- 1Neuroscience Training Program, University of Wisconsin-Madison, Madison, WI, United States
- 2Department of Comparative Biosciences, University of Wisconsin-Madison, Madison, WI, United States
Sleep disordered breathing (SDB) affects 3–5% of the pediatric population, including neonates who are highly susceptible due to an underdeveloped ventilatory control system, and REM-dominated sleep. Although pediatric SDB is associated with poor cognitive outcomes, very little research has focused on models of pediatric SDB, particularly in neonates. In adults and neonates, intermittent hypoxia (IH), a hallmark of SDB, recapitulates multiple physiological aspects of severe SDB, including neuronal apoptosis, sex-specific cognitive deficits, and neuroinflammation. Microglia, resident CNS immune cells, are important mediators of neurodevelopment and neuroinflammation, but to date, no studies have examined the molecular properties of microglia in the context of neonatal IH. Here, we tested the hypothesis that neonatal IH will enhance microglial inflammation and sex-specifically lead to long-term changes in working memory. To test this hypothesis, we exposed post-natal day (P1) neonates with dams to an established adult model of pathological IH consisting of 2 min cycles of 10.5% O2 followed by 21% O2, 8 h/day for 8 days. We then challenged the offspring with bacterial lipopolysaccharide (LPS) at P9 or at 6–8 weeks of age and immunomagnetically isolated microglia for gene expression analyses and RNA-sequencing. We also characterized neonatal CNS myeloid cell populations by flow cytometry analyses. Lastly, we examined working memory performance using a Y-maze in the young adults. Contrary to our hypothesis, we found that neonatal IH acutely augmented basal levels of microglial anti-inflammatory cytokines, attenuated microglial responses to LPS, and sex-specifically altered CNS myeloid populations. We identified multiple sex differences in basal neonatal microglial expression of genes related to chemotaxis, cognition, and aging. Lastly, we found that basal, but not LPS-induced, anti-inflammatory cytokines were augmented sex-specifically in the young adults, and that there was a significant interaction between sex and IH on basal working memory. Our results support the idea that neonates may be able to adapt to IH exposures that are pathological in adults. Further, they suggest that male and female microglial responses to IH are sex-specific, and that these sex differences in basal microglial gene expression may contribute to sexual dimorphisms in vulnerability to IH-induced cognitive disruption.
Introduction
Obstructive sleep apnea (OSA), a form of sleep disordered breathing (SDB) characterized by repetitive episodes of intermittent hypoxia (IH), is present in up to 5% of the total pediatric population including infants (1). Further, up to 80% of infants with craniofacial abnormalities, such as those associated with Down Syndrome will present with OSA (2), and some evidence suggests a higher occurrence in males (3). Despite the common occurrence of OSA in infants, it remains poorly characterized in terms of clinical presentation, partly due to its co-morbidity with other early life complications (4, 5). In addition to the poorly characterized clinical presentations, the long-term effects of infant OSA are unknown. In older pediatric populations, OSA is associated with poor behavioral outcomes and neurocognitive dysfunction (6). Although vulnerable infant populations (i.e., preterm or low-birthweight) are at risk for OSA (7), systemic infection (8), and adverse neurodevelopment (9), little is known about how OSA contributes to these co-morbid processes.
Rodent models of OSA suggest IH alone recapitulates multiple deficits that characterize OSA, including neuronal apoptosis (10), peripheral and central inflammation (11, 12), and cognitive deficits (13). In neonates, long term exposure to IH causes lower weight gain (14), hippocampal apoptosis (15), hypomyelination (16), changes in sympathetic nerve activity (17–19) and attenuation of adult male spatial memory (10). Additionally, neonatal IH enhances the male, but not female, hypoxic ventilatory response (20). Thus, IH recapitulates some of the sex-differences observed in human neonatal populations, and suggests that similar to the findings for preterm infants [reviewed in (21)], males may be a particularly vulnerable population.
Microglia, CNS resident macrophages, are important mediators of both neuroinflammation and neurodevelopmental processes (22–28), including learning and memory (29). Further, changes in microglia morphology and/or function are associated with abnormal neurodevelopment (30–32). Preterm infants, who are susceptible to both OSA (7) and long-term cognitive dysfunction (33), have altered microglia accumulation at sites of white matter injury (34). In adult rats, IH augments microglial expression of classic inflammatory molecules such as toll-like receptor 4 (TLR4), cyclooxygenase-2 (COX-2), inducible nitrogen oxide synthase (iNOS), and interleukin-1 (IL-1) (11), and attenuation of inflammation using inhibitors of COX-2 and iNOS improves cognitive outcomes (13, 35). While pediatric OSA and neonatal IH are associated with systemic and carotid body inflammation (19, 36–38), it is unknown if OSA or IH alters microglial inflammation during postnatal development, or if this is associated with differences in cognitive outcomes.
Similar to neonatal hypoxic responses, there are developmental sex differences in microglia, including their morphology (39) and dynamics (40), which, when perturbed, may account for the sex differences in neurodevelopmental disorders (41) [reviewed in (42)]. The phagocytic capacity of microglia is also sexually dimorphic, with female microglia phagocytosing more neural progenitor cells in the early postnatal period (43). Although not yet directly linked to a microglial source, brain expression of key inflammatory cytokines, such as interleukin-1β, and C-x-c motif cluster inflammatory chemokines including Cxcl9, Cxcl10, and Cxcl11, also differs between males and females in the neonatal rat (39), suggesting basal sex differences in microglial inflammatory responses. The sex-specific effects of neonatal IH on microglial expression of these immune related genes has never been tested.
We thus hypothesized that neonatal IH would sex-specifically augment microglial pro-inflammatory gene expression both acutely and long-term, and correlate with long-term deficits in working memory. Surprisingly, we found that neonatal IH enhanced gene expression related to type I interferon responses and “anti-inflammatory” signaling in both males and females, effects which persisted long-term in males. Additionally, in the presence of systemic inflammation, IH-exposed females, but not males had attenuated cytokine expression, and enrichment of a putative CNS monocyte-derived population. RNAseq analyses of male and female microglia confirmed these results and identified sex differences in genes related to leukocyte chemotaxis and cognition. Finally, behavioral analyses of adults exposed to IH as neonates demonstrated that while there was a significant interaction between the effects of IH and sex, neonatal IH had no effect on working memory.
Methods
Animals and Intermittent Hypoxia (IH) Exposures
All animal experimental procedures were performed according to the NIH guidelines set forth in the Guide for the Care and Use of Laboratory Animals and were approved by the University of Wisconsin-Madison Institutional Animal Care and Use Committee. Timed-pregnant Sprague-Dawley rats (E16) were obtained from Charles River (RRID:RGD_734476; Wilmington, MA) and housed in AAALAC-accredited facilities with 12 h:12 h light–dark conditions. Food and water were provided ad libitum. Beginning at postnatal day (P) 1, dams with neonates were exposed to 8 days of an IH paradigm which consisted of alternating 2-min hypoxic (45 s down to 10.5% O2) and normoxic (15 s up to 21% O2) episodes for 8 h/day (9:00 am−5:00 pm) as previously reported (44). This produces an apnea hypopnea index (AHI) of 15 events/h which mimics kinetics of moderate sleep apnea in pediatric populations and adults (45). Normoxic (Nx) controls received alternating room air. At ~16 h or 6 weeks following the final IH episode, animals were weighed and injected intraperitoneally with either HBSS vehicle (Cellgro; Herndon, VA) or a sublethal 0.1 mg/kg lipopolysaccharide (LPS E. coli O11:B4; 500,000 EU/mg; Sigma-Aldrich; St. Louis, MO) for 3 h. Following LPS, pups were sacrificed via decapitation and brains isolated in HBSS for removal of meninges and blood. Pulse-oximetry was performed at P1 on sentinel rats (n = 4) using STARR MouseOx Rev 6.3.13; these rats were excluded from microglial analyses.
