- Department of Basic Sciences, College of Veterinary Medicine, Mississippi State University, Starkville, MS, United States
Channel catfish farming dominates the aquaculture industry in the United States. However, epidemic outbreaks of motile Aeromonas septicemia (MAS), caused by virulent Aeromonas hydrophila (vAh), have become a prominent problem in the catfish industry. Although vaccination is an effective preventive method, there is no vaccine available against MAS. Recombinant proteins could induce protective immunity. Thus, in this work, vAh ATPase protein was expressed, and its protective capability was evaluated in catfish. The purified recombinant ATPase protein was injected into catfish, followed by experimental infection with A. hydrophila strain ML09-119 after 21 days. Results showed catfish immunized with ATPase exhibited 89.16% relative percent survival after challenge with A. hydrophila strain ML09-119. Bacterial concentrations in liver, spleen, and anterior kidney were significantly lower in vaccinated fish compared with the non-vaccinated sham group at 48 h post-infection (p < 0.05). Catfish immunized with ATPase showed a significant (p < 0.05) higher antibody response compared to the non-vaccinated groups. Overall, ATPase recombinant protein has demonstrated potential to stimulate protective immunity in catfish against virulent A. hydrophila infection.
Introduction
Aquaculture is an approximately $1.2 billion industry, and catfish production is a mainstay of the U.S. aquaculture industry, accounting for $386 million in 2016 (1). Disease outbreaks are among the primary limiting factors in catfish production (2). Infectious diseases account for the most significant percentage of losses, with around 65% of the fry and fingerlings lost during production (3). The three bacterial species responsible for most of these losses are Edwardsiella ictaluri, Flavobacterium columnare, and Aeromonas hydrophila. These pathogens are the causative agents of enteric septicemia of catfish (ESC), columnaris disease, and motile Aeromonas septicemia (MAS), respectively (4, 5).
Since 2009, a clonal group of A. hydrophila strains (referred to as virulent A. hydrophila or vAh) has become a major pathogen of farm-raised channel catfish, causing motile Aeromonas septicemia (MAS) outbreaks (6). The Aquatic Diagnostic Laboratory at Mississippi State University has reported a continued increase of vAh for the past 5 years. The disease is most common in summer months (7). Estimated losses in ponds with disease outbreaks of vAh infection ranged from 4,000 to 10,000 pounds lost (about 8,000–15,000 fish), and pond mortality rates can be very high (close to 100%). vAh is distinguishable from previous Aeromonas catfish isolates, but it is very similar to an Asian grass carp isolate (8). In the last decade, $60–70 million in losses to the U.S. aquaculture industry have been attributed to MAS outbreaks due to mortalities, lost feeding days, and costs associated with antimicrobial therapy (9).
The lack of preventive measures to control vAh infection has emphasized the need to develop techniques for disease prevention. Recombinant protein technology is a promising technology for development of vaccines against many human and animal pathogens (10, 11). To select potential vAh recombinant protein candidates for use as a vaccine, genomic sequences from vAh strain ML09-119 (CP005966.1) were assembled against the genome of A. hydrophila reference strain ATCC 7966T (NC_008570), revealing that A. hydrophila ML09-119, along with all other sequenced vAh strains, contains specific unique outer membrane and secreted proteins (6). These proteins include pilin protein, fimbrial biogenesis outer membrane usher protein, TonB-dependent siderophore receptor protein, TonB-dependent transferrin receptor, OmpA-like protein, and ATPase. We postulate that these proteins could be effective in stimulating protective immunity in catfish against vAh infection.
vAh ATPase has 717 aa and contains two domains. The AAA (ATPases Associated with diverse cellular Activities) domain has 284 aa and is found in the AAA superfamily of ring-shaped P-loop NTPases, which exert their activity through energy-dependent remodeling or translocation of macromolecules (12, 13). The AAA superfamily of ATPases is found in all kingdoms of living organisms and catalyzes many cellular processes in which energy released from ATP hydrolysis is used in molecular remodeling functions (14). In bacteria, ATPases participate in diverse cellular processes including DNA replication, protein degradation, membrane fusion, microtubule severing, peroxisome biogenesis, signal transduction, and regulation of gene expression (15).
The second domain, putative AbiEii toxin domain, is a Type IV toxin-antitoxin (TA) system belonging to the nucleotidyltransferase superfamily (16). It is similar to proteins predicted to be members of the bacterial abortive infection (Abi) system, which enables bacteria to resist bacteriophage infection. Resistance strategies include promoting bacterial death, thus limiting phage replication within a bacterial population. There are 20 or more Abis, and they are predominantly plasmid-encoded lactococcal systems. The putative AbiEii domain is a type of TA system that functions by killing bacteria that lose the plasmid upon division. AbiE phage resistance systems function as novel Type IV TAs and are widespread in bacteria and archaea. Here, we describe the expression and purification of VAh ATPase protein (AHML_21010) and its immune stimulation properties to protect channel catfish against vAh infection.
Materials and Methods
Ethics Statement
Catfish experiments were performed according to guidelines of an approved protocol by the Institutional Animal Care and Use Committee at Mississippi State University.
