit the function of effector T cells and the secretion of IFN-γ. Stimulated OX40 was found to not only obviously suppress the inhibition conducted by Tregs but also reduce the number of Tregs in tumor microenvironments by activating FccRs, finally inhibiting tumor growth (32–36). However, some studies showed that OX40-stimulated Tregs by agonist mAbs retained suppressive qualities, and Tregs function had not intrinsically been impaired. The expression of IFN-γ, TNF-α, and granzyme B, which had potent anti-tumor effects, was increased significantly, and this may provide another explanation for the mechanism of OX40 (37).
OX40 could be expressed on the surface of T cells in HNSCC patients (38). Recent studies have found that the expression of OX40 on CD4(+) T cell surfaces in HNSCC patients was lower than in healthy people. Compared to patients with early tumors, the level of OX40 expressed on the CD4+ T cell surface was significantly decreased in patients with advanced tumors (39). In HNSCC, the low expression of OX40L could not help secrete adequate cytokines with anti-tumor effects (40).
A series of pre-clinical experiments have shown that anti-OX40 dose-tolerant mAb could enhance the humoral and cellular immunity of cancer patients by amplifying the effector T cells and inhibiting the function of Tregs (41, 42). In a mouse ovarian tumor, the combined application of anti-PD-1/OX40 mAb had greatly improved the anti-tumor effect (43). Besides, Gough, et al. showed that, in tumor animal models, the overall survival could be effectively improved from 50% to 100% by combining anti-OX40 therapies after complete surgery or radiotherapy (44). It indicated that OX-40 mAbs could play a synergistic role with traditional treatment (45), which provided a new promising combination treatment for HNSCC patients.
CD40 is a costimulatory receptor molecule on the surface of APCs (DCs), monocytes and tumor cells. CD154, the ligand of CD40, is generally expressed on the surface of T cells and some innate immune cells, such as activated DCs and NK cells (46). Circulating sCD40L was higher in tumor patients, which may have a predictive role and could be an ambiguous therapeutic target (47). Binding with its ligand CD154, CD40 without enzymatic activity in the cytoplasmic domain recruits and interacts with TNF-receptor-associated factors (TRAFs), promoting the activation of the NF-κB signaling to maintain homeostasis and immunogenic pathogenic processes (48, 49). The activation of the CD40/CD154 axis results in the secretion of cytokine, transformation of immunoglobulin gene, prevention of B-cell apoptosis, increased expression of costimulatory molecules such as CD80 and CD86, formation of germinal center, production of high-affinity antibodies and formation of B memory cells (50). Furthermore, a combination of CD40/CD154 could promote antigen presentation, help effector T cells exert their role, activate mononuclear cells and down-regulate the expression of inhibitory molecules, such as PD-1 (15).
Stimulated CD40 could play a direct role in killing tumor cells (51). CD40 agonists promoted the secretion of lL-12 and reduced the expression of PD-1 on the surface of CD8+ T cells (52). Besides, anti-CD40 mAb treatment reversed phenotypic T cell exhaustion and increased the sensitivity of mAbs against anti-PD1 refractory tumors (53). In mouse tumor models, high expression of CD40/CD154 had an anti-tumor effect, and a low level of CD40/CD154 was shown to promote tumor growth. A possible explanation for this was that the former was related to IL-12, while the latter was associated with IL-10 (54–56).
As for HNSCC patients with tumor high stage, the expression of CD40 on APCs as well as tumor cells decreased, and the same applies the level of CD154 on T cells, while soluble CD40 increased in body fluids, representing a state of reduced immunity. During the whole process, the proportion of IL-12 did not change much while the content of IL-10 increased, showing an overall favorable environment for tumor growth (57). Moreover, the activation of CD40 was beneficial to the secretion of VEGF, which promoted the formation of tumor blood vessels and the growth of tumors (58).
In a study of phase III and IV of esophageal squamous cell carcinomas, the survival rate of CD40+ tumor patients was significantly lower compared with CD40– tumor patients. Besides, CD40+ tumor patients performed poorer in terms of pathological stage, distant metastasis and clinical prognosis (59). CD40+ tumor cells interacting with CD154+ activated T cells promoted the secretion of TGF and the differentiation of Th17, which contributed to the proliferation of tumor cells. Activated by CD154 or IFN-γ, the CD40 pathway in tumor cells induced the production of IL-6, promoting the progression of a variety of tumors (59, 60). However, several studies reported that stimulated CD40 may help protect bladder cancer cells from apoptosis. However, the low expression of CD40 in HNSCC was not associated with tumor cell growth. Therefore, the relationship between the expression of CD40 and tumor growth may be related to tumor types (61, 62).
So far, CD40 agonist antibodies (SGN-40, CP-870,893) are being tested in early clinical trials either alone or in combination with mAbs for lymphoma and solid tumors in humans (63). However, considering the side-effect of CD40 agonists, such as potential toxicity due to autoimmune reactions, severe cytokine release syndrome (CRS), hyper-immune stimulation syndrome leading to AICD, thromboembolic disease and tumor proliferation or angiogenesis, the use of CD40 agonist antibodies is still limited and need much more study to verify their availability.
The surface of immune cells will also express coinhibitory molecules such as CTLA-4, PD-1, LAG3, TIM, and KIR. Modest activation of coinhibitory molecules under normal conditions coordinates the immune response and avoids excessive immunity injury. However, when the suppressive signals overexpress in the TME, the function of T cells could be inhibited, and tumor cells could get the chance to immune escape (64–70).
Stimulated by antigens or B7, CTLA-4 is expressed mainly on the surface of T cells, especially Tregs (71, 72). In a normal immune response, CTLA-4 can combine its ligand B7 with stronger affinity than CD28 does (64), resulting in two different mechanisms for T cell suppression, the rapid inhibition of T cell activation and the induction anergy of T cells. The first mechanism depends on inhibiting Akt directly by activating phosphatase PP2A, and the latter aims to replace CD28 binding with B7 (73, 74). sCTLA-4(soluble CTLA-4), mostly derived from Tregs, could play the same immunosuppression role by binding with B7 on APCs, like membrane-CTLA-4, to inhibit T-cell response. The blocking of sCTLA-4 also activated the proliferation of CD8+ and CD4+ T cells and promoted the secretion of cytokines (75).
In HNSCC patients, the expression of CTLA-4 in tumor-infiltrated lymphocytes was significantly higher than that in peripheral lymphocytes (76). In laryngeal squamous cell carcinoma, CD8+ lymphocytes showed higher expression of CTLA-4 (77). Besides, HNSCC, esophageal squamous cell carcinoma and nasopharyngeal cancer (NPC) patients with a higher expression of CTLA-4 had a worse prognosis to those with lower CTLA-4 level (78–80). It may suggest that anti-CTLA-4 mAbs could be a promising therapeutic target for cancers.
In HNSCC, CTLA-4 on Tregs had a stronger inhibitory effect on the proliferation of CD4+T cells compared with cyclic Tregs (81). The expression of CTLA-4 on CD4 (+) FOXP3 (+) Tregs in the circulation and TME increased in HNSCC patients treated with cetuximab. FOXP3(+) CTLA4(+) suppressor cells might suppress the activation of NK cells in oral squamous cell carcinomas (71). Furthermore, by using an anti-CTLA-4 antibody (ipilimumab), the inhibition capacity of Tregs to NK cells was found to be weakened (81, 82). However, the exact inhibitory mechanism between Tregs and CTLA-4 is still unclear. Some scholars believe that Tregs could achieve immunosuppression through CTLA-4 (63, 83), while other figures do not support this view (84). Currently, the preferred school of thought is that both of them play independent inhibitory roles in tumor immunity, but the inhibitory effects are overlapped (85).
Some CTLA4-blocking mAbs, such as ipilimumab and tremelimumab, are under study for further clinical importance. Ipilimumab has now been approved for the treatment of advanced melanoma by the Food and Drug Administration of America (FDA). Ipilimumab has shown astonishingly positive effects in the treatment of a variety of malignant tumors due to its synergistic effect with chemotherapy and radiotherapy (86–88). There is a new viewpoint indicating that anti-CTLA-4 antibodies induce tumor recession by the selective depletion of Tregs in tumors rather than the blocking of B7-CTLA-4 interaction in lymphoid organs (89).
PD-1 expresses on the surface of activated immune cells, such as CD4+T cells, CD8+T cells, B cells, natural killer T cells, activated monocytes, dendritic cells and macrophages (90, 91). sPD-1(soluble PD-1), interacting with PD-L1, could prevent PD-1 from binding with PD-L1 and promote effective tumor immunity, possibly resulting from decreased IL-10, TGF-β and increased IL-2 TNF-α and IFN-γ (92, 93). However, a different phenomenon had taken place: sPD-1 could inhibit T cell proliferation and IL-2 production when DCs and T cells were cocultured with sPD-1 (94).
The ligands of PD-1 are PD-L1 and PD-L2. PD-L1 expresses mainly on T cells, B cells, DCs and macrophages (95), while on some tumors surface, such as glioblastoma multiforme, NSCLC and some hematologic malignancies (96, 97). PD-L1 mRNA and proteins were up-regulated by the effect of IFN-γ, IL-4, IL-10, growth cell stem factors, LPS and VEGF (96, 98), which indicated that PD-L1 overexpression may be accompanied by immune inhibition in TME. On the other hand, the expression of PD-L1 on tumor cells could also be increased by activating intracellular signaling pathways, such as IFN-γ/JAK2/IFN, ALK/STAT3, PI3K and MEK/ERK/STAT1 (99, 100). Hypoxia-inducible factor-1 (HIF-1α) is an important factor for making tumor cells over-express PD-L1 (101). Another important source of PD-L1 is tumor exosomes, which can suppress the draining lymph node activation, inhibit IFN-γ secretion, promote immune escape and facilitate tumor growth (102, 103). sPD-L1 binding with membrane-PD-1 could also exert a wide range of inhibitory effects through the blood and lymphatic circulation (104).