Microglia Isolation
CD11b/c+ cells were immunomagnetically isolated from the whole brain (minus the cerebellum and olfactory bulbs) as previously described (11) using the Miltenyi OX-42 antibody (Miltenyi Biotec Inc., Auburn, CA Cat# 130-105-634). Myelin was removed using 26% Percoll (GE HealthCare; Madison, WI). Cells were then either resuspended in a modified zinc-based fixative (mZBF) for subsequent flow cytometry analyses (46) or column separated according to the Miltenyi MACs protocol. Column separation results in 97.9% viable cells and 88–96% of CD11b+ cells were microglia (CD11b/CD45low) (47). Isolated CD11b+ cells were resuspended in TriReagent (Invitrogen, Carlsbad, CA), and total RNA extracted according to the manufacturer's instructions.
Quantitative RT-PCR
cDNA was synthesized as previously described (48). MMLV Reverse Transcriptase was purchased from Invitrogen. Oligo dT, Random Primers, and RNAse inhibitor were purchased from Promega (Madison, WI). qRT-PCR using power SYBR green (ThermoFisher Scientific) was performed as previously described using an Applied Biosystems 7500 Fast Real Time PCR System (11). All primers (Table 1) were tested for efficiency using serial dilutions, and results were normalized to 18S RNA levels; data analyses were performed using the delta-delta CT method (49).
PCR Gene Array
cDNA for microglia derived from male Nx LPS- and IH LPS-treated neonates was synthesized as for RT-PCR, aliquotted to the Qiagen (Hilden, Germany) NF-κB Signaling Targets RT2 Profiler PCR Array (cat # PARN-225Z) and run on the Applied Biosystems 7500 Fast Real Time PCR System. Results were analyzed using Qiagen software and exported to Microsoft Excel to generate heatmaps.
Total RNA Sequencing and Bioinformatics Analyses
Total microglial RNA was isolated from neonatal pups exposed to 8 days of IH followed by vehicle or LPS challenge. Three biological replicates from each treatment were submitted to the UW-Madison Biotechnology Resource Center for sequencing and quality control. Stranded cDNA libraries were prepared using the Truseq Stranded mRNA kit (Illumina; San Diego, CA). Sequencing was performed using an Illumina HiSeq 2500. After sequencing and quality control, Fastq files containing ~10 million reads for each biological replicate (n = 3/treatment) were aligned using TopHat 2.1.0 with the following parameters: –no-novel-juncs, – Library type fr – firststrand. BAM files were converted to SAM with SAMtools. Read counts were obtained using HTSeq v. 0.6.1 with the stranded reverse option. Count files were imported to R and filtered such that only genes with a CPM >1 expressed in three samples were retained. Counts were normalized using the Trimmed Mean of M-Values method (TMM), and analyzed for differential expression using EdgeR (50, 51). Genes with an FDR < 0.05 were considered differentially expressed. Results were uploaded to NCBI Geo Datasets (GEO GSE126946). To specifically probe RNA-seq data for changes in NFκB signaling target genes obtained from the Qiagen PCR array (section PCR Gene Array), NFκB target genes were ranked by p-value and FDRs calculated for the specific set of genes. NFκB target genes with an FDR < 0.05 were considered differentially expressed. Gene ontology was performed on differentially expressed genes using both DAVID (52, 53) and Panther (54) for GO class Biological Process. For GO analyses, background files were generated using the filtered count files. Motif analysis of rat promoters for gene lists was performed using the find.Motifs.pl script in Homer (55). Protein-protein interaction networks were created using STRING (56).
Flow Cytometry
Single cell suspensions of P9 whole brain homogenates were created by pushing the tissue through 40 μm filter with ice-cold HBSS supplemented with 0.01 mg/ml DNase and 0.1 mM EDTA. Myelin was removed by high-speed centrifugation at 850 g for 15 min in a solution consisting of 26% Percoll in 0.1 M PBS. Dissociated cells were washed in ice-cold HBSS and pelleted by centrifugation at 350 g for 5 min. Cells were resuspended in mZBF (0.5% zinc chloride, 0.5% zinc trifluoroacetate, 0.05% calcium acetate in 0.1 M Tris–HCL, pH 6.4–6.7) and glycerol (1:1) (46). Prior to fixation, cells were incubated with eFluor 780 live/dead (eBioscience cat # 65-0865-14). The following mouse anti-rat antibodies were used: CD11b/c-PE (BD Pharmingen cat # 554862; RRID:AB_395562), CD45-PE-Cy7 (BD Pharmingen cat # 561588; RRID:AB_10893200), and CD4-APC (BD Pharmingen cat # 550057; RRID:AB_398458). Flow cytometry was performed using a BD Fortessa Flow Cytometer (gating strategy, Supplementary Figure 1). FCS files were analyzed using FlowJO v.10.0.7 software.
Spontaneous Alternation Test
The Y-maze is a measure of working memory and was performed as previously described (57, 58). Animals were between 6 and 8 weeks of age and behaviorally naïve prior to the start of testing. Arm entry was quantified when an animal had its hind paws completely within a maze arm. Animals were placed into the Y-maze and allowed 15 min to explore. Analysis of arm entries began after the animal entered each arm once and arm choices were recorded for 10 min. The number of times 3 successive arm alternations were made correctly (clockwise or counterclockwise) was summed and then divided by the total number of arm entries minus two. The resulting number is the % of spontaneous alternations, with 50% being considered chance levels.
Statistical Analyses
All statistical analyses were performed using SigmaPlot (Systat Software, San Jose CA) unless otherwise noted. Data that were not normally distributed were natural log transformed. The Grubb's Test was used to identify outliers. Statistical significance (set at p < 0.05) was determined using a Two-way analysis of variance (Two-way ANOVA) or Student's t-test, followed by a Holm-Sidak post hoc test when applicable. P-values between 0.05 and 0.1 were considered to be statistical trends.
Results
Neonatal IH for 8 Days Reduces SpO2 and Weight in Both Males and Females and Increases CNS Cell Death
To test the effects of 8 days of neonatal IH on microglial gene expression, we used a model of IH that is used in adult rat microglial studies (11) and is similar to models used in neonate studies (14, 38). We recorded blood oxygen saturation (SpO2) using pulse-oximetry at P1 (Figure 1A). Weights were recorded after 8 days of Nx or IH, just prior to LPS injections (Figure 1B). IH reduced SpO2 to just below 60% in both males and females. Additionally, pups exposed to 8 days of IH weighed less than their normoxic counterparts by P9; this was true for both males (p = 0.032, n = 20/tx) and females (p < 0.001, n = 20/tx) and is consistent with previous observations (14). In a separate cohort of animals, whole brains were isolated to assess cell death by flow cytometric analysis (Figure 1C). Using the live/dead dye eFluor 780, we found that IH significantly increased the percent of labeled cells (p = 0.017, n = 12/tx, mixed sex), indicating that IH induces neonatal CNS cell death. Thus, this model of IH which mimics moderate OSA and an infant apnea-hypopnea index (AHI) of 15 (45) causes blood O2 desaturations, reduces weight gain, and increases CNS cell death.