Bacterial Strains, Media, Plasmid, and Reagents
Escherichia coli strains NovaBlue (Novagen, Madison, WI, USA) and BL21 (DE3) (Invitrogen, Carlsbad, CA, USA) were used for cloning and expression, respectively. E. coli strains were cultured on Luria–Bertani (LB) agar or broth (Difco, Sparks, MD, USA) and incubated at 37°C throughout the study. A. hydrophila strain ML09-119 was cultured in brain heart infusion (BHI) agar or broth (Difco) and incubated at 30°C. Plasmid pET-28a (Novagen) was used as an expression vector. When required, isopropyl-β-D-thiogalactopyranoside (IPTG), kanamycin (Kan, 50 μg/ml), ampicillin (Ap, 100 μg/ml), and colistin (Col, 2.5 μg/ml) (Sigma–Aldrich, Saint Louis, MO, USA) were added to culture media.
Cloning and Expression of ATPase Protein in E. coli
The coding region of ATPase (AHML_21010) was amplified from A. hydrophila strain ML09-119 genomic DNA by PCR using primers ATPaseF01 (AAGGATCCCAAGAGGGTGTTATGTCAGAGC) and ATPaseR01 (AAGTCGACCCTGATGTCCAAGTTCATGTAT). Primers were designed using Primer3 (http://bioinfo.ut.ee/primer3-0.4.0/) based on the ML09-119 genome sequence and synthesized by Sigma-Aldrich. Amplified ATPase region was confirmed by sequencing. For cloning, EcoRI and SacI restriction sites (bold letters) were incorporated into primers' 5′ ends. The amplified ATPase coding region (2,160 bp) was cloned into the EcoRI and SacI restriction sites in pET-28a. Positive clones were selected on LB Kan plates and verified by colony PCR. ATPase sequence was confirmed using T3 and T7 terminator primers. E. coli BL21(DE3) competent bacteria were transformed by positive plasmid using chemical transformation and stored at −80°C in 20% glycerol.
For ATPase protein expression, LB broth containing Kan was inoculated with E. coli BL21 (DE3) (1:100) and cultured at 37°C with shaking at 200 rpm until OD600 reached 0.6–0.8, after which bacteria were induced with 1 mM IPTG and incubated for 8 more h. Whole bacteria proteins were solubilized in tricine sample buffer (Bio-Rad Laboratories, Hercules, CA, USA) for 5 min at 80°C, and protein separation was conducted using 12% SDS-PAGE to confirm expression of ATPase protein. Whole bacteria proteins from competent E. coli BL21 (DE3) and uninduced E. coli BL21 with recombinant clone were used as controls.
Purification of Recombinant ATPase Protein
Recombinant ATPase protein containing six histidine tags (His6) was purified by His-Bind resin columns (Novagen). IPTG induced recombinant bacteria were harvested by centrifugation (12,000 x g for 20 min at 4°C), and pellets were lysed using Bug Buster protein extraction reagent (Novagen) with benzonase nuclease and protease inhibitor cocktail set III (Sigma). Soluble fractions were removed by centrifugation, and recombinant ATPase protein was purified from inclusion body pellets by suspending in lysis buffer (Tris-HCl buffer pH 8.0, 6 M urea) with gentle sonication (4 cycles, 10 s) on ice. After centrifugation, the recombinant protein was loaded onto a resin column (5 mL/column). The resin was washed with wash buffer (0.5 M NaCl, 60 mM imidazole, 20 mM Tris-HCl, pH 7.9), and eluted using elution buffer (1 M imidazole, 0.5 M NaCl, 20 mM Tris-HCl, pH 7.9). Elution fractions were collected for SDS-PAGE analysis. Quantification of the eluted ATPase fractions was determined on a spectrophotometer at 280 nm and Bradford assay (Bio-Rad) according to the supplier's instructions. The identity of recombinant ATPase protein was confirmed by MALDI-TOF mass spectrometry.
Fish Vaccination
Specific pathogen free channel catfish (n = 300; mean weight: 68.77 g) were randomly stocked in 15 40-L tanks (20 fish/tank) supplied with flow-through dechlorinated municipal water and continuous aeration. Fish were acclimated for 1 week by feeding twice a day and monitoring water temperature (30°C) and water quality parameters. Fish were assigned to three groups randomly, and each group included five replicate tanks. Group A consisted of intraperitoneal injection of 100 μl of purified ATPase protein at concentration of 250 μg/ml emulsified with non-mineral oil adjuvant Montanide ISA 763 AVG (Seppic, Paris, France) at a ratio of 30:70 protein to adjuvant. Group B included fish injected with 100 μl of sterile phosphate buffered saline (PBS) emulsified with adjuvant, and group C included fish injected with 100 μl sterile PBS (sham-vaccinated). Fish were anesthetized with tricaine methanesulfonate (MS-222; Sigma) before handling.