Phosphorylation of the tyrosine residues in the ITIM and ITSM motifs in the cytoplasmic tail of PD-1 recruits SHP-1 and SHP-2, which, in turn, dephosphorylate proximal signaling molecules downstream of the TCR and CD28 and inhibit the activation of the PI3K/Akt and the Ras/MEK/Erk pathway (105). Hence, T cell proliferation activity, cytokine secretion capacity and cytotoxic effects are weakened, and tumor cells finally get immune tolerance (67, 68).
In HNSCC patients, PD-1 on the surface of tumor infiltrating CD8+ T cells had a higher expression, which resulted in impaired function of PD-1(+) CD8(+) T cells and facilitated tumor growth (106). In the HNSCC microenvironment, the percentage of tumor cells expressing PD-L1 was about 50–60% (107, 108). In a survey of 74 cases of primary HNSCC, Roper et al. suggested that the expression of PD-L1 was higher on tumor cells and TILs, while individual higher expression of PD - L1 (>5%) on primary tumor cells, primary TILs, and metastatic TILs was associated with longer diseases-free survival (109). Previous studies have found that higher expression of PD-L1 in gastric, breast, renal and pancreatic cancer led to poorer prognosis (110–113). However, in metastatic melanoma, Merkel cell carcinoma, HPV-associated HNSCC, mismatch-repair-proficient colorectal cancer, NSCLC and small cell lung cancer, higher expression of PD-L1 indicated a better prognosis (68, 114–118). The possible explanation may be that the expression of PD-L1 on the latter tumor cells could be induced by IFN-γ in a local inflammatory tumor microenvironment (114). In HNSCC, epithelial-mesenchymal transformation (EMT) could independently up-regulate the expression of PD-L1 on tumor cells. Compared with EMT without PD-L1 expression, the prognosis of patients with EMT-related PD-L1 expression was poorer (107). In HNSCC patients, PD-L1 levels on exosomes were associated with disease progression. The emergence of circulating PD-L1+ exosomes may be a useful metric for disease and immune activity in these patients (119).
According to the immunosuppressive function of PD-1/PD-L1, more and more blocking monoclonal antibodies have been studied and applied in clinical practice. The phase I, II, and III clinical studies have all showed that, in recurrent or metastatic HNSCC, pembrolizumab demonstrated clinically meaningful anti-tumor activity and took on a favorable safety profile (120–122).
LAG3, an inhibitory checkpoint receptor, expresses on the activated CD4+T cells (69), CD8+T cells and a subset of natural killer (NK) cells (123). For CD4+T cells, LAG3 binds to MHC II molecules with an affinity higher than CD40, while most of the molecular mechanisms remain unclear (124). For CD8+T cells and NK cells, the ligand of LAG3 is LSECtin (125). Binding to LAG3 expressing on CD8+T cells and NK cells, tumor cells could get the capacity to escape immune clearance. Besides, Tr1 cells could be identified in both humans and mice by the expression of LAG3 together with CD49b (126). But it has not been confirmed whether LAG3 is necessary for the immunosuppressive function of Tr1 cells.
In HNSCC, the increased expression of LAG3 in TILs was related to higher pathological grades, larger tumor size and positive lymph node status. However, this expression had nothing to do with several risk factors such as HPV infection. For patients with recurrent and distant metastatic HNSCC, the LAG3 level in TILs was up-regulated (127). In an immunocompetent HNSCC mouse model, Deng et al. revealed that blocking LAG-3 could suppress tumor development, potentiate antitumor response of CD8+ T cells and reduce the population of immunosuppressive cells (128). mAbs targeting LAG3 could inhibit the interaction between LAG3 and MHC-II and induce IL-2 production in a T cell assay (129).
TIM-3 is a coinhibitory receptor on IFN-γ-producing T cells, FoxP3+ Tregs and innate immune cells and suppress immune responses by interacting with TIM-3 ligand (130). Galectin-9 has the highest affinity for TIM-3. The interaction between Galectin-9 and TIM-3 triggers cell death in effector Th1 cells, dampening tissue inflammation and inhibiting autoimmune disease EAE (131). Moreover, Galectin-9 also induces cell death in Tim-3 + CD8 + TIL in colon cancer (132). Another important ligand of TIM-3 is carcinoembryonic antigen cell adhesion molecule 1 (Ceacam-1). TIM-3 was activated by the action of Ceacam-1, resulting in a weakened interaction between TIM-3 and BAT3 (an inhibitory molecule downstream of TIM3) in T cells in TIM-3 transgenic mice (133). Galectin-9 and Ceacam-1 are combined in different sections of TIM3 IgV domain (133, 134). The two ligands may therefore play a synergistic role in regulating TIM3 signals (135, 136).
In TME, tumor infiltration DCs showed higher expression of TIM-3 than normal tissue. Binding to HMGB1, TIM-3 could block the transport of nucleic acids into endosomes, suppressing pattern-recognition receptor-mediated innate immune responses to tumor-derived nucleic acids (137). TIM-3 could activate the NF-κB signaling pathway to promote tumor cell metastasis (137). In patients or animal tumor models with chronic HIV infection, the expression of TIM-3 on T cells was significantly high (138, 139). On CD8+TILs, TIM-3 often expressed together with PD-1. Besides, their co-expression had a more potent capacity to exhaust T cells compared with PD-1 alone (140–143). In advanced melanomas and NSCLCs, about 1/3 of CD8+TILs expressed TIM-3, which, co-expressed with PD-1, caused defects in the proliferation of T cells and production of effector cytokines (144, 145).
In HNSCC patients, TIM-3+ Tregs are functionally and phenotypically distinct with TILs and are highly effective in inhibiting T cell proliferation. IFN-γ induced by anti-PD-1 immunotherapy may be beneficial to reverse TIM-3+ Tregs suppression (146). In the HNSCC mouse model, the expression of TIM-3, the percent of Tregs and CD206 + macrophages were increased, while the amount of effector T cells (CD4+, CD8+ T cells) was decreased. However, blockade of TIM-3 induced a decrease of Tregs and promoted IFN-γ production on CD8+ T cells (147). The use of anti-TIM3 antibodies could not only reduce the expression of TIM-3 on the surface of T cells, but also decrease the number of MDSCs, inhibiting tumor growth (148). Moreover, the treatment of anti-TIM-3 monoclonal antibodies could restore the function of T cells to inhibit tumor growth (149). However, some of the data indicated that TIM-3 could function as a co-stimulatory receptor to enhance CTLs and other immune cell responses, which indicated TIM-3 might play a more complex role in regulating anti-tumor responses (150–152), and much work should be done in this area (Figure 2).
Figure 2. Costimulatory and coinhibitory signal molecules can be expressed on different cell surfaces and play different roles. In the process of T cells' immune response to tumors, costimulatory and coinhibitory signal molecules regulate the immune response in opposite ways. The same signal molecules may exist on the different cell surface, and the same cell surface may express many different signal molecules. The adding of TEX and soluble molecules makes the regulation of the whole immune response more complex. The blue represents the costimulatory molecules, and the gray represents the coinhibitory molecules. The signaling pathways of OX40 and PD-1 are explained in the figure.
HNSCC is in a status of immune suppression, relating not only to the abnormal competition between costimulatory and coinhibitory signal molecules, but also a general lower immunogenicity. What's worse, about 85% of HNSCC patients are found to be resistant to immune checkpoint receptor (ICR) blockades (153). Thus, we need an effective strategy to augment the immunogenicity and inject the T cells with a “cardiac stimulant” for their anti-tumor function. Tumor specific T cell response could be induced by three classes of antigens: antigens from viral proteins (e.g., HPV), somatic mutations and those encoded by cancer-germline genes (153); vaccines may be appropriate.
In HPV-related HNSCC, vaccines containing long HPV peptides have been regarded as a new treatment to enhance tumor immunogenicity. Recently, Dharmaraj et al. produced a new type of cancer vaccine system with mesoporous silica rods (MSR), which could provide virus antigens, recruit DC cells to facilitate their maturation and transfer DCs to draining lymph nodes to promote T cell maturation (154). It suggested that the combination of targeted vaccines and an appropriate controlled-release system could produce a better synergistic immune effect.
Another effective vaccine is through the stimulation of interferon genes (STING). STING could activate STING-TBK1-IRF3 signaling pathways and secret INF-I, which plays an anti-tumor role by promoting the maturation and migration of DCs, enhancing cytotoxic T lymphocyte- or NK cell-mediated cytotoxicity effects and protecting effector cells from apoptosis (155). In humans, STING could express in the basal layer of normal skin, while STING ligands are an effective therapy for premalignant and malignant disease (156). However, SOX2 enhances the degradation of STING in an autophagy dependent manner, thereby blocking IFN-I activation. This immunosuppression state could be mitigated with a STING-inducing nanosatellite vaccine (containing a cGAMP and HPV16 E6/E7 peptide), which promotes APC maturation and enlarges tumor-specific CTLs to inhibit the immune escape of HNSCC.