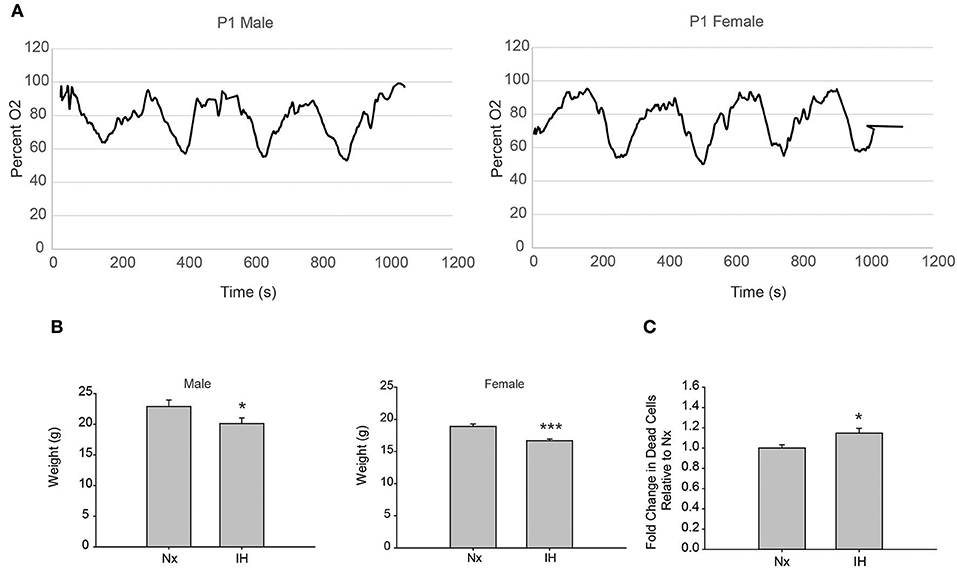
Figure 1. Neonatal IH decreases SpO2 and weight. (A) Pulse-oximetry was recorded in P1 neonatal males (left) and females (right). (B) At P9, after 8 days of Nx or IH exposure, male (left; n = 20/tx) and female (right; n = 20/tx) pups were weighed. (C) At P9 after 8 days of Nx or IH exposures, whole brains from male (n = 6/treatment) and female (n = 6/treatment) pups were isolated for flow cytometric analysis of cell death. *p < 0.05, ***p < 0.001, t-test.
Neonatal IH Sex-Specifically Upregulates Microglial Anti-inflammatory Cytokine and Attenuates LPS-Induced Cytokine Gene Expression
Based on observations in adult rats, we predicted that IH exposure would enhance microglial expression of classic pro-inflammatory cytokines/enzymes under basal and inflammatory (LPS) conditions. Prior to testing this prediction in all treatments and samples, we performed a preliminary screen for candidate genes altered by IH in whole brain microglia immunomagnetically isolated from LPS-treated pups exposed to 8 days of either Nx or IH, using the Qiagen NF-κB Signaling Pathway PCR Array (Figure 2). These preliminary results suggested that the most pronounced gene expression changes (with fold changes > 4 in IH LPS vs. Nx LPS) were not in classic inflammatory cytokines as we had originally hypothesized. Rather, the changes appeared to be in cytokines related to anti-inflammatory responses or T-helper cell responses, such as interferon-β (Ifnβ1), interleukin-4 (Il4), and interleukin-2 (Il2). In further support of this anti-inflammatory effect in microglia isolated from the IH-exposed neonate, IH exposure tended to attenuate LPS-induced inflammatory gene expression instead of augmenting it (Figure 2). Thus, for the remaining experiments, we focused on genes identified in the array, in addition to the key inflammatory cytokines/enzymes identified from previous microglia research (11, 39).
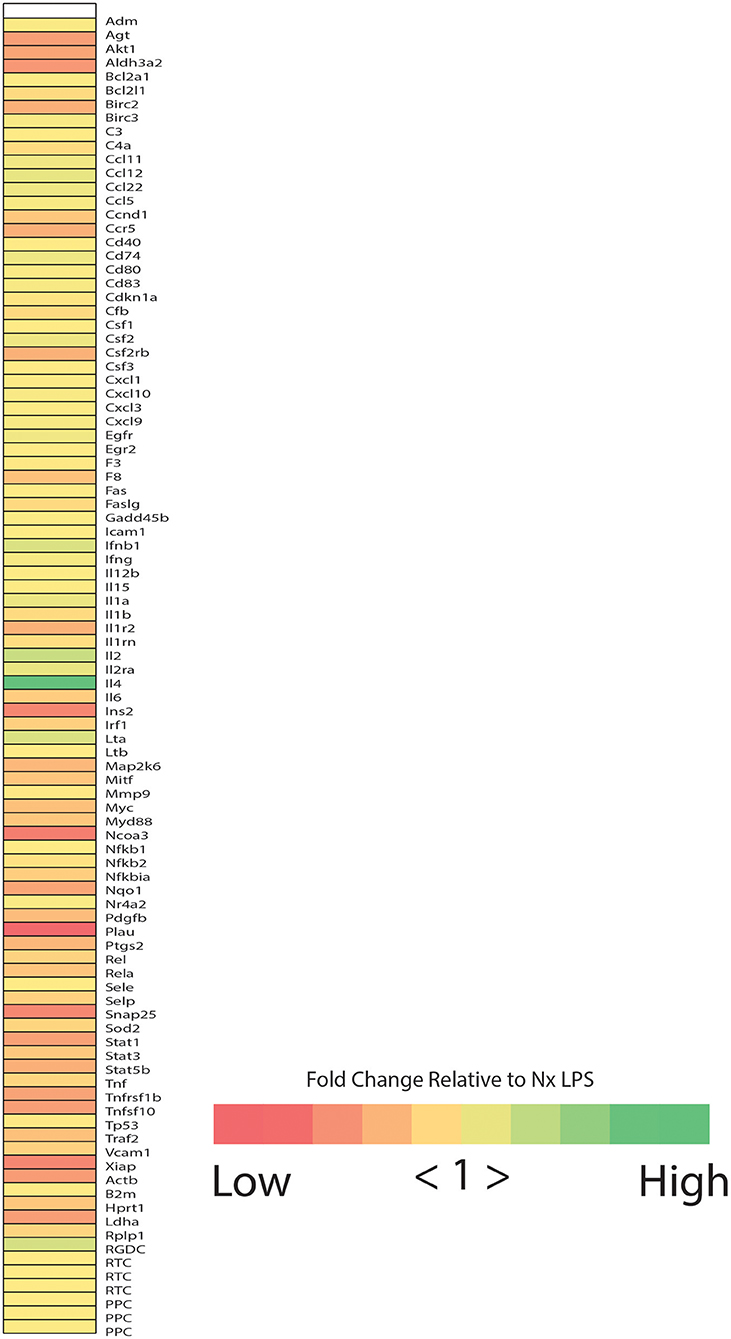
Figure 2. Neonatal IH increases the expression of NFκB-related cytokines. Heatmap represents the results of the Qiagen NF-κB signaling targets gene array. Colors represent the fold change between IH LPS and Nx LPS samples. “High” represents the highest fold change and “Low” represents the lowest fold change.