At 3 weeks post-immunization, catfish were experimentally infected by bath immersion with 2.8 × 1010 CFU/ml of A. hydrophila ML09-119 for 6 h at 30°C (17). Bacterial infection dose was chosen based on previous experimental infection doses (8, 18). Bacteria numbers (CFU/ml) in the overnight cultures were determined by plating serial 10-fold dilutions on agar plates followed by viable colony counts. At 48 h post-infection, five fish from each group were euthanatized, and liver, spleen, and anterior kidney tissues were collected aseptically. Tissues were homogenized in 1 ml PBS, and tissue suspensions were diluted serially and spread in triplicate on BHI agar plates. Viable bacterial colonies were enumerated after incubating plates at 37°C for 48 h. The remaining ten fish in each group were monitored daily for 2 weeks to assess relative percent survival (RPS), which is calculated by [1– (% mortality of vaccinated fish / % mortality of control fish)] × 100 (19).
Serum Antibody Response
Before and after immunization, blood was collected from the caudal vein of ten fish per group (two fish per tank), and after clotting the blood overnight at 4°C, serum was obtained by centrifugation at 3500 x g for 10 min.
Antibody titers were determined by enzyme-linked immunosorbent assay (ELISA) as described (20). In the whole-bacteria ELISA, 96-well Immulon™ plates (Bloomington, MN, U.S.A.) were coated with heat-killed whole bacteria (108 CFU/ml) overnight at 4°C. For ELISA with purified protein, 96-well plates were coated with 100 μl/well of purified ATPase protein at a concentration of 20 μg/ml in PBS. Subsequently, wells were washed and blocked with 5% nonfat dry milk (Bio-Rad) in PBS for 1 h at room temperature. Wells were washed three times in PBS containing 0.05% Tween-20 (PBS-T). Diluted serum (1:100) was added to each well (50 μl /well), incubated for 1 h at 37°C, and washed with PBS-T. Fifty microliters of a 1:4 dilution of monoclonal antibody 9E1 (anti-catfish Ig) (21, 22) were added to each well. After 1 h incubation at 37°C, plates were washed with PBS-T, and goat anti-mouse antibody conjugate (Fisher Scientific, Pittsburg, PA, USA) was added. Plates were then incubated at room temperature for 1 h and washed. Finally, 100 μl of p-nitrophenyl phosphate substrate (Sigma 104 phosphatase substrate) dissolved in 10% diethanolamine buffer was added to each well, and plates were incubated for 45 min at room temperature. Absorbance at 405 nm was measured in an ELISA Microplate Reader (CA, USA). Control wells containing PBS buffer in place of serum were present in each plate and prepared in the same manner. To standardize, average background absorbance for each plate was subtracted from measured absorbance.
Statistical Analysis
The effect of vaccination with ATPase protein on survival of catfish challenged with vAh was assessed with mixed model logistic regression using PROC GLIMMIX in SAS for Windows 9.4 (SAS Institute, Inc., Cary, NC, USA). The number of live catfish in a tank at the end of the trial was the outcome assessed using an events/trials syntax. Protein was the fixed effect assessed in the model. Tank within protein was included as a random effect in the model. The wild-type strain was the referent for comparisons of protein effect.
Effects of ATPase on the number of CFU in fish tissues and on antibody response were assessed by analysis of variance using PROC GLM. Separate models were used to assess CFU in liver, spleen, and anterior kidney as well as the ELISA results. The CFU data were transformed by first adding 1 to each CFU value and then taking the base 10 logarithm. ELISA data were transformed by taking the base 10 logarithm of each value. The distribution of the residuals was evaluated for each model to determine the appropriateness of the statistical model for the data. If the effect of protein was found to be statistically significant, least squares means were compared using the Dunnett adjustment for multiple comparisons with wild-type strain as the referent. A significance level of 0.05 was used for all analyses.
Results
ATPase Protein Purification
The recombinant protein was purified successfully from soluble fraction at 0.2 mg/ml concentration, and amino acid sequences were confirmed by MALDI-TOF mass spectrometry. The SDS-PAGE result indicated the molecular mass of purified ATPase protein was approximately 81.5 kDa (Figure 1), which was the same size as the deduced molecular mass based on amino acid composition. Protein identification by peptide sequence using MALDI-TOF mass spectrometry revealed 97% identity of the purified protein to ATPase sequence (accession number: AGM45958).
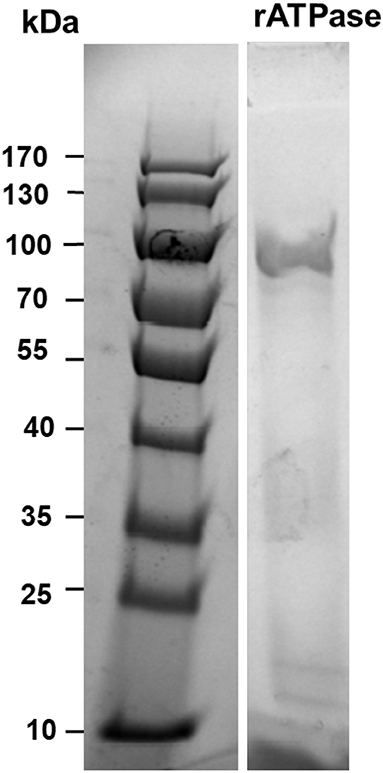
Figure 1. SDS-PAGE with Coomassie blue stain showing purified recombinant ATPase. Molecular weights in kilodaltons are shown for the standard protein marker in the right column. Intervening lanes between the molecular weight marker and the lane containing recombinant ATPase were removed.