Notably, the use of STING vaccines greatly improved the response of ICR-resistant HNSCC to the immune checkpoint blockade (ICB) treatment (157, 158). In the pancreatic cancer model, STING could be stimulated by the tumor antigen released after radiotherapy, or the artificial agonist that blocks M2 macrophage differentiation and decreases IL-10 secretion, and control local and distant tumors (159). Moreover, the Ataxia Telangiectasia Mutated (ATM)-inhibition could directly activate TBK1 and enhanced radiation-induced T1IFN reporter activity (160). They are all synergistic with checkpoint blockade therapy. What's more, the local delivery of STING agonists could also enhance the efficacy of surgical resection by serving as a platform to generate systemic immunity to treat or control metastatic diseases. However, the anti-tumor effect of STING agonists could be weakened by IL-10 (161). Hence, vaccines for STING combined with mAbs for signal molecules and traditional treatment methods (surgical, radiotherapy and chemotherapy) may produce more powerful anti-tumor effects (158).
A variety of inhibitory and stimulatory receptors could co-express on tumor antigen-specific CD8+ T cells (including CD160, KLRG-1, TIM-3, 2B4, BTLA, and LAG3) (162). LAG3 and PD-1 could co-express in human ovarian tumor antigen-specific CD8+T cells, leading to T cell dysfunction. Simultaneous blocking of PD-1 and LAG3 could more effectively restore the function of effector T cells (163, 164). In some tumor models, anti-TIM3 has almost the same effect as anti-PD-1 and anti-CTLA-4. Blocking PD-1 and TIM3 simultaneously plays a stronger synergistic anti-tumor role. Similarly, antibodies against PD-L1, TIM3, or LAG3 could restore responses of HCC-derived T cells to tumor antigens, and combinations of those antibodies had additive effects (165). Thus, the combined application of multiple mAbs targeting at different signal molecules may bring about preferable outcomes.
Since HPV (+) and HPV (−) have been regarded as two distinct subtypes, immunotherapy for them could be different. Results displayed that HNSCC with a high T-cell inflamed phenotype (TCIP-H) were enriched in multiple immune checkpoints (particularly PD-L1, PD-L2, PD-1, TIM3, CEACAM1, LAG3, and CTLA4), had frequent mutations in CASP8, EP300, EPHA2, and HRAS, and frequent co-amplification of JAK2 and CD274. HNSCC tumors with a low T-cell inflamed phenotype (TCIP-L) were enriched in the WNT/β-catenin and Hedgehog signaling pathways, had frequent NSD1 mutations, EGFR, YAP1 amplifications and CDKN2A deletions. HPV (+) tumors were enriched in markers of Tregs, while HPV (–) tumors were enriched in M2 macrophages (166). For HNSCC patients, immunotherapy for a single molecule cannot achieve full efficiency; for example, only 13.3% of the HNSCC patients responded to anti -PD-1 (167). A combination of treatments for individuals including mAbs, vaccines, traditional methods as well as signal pathway blocking is needed.
In addition, tumor-derived exosomes (TEX) have received more attention and have been regarded as special immune checkpoints. TEX could express many different inhibitory molecules, including TGF-β1, PD-L1, CD73, and FasL on the membrane (168, 169). It could therefore suppress immune cells function and promote tumor growth (170, 171). A study of mice using an OSCC model showed that TEX could suppress tumor immune response by inhibiting proliferation of both CD4+ and CD8+ T cells and reducing infiltration of T cells into tumors, thereby promoting the carcinogenesis of murine oral squamous cell carcinomas (172). The expression of exosomes is closely related to tumor progression and immunosuppression, which may make it another promising biomarker of tumor development and immune suppression (173).
All authors listed have made a substantial, direct and intellectual contribution to the work, and approved it for publication.
This work was supported by National Natural Science Foundation of China grants (Nos. 81672672, 81572650, 81972542, and 81502357) and by State Key Laboratory of Oral Diseases Special Funded Projects.
The authors declare that the research was conducted in the absence of any commercial or financial relationships that could be construed as a potential conflict of interest.
APC, antigen-presenting cells; AICD, Activation-Induced Cell Death; CTL, cytotoxic lymphocyte; CTLA-4, Cytotoxic T-Lymphocyte Antigen-4; ESCC, esophageal squamous cell carcinoma; EMT, epithelial-mesenchymal transition; FDA, Food and Drug Administration of America; HPV, human papillomavirus; HNSCC, Head and neck squamous cell carcinoma; TCIP-H, high T-cell inflamed phenotype; ICB, immune checkpoint blockade; TCIP-L, low T-cell inflamed phenotype; MDSC, myeloid-derived suppressor cell; mAb, monoclonal antibody; PD-1, programmed death-1; Treg, regulatory T cell; Breg, regulatory B cell; DCreg, regulatory dendritic cell; sCD137, soluble CD137; sCTLA-4, soluble CTLA-4; sPD-1, soluble PD-1; TAM, tumor-associated macrophage; Th17, T helper 17 cell; TAN, tumor-associated neutrophil; ADCC, the antibody-dependent cell-mediated cytotoxicity; TEX, tumor-derived exosomes.
1. Siegel R, Naishadham D, Jemal A. Cancer statistics, 2013. CA Cancer J Clin. (2013) 63:1–30. doi: 10.3322/caac.21166
2. Gildener-Leapman N, Ferris RL, Bauman JE. Promising systemic immunotherapies in head and neck squamous cell carcinoma. Oral Oncol. (20 13) 12:1089–96. doi: 10.1016/j.oraloncology.2013.09.009
3. Hashibe M, Brennan P, Chuang SC, Boccia S, Castellsague X, Chen C, et al. Interaction between tobacco and alcohol use and the risk of head and neck cancer: pooled analysis in the International Head and Neck Cancer Epidemiology Consortium. Cancer Epidemiol Biomarkers Prev. (2009) 18:541–50. doi: 10.1158/1055-9965.EPI-08-0347
4. Gillison ML, D'Souza G, Westra W, Sugar E, Xiao W, Begum S, et al. Distinct risk factor profiles for human papillomavirus type 16-positive and human papillomavirus type 16-negative head and neck cancers. J Natl Cancer Inst. (2008) 100:407–20. doi: 10.1093/jnci/djn025
5. Liu Y, Cao X. Immunosuppressive cells in tumor immune escape and metastasis. J Mol Med. (2016) 94:509–22. doi: 10.1007/s00109-015-1376-x
6. Acuto O, Michel F. CD28-mediated co-stimulation: a quantitative support for TCR signalling. Nat Rev Immunol. (2003) 3:939–51. doi: 10.1038/nri1248
7. Esensten JH, Helou YA, Chopra G, Weiss A, Bluestone JA. CD28 costimulation: from mechanism to therapy. Immunity. (2016) 44:973–88. doi: 10.1016/j.immuni.2016.04.020
8. Boise LH, Minn AJ, Noel PJ, June CH, Accavitti MA, Lindsten T, et al. CD28 costimulation couldpromote T cell survival by enhancing the expression of Bcl-xL. Immunity. (2010) 3:87–98. doi: 10.1016/1074-7613(95)90161-2
9. Qureshi OS, Zheng Y, Nakamura K, Attridge K, Manzotti C, Schmidt EM, et al. Trans-endocytosis of CD80 and CD86: a molecular basis for the cell-extrinsic function of CTLA-4. Science. (2011) 332:600–3. doi: 10.1126/science.1202947
10. Malm IJ, Bruno TC, Fu J, Zeng Q, Taube JM, Westra W, et al. Expression profile and in vitro blockade of programmed death-1 in human papillomavirus–negative head and neck squamous cell carcinoma. Head Neck. (2015) 37:1088–95. doi: 10.1002/hed.23706
11. Riha P, Rudd CE. CD28 co-signaling in the adaptive immune response. Self Nonself . (2010) 1:231–40. doi: 10.4161/self.1.3.12968
12. Watts TH. TNF/TNFR family members in costimulation of T cell responses. Annu Rev Immunol. (2005) 23:23–68. doi: 10.1146/annurev.immunol.23.021704.115839
13. Godfrey WR, Fagnoni FF, Harara MA, Buck D, Engleman EG. Identification of a human OX-40 ligand, a costimulator of CD4+ T cells with homology to tumor necrosis factor. J Exp Med. (1994) 180:757–62. doi: 10.1084/jem.180.2.757
14. Mestas J, Crampton SP, Hori T, Hughes CC. Endothelial cell co-stimulation through OX40 augments and prolongs T cell cytokine synthesis by stabilization of cytokine mRNA. Int Immunol. (2005) 17:737–47. doi: 10.1093/intimm/dxh255
15. Quezada SA, Jarvinen LZ, Lind EF, Noelle RJ. CD40/CD154 interactions at the interface of tolerance and immunity. Annu Rev Immunol. (2004) 22:307–28. doi: 10.1146/annurev.immunol.22.012703.104533
16. Pollok KE, Kim YJ, Zhou Z, Hurtado J, Kim KK, Pickard RT, et al. Inducible T cell antigen 4-1BB. Analysis of expression and function. J Immunol. (1993) 150:771e81.