We next tested the effects of neonatal IH on inflammatory gene expression in male (Figure 3A) and female (Figure 3B) microglia, as well as peripheral spleen macrophages (Supplementary Figure 2) following either vehicle or LPS treatment (Figure 3). In basal conditions (Figures 3A,B), there were no main effects of IH on male nor female neonatal microglial expression of the pro-inflammatory molecules Inos, Cox-2, and Il1β, nor the pro-inflammatory type I interferon targets Il1a and Cxcl10 (Tables 2, 3; each table contains F-values, p-values, and n for all genes). Therefore, IH was not a strong stimulus for classic pro-inflammatory molecules. However, as expected, there was a main effect of LPS (Figures 3C,D) on each of these cytokines/enzymes (p-values for each gene in Tables 2, 3), indicating that neonatal microglia from these pups retain the capacity to respond to an inflammatory challenge. Surprisingly, IH appeared to have sexually dimorphic effects on the anti-inflammatory molecules Ifnβ1 and Il4. For males, there was a main effect of IH on Ifnβ1 (Table 2); post-hoc analyses showed that IH increased Ifnβ1 in both vehicle (p = 0.017) and LPS conditions (p = 0.012). There was a statistical trend for a main effect of LPS on Ifnβ1 expression in males (p = 0.071), but there was no interaction with IH (p = 0.961). In females however, while there was no main effect IH on Ifnβ1 expression (Table 3), there was a statistically significant effect of LPS (p = 0.007) and a significant interaction with IH (p = 0.016); post-hoc analyses showed a significant increase in Ifnβ1 only in vehicle conditions (p = 0.010), and not after LPS treatment (p = 0.407). Il4 followed a similar trend to Ifnβ1; in males, IH increased Il4 (Table 2) in vehicle-treated animals (p = 0.012) and trended to increase it after LPS treatment (p = 0.054), but there was no main effect of LPS (p = 0.524), nor any interaction with IH (p = 0.643). In contrast, there was no main effect of IH on Il4 in females (Table 3), although there was a main effect of LPS (p = 0.001) and a trending interaction with IH (p = 0.061). Post-hoc analyses for female Il4 showed a statistical trend for IH to increase Il4 in microglia from vehicle-treated pups (p = 0.067) but not from LPS-treated pups (p = 0.410).
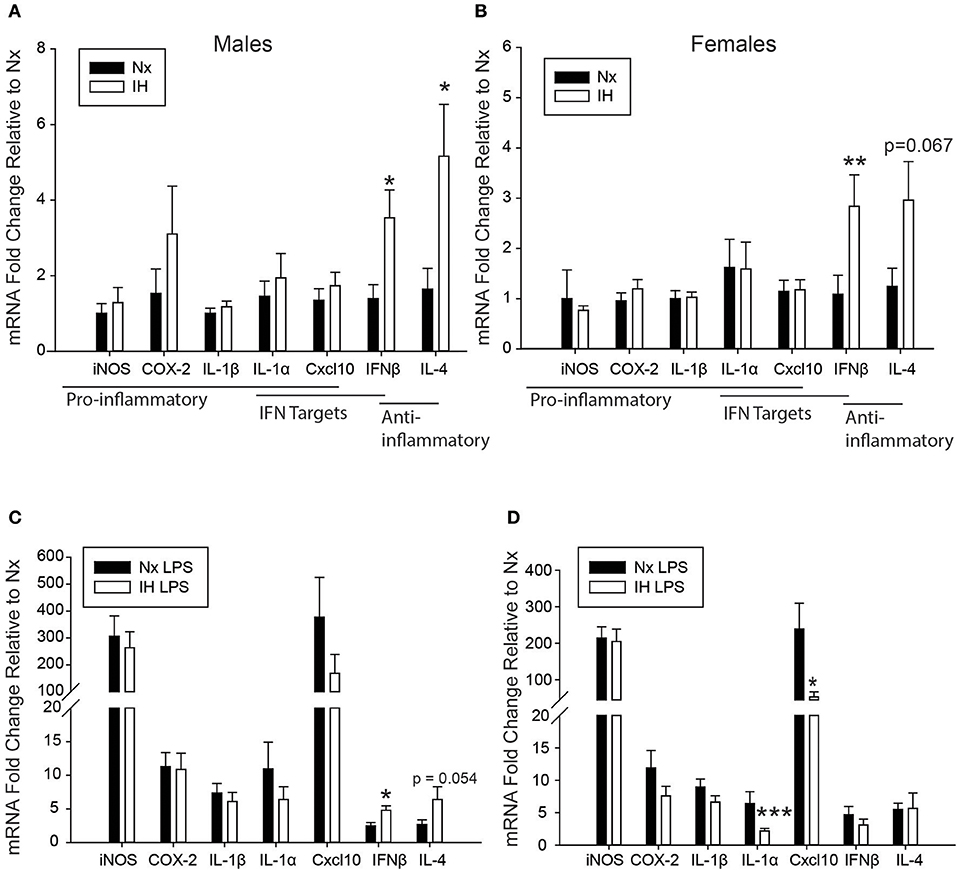
Figure 3. Neonatal IH augments anti-inflammatory cytokine and sex-specifically attenuates LPS-induced inflammatory cytokine gene expression. (A,B) Basal gene expression was analyzed in neonatal male (A) and female (B) microglia after 8 days of IH. (C,D) Microglial gene expression was analyzed in male (C) and female (D) pups exposed to IH followed by LPS challenge. Values represent average fold change +/– SEM *p < 0.05, **p < 0.01, ***p < 0.001 relative to respective Nx control, Two-way ANOVA.
While there were no statistically significant interactions between IH and LPS for most inflammatory cytokines, post-hoc analyses of the main effects of LPS revealed that IH significantly attenuated the LPS-induced interferon targets Il1a (p = 0.009) and Cxcl10 (p = 0.049) in females but not males (Figures 3C,D), corroborating the findings for Ifnb1 and Il4. Interestingly, all these effects were specific to CNS macrophages, as these observations were not present in peripheral (spleen) macrophages (Supplementary Figure 2). There was no main effect of IH nor any interaction between LPS and IH in spleen macrophages from males (Supplementary Figure 2A) or females (Supplementary Figure 2B) at this developmental stage. Therefore, even though both male and female microglia had similar basal levels of IH-induced cytokine gene expression, IH attenuated female, but not male, microglial responses to a subsequent inflammatory LPS challenge, effects that were specific to the CNS.
Since 8 days of IH resulted in altered microglial cytokine expression, we further assessed if these changes occurred earlier in the IH time course. For these analyses, we exposed a separate cohort of pups to IH at P1 for 1 day only, and then challenged them (i.p.) with vehicle or LPS at P2. Microglia were assessed for cytokine expression (Supplementary Figures 2C,D). Although there was a main effect of IH on male Ifnβ1 expression (p = 0.027), post-hoc analyses only revealed a trend for increased Ifnβ1 expression in IH LPS conditions (p = 0.059). No other significant changes were identified for any cytokine in males or females. However, these results suggest that males may be more sensitive to IH effects at an earlier time point than females, and that Ifnβ1 may be one of the cytokines driving the effects of IH.
Neonatal IH Sex-Specifically Changes Leukocyte Recruitment in the CNS
Previous studies demonstrated brain region-specific sex-differences in microglia number during early postnatal development (39). To determine if changes in microglia frequency and/or leukocyte recruitment could explain the sex-specific effects of IH on gene expression (Figure 4), we performed flow cytometry for CD11b, CD45, and CD4. Surprisingly, we found no main effect of IH (F = 0.290, p = 0.630) nor sex (F = 0.974, p = 0.335) on the frequency of CD11b+/CD45low microglia in the whole brain. However, we found a leukocyte population (~0.8–1% of cells) that was CD11b+/CD45high in both males (Figures 4, 5A) and females (Figures 4, 5B), which was augmented by IH only in females (p = 0.005) and not in males (p = 0.550). Because the antibody we used to label CD11b shares a common antigen with CD11c, it is possible that this population is comprised of monocyte-derived dendritic cells given the intermediate CD11b/c expression and their high CD45 levels. However, further analyses are needed to conclusively identify this leukocyte population.