Fish Vaccination
Catfish fingerlings immunized with recombinant ATPase protein showed 4.72% mortality (89.16% RPS), which was significantly lower (p < 0.01) than both non-vaccinated groups: PBS-adjuvant (29.55% mortality) and PBS-only (43.51% mortality) groups (Figure 2).
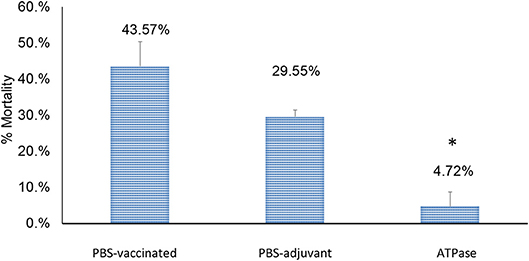
Figure 2. Percent mortalities in catfish vaccinated with recombinant ATPase protein following experimental infection with A. hydrophila ML09-119 at 3 weeks post-vaccination. Significant differences between treatments are indicated with asterisks (p < 0.05).
The mean number of viable bacteria in the liver, spleen, and anterior kidney was significantly lower in fish immunized with recombinant ATPase protein compared to non-vaccinated fish (p < 0.005) (Figure 3).
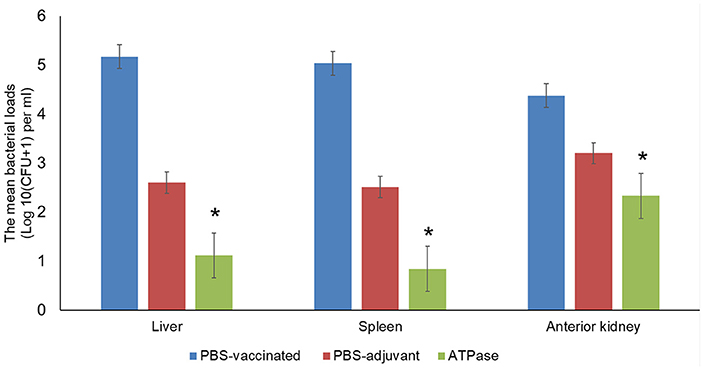
Figure 3. Mean bacterial concentrations (CFU/g) in liver, spleen, and anterior kidney of catfish vaccinated with recombinant ATPase protein at 48 h post-infection with A. hydrophila ML09-119. Data are presented as means ± SE (N = 5). Significant differences between treatments are indicated with asterisks (p < 0.05).
Fish Serum Antibody Response
There was no significant difference (p > 0.05) in antibody response between recombinant ATPase vaccinated and non-vaccinated catfish when ELISA plates were coated with whole bacteria lysate (Figure 4A). In ELISA plates coated with purified protein, significantly higher antibody titers were detected in serum of fish vaccinated with ATPase compared with PBS-only and PBS-adjuvant groups (Figure 4B).
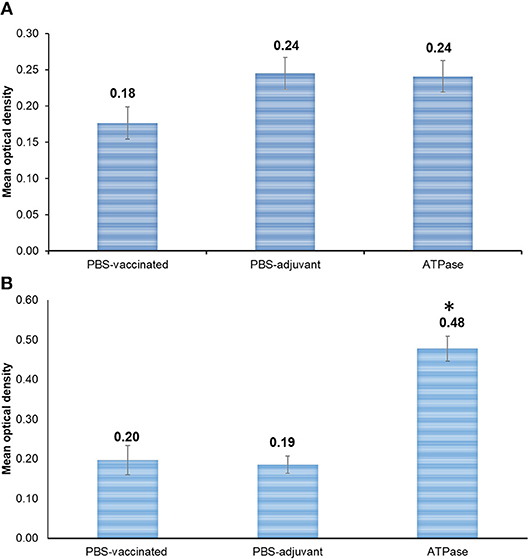
Figure 4. Antibody response in channel catfish serum at day 21 post-vaccination with recombinant ATPase protein. (A) Plates were coated with heat-killed whole bacteria. (B) Plates were coated with purified ATPase protein. Optical densities at 405 nm are means of 10 fish. Vertical bars denote standard errors of the mean. Significant differences are indicated with asterisks (p < 0.05).
Discussion
This study aimed to determine the potential utility of recombinant ATPase protein as a possible vaccine against virulent A. hydrophila, an important pathogen responsible for MAS in catfish. Several research groups have identified candidate DNA or recombinant protein vaccines for MAS (23–28), but to date, there is no protective vaccine available against MAS caused by vAh. Previously, we purified four fimbrial proteins (FimA, Fim, MrfG, and FimOM) and three outer membrane proteins (major outer membrane protein OmpA1, TonB-dependent receptor, and transferrin-binding protein A) and assessed their ability to stimulate protective immunity in channel catfish fingerlings against vAh infection (17, 29). In the present study, expression and purification of vAh ATPase protein in E. coli were successful, and purified ATPase protein was recovered from the inclusion body.