17. Melero I, Johnston JV, Shufford WW, Mittler RS, Chen L. NK1.1 cells express 4-1BB (CDw137) costimulatory molecule and are required for tumor immunity elicited by anti-4-1BB monoclonal antibodies. Cell Immunol. (1998) 190:167e72. doi: 10.1006/cimm.1998.1396
18. Sanchez-Paulete AR, Labiano S, Rodriguez-Ruiz ME, Azpilikueta A, Etxeberria I, Bolaños E, et al. Deciphering CD137 (4-1BB) signaling in T-cell costimulation for translation into successful cancer immunotherapy. Eur J Immunol. (2016) 46:513–22. doi: 10.1002/eji.201445388
19. Vidard L, Dureuil C, Baudhuin J, Vescovi L, Durand L, Sierra V, et al. CD137 (4-1BB) engagement fine-tunes synergistic IL-15– and IL-21–driven NK cell proliferation. J Immunol. (2019) 203:676–685. doi: 10.4049/jimmunol.1801137
20. Kohrt HE. Stimulation of natural killer cells with a CD137-specific antibody enhances trastuzumab efficacy in xenotransplant models of breast cancer. J Clin Invest. (2012) 122:1066–75. doi: 10.1172/JCI61226
21. Lynch DH. The promise of 4-1BB (CD137)-mediated immuno-modulation and the immunotherapy of cancer. Immunol Rev. (2008) 222:277e86. doi: 10.1111/j.1600-065X.2008.00621.x
22. Kohrt HE, Houot R, Goldstein MJ, Eiskopf K, Alizadeh AA, Brody J, et al. CD137 stimulation enhances the antilymphoma activity of anti-CD20 antibodies. Blood. (2011) 117:2423–32. doi: 10.1182/blood-2010-08-301945
23. Seo SK, Choi JH, Kim YH, Kang WJ, Park HY, Suh JH, et al. 4-1BB-mediated immunotherapy of rheumatoid arthritis. Nat Med. (2004) 10:1088–94. doi: 10.1038/nm1107
24. Vinay DS, Kwon BS. Immunotherapy of cancer with 4-1BB. Mol Cancer Ther. (2012) 11:1062–70. doi: 10.1158/1535-7163.MCT-11-0677
25. Labiano S, Palazón A, Bolaños E, Azpilikueta A, Sánchez-Paulete AR, Morales-Kastresana A, et al. Hypoxia-induced soluble CD137 in malignant cells blocks CD137L-costimulation as an immune escape mechanism. Oncoimmunology. (2016) 5:e1062967. doi: 10.1080/2162402X.2015.1062967
26. Lucido CT, Vermeer PD, Wieking BG, Vermeer DW, Lee JH. CD137 enhancement of HPV positive head and neck squamous cell carcinoma tumor clearance. Vaccines (Basel). (2014) 2: 841–53. doi: 10.3390/vaccines2040841
27. Srivastava RM, Trivedi S, Concha-Benavente F, Gibson SP, Reeder C, Ferrone S, et al. CD137 stimulation enhances cetuximab induced natural killer (NK): dendritic cell (DC) priming of anti-tumor T cell immunity in head and neck cancer patients. Clin Cancer Res. 23:707–16. doi: 10.1158/1078-0432.CCR-16-0879
28. Sznol M, Hodi FS, Margolin K, McDermott DF, Ernstoff MS, Kirkwood JM, et al. Phase I study of BMS-663513, a fully human anti-CD137 agonist monoclonal antibody, in patients (pts) with advanced cancer (CA). J Clin Oncol. (2008) 26(15 Suppl.):3007. doi: 10.1200/jco.2008.26.15_suppl.3007
29. Chen S, Lee LF, Fisher TS, Jessen B, Elliott M, Evering W, et al. Combination of 4-1BB agonist and PD-1 antagonist promotes antitumor effector/memory CD8 T cells in a poorly immunogenic tumor model. Cancer Immunol Res. (2015) 3:149–60. doi: 10.1158/2326-6066.CIR-14-0118
30. Croft M, So T, Duan W, Soroosh P. The significance of OX40 and OX40L to T-cell biology and immune disease. Immunol Rev. (2009) 229:173–91. doi: 10.1111/j.1600-065X.2009.00766.x
31. Munks MW, Mourich DV, Mittler RS, Weinberg AD, Hill AB. 4-1BB and OX40 stimulation enhance CD8 and CD4 T-cell responses to a DNA prime, poxvirus boost vaccine. Immunology. (2004) 112:559–66. doi: 10.1111/j.1365-2567.2004.01917.x
32. Bulliard Y, Jolicoeur R, Zhang J, Dranoff G, Wilson NS, Brogdon JL. OX40 engagement depletes intratumoral Tregs via activating FccRs, leading to antitumor efficacy. Immunol. Cell Biol. (2014) 92:475–80. doi: 10.1038/icb.2014.26
33. Lai C, August S, Albibas A, Behar R, Cho SY, Polak ME, et al. OX40+ Regulatory T cells in cutaneous squamous cell carcinoma suppress effector T-cell responses and associate with metastatic potential. Clin Cancer Res. (2016) 22:4236–48. doi: 10.1158/1078-0432.CCR-15-2614
34. Li F, Wang Y, Lin L, Wang J, Xiao H, Li J, et al. Mast cell-derived exosomes promote Th2 cell differentiation via OX40L-OX40 ligation. J Immunol Res. (2016) 2016:3623898. doi: 10.1155/2016/3623898
35. Huang L, Zhang X, Wang M, Chen Z, Yan Y, Gu W, et al. Exosomes from thymic stromal lymphopoietin-activated dendritic cells promote Th2 differentiation through the OX40 ligand. Pathobiology. (2019) 86:111–7. doi: 10.1159/000493013
36. Willoughby J, Griffiths J, Tews I, Cragg MS. OX40: Structure and function - What questions remain? Mol Immunol. (2017) 83:13–22. doi: 10.1016/j.molimm.2017.01.006
37. Polesso F, Sarker M, Weinberg AD, Murray SE, Moran AE. OX40 agonist tumor immunotherapy does not impact regulatory T cell suppressive function. J Immunol. (2019) 203:2011–9. doi: 10.4049/jimmunol.1900696
38. Vetto JT, Lum S, Morris A, Sicotte M, Davis J, Lemon M, et al. Presence of the T cell activation marker OX-40 on tumor infiltrating lymphocytes and draining lymph node cells from patients with melanoma and head and neck cancers. Am J Surg. (1997) 174:258–65. doi: 10.1016/S0002-9610(97)00139-6
39. Baruah P, Lee M, Odutoye T, Williamson P, Hyde N, Kaski JC, et al. Decreased levels of alternative co-stimulatory receptors OX40 and 4-1BB characterise T cells from head and neck cancer patients. Immunobiology. (2012) 217:669–75. doi: 10.1016/j.imbio.2011.11.005
40. Bell RB, Leidner RS, Crittenden MR, Curti BD, Feng Z, Montler R, et al. OX40 signaling in head and neck squamous cell carcinoma: overcoming immunosuppression in the tumor microenvironment. Oral Oncol. (2016) 52:1–10. doi: 10.1016/j.oraloncology.2015.11.009
41. Curti BD, Kovacsovics-Bankowski M, Morris N, Walker E, Chisholm L, Floyd K, et al. OX40 is a potent immune stimulating target in late stage cancer patients. Cancer Res. (2013) 73: 7189–98. doi: 10.1158/0008-5472.CAN-12-4174
42. Voo KS, Bover L, Harline ML, Vien LT, Facchinetti V, Arima K, et al. Antibodies targeting human OX40 expand effector T cells and block inducible and natural regulatory T cell function. J Immunol. (2013) 191:3641–50. doi: 10.4049/jimmunol.1202752
43. Guo Z, Wang X, Cheng D, Xia Z, Luan M, Zhang S. PD-1 blockade and OX40 triggering synergistically protects against tumor growth in a murine model of ovarian cancer. PLoS ONE. (2014) 9:e89350. doi: 10.1371/journal.pone.0089350
44. Gough MJ, Crittenden MR, Sarff M, Pang P, Seung SK, Vetto JT, et al. Adjuvant therapy with agonistic antibodies to CD134 (OX40) increases local control after surgical or radiation therapy of cancer in mice. J Immunother. (2010) 33:798–809. doi: 10.1097/CJI.0b013e3181ee7095
45. Moy JD, Moskovitz JM, Ferris RL. Biological mechanisms of immune escape and implications for immunotherapy in head and neck squamous cell carcinoma. Eur J Cancer. (2017) 76:152–66. doi: 10.1016/j.ejca.2016.12.035
46. Grewal IS, Flavell RA. CD40 and CD154 in cell-mediated immunity. Annu Rev Immunol. (1998) 16:111–35. doi: 10.1146/annurev.immunol.16.1.111
47. Angelou A, Antoniou E, Garmpis N, Damaskos C, Theocharis S, Margonis GA. The role of soluble CD40L ligand in human carcinogenesis. Anticancer Res. (2018) 38:3199–201. doi: 10.21873/anticanres.12616
48. Dejardin E. The alternative NF-kappaB pathway from biochemistry to biology: pitfalls and promises for future drug development. Biochem Pharmacol. (2006) 72:1161–79. doi: 10.1016/j.bcp.2006.08.007
49. Shih VF, Tsui R, Caldwell A, Hoffmann A. A single NFkappaB system for both canonical and non-canonical signaling. Cell Res. (2011) 21:86–102. doi: 10.1038/cr.2010.161
50. Lai JH, Luo SF, Ho LJ. Targeting the CD40-CD154 Signaling Pathway for Treatment of Autoimmune Arthritis. Cells. (2019) 8:927. doi: 10.3390/cells8080927
51. Ngiow SF, Young A, Blake SJ, Hill GR, Yagita H, Teng MW, et al. Agonistic CD40 mAb-driven IL12 reverses resistance to anti-PD1 in a T-cell–rich tumor. Cancer Res. (2016) 76:6266–77. doi: 10.1158/0008-5472.CAN-16-2141
52. Murugaiyan G, Agrawal R, Mishra GC, Mitra D, Saha B. Functional dichotomy in CD40 reciprocally regulates effector T cell functions. J Immunol. (2006) 177:6642–9. doi: 10.4049/jimmunol.177.10.6642
53. Zhang J, Li Y, Yang S, Zhang L, Wang W. Anti-CD40 mAb enhanced efficacy of anti-PD1 against osteosarcoma. J Bone Oncol. (2019) 17:100245. doi: 10.1016/j.jbo.2019.100245
54. Murugaiyan G, Agrawal R, Mishra GC, Mitra D, Saha B. Differential CD40/CD40L expression results in counteracting anti-tumor immune responses. J Immunol. (2007) 178:2047–55 doi: 10.4049/jimmunol.178.4.2047
55. Murugaiyan G, Martin S, Saha B. Levels of CD40 expression on dendritic cells dictate tumour growth or regression. Clin Exp Immunol. (2007) 149:194–202. doi: 10.1111/j.1365-2249.2007.03407.x
56. Sathawane D, Kharat RS, Halder S, Roy S, Swami R, Patel R, et al. Monocyte CD40 expression in head and neck squamous cell carcinoma (HNSCC). Hum Immunol. (2013) 74:1–5. doi: 10.1016/j.humimm.2012.09.004
57. Cao W, Cavacini LA, Tillman KC, Posner MR. CD40 function in squamous cell cancer of the head and neck. Oral Oncol. (2005) 41:462e9. doi: 10.1016/j.oraloncology.2004.11.005
58. Matsumura Y, Hiraoka K, Ishikawa K, Shoji Y, Noji T, Hontani K, et al. CD40 expression in human esophageal squamous cell carcinoma is associated with tumor progression and lymph node metastasis. Anticancer Res. (2016) 36:4467–75. doi: 10.21873/anticanres.10991
59. Oka M, Iizuka N, Yamamoto K, Gondo T, Abe T, Hazama S, et al. The influence of interleukin-6 on the growth of human esophageal cancer cell lines. J Interferon Cytokine Res. (1996) 16:1001–6. doi: 10.1089/jir.1996.16.1001
60. Jakobson E, Jonsson G, Bjorck P, Paulie S. Stimulation of CD40 in human bladder carcinoma cells inhibits anti-Fas/APO-1 (CD95)-induced apoptosis. Int J Cancer. (1998) 77:849–53.