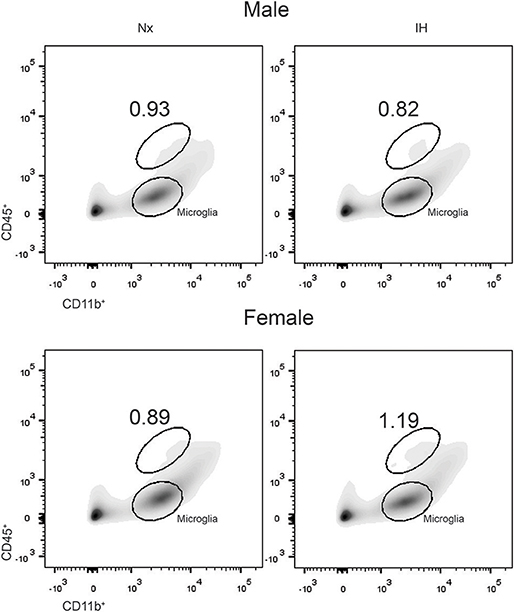
Figure 4. IH increases a novel population of CD11b+/CD45high cells in females. Whole brains from males (Top) and females (Bottom) were dissociated into single cell suspensions and assessed by flow cytometry for CD11b and CD45 expression. Each panel shows a representative flow density plot for neonates exposed to 8 days of Nx or IH. The numbers represent the % of live cells gated.
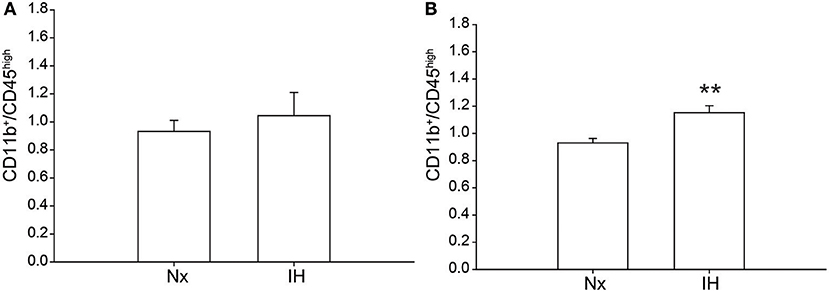
Figure 5. Quantification of the novel CD11b+/CD45high population. Whole brains from IH or Nx-exposed neonatal males (A); n = 6/treatment) and females (B); n = 6/treatment) were assessed by flow cytometry for % of live cells expressing CD11b and CD45. Values represent the average % of live cells +/– SEM, **p < 0.001, t-test.
Given our gene expression analyses suggesting that microglia are increasing their expression of cytokines are associated with T-helper cell immunity (59, 60), we next tested whether the increases in Ifnβ1 and Il4 expression corresponded to recruitment of CD4+ T-helper cells (Figure 6A). While we were able to detect a low frequency (~1%) population of CD4+/Cd45high/CD11b− cells indicative of T cells, they did not differ by sex or treatment. Interestingly however, ~3–5% of CNS cells were CD4+, the majority of which were CD11bhigh (Figure 6B). While very few of these cells were microglia (CD11b+/CD45low), it is established that CD4 labels monocytes in the rat (61), suggesting that CD4 may be a marker of bone-marrow derived macrophages/monocytes. The total number of CD4+ cells did not change by sex or treatment. Together, these findings demonstrate that CD4+ T-cell recruitment to the CNS is not altered by IH.
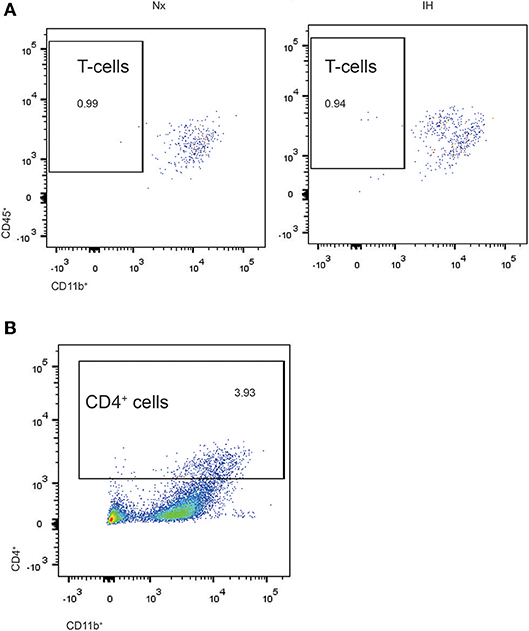
Figure 6. Flow cytometry analysis of CD4+ cells in whole brains isolated from neonatal rats. (A) CD4+ cells were gated and analyzed for concomitant CD45 and CD11b expression in Nx and IH conditions. T cells exhibit high CD45 with low CD11b expression. (B) Approximately 3–4% of total live cells were CD4+, the majority of which were high in both CD11b and CD45 expression; microglia are CD11b+/CD45low.
Microglia RNA-Seq Reveals Sex-Differences in Basal and Inflammatory Conditions
The sex differences we identified in IH-induced gene expression and leukocyte recruitment may be indicative of gene pathways that are sexually dimorphic in either basal or inflammatory conditions, independent of IH exposure. Therefore, we used RNA-seq to assess sex differences in the neonatal microglial transcriptome after 8 days of IH exposure following vehicle or LPS treatment (Nx, IH, Nx LPS, IH LPS) (Figure 7). EdgeR bioinformatics analyses revealed that in basal (no LPS) conditions, 39 genes differed between males and females, independent of IH effects (Figure 7A, left). Males had more genes upregulated (than downregulated) relative to females. MDS analysis revealed that samples had high variability overall (Figure 7A, right), but clustered together based on sex, rather than IH treatment, suggesting that sex accounted for more of the variability between samples than IH treatment. Indeed, when analyzing vehicle-treated samples for an effect of IH, only a few genes were modified by IH in both males and females (Table 4).