A. hydrophila strain ML09-119 is representative of the vAh clonal group and exhibits high virulence in channel catfish (8, 30). Intraperitoneal injection of catfish with recombinant ATPase protein elicited a higher survival rate compared with non-immunized fish when challenged with vAh. Vaccination with recombinant ATPase protein also effectively reduced colonization of vAh in liver, spleen, and anterior kidney. No statistically significant difference was observed for antibody production in vaccinated vs. non-vaccinated fish when ELISA plates were coated with whole bacterial lysate. However, significant increase in antibody titer was detected in vaccinated fish when ELISA plates were coated with the purified recombinant protein, indicating that ATPase antigen concentration was insufficient in the whole bacterial lysate to detect ATPase-specific antibodies. In fish, antibody titers do not always correlate with protection (31). Protection generated by ATPase in our experiment could be mediated by antibody, and other factors could contribute such as cell-mediated immunity or innate immune components such as complement, lysozyme, antimicrobial peptides, or acute phase proteins (32–34). Innate immunity was stimulated in grass carp (Ctenopharyngodon idella) following immunization with F0F1 ATP synthase subunit beta. This was supported by a significant increase in the expression of pro-inflammatory cytokine genes in blood plasma, including IL-1β, IL-10, TNF-a, CRP, IFN, and MHC II (33). Innate immune response was significantly increased in rainbow trout (Oncorhynchus mykiss) infected with Yersinia ruckeri (35).
In some fish diseases, a combination of humoral, cell-mediated, and innate immune responses work in concert to provide protection. Recombinant outer membrane protein C of Edwardsiella tarda induced a significant innate immune response and humoral immune response in flounder (Paralichthys olivaceus); it also evoked significant protection against E. tarda challenge (36). Up-regulation of the immune-related genes encoding lysozyme G, complement factor 4, immunoglobulin M, β2-microglobulin, major histocompatibility complex I and II, and interleukin-1β was observed in Indian major carp (Labeo rohita) vaccinated with rOmpR, indicating that humoral, cellular, and innate immunity contribute to the protective response against A. hydrophila infection (34). In channel catfish, genes encoding iron homeostasis, transport proteins, complement components, acute phase response, and inflammatory and humoral immune response were upregulated following E. ictaluri infection, indicating a multifactorial catfish immune response against this pathogen (32).
Despite the considerable research on function of proteins in the AAA ATPases superfamily, there is not much information about their use as vaccine antigens or contribution to virulence (37). A few studies have utilized ATPase as a vaccine antigen against protozoan parasites in animal models. For example, DNA immunization of mice with Na+-K+ATPase from Strongyloides stercoralis induced protective immunity and a significant reduction in larval survival, thus suggesting that the Na+-K+ATPase may be a good potential target for the immune response (38). Vaccination with a recombinant chlamydial ATPase protein combined with Alum adjuvant resulted in reduction of the number of viable Chlamydophila pneumoniae in lungs of mice, indicating that chlamydial ATPase induces protective immunity in mice (39).
Another strategy investigated the use of ATPase for vaccine development in the form of fusion proteins. For example, a surface protein (TcSP2) of Trypanosoma cruzi fused to ATPase (ATP) domains of heat shock protein 70 (TcHSP70) induced high antibody titers and increased survival in immunized mice after T. cruzi infection (40). Moreover, TcHSP70, as well as an internal fragment of 242 amino acids within the ATPase domain, activated the maturation of dendritic cells macrophages to produce proinflammatory cytokines and chemokines in a mouse model (41).
No information is available on ATPase as a recombinant antigen in fish or other animals against bacterial pathogen infections. However, some proteins characterized by ATPase activity were successfully used as subunit vaccines against fish bacterial pathogens. For example, heat shock proteins (HSP 60 and HSP 70) have a N-terminal ATPase domain and are usually in an ATP bound state (42, 43). Wilhelm et al. (44) reported protection of salmon (95% RPS) against Piscirickettsia salmonis following immunization with HSP as a recombinant vaccine (44), and Sudheesh et al. (45) found that HSP60, HSP70, and two other proteins (ATP synthase and thermolysin) were highly immunogenic proteins against Flavobacterium psychrophilum (45).
In conclusion, ATPase was successfully expressed and purified using a pET-28a vector. The recombinant ATPase protein protected catfish against vAh infection and significantly reduced bacterial quantities in catfish tissue, and it stimulated significant antibody titers against the protein. This is the first study to report an ATPase protein as a potential vaccine for a bacterial disease in fish.
Ethics Statement
Catfish experiments were performed according to guidelines of an approved protocol by the Institutional Animal Care and Use Committee at Mississippi State University.
Author Contributions
ML, AK, and HA designed the experiments. ML supervised the overall project. HA performed the laboratory work, analyzed the data, and wrote the manuscript. MB helped with fish experiments and ELIZA. AK and ML reviewed the manuscript.