61. Posner MR, Cavacini LA, Upton MP, Tillman KC, Gornstein ER, Norris CM Jr. Surface membrane-expressed CD40 is present on tumor cells from squamous cell cancer of the head and neck in vitro and in vivo and regulates cell growth in tumor cell lines. Clin Cancer Res. (1999) 5:2261–70.
62. Rakhmilevich AL, Alderson KL, Sondel PM. T-cell-independent antitumor effects of CD40 ligation. Int Rev Immunol. (2012) 31:267–78. doi: 10.3109/08830185.2012.698337
63. Takahashi T, Tagami T, Yamazaki S, Uede T, Shimizu J, Sakaguchi N, et al. Immunologic self-tolerance maintained by CD25(+) CD4(+) regulatory T cells constitutively expressing cytotoxic T lymphocyte-associated antigen 4. J Exp Med. (2000) 192:303–10. doi: 10.1084/jem.192.2.303
64. Tai X1, Van Laethem F, Sharpe AH, Singer A. Induction of autoimmune disease in CTLA-4-/- mice depends on a specific CD28 motif that is required for in vivo costimulation. Proc Natl Acad Sci USA. (2007) 104:13756–61. doi: 10.1073/pnas.0706509104
65. Mandelbrot DA, McAdam AJ, Sharpe AH. B7-1 or B7-2 is required to produce the lymphoproliferative phenotype in mice lacking cytotoxic T lymphocyte-associated antigen 4 (CTLA-4). J Exp Med. (1999) 189:435–40. doi: 10.1084/jem.189.2.435
66. Miska J, Abdulreda MH, Devarajan P, Lui JB, Suzuki J, Pileggi A, et al. Real-time immune cell interactions in target tissue during autoimmune-induced damage and graft tolerance. J Exp Med. (2014) 211:441–56. doi: 10.1084/jem.20130785
67. Riley JL. PD-1 signaling in primary T cells. Immunol Rev. (2009) 229:114–25. doi: 10.1111/j.1600-065X.2009.00767.x
68. Badoual C, Hans S, Merillon N, Van Ryswick C, Ravel P, Benhamouda N, et al. PD-1-expressing tumor-infiltrating T cells are a favorable prognostic biomarker in HPV-associated head and neck cancer. Cancer Res. (2013) 73:128–38. doi: 10.1158/0008-5472.CAN-12-2606
69. Huang CT, Workman CJ, Flies D, Pan X, Marson AL, Zhou G, et al. Role of LAG-3 in regulatory T cells. Immunity. (2004) 21:503–13. doi: 10.1016/j.immuni.2004.08.010
70. Monney L, Sabatos CA, Gaglia JL, Ryu A, Waldner H, Chernova T, et al. Th1-specific cell surface protein Tim-3 regulates macrophage activation and severity of an autoimmune disease. Nature. (2002) 415:536–41. doi: 10.1038/415536a
71. Read S, Malmström V, Powrie F. Cytotoxic T lymphocyte-associated antigen 4 plays an essential role in the function of CD25(+) CD4(+) regulatory cells that control intestinal inflammation. J Exp Med. (2000) 192:295–302. doi: 10.1084/jem.192.2.295
72. Krummey SM, Ford ML. Braking bad: novel mechanisms of CTLA-4 inhibition of T cell esponses. Am J Transplant. (2014) 14:2685–90. doi: 10.1111/ajt.12938
73. Parry RV, Chemnitz JM, Frauwirth KA, Lanfranco AR, Braunstein I, Kobayashi SV, et al. CTLA-4 and PD-1 receptors inhibit T-cell activation by distinct mechanisms. Mol Cell Biol. (2005) 25:9543–53. doi: 10.1128/MCB.25.21.9543-9553.2005
74. Carreno BM, Bennett F, Chau TA, Ling V, Luxenberg D, Jussif J, et al. CTLA-4 (CD152) can inhibit T cell activation by two different mechanisms depending on its level of cell surface expression. J Immunol. (2000) 165:1352–6. doi: 10.4049/jimmunol.165.3.1352
75. Ward FJ, Dahal LN, Khanolkar RC, Shankar SP, Barker RN. Targeting the alternatively spliced soluble isoform of CTLA-4: prospects for immunotherapy? Immunotherapy. (2014) 6:1073–84. doi: 10.2217/imt.14.73
76. Karpathiou G, Casteillo F, Giroult JB, Forest F, Fournel P, Monaya A, et al. Prognostic impact of immune microenvironment in laryngeal and pharyngeal squamous cell carcinoma: Immune cell subtypes, immuno-suppressive pathways and clinicopathologic characteristics. Oncotarget. (2017) 8:19310–22. doi: 10.18632/oncotarget.14242
77. Huang PY, Guo SS, Zhang Y, Lu JB, Chen QY, Tang LQ, et al. Tumor CTLA-4 overexpression predicts poor survival in patients with nasopharyngeal carcinoma. Oncotarget. (2016) 7:13060–8. doi: 10.18632/oncotarget.7421
78. Erfani N1, Khademi B, Haghshenas MR, Mojtahedi Z, Khademi B, Ghaderi A. Intracellular CTLA4 and regulatory T cells in patients with laryngeal squamous cell carcinoma. Immunol Invest. (2013) 42:81–90. doi: 10.3109/08820139.2012.708376
79. Zhang XF, Pan K, Weng DS, Chen CL, Wang QJ, Zhao JJ, et al. Cytotoxic T lymphocyte antigen-4 expression in esophageal carcinoma: implications for prognosis. Oncotarget. (2016) 7:26670–9. doi: 10.18632/oncotarget.8476
80. Zhang N, Schröppel B, Lal G, Jakubzick C, Mao X, Chen D, et al. Regulatory T cells sequentially migrate from inflamed tissues to draining lymph nodes to suppress the alloimmune response. Immunity. (2009) 30:458–69. doi: 10.1016/j.immuni.2008.12.022
81. Jie HB, Schuler PJ, Lee SC, Srivastava RM, Argiris A, Ferrone S, et al. CTLA-4 + Regulatory T cells increased in cetuximab treated head and neck cancer patients suppress NK cell cytotoxicity and correlate with poor prognosis. Cancer Res. (2015) 75:2200–10. doi: 10.1158/0008-5472.CAN-14-2788
82. Dutta A, Banerjee A, Saikia N, Phookan J, Baruah MN, Baruah S. Negative regulation of natural killer cell in tumor tissue and peripheral blood of oral squamous cell carcinoma. Cytokine. (2015) 76:123–30. doi: 10.1016/j.cyto.2015.09.006
83. Chai JG1, Tsang JY, Lechler R, Simpson E, Dyson J, Scott D. CD4+CD25+ T cells as immunoregulatory T cells in vitro. Eur J Immunol. (2002) 32:2365–75.