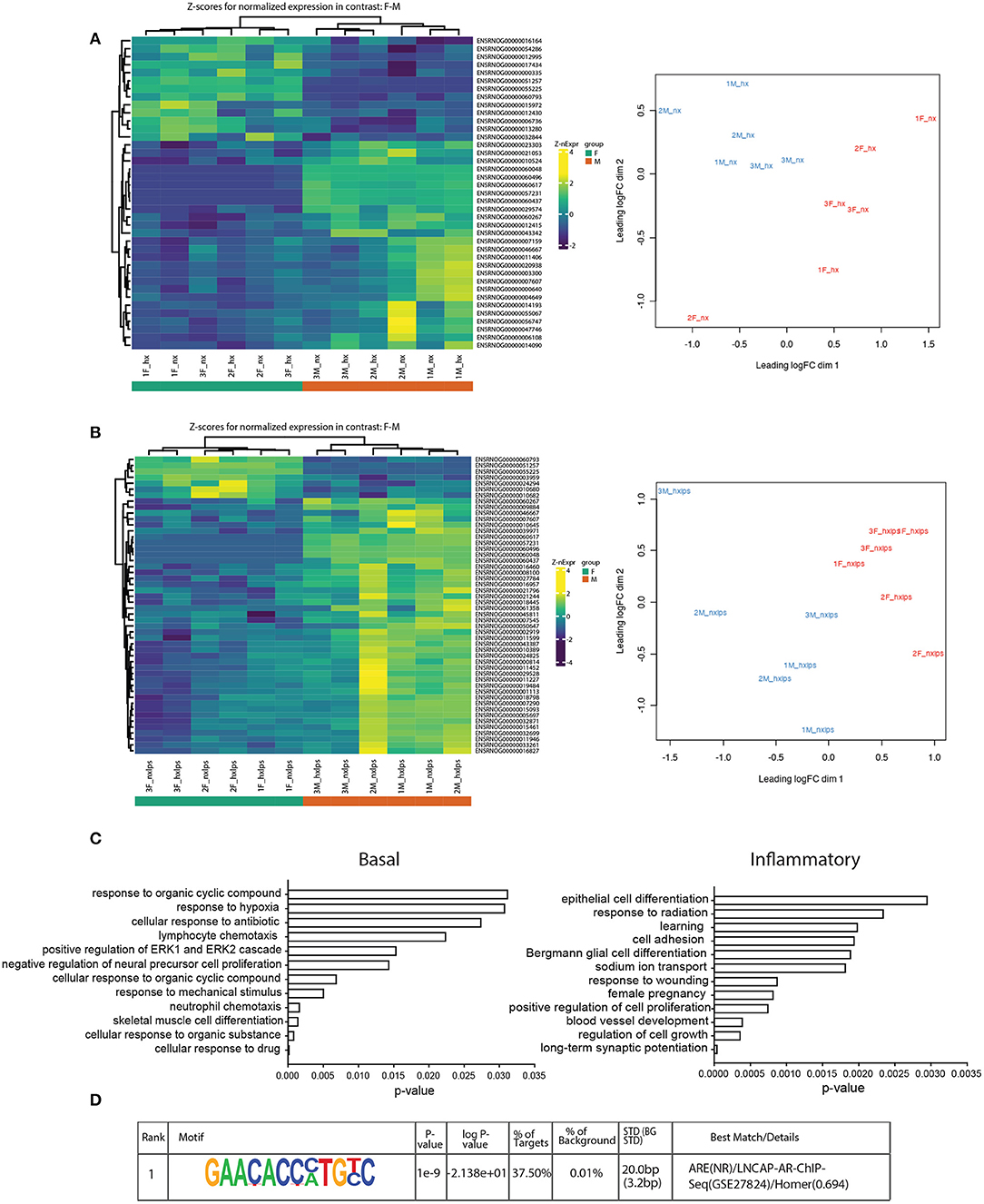
Figure 7. RNA-seq analyses of neonatal male and female microglia. (A) Heatmap of z-scores (left) and MDS analysis (right) for normalized gene expression in male and female microglia in basal conditions. (B) Heatmap (left) and MDS analysis (right) of normalized gene expression in male and female microglia in inflammatory (LPS) conditions. (C) Gene ontology for genes upregulated in males relative to females in basal (left) and inflammatory (right) conditions. (D) The top-ranking match for Homer motif analysis of the 8 genes identified in the GO category “cognition”.
In LPS-treated samples (Figure 7B), 98 genes were differentially regulated between male and female pups. Similar to basal conditions, MDS analysis revealed that samples clustered based on sex and not IH treatment (Figure 7B, right). Further, when compared to females, more genes were upregulated in males than downregulated. Interestingly, although the effects of IH were not strong enough to reveal many significant differences with n = 3 biological replicates (and 10 million reads), the bioinformatics analyses did confirm that multiple LPS-induced inflammatory genes, including Il1a, were attenuated by IH in females, but not in males (Table 4 and Figure 3D). When we probed RNA-seq samples for the effect of IH on LPS-stimulated NF-κB signaling targets previously identified using the gene array, we found that multiple LPS-induced type I IFN genes were attenuated (Table 4) by IH pre-exposure in females only, confirming our previous findings.
Genes upregulated in males relative to females in both basal (Figure 7C, left) and inflammatory conditions (Figure 7C, right) were functionally annotated using DAVID for Gene Ontology (GO) class Biological Process. In basal conditions, males and females differed in genes related to the categories of “hypoxia,” “lymphocyte chemotaxis,” and “neutrophil chemotaxis,” observations that may contribute to the previous findings that certain leukocyte populations are sex-specifically recruited to the brain during IH. Additionally, several of the genes involved in chemotaxis, including the chemokine receptor Ccr3, were differentially expressed between males and females, as has been reported previously in the P4 rat brain (39).
To further examine relationships between all the genes that were significantly changed by sex irrespective of IH, we used STRING analysis of functional protein association networks (Supplementary Figure 3). The results showed two main gene networks: one involving cytokines/chemokines, and the other involving cellular proliferation/survival (Supplementary Figure 3A). These findings support the notion that IH-induced differences in cytokine expression (Figure 3) may be related to basal and IH-induced differences in leukocyte trafficking (supported by Figures 4, 5), and/or the ability of certain cell populations to proliferate.
Surprisingly, in inflammatory conditions (irrespective of IH), sex-specific changes were identified in gene categories related to “learning” and “long-term synaptic potentiation” (Figure 7C). Additional analyses examining Biological Process in Panther similarly revealed 8 genes enriched in the category “cognition”: Ptn, BDNF/NT-3, Dbi, Atp1a, Stra6, Fam107a, Agt, and Slc6a1 (Supplementary Figure 3B). Motif analyses of the promoters for these cognition-related genes revealed a common binding motif for the androgen receptor (Figure 7D), suggesting that the androgen receptor may play an important role in sex-related differences in neonatal susceptibility to cognitive deficits. Together, these results support the idea that sex-differences exist in P9 whole brain microglia in both basal and inflammatory conditions and suggest multiple candidate gene targets by which early life insults may change microglial function and impact cognition.
Weight Gain in Young Adults Is Not Altered by Neonatal IH
Because weight changes observed acutely following neonatal IH exposure were previously reported to persist into adulthood (14), we also tested this in 6 week-old young adults that were neonatally exposed to 8 days of IH starting at P1 (Figures 8A,B). The average weight of adult males (Figure 8A) exposed to neonatal Nx (n = 21) was 235.14 g +/– 4.25 g and neonatal IH (n = 19) was 231.79 g +/– 5.16 g. Similarly, the average weight of females (Figure 8B) exposed to neonatal Nx (n = 14) was 176.14 g +/– 3.63 g and neonatal IH (n = 18) was 174.42 g +/– 3.97 g. There was no statistical difference in weight for males (t = 0.505, p = 0.616) nor females (t = 0.312, p = 0.757), suggesting that the paradigm of IH used in these studies does not exert effects on weight that persist into adulthood.