Funding
This work was funded by Agriculture and Food Research Initiative Competitive Grant no. 2013-67015-21313 from the USDA National Institute of Food and Agriculture. Part of HA salary was provided by the Center for Biomedical Research Excellence in Pathogen–Host Interactions, National Institute of General Medical Sciences, National Institutes of Health awarded grant number P20GM103646-05.
Conflict of Interest Statement
The authors declare that the research was conducted in the absence of any commercial or financial relationships that could be construed as a potential conflict of interest.
Acknowledgments
We thank Dr. Robert Wills at Mississippi State University for his assistance with statistical analyses. We are grateful for Lionel Genix at SEPPIC INC for providing the adjuvant used in this study. We thank John Harkness for proof reading this manuscript. Finally, we thank Laboratory Animal Resources and Care (LARAC) at the Mississippi State University College of Veterinary Medicine for providing us with SPF catfish.
References
1. USDA. Catfish Production, by the National Agricultural Statistics Service (NASS). Agricultural Statistics Board, United States Department of Agriculture (USDA) (2017). Available online at: https://downloads.usda.library.cornell.edu/usda-esmis/files/bg257f046/1v53k047h/fj236430r/CatfProd-02-03-2017.pdf
2. Lewis DH, Plumb JA. Bacterial diseases. In: Plumb JA, editor. Principal Diseases of Farm Raised Catfish. South. Coop. Ser. Bull No. 225. (1985). pp. 13–7.
3. Center for Emerging Issues (U.S.). Assessing Infectious Disease Emergence Potential in the U.S. Aquaculture Industry [electronic resource]: Phase 1, U.S. Aquaculture Industry Profile. Fort Collins, CO: U.S. Dept. of Agriculture, Animal and Plant Health Inspection Service, Veterinary Services, Center for Emerging Issues (2007).
4. Wagner BA, Wise DJ, Khoo LH, Terhune JS. The epidemiology of bacterial diseases in food-size channel catfish. J Aquat Anim Health. (2006) 18:263–72. doi: 10.1577/1548-8667(2002)014<0263:TEOBDI>2.0.CO;2
5. Plumb JA, Hanson LA. Catfish bacterial diseases. In: Plumb JA, Hanson LA, editors. Health Maintenance and Principal Microbial Diseases of Cultured Fishes. (2011). p. 275–313. doi: 10.1002/9780470958353.ch11
6. Hemstreet B. An update on Aeromonas hydrophila from a fish health specialist for summer 2010. Catfish J. (2010) 24.
7. Baumgartner WA, Ford L, Hanson L. Lesions caused by virulent Aeromonas hydrophila in farmed catfish (Ictalurus punctatus and I. punctatus x I. furcatus) in Mississippi. J Vet Diagn Invest. (2017) 29:747–51. doi: 10.1177/1040638717708584
8. Hossain MJ, Sun D, McGarey DJ, Wrenn S, Alexander LM, Martino ME, et al. An Asian origin of virulent Aeromonas hydrophila responsible for disease epidemics in United States-farmed catfish. MBio. (2014) 5:e00848–14. doi: 10.1128/mBio.00848-14
9. Dunhua Zhang, De-Hai Xu, Craig Shoemaker, Hemstreet WB. Virulent Aeromonas hydrophila in channel catfish. In: Global Aquaculture Advocate. (2016). Available online at: https://m.2lua.vn/article/virulent-aeromonas-hydrophila-in-channel-catfish-58744b32e4951993688b456b.html
10. Vishwanath S, McDonald GA, Watkins NG. A recombinant Rickettsia conorii vaccine protects guinea pigs from experimental boutonneuse fever and Rocky Mountain spotted fever. Infect Immun. (1990) 58:646–53.
11. Kuzyk MA, Burian J, Machander D, Dolhaine D, Cameron S, Thornton JC, et al. An efficacious recombinant subunit vaccine against the salmonid rickettsial pathogen Piscirickettsia salmonis. Vaccine. (2001) 19:2337–44. doi: 10.1016/S0264-410X(00)00524-7
12. Iyer LM, Leipe DD, Koonin EV, Aravind L. Evolutionary history and higher order classification of AAA+ ATPases. J Struct Biol. (2004) 146:11–31. doi: 10.1016/j.jsb.2003.10.010
13. Frickey T, Lupas AN. Phylogenetic analysis of AAA proteins. J Struct Biol. (2004) 146:2–10. doi: 10.1016/j.jsb.2003.11.020
14. Hanson PI, Whiteheart SW. AAA+ proteins: have engine, will work. Nat Rev Mol Cell Biol. (2005) 6:519–29. doi: 10.1038/nrm1684
15. Erzberger JP, Berger JM. Evolutionary relationships and structural mechanisms of AAA+ proteins. Annu Rev Biophys Biomol Struct. (2006) 35:93–114. doi: 10.1146/annurev.biophys.35.040405.101933
16. Kuchta K, Knizewski L, Wyrwicz LS, Rychlewski L, Ginalski K. Comprehensive classification of nucleotidyltransferase fold proteins: identification of novel families and their representatives in human. Nucleic Acids Res. (2009) 37:7701–14. doi: 10.1093/nar/gkp854
17. Abdelhamed H, Nho SW, Turaga G, Banes MM, Karsi A, Lawrence ML. Protective efficacy of four recombinant fimbrial proteins of virulent Aeromonas hydrophila strain ML09-119 in channel catfish. Vet Microbiol. (2016) 197:8–14. doi: 10.1016/j.vetmic.2016.10.026
18. Abdelhamed H, Ibrahim I, Baumgartner W, Lawrence ML, Karsi A. Characterization of histopathological and ultrastructural changes in channel catfish experimentally infected with virulent Aeromonas hydrophila. Front Microbiol. (2017) 8:1519. doi: 10.3389/fmicb.2017.01519