84. Walker LS. Treg and CTLA-4: two intertwining pathways to immune tolerance. J Autoimmun. (2013) 45:49–57. doi: 10.1016/j.jaut.2013.06.006
85. Lynch TJ, Bondarenko I, Luft A, Serwatowski P, Barlesi F, Chacko R, et al. Ipilimumab in combination with paclitaxel and carboplatin as first-line treatment in stage IIIB/IV non-small-cell lung cancer: results from a randomized, double-blind, multicenter phase II study. J Clin Oncol. (2012) 30:2046–54. doi: 10.1200/JCO.2011.38.4032
86. Le DT, Lutz E, Uram JN, Sugar EA, Onners B, Solt S, et al. Evaluation of ipilimumab in combination with allogeneic pancreatic tumor cells transfected with a GM-CSF gene in previously treated pancreatic cancer. J Immunother. (2013) 36:382–9. doi: 10.1097/CJI.0b013e31829fb7a2
87. Slovin SF, Higano CS, Hamid O, Tejwani S, Harzstark A, Alumkal JJ, et al. Ipilimumab alone or in combination with radiotherapy in metastatic castration-resistant prostate cancer: results from an open-label, multicenter phase I/II study. Oncology. (2013) 24:1813–21. doi: 10.1093/annonc/mdt107
88. Postow MA, Callahan MK, Barker CA, Yamada Y, Yuan J, Kitano S, et al. Immunologic correlates of the abscopal effect in a patient with melanoma. J Med. (2012) 366:925–31. doi: 10.1056/NEJMoa1112824
89. Tang F, Du X, Liu M, Zheng P, Liu Y. Anti-CTLA-4 antibodies in cancer immunotherapy: selective depletion of intratumoral regulatory T cells or checkpoint blockade? Cell Biosci. (2018) 8:30. doi: 10.1186/s13578-018-0229-z
90. Keir ME, Butte MJ, Freeman GJ, Sharpe AH. PD-1 and its ligands in tolerance and immunity. Annu Rev Immunol. (2008) 26:677e704. doi: 10.1146/annurev.immunol.26.021607.090331
91. Stamell EF, Wolchok JD, Gnjatic S, Lee NY, Brownell I. The abscopal effect associated with a systemic anti-melanoma immune response. Oncol Biol Phys. (2013) 85:293–5. doi: 10.1016/j.ijrobp.2012.03.017
92. Geng H, Zhang GM, Xiao H, Yuan Y, Li D, Zhang H, et al. HSP70 vaccine in combination with gene therapy with plasmid DNA encoding sPD-1 overcomes immune resistance and suppresses the progression of pulmonary metastatic melanoma. Int J Cancer. (2006) 118:2657–64. doi: 10.1002/ijc.21795
93. Qiu H, Liu S, Xie C, Long J, Feng Z. Regulating immunity and inhibiting tumor growth by the recombinant peptide sPD-1-CH50. Anticancer Res. (2009) 29:5089–94.
94. Kuipers H, Muskens F, Willart M, Hijdra D, van Assema FB, Coyle AJ, et al. Contribution of the PD-1 ligands/PD-1 signaling pathway to dendritic cell-mediated CD4+ T cell activation. Eur J Immunol. (2006) 36:2472–82. doi: 10.1002/eji.200635978
95. Kuipers H, Muskens F, Willart M, Hijdra D, van Assema FB, Coyle AJ, et al. Human cancer immunotherapy with antibodies to the PD-1 and PD-L1 pathway. Trends Mol Med. (2015) 21:24–33. doi: 10.1016/j.molmed.2014.10.009
96. Gatalica Z, Snyder C, Maney T, Ghazalpour A, Holterman DA, Xiao N, et al. Programmed cell death 1 (PD-1) and its ligand (PD-L1) in common cancers and their correlation with molecular cancer type. Cancer Epidemiol Biomarkers Rev. (2014) 23:2965–70. doi: 10.1158/1055-9965.EPI-14-0654
97. Riella LV, Paterson AM, Sharpe AH, Chandraker A. Role of the PD-1 pathway in the immune response. J Transplant. (2012) 12:2575–87. doi: 10.1111/j.1600-6143.2012.04224.x
98. Chen N, Fang W, Zhan J, Hong S, Tang Y, Kang S, et al. Upregulation of PD-L1 by EGFR activation mediates the immune escape in EGFR-driven NSCLC: implication for optional immune targeted therapy for NSCLC patients with EGFR mutation. J Thorac Oncol. (2015) 10:910–23. doi: 10.1097/JTO.0000000000000500
99. Ota K, Azuma K, Kawahara A, Hattori S, Iwama E, Tanizaki J, et al. Induction of PD-L1 expression by the EML4-ALK oncoprotein and downstream signaling pathways in non-small cell lung cancer. Clin Cancer Res. (2015) 21:4014–21. doi: 10.1158/1078-0432.CCR-15-0016
100. Dondero A, Pastorino F, Della Chiesa M, Corrias MV, Morandi F, Pistoia V, et al. PD-L1 expression in metastatic neuroblastoma as an additional mechanism for limiting immune surveillance. Oncoimmunology. (2015) 5:e1064578. doi: 10.1080/2162402X.2015.1064578
101. Francisco LM, Sage PT, Sharpe AH. The PD-1 pathway in tolerance and autoimmunity. Immunol Rev. (2010) 236:219–42. doi: 10.1111/j.1600-065X.2010.00923.x
102. Kim DH, Kim H, Choi YJ, Kim SY, Lee JE, Sung KJ, et al. Exosomal PD-L1 promotes tumor growth through immune escape in non-small cell lung cancer. Exp Mol Med. (2019) 51:94. doi: 10.1038/s12276-019-0295-2
103. Poggio M, Hu T, Pai CC, Chu B, Belair CD, Chang A, et al. Suppression of exosomal PD-L1 induces systemic anti-tumor immunity and memory. Cell. (2019) 177:414–427.e13. doi: 10.1016/j.cell.2019.02.016
104. Li Y, Xiao Y, Su M, Zhang R, Ding J, Hao X, et al. Role of soluble programmed death-1 (sPD-1) and sPD-ligand 1 in patients with cystic echinococcosis. Exp Ther Med. (2016) 11:251–6. doi: 10.3892/etm.2015.2876
105. Bardhan K, Anagnostou T, Boussiotis VA. The PD1:PD-L1/2 pathway from discovery to clinical implementation. Front Immunol. (2016) 7:550. doi: 10.3389/fimmu.2016.00550
106. Lyford-Pike S, Peng S, Young GD, Taube JM, Westra WH, Akpeng B, et al. Evidence for a role of the PD-1: PD-L1 pathway in immune resistance of HPV-associated head and neck squamous cell carcinoma. Cancer Res. (2013) 73:1733–41. doi: 10.1158/0008-5472.CAN-12-2384
107. Ock CY, Kim S, Keam B, Kim M, Kim TM, Kim JH, et al. PD-L1 expression is associated with epithelial-mesenchymal transition in head and neck squamous cell carcinoma. Oncotarget. (2016) 7:15901–14. doi: 10.18632/oncotarget.7431
108. Lin W, Chen M, Hong L, Zhao H, Chen Q. Crosstalk between PD-1/PD-L1 blockade and its combinatorial therapies in tumor immune microenvironment: a focus on HNSCC. Front Oncol. (2018) 8:532. doi: 10.3389/fonc.2018.00532
109. Roper E, Lum T, Palme CE, Ashford B, Ch'ng S, Ranson M, et al. PD-L1 expression predicts longer disease free survival in high risk head and neck cutaneous squamous cell Carcinoma. Pathology. 49:499–505. doi: 10.1016/j.pathol.2017.04.004
110. Xu F, Feng G, Zhao H, Liu F, Xu L, Wang Q, et al. Clinicopathologic significance and prognostic value of B7 homolog 1 in gastric cancer: a systematic review and meta-analysis. Medicine (Baltimore). (2015) 94:e1911. doi: 10.1097/MD.0000000000001911
111. Guo Y, Yu P, Liu Z, Maimaiti Y, Wang S, Yin X, et al. Clinicopathological and prognostic value of programmed death ligand-1 in breast cancer: a meta-analysis. PLoS ONE. (2016) 11:e0156323. doi: 10.1371/journal.pone.0156323
112. Xu F, Xu L, Wang Q, An G, Feng G, Liu F. Clinicopathological and prognostic value of programmed death ligand-1 (PD-L1) in renal cell carcinoma: a meta-analysis. Int J Clin Exp Med. (2015) 8:14595–603.
113. Bigelow E, Bever KM, Xu H, Yager A, Wu A, Taube J, et al. Immunohistochemical staining of B7-H1 (PD-L1) on paraffin-embedded slides of pancreatic adenocarcinoma tissue. J Vis Exp. (2013) 71:4059. doi: 10.3791/4059
114. Taube JM, Anders RA, Young GD, Xu H, Sharma R, McMiller TL, et al. Colocalization of inflammatory response with B7-h1 expression in human melanocytic lesions supports an adaptive resistance mechanism of immune escape. Sci Transl Med. (2012) 4:127ra37. doi: 10.1126/scitranslmed.3003689
115. Lipson EJ, Vincent JG, Loyo M, Kagohara LT, Luber BS, Wang H, et al. PD-L1 expression in the Merkel cell carcinoma microenvironment: association with inflammation, Merkel cell polyomavirus and overall survival. Cancer Immunol Res. (2013) 1:54–63. doi: 10.1158/2326-6066.CIR-13-0034
116. Droeser RA, Hirt C, Viehl CT, Frey DM, Nebiker C, Huber X, et al. Clinical impact of programmed cell death ligand 1 expression in colorectal cancer. Eur J Cancer. (2013) 49:2233–42. doi: 10.1016/j.ejca.2013.02.015
117. Velcheti V, Schalper KA, Carvajal DE. Programmed death ligand-1 expression in non-small cell lung cancer. Lab Invest. (2014) 94:107–116. doi: 10.1038/labinvest.2013.130
118. Toyokawa G, Takada K, Haratake N, Takamori S, Akamine T, Katsura M, et al. Favorable disease-free survival associated with programmed death ligand 1 expression in patients with surgically resected small-cell lung cancer. Anticancer Res. (2016) 36:4329–36.