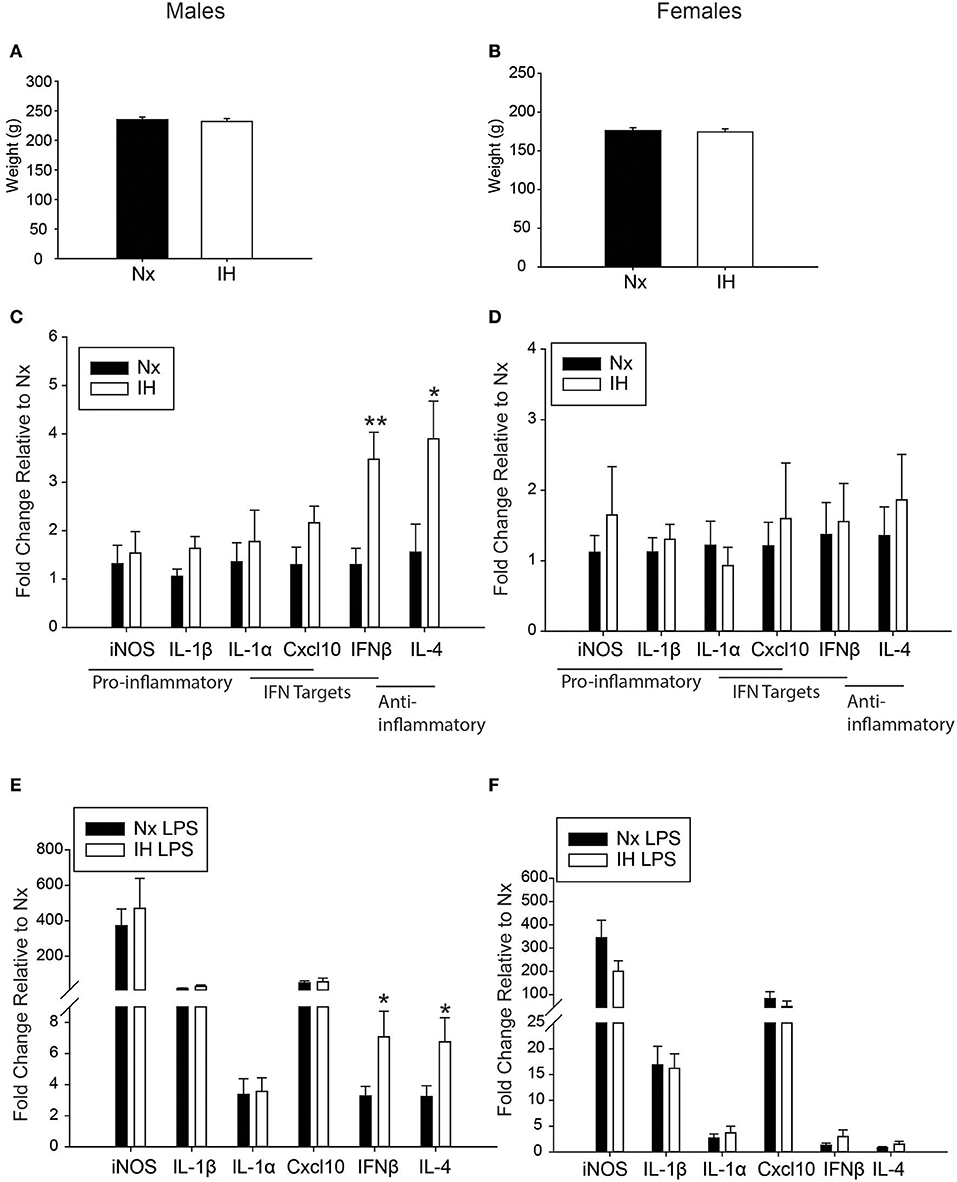
Figure 8. Neonatal IH augments anti-inflammatory cytokine gene expression in adult males. (A,B) Adult weights were unchanged in males (A) and females (B) 6 weeks following the last IH exposure. (C,D) Basal gene expression was analyzed in adult male (C) and female (D) microglia 6 weeks post neonatal IH exposure. (E,F) Microglial gene expression was analyzed in male (E) and female (F) neonates exposed to IH followed by LPS challenge. Values represent average fold change +/– SEM *p < 0.05, **p < 0.01, relative to respective Nx control, Two-way ANOVA.
Neonatal IH Sex-Specifically Disrupts Microglial Ifnβ1 and Il4 Expression Long-Term
We next assessed whether neonatal IH-induced changes in microglial cytokine gene expression were maintained long-term, into early adulthood. Six week-old males and females exposed to Nx or IH as neonates were injected with LPS (i.p. for 3 h), and microglia were isolated for gene expression analyses (Figures 8C–F). The majority of cytokines in adult males (Figure 8C and Table 5) and females (Figure 8D and Table 6) were unchanged in basal conditions, and 3 h of LPS was sufficient to increase the expression of most inflammatory cytokines. Interestingly, in adult males (but not females) there was a significant main effect of neonatal IH on Ifnβ1 expression (p < 0.001) and Il4 (p = 0.003). Post-hoc analyses showed that IH augmented both Ifnβ1 and Il4 expression in vehicle- (Ifnβ1 p = 0.004; Il4 p = 0.020) and LPS-treated (Ifnβ1 p = 0.020; Il4 p = 0.047) conditions. Thus, the effects of neonatal IH on male Ifnβ1 and Il4 microglial gene expression persisted into adulthood, whereas those effects on female microglia did not. Surprisingly, while LPS significantly increased both Ifnβ1 and Il4 in adult males, there was no effect of LPS on these cytokines in adult females (Table 6), despite the interaction between LPS and IH in neonatal females (Table 3). Together, these results support the idea that neonatal IH has sex-specific effects that persist into early adulthood in males, and that some responses to LPS differ developmentally.
Neonatal IH Does Not Impair Adult Working Memory
Since adult males exposed to neonatal IH demonstrated changes in the expression of microglial Ifnβ1 and Il4, cytokines with mixed effects on learning and memory (62–65), we next examined working memory in young adult (6–8 wk) male and female rats (Figure 9). Spontaneous alternation in behaviorally naïve rats was tested in a Y-maze, a frontal cortex and hippocampal-dependent working memory task (57, 58, 66). While there was a significant interaction between sex and IH treatment (F = 4.568, p = 0.038, Two-way ANOVA), post-hoc analyses identified no significant differences between the treatment groups. There was a statistical trend for better performance in females compared to males exposed to neonatal Nx (t = 1.879, p = 0.067), and a trending decrease in spontaneous alternation performance in neonatal IH-exposed females compared to neonatal Nx-exposed females (t = 1.613, p = 0.114); however, the effects were modest at best. Together, these results suggest that 8 days of neonatal IH does not strongly impair spatial memory in otherwise naïve animals, although there are sexual dimorphisms in the effects of IH on spatial working memory.
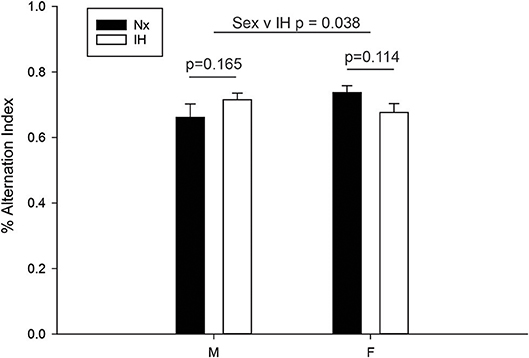
Figure 9. IH sex-specifically affects spontaneous alternation in naïve young adults. Behaviorally naïve adults who were exposed to Nx or IH as neonates were tested in the Y-maze spontaneous alternation task. Nx males (n = 9), IH males (n = 14), Nx females (n = 11) females and IH females (n = 12). The spontaneous alternation index is defined as the number of times a rat made three consecutively correct arm entries/total arm entries minus two. Results represent the average % alternation index in rats from three independent litters +/– SEM, Two-way ANOVA.
Discussion
In this work, we initially hypothesized that neonatal IH would sex-specifically augment microglial inflammatory gene expression in both basal and inflammatory conditions, and that these effects would persist into adulthood. Contrary to our hypothesis, we found that neonatal IH augmented anti-inflammatory and type I interferon-related cytokines, and sex-specifically altered CNS myeloid populations, while attenuating LPS-induced inflammatory cytokine/chemokine expression acutely following IH. While these changes persisted into adulthood only in males, they were not associated with overall impairments in working memory nor alterations in spleen macrophage gene expression.
Our work also demonstrated sex differences in the effects of IH both acutely and long term. Acutely, neonatal IH increased anti-inflammatory cytokines in both P9 males and females, but only impaired LPS responses in P9 females. One intriguing explanation for the acute effects of IH on LPS responses in female vs. male microglia is a sex difference in the capacity to respond apoptotic CNS cells via phagocytosis (efferocytosis). Our results showed that IH increased CNS cell death by ~13–16% in both sexes. While we were not able to determine by flow cytometry if cell death was apoptotic (vs. necrotic), the lack of basal pro-inflammatory gene expression at both P1 and P9 following neonatal IH, and previous work in neonatal IH models (15), would support a non-inflammatory mechanism of cell death (i.e., apoptosis). It is well-established that macrophage efferocytosis is an anti-inflammatory process which can attenuate LPS responses (67–69). Since males and females exhibit differences in microglial phagocytosis in early postnatal life (43), female microglia may be more responsive to CNS apoptosis/cell death. Our RNA-seq data support sex differences in genes associated with apoptosis and efferocytosis; for example, the nuclear receptor Nr4a1, which contributes to the anti-inflammatory effects of efferocytosis in peripheral macrophages (70), is differentially expressed in our male and female microglia under basal conditions (Supplementary Figure 3). The idea that CNS cell death may account for the sex differences in LPS response would explain why changes in female LPS responses are acute, as IH-induced cell death would no longer be present in young adulthood and therefore would not induce an anti-inflammatory effect in microglia.