19. Amend DF. Potency testing of fish vaccines. Developments in Biological Standardization. Sci Res. (1981) 49:447–54.
20. Waterstrat PR, Ainsworth AJ, Capley G. In vitro responses of channel catfish, Ictalurus punctatus, neutrophils to Edwardsiella ictaluri. Dev Comp Immunol. (1991) 15:53–63. doi: 10.1016/0145-305X(91)90047-3
21. Lobb CJ, Clem LW. Fish lymphocytes differ in the expression of surface immunoglobulin. Dev Comp Immunol. (1982) 6:473–9. doi: 10.1016/S0145-305X(82)80033-5
22. Miller NW, Bly JE, van Ginkel F, Ellsaesser CF, Clem LW. Phylogeny of lymphocyte heterogeneity: identification and separation of functionally distinct subpopulations of channel catfish lymphocytes with monoclonal antibodies. Dev Comp Immunol. (1987) 11:739–47. doi: 10.1016/0145-305X(87)90061-9
23. Wang N, Yang Z, Zang M, Liu Y, Lu C. Identification of Omp38 by immunoproteomic analysis and evaluation as a potential vaccine antigen against Aeromonas hydrophila in Chinese breams. Fish Shellfish Immunol. (2013) 34:74–81. doi: 10.1016/j.fsi.2012.10.003
24. Maiti B, Shetty M, Shekar M, Karunasagar I. Evaluation of two outer membrane proteins, Aha1 and OmpW of Aeromonas hydrophila as vaccine candidate for common carp. Vet Immunol Immunopathol. (2012) 149:298–301. doi: 10.1016/j.vetimm.2012.07.013
25. Wu L, Jiang YN, Tang Q, Lin HX, Lu CP, Yao HC. Development of an Aeromonas hydrophila recombinant extracellular protease vaccine. Microb Pathog. (2012) 53:183–8. doi: 10.1016/j.micpath.2012.07.007
26. Khushiramani RM, Maiti B, Shekar M, Girisha SK, Akash N, Deepanjali A, et al. Recombinant Aeromonas hydrophila outer membrane protein 48 (Omp48) induces a protective immune response against Aeromonas hydrophila and Edwardsiella tarda. Res Microbiol. (2012) 163:286–91. doi: 10.1016/j.resmic.2012.03.001
27. Yeh HY, Klesius PH. Over-expression, purification and immune responses to Aeromonas hydrophila AL09-73 flagellar proteins. Fish Shellfish Immunol. (2011) 31:1278–83. doi: 10.1016/j.fsi.2011.09.016
28. Guan R, Xiong J, Huang W, Guo S. Enhancement of protective immunity in European eel (Anguilla anguilla) against Aeromonas hydrophila and Aeromonas sobria by a recombinant Aeromonas outer membrane protein. Acta Biochim Biophys Sin. (2011) 43:79–88. doi: 10.1093/abbs/gmq115
29. Abdelhamed H, Ibrahim I, Nho SW, Banes MM, Wills RW, Karsi A, et al. Evaluation of three recombinant outer membrane proteins, OmpA1, Tdr, and TbpA, as potential vaccine antigens against virulent Aeromonas hydrophila infection in channel catfish (Ictalurus punctatus). Fish Shellfish Immunol. (2017) 66:480–6. doi: 10.1016/j.fsi.2017.05.043
30. Tekedar HC, Waldbieser GC, Karsi A, Liles MR, Griffin MJ, Vamenta S, et al. Complete genome sequence of a channel catfish epidemic isolate, Aeromonas hydrophila strain ML09-119. Genome Announc. (2013) 1:e00755-13. doi: 10.1128/genomeA.00755-13
31. Ellis AE. Immunity to bacteria in fish. Fish Shellfish Immunol. (1999) 9:291–308. doi: 10.1006/fsim.1998.0192
32. Peatman E, Baoprasertkul P, Terhune J, Xu P, Nandi S, Kucuktas H, et al. Expression analysis of the acute phase response in channel catfish (Ictalurus punctatus) after infection with a Gram-negative bacterium. Dev Comp Immunol. (2007) 31:1183–96. doi: 10.1016/j.dci.2007.03.003
33. Luo Z, Fu J, Li N, Liu Z, Qin T, Zhang X, et al. Immunogenic proteins and their vaccine development potential evaluation in outer membrane proteins (OMPs) of Flavobacterium columnare. Aquaculture Fish. (2016) 1:1–8. doi: 10.1016/j.aaf.2016.10.002
34. Dash P, Sahoo PK, Gupta PK, Garg LC, Dixit A. Immune responses and protective efficacy of recombinant outer membrane protein R (rOmpR)-based vaccine of Aeromonas hydrophila with a modified adjuvant formulation in rohu (Labeo rohita). Fish Shellfish Immunol. (2014) 39:512–23. doi: 10.1016/j.fsi.2014.06.007