119. Theodoraki MN, Yerneni SS, Hoffmann TK, Gooding WE, Whiteside TL. Clinical significance of PD-L1+ exosomes in plasma of Head and Neck Cancer patients. Clin Cancer Res. (2018) 24:896–905. doi: 10.1158/1078-0432.CCR-17-2664
120. Seiwert TY, Burtness B, Mehra R, Weiss J, Berger R, Eder JP, et al. Safety and clinical activity of pembrolizumab for treatment of recurrent or metastatic squamous cell carcinoma of the head and neck (KEYNOTE-012): an open-label, multicentre, phase 1b trial. Lancet Oncol. (2016) 17:956–65. doi: 10.1016/S1470-2045(16)30066-3
121. Bauml J, Seiwert TY, Pfister DG, Worden F, Liu SV, Gilbert J, et al. Pembrolizumab for platinum- and cetuximab-refractory head and neck cancer: results from a single-arm, phase II study. J Clin Oncol. (2017) 35:1542–9. doi: 10.1200/JCO.2016.70.1524
122. Cohen EEW, Soulières D, Le Tourneau C, Dinis J, Licitra L, Ahn MJ, et al. Pembrolizumab versus methotrexate, docetaxel, or cetuximab for recurrent or metastatic head-and-neck squamous cell carcinoma (KEYNOTE-040): a randomised, open-label, phase 3 study. Lancet. (2019) 393:156–167. doi: 10.1016/S0140-6736(18)31999-8
123. Triebel F, Jitsukawa S, Baixeras E, Roman-Roman S, Genevee C, Viegas-Pequignot E, et al. LAG-3, a novel lymphocyte activation gene closely related to CD4. J Exp Med. (1990) 171:1393–405. doi: 10.1084/jem.171.5.1393
124. Huard B, Prigent P, Tournier M, Bruniquel D, Triebel F, et al. CD4/major histocompatibility complex class II interaction analyzed with CD4- and lymphocyte activation gene-3 (LAG-3)-Ig fusion proteins. J Immunol. (1995) 25:2718–21. doi: 10.1002/eji.1830250949
125. Xu F, Liu J, Liu D, Liu B, Wang M, Hu Z, et al. LSECtin expressed on melanoma cells promotes tumor progression by inhibiting antitumor T-cell responses. Cancer Res. (2014) 74:3418–28. doi: 10.1158/0008-5472.CAN-13-2690
126. Gagliani N, Magnani CF, Huber S, Gianolini ME, Pala M, Licona-Limon P, et al. Coexpression of CD49b and LAG-3 identifies human and mouse T regulatory type 1 cells. Nat Med. (2013) 19:739–46. doi: 10.1038/nm.3179
127. Keski-Säntti H, Atula T, Törnwall J, Koivunen P, Mäkitie A. Elective neck treatment versus observation in patients with T1/T2 N0 squamous cell carcinoma of oral tongue. Oral Oncol. (2006) 42:96–101. doi: 10.1016/j.oraloncology.2005.06.018
128. Deng WW, Mao L, Yu GT, Bu LL, Ma SR, Liu B, et al. LAG-3 confers poor prognosis and its blockade reshapes antitumor response in head and neck squamous cell carcinoma. Oncoimmunology. (2016) 5:e1239005. doi: 10.1080/2162402X.2016.1239005
129. Solinas C, Migliori E, De Silva P, Willard-Gallo K. LAG3: the biological processes that motivate targeting this immune checkpoint molecule in human cancer. Cancers (Basel). (2019) 11:E1213. doi: 10.3390/cancers11081213
130. Liu Z, McMichael EL, Shayan G, Li J, Chen K, Srivastava R, et al. Novel effector phenotype of Tim-3+ regulatory T cells leads to enhanced suppressive function in head and neck cancer patients. Clin Cancer Res. (2018) 24:4529–38. doi: 10.1158/1078-0432.CCR-17-1350
131. Zhu C, Anderson AC, Schubart A, Xiong H, Imitola J, Khoury SJ, et al. The Tim-3 ligand galectin-9 negatively regulates T helper type 1 immunity. Nat Immunol. (2005) 6:1245–52. doi: 10.1038/ni1271
132. Kang CW, Dutta A, Chang LY, Mahalingam J, Lin YC, Chiang JM, et al. Apoptosis of tumor infiltrating effector TIM-3+ CD8+ T cells in colon cancer. Sci Rep. (2015) 5:15659. doi: 10.1038/srep15659
133. Dankner M, Gray-Owen SD, Huang YH, Blumberg RS, Beauchemin N. CEACAM1 as a multi-purpose target for cancer immunotherapy. Oncoimmunology. (2017) 6: e1328336. doi: 10.1080/2162402X.2017.1328336
134. Cao E, Zang X, Ramagopal UA, Mukhopadhaya A, Fedorov A, Fedorov E, et al. T cell immunoglobulin mucin-3 crystal structure reveals a galectin-9-independent ligand-binding surface. Immunity. (2007) 26:311–21. doi: 10.1016/j.immuni.2007.01.016
135. Huang YH, Zhu C, Kondo Y, Anderson AC, Gandhi A, Russell A, et al. CEACAM1 regulates TIM-3-mediated tolerance and exhaustion. Nature. (2015) 517:386–90. doi: 10.1038/nature13848
136. Rangachari M, Zhu C, Sakuishi K, Xiao S, Karman J, Chen A, et al. Bat3 promotes T cell responses and autoimmunity by repressing Tim-3-mediated cell death and exhaustion. Nat Med. (2012) 18:1394–400. doi: 10.1038/nm.2871
137. Chiba S, Baghdadi M, Akiba H, Yoshiyama H, Kinoshita I, Dosaka-Akita H, et al. Tumor-infiltrating DCs suppress nucleic acid-mediated innate immune responses through interactions between the receptor TIM-3 and the alarmin HMGB1. Nat Immunol. (2012) 13:832–42. doi: 10.1038/ni.2376
138. Wu FH, Yuan Y, Li D, Lei Z, Song CW, Liu YY, et al. Endothelial cell-expressed Tim-3 facilitates metastsis of melanoma cells by activating the NF-κB pathway. Oncol Rep. (2010) 24:693–9. doi: 10.3892/or_00000909
139. Jones RB, Ndhlovu LC, Barbour JD, Sheth PM, Jha AR, Long BR, et al. Tim-3 expression defines a novel population of dysfunctional T cells with highly elevated frequencies in progressive HIV-1 infection. J Exp Med. (2008) 205:2763–79. doi: 10.1084/jem.20081398
140. Sakuishi K, Apetoh L, Sullivan JM, Blazar BR, Kuchroo VK, Anderson AC. Targeting Tim-3 and PD-1 pathways to reverse T cell exhaustion and restore anti-tumor immunity. J Exp Med. (2010) 207:2187–94. doi: 10.1084/jem.20100643
141. Jin HT, Anderson AC, Tan WG, West EE, Ha SJ, Araki K, et al. Cooperation of Tim-3 and PD-1 in CD8 T-cell exhaustion during chronic viral infection. Proc Natl Acad Sci U.S.A. (2010) 107:14733–8. doi: 10.1073/pnas.1009731107
142. Li H, Wu K, Tao K, Chen L, Zheng Q, Lu X, et al. Tim-3/galectin-9 signaling pathway mediates T-cell dysfunction and predicts poor prognosis in patients with hepatitis B virus-associated hepatocellular carcinoma. Hepatology. (2012) 56:1342–51. doi: 10.1002/hep.25777
143. Japp AS, Kursunel MA, Meier S, Mälzer JN, Li X, Rahman NA, et al. Dysfunction of PSA-specific CD8+ T cells in prostate cancer patients correlates with CD38 and Tim-3 expression. Cancer Immunol Immunother. (2015) 64:1487–94. doi: 10.1007/s00262-015-1752-y
144. Fourcade J, Sun Z, Benallaoua M, Guillaume P, Luescher IF, Sander C, et al. Upregulation of Tim-3 and PD-1 expression is associated with tumor antigen-specific CD8+ T cell dysfunction in melanoma patients. J Exp Med. (2010) 207:2175–86. doi: 10.1084/jem.20100637
145. Gao X, Zhu Y, Li G, Huang H, Zhang G, Wang F, et al. TIM-3 expression characterizes regulatory T cells in tumor tissues and is associated with lung cancer progression. PLoS ONE. (2012) 7:e30676. doi: 10.1371/journal.pone.0030676
146. Bu M, Shen Y, Seeger W, An S1, Qi R, Sanderson JA, et al. Ovarian carcinoma-infiltrating regulatory T cells were more potent suppressors of CD8(+) T cell inflammation than their peripheral counterparts, a function dependent on TIM3 expression. Tumour Biol. (2016) 37:3949–56. doi: 10.1007/s13277-015-4237-x
147. Liu JF, Wu L, Yang LL, Deng WW, Mao L, Wu H, et al. Blockade of TIM3 relieves immunosuppression through reducing regulatory T cells in head and neck cancer. J Exp Clin Cancer Res. (2018) 37:44. doi: 10.1186/s13046-018-0713-7
148. Liu JF, Ma SR, Mao L, Bu LL, Yu GT, Li YC, et al. T-cell immunoglobulin mucin 3 blockade drives an antitumor immune response in head and neck cancer. Mol Oncol. (2017) 11:235–247. doi: 10.1002/1878-0261.12029
149. Zhou G, Sprengers D, Boor PPC, Doukas M, Schutz H, Mancham S, et al. Antibodies against immune checkpoint molecules restore functions of tumor-infiltrating T cells in hepatocellular carcinomas. Gastroenterology. (2017) 153:1107–1119.e10. doi: 10.1053/j.gastro.2017.06.017
150. Gorman JV, Starbeck-Miller G, Pham NL, Traver GL, Rothman PB, Harty JT, et al. Tim-3 directly enhances CD8 T cell responses to acute Listeria monocytogenes infection. J Immunol. (2014) 192:3133–42. doi: 10.4049/jimmunol.1302290
151. Gleason MK, Lenvik TR, McCullar V, Felices M, O'Brien MS, Cooley SA, et al. Tim-3 is an inducible human natural killer cell receptor that enhances interferon gamma production in response to galectin-9. Blood. (2012) 119:3064–72. doi: 10.1182/blood-2011-06-360321
152. Nakae S, Iikura M, Suto H, Akiba H, Umetsu DT, Dekruyff RH, et al. TIM-1 and TIM-3 enhancement of Th2 cytokine production by mast cells. Blood. (2007) 110:2565–8. doi: 10.1182/blood-2006-11-058800
153. Tan YS, Sansanaphongpricha K, Prince MEP, Sun D, Wolf GT, Lei YL. Engineering vaccines to reprogram immunity against head and neck cancer. J Dent Res. (2018) 97:627–34. doi: 10.1177/0022034518764416
154. Dharmaraj N, Piotrowski SL, Huang C, Newton JM, Golfman LS, Hanoteau A, et al. Anti-tumor immunity induced by ectopic expression of viral antigens is transient and limited by immune escape. Oncoimmunology. (2019) 8:e1568809. doi: 10.1080/2162402X.2019.1568809
155. Li A, Yi M, Qin S, Song Y, Chu Q, Wu K, et al. Activating cGAS-STING pathway for the optimal effect of cancer immunotherapy. J Hematol Oncol. (2019) 12:35. doi: 10.1186/s13045-019-0721-x
156. Li A, Yi M, Qin S, Song Y, Chu, Wu K. STING expression and response to treatment with STING ligands in premalignant and malignantdisease. PLoS ONE. (2017) 12:e0187532. doi: 10.1371/journal.pone.0187532
157. Tan YS, Sansanaphongpricha K, Xie Y, Donnelly CR, Luo X, Heath BR, et al. Mitigating SOX2-potentiated immune escape of Head and Neck Squamous Cell Carcinoma with a STING-inducing nanosatellite vaccine. Clin Cancer Res. (2018) 24: 4242–55. doi: 10.1158/1078-0432.CCR-17-2807
158. Heath BR, Michmerhuizen NL, Donnelly CR, Sansanaphongpricha K, Sun D, Brenner JC, et al. Head and neck cancer immunotherapy beyond the checkpoint blockade. J Dent Res. (2019) 98:1073–80. doi: 10.1177/0022034519864112
159. Baird JR, Friedman D, Cottam B, Dubensky TW Jr, Kanne DB, Bambina S, et al. Radiotherapy combined with novel STING-targeting oligonucleotides results in regression of established tumors. Cancer Res. (2016) 76:50–61. doi: 10.1158/0008-5472.CAN-14-3619
160. Zhang Q, Green MD, Lang X, Lazarus J, Parsels JD, Wei S, et al. Inhibition of ATM increases interferon signaling and sensitizes pancreatic cancer to immune checkpoint blockade therapy. Cancer Res. (2019)79:3940–51. doi: 10.1158/0008-5472.CAN-19-0761
161. Baird JR, Bell RB, Troesch V, Friedman D, Bambina S, Kramer G, et al. Evaluation of explant responses to STING ligands: personalized immunosurgical therapy for head and neck squamous cell carcinoma. Cancer Res. (2018) 78:6308–19. doi: 10.1158/0008-5472.CAN-18-1652
162. Baitsch L, Legat A, Barba L, Fuertes Marraco SA, Rivals JP, Baumgaertner P, et al. Extended co-expression of inhibitory receptors by human CD8 T-cells depending on differentiation, antigen-specificity and anatomical localization. PLoS ONE. (2012) 7:e30852. doi: 10.1371/journal.pone.0030852
163. Matsuzaki J, Gnjatic S, Mhawech-Fauceglia P, Beck A, Miller A, Tsuji T, et al. Tumor-infiltrating NY-ESO-1-specific CD8+ T cells are negatively regulated by LAG-3 and PD-1 in human ovarian cancer. Proc Natl Acad Sci USA. (2010) 107:7875–80. doi: 10.1073/pnas.1003345107
164. Huang RY, Eppolito C, Lele S, Shrikant P, Matsuzaki J, Odunsi K. LAG3 and PD1 co-inhibitory molecules collaborate to limit CD8+ T cell signaling and dampen antitumor immunity in a murine ovarian cancer model. Oncotarget. (2015) 6:27359–77. doi: 10.18632/oncotarget.4751
165. Liu F, Zeng G, Zhou S, He X, Sun N, Zhu X, et al. Blocking Tim-3 or/and PD-1 reverses dysfunction of tumor-infiltrating lymphocytes in HBV-related hepatocellular carcinoma. Bull Cancer. (2018) 105:493–501. doi: 10.1016/j.bulcan.2018.01.018
166. Saloura V, Izumchenko E, Zuo Z, Bao R, Korzinkin M, Ozerov I, Zhavoronkov A, et al. Immune profiles in primary squamous cell carcinoma of the head and neck. Oral Oncol. (2019) 96:77–88. doi: 10.1016/j.oraloncology.2019.06.032
167. Ferris RL, Blumenschein G Jr, Fayette J. Nivolumab for recurrent squamous-cell carcinoma of the head and neck. N Engl J Med. (2016) 375:1856–67. doi: 10.1056/NEJMoa1602252
168. Kim JW, Wieckowski E, Taylor DD, Reichert TE, Watkins S, Whiteside TL. Fas ligand-positive membranous vesicles isolated from sera of patients with oral cancer induce apoptosis of activated T lymphocytes. Clin Cancer Res. (2005) 11:1010–20.
169. Melo SA, Luecke LB, Kahlert C, Fernandez AF, Gammon ST, Kaye J, et al. Glypican-1 identifies cancer exosomes and detects early pancreatic cancer. Nature. (2015) 523:177–82. doi: 10.1038/nature14581
170. Whiteside TL. Exosomes carrying immunoinhibitory proteins and their role in cancer. Clin Exp Immunol. (2017) 189:259–67. doi: 10.1111/cei.12974
171. Whiteside TL. Exosome and mesenchymal stem cell cross-talk in the tumor microenvironment. Semin Immunol. (2018) 35:69–79. doi: 10.1016/j.smim.2017.12.003
172. Razzo BM, Ludwig N, Hong CS, Sharma P, Fabian KP, Fecek RJ, et al. Tumor-derived exosomes promote carcinogenesis of murine oralsquamous cell carcinoma. Carcinogenesis. (2019). doi: 10.1093/carcin/bgz124. [Epub ahead of print].
Keywords: HNSCC, costimulatory signaling molecules, coinhibitory signaling molecules, tumor immunity, immunotherapy
Citation: Liao P, Wang H, Tang Y, Tang Y-J and Liang X (2019) The Common Costimulatory and Coinhibitory Signaling Molecules in Head and Neck Squamous Cell Carcinoma. Front. Immunol. 10:2457. doi: 10.3389/fimmu.2019.02457
Received: 22 July 2019; Accepted: 01 October 2019;
Published: 23 October 2019.
Edited by:
John-Maher, King's College London, United KingdomReviewed by:
Alessandro Poggi, San Martino Hospital (IRCCS), ItalyCopyright © 2019 Liao, Wang, Tang, Tang and Liang. This is an open-access article distributed under the terms of the Creative Commons Attribution License (CC BY). The use, distribution or reproduction in other forums is permitted, provided the original author(s) and the copyright owner(s) are credited and that the original publication in this journal is cited, in accordance with accepted academic practice. No use, distribution or reproduction is permitted which does not comply with these terms.
*Correspondence: Ya-ling Tang, dGFuZ3lhbGluZ0BzY3UuZWR1LmNu; Ya-Jie Tang, eWFqaWV0YW5nQHFxLmNvbQ==; Xin-hua Liang, bHhoODg4NjZAc2N1LmVkdS5jbg==
Disclaimer: All claims expressed in this article are solely those of the authors and do not necessarily represent those of their affiliated organizations, or those of the publisher, the editors and the reviewers. Any product that may be evaluated in this article or claim that may be made by its manufacturer is not guaranteed or endorsed by the publisher.
Research integrity at Frontiers
Learn more about the work of our research integrity team to safeguard the quality of each article we publish.