35. Raida MK, Buchmann K. Innate immune response in rainbow trout (Oncorhynchus mykiss) against primary and secondary infections with Yersinia ruckeri O1. Dev Com Immunol. (2009) 33:35–45. doi: 10.1016/j.dci.2008.07.001
36. Liu F, Tang X, Sheng X, Xing J, Zhan W. Edwardsiella tarda outer membrane protein C: an immunogenic protein induces highly protective effects in flounder (Paralichthys olivaceus) against Edwardsiellosis. Int J Mol Sci. (2016) 17:1117. doi: 10.3390/ijms17071117
37. Snider J, Thibault G, Houry WA. The AAA+ superfamily of functionally diverse proteins. Genome Biol. (2008) 9:216. doi: 10.1186/gb-2008-9-4-216
38. Kerepesi LA, Keiser PB, Nolan TJ, Schad GA, Abraham D, Nutman TB. DNA immunization with Na+-K+ ATPase (Sseat-6) induces protective immunity to larval Strongyloides stercoralis in mice. Infect Immun. (2005) 73:2298–305. doi: 10.1128/IAI.73.4.2298-2305.2005
39. Szabó AM, Sipák Z, Miczák A, Faludi I. ABC transporter ATPase of Chlamydophila pneumoniae as a potential vaccine candidate. Acta Microbiol Immunol Hung. (2013) 60:11–20. doi: 10.1556/AMicr.60.2013.1.2
40. Carabarin-Lima A, González-Vázquez MC, Baylon-Pacheco L, Patricia-Talamas R. Immunization with the recombinant surface protein rTcSP2 alone or fused to the CHP or ATPase domain of TcHSP70 induces protection against acute Trypanosoma cruzi Infection. J Vaccin Vaccinat. (2010) 1:130. doi: 10.4172/2157-7560.1000110
41. Planelles L, Thomas M, Pulgar M, Maranon C, Grabbe S, Lopez MC. Trypanosoma cruzi heat-shock protein-70 kDa,alone or fused to the parasite KMP11 antigen, induces functional maturation of murine dendritic cells. Immunol Cell Biol. (2002) 80:241–7. doi: 10.1046/j.1440-1711.2002.01081.x
42. Flaherty KM, DeLuca-Flaherty C, McKay DB. Three-dimensional structure of the ATPase fragment of a 70K heat-shock cognate protein. Nature. (1990) 346:623–8. doi: 10.1038/346623a0
43. Chamberlain LH, Burgoyne RD. Activation of the ATPase activity of heat-shock proteins Hsc70/Hsp70 by cysteine-string protein. Biochem J. (1997) 322:853–8. doi: 10.1042/bj3220853
44. Wilhelm V, Soza C, Martinez R, Rosemblatt M, Burzio LO, Valenzuela PD. Production and immune response of recombinant Hsp60 and Hsp70 from the salmon pathogen Piscirickettsia salmonis. Biol Res. (2005) 38:69–82. doi: 10.4067/S0716-97602005000100009
45. Sudheesh PS, LaFrentz BR, Call DR, Siems WF, LaPatra SE, Wiens GD, et al. Identification of potential vaccine target antigens by immunoproteomic analysis of a virulent and a non-virulent strain of the fish pathogen Flavobacterium psychrophilum. Dis Aquat Organ. (2007) 74:37–47. doi: 10.3354/dao074037
Keywords: Aeromonas hydrophila, motile Aeromonas septicemia, aquaculture, recombinant vaccine, catfish
Citation: Abdelhamed H, Banes M, Karsi A and Lawrence ML (2019) Recombinant ATPase of Virulent Aeromonas hydrophila Protects Channel Catfish Against Motile Aeromonas Septicemia. Front. Immunol. 10:1641. doi: 10.3389/fimmu.2019.01641
Received: 31 October 2018; Accepted: 01 July 2019;
Published: 16 July 2019.
Edited by:
Hetron Mweemba Munang'andu, Norwegian University of Life Sciences, NorwayReviewed by:
Biswajit Maiti, Nitte University, IndiaGirisha S. Kallappa, Karnataka Veterinary, Animal and Fisheries Sciences University, India
Copyright © 2019 Abdelhamed, Banes, Karsi and Lawrence. This is an open-access article distributed under the terms of the Creative Commons Attribution License (CC BY). The use, distribution or reproduction in other forums is permitted, provided the original author(s) and the copyright owner(s) are credited and that the original publication in this journal is cited, in accordance with accepted academic practice. No use, distribution or reproduction is permitted which does not comply with these terms.
*Correspondence: Mark L. Lawrence, lawrence@cvm.msstate.edu