Another possible explanation for the acute effects of IH on LPS responses is the recruitment of other innate immune cells, which we predict is transient, but have not yet confirmed. By flow cytometry, we observed that IH sex-specifically increased a population of CD11b+/CD45high cells that are likely to be other monocyte-derived cell types. Recent research in mice has identified a neonatal subpopulation of CD11c+ microglia that express CD4 and contribute to myelogenesis; it is possible that the myeloid population identified here has similar function (71). Alternatively, this myeloid cell population could be dendritic cells, which are important antigen presenting cells present in the choroid plexus under homeostatic conditions (72), but can also be derived from monocytes in the presence of IL-4 and IFNβ (73), two of the genes we find to be augmented by IH in the brain. Additionally, dendritic cells are strong producers of IFNβ (74). Thus, it remains unclear whether the changes we observe in Ifnβ1 and Il4 gene expression are truly a reflection of alterations in microglial gene expression, or if they reflect responses to the unidentified alternative monocyte cell population. However, if the changes in Ifnβ1 and Il4 are a consequence of changing CNS myeloid populations, it would suggest that males and females have different sources of these two genes, as only females exhibited a change in myeloid populations. Future work will need to investigate this possibility by characterizing the different myeloid populations identified for the first time here. Our RNA-seq data further supported the potential for a sex difference in the myeloid cell recruitment to the CNS, as a network of chemokine-related genes was differentially expressed (Supplementary Figure 3).
In addition to the sex differences in microglial gene expression following acute IH, there were multiple sex effects identified by RNA-seq that were independent of IH treatment. From the RNA-seq data, we observed changes in “cognition”-related genes in the context of inflammation, not in basal conditions. This is important given that OSA is often comorbid with other illnesses involving systemic inflammation. These results suggest that cognitive vulnerabilities may be unmasked because of infection. Motif analyses for DNA binding proteins that may be common regulators of these cognition genes identified a binding motif for the androgen receptor. Androgen receptors mediate inflammatory responses (75, 76) and CNS pathology (77), and androgens activate signaling pathways that influence the cognition-related genes identified in this study, including the GABA transporter Slc6a (78–81). Indeed, at least one study has even demonstrated that androgen receptors contribute to male-specific GABAA-mediated excitotoxicity in the hippocampus (79), which might underlie male vulnerability to cognitive dysfunction in early life. Thus, the microglial genes identified in this study may be useful candidates for future studies that investigate sexual dimorphism in cognitive dysfunction after neonatal stressors, such as IH.
Unlike females, males maintained their basal gene expression of anti-inflammatory cytokines acutely and long-term. Since males did not exhibit an acute increase in CNS myeloid populations, there is an as yet unidentified mechanism contributing to these long-term changes in Ifnβ1 and Il4 expression in males. We posit that IH-induces modifications to the chromatin landscape, as has been reported for the carotid body (82). While the consequences of upregulated microglial cytokines in the male are unknown, type I interferons can inhibit synaptic plasticity associated with learning (83), and increased levels of IFNβ and IL-4 in the choroid plexus are associated with cognitive dysfunction in aging (62, 63). Although our results suggest that basal working memory is not impaired in young adults (6–8 wks) exposed to IH as neonates, it is unknown for how long the augmentation of these anti-inflammatory cytokines persists, or if they contribute to cognitive dysfunction in aging.
Our IH model recapitulates multiple aspects of other models of infant OSA, including SpO2 nadir to ~60% and poor weight gain (5). However, the severity of O2 desaturation caused by IH exposures in rats compared to infant OSA is questionable due to species differences in the oxygen reserve, suggesting that the 60%SpO2 nadir in neonatal rats is likely less severe than 60% in human newborns (84). Nonetheless, for experimentally modeling the effects of OSA, rats remain a good mammalian option (as compared to mice) since their oxygen dissociation curve is closer to that of humans (85). Other limitations include exposure of pups with dams during IH treatment, which others have suggested might reduce lactation, resulting in reduced neonatal weight gain (16); this has not been directly tested. Nevertheless, repeating these findings using a cross-fostering model which does not alter weight gain (16) may be beneficial to better understand the direct effects of IH on the pups independent of the mother. Lastly, the neonates were exposed to 15, uniformly distributed, hypoxic episodes/h, for 8 h periods during the light phase of each day. Since rat neonates do not establish diurnal/nocturnal patterns for wake/sleep until P16-P20 (86), this IH model does not necessarily recapitulate apneas experienced only during sleep. However, despite these constraints, the weight and SpO2 results we obtained are similar to those in other neonatal IH models used by others (14, 20, 38, 87).
Overall, the results presented here support the idea that IH is an early life stimulus that acutely modifies microglial function and induces long-lasting changes in microglial gene expression. Future studies will need to establish the functional consequences of IH-induced microglial gene changes in the context of long-term CNS inflammation and cognitive function.
Data Availability
The sequencing datasets generated in this study are available in the NCBI GEO Datasets: GSE126946. All other data are presented in manuscript or additional files, and are available from the corresponding author upon reasonable request.
Ethics Statement
This study was carried out in accordance with the recommendations of University of Wisconsin-Madison Institutional Animal Care and Use Committee. The protocol was approved by the University of Wisconsin-Madison Institutional Animal Care and Use Committee.
Author Contributions
EK and JW prepared the manuscript and figures. EK collected and analyzed data for flow cytometry, PCR, and RNA-seq experiments. TW analyzed adult spleen samples. SC, AV, and MC performed behavioral experiments and analyses.
Funding
This study was supported by research funding from the National Institutes of Health F31NS100229 and T32 GM0075007 (EK), R0I HL111598 and NS085226 (JW), and a Vilas Award from the University of Wisconsin-Madison (JW).
Conflict of Interest Statement
The authors declare that the research was conducted in the absence of any commercial or financial relationships that could be construed as a potential conflict of interest.
Acknowledgments
The authors thank the University of Wisconsin Biotechnology Center DNA Sequencing Facility for providing DNA sequencing facilities and services, the UW Comprehensive Cancer Center Flow Cytometry core (NIH P30 CA014520 and 1S100OD018202-01) and the Bioinformatics Resource Center for help and feedback on data analyses. We thank Jonathan Ouellette, Andrea Ewald, and Dr. Bailey Kermath for assistance with lab procedures. We also wish to thank Drs. John Svaren and Reid Alisch for helpful discussions and suggestions throughout this project, and for providing guidance and expertise with bioinformatics analyses.
Supplementary Material
The Supplementary Material for this article can be found online at: https://www.frontiersin.org/articles/10.3389/fimmu.2019.01479/full#supplementary-material
References
1. Lumeng JC, Chervin RD. Epidemiology of pediatric obstructive sleep apnea. Proc Am Thorac Soc. (2008) 5:242–52. doi: 10.1513/pats.200708-135MG
2. Cielo CM, Montalva FM, Taylor JA. Craniofacial disorders associated with airway obstruction in the neonate. Semin Fetal Neonatal Med. (2016) 21:254–62. doi: 10.1016/j.siny.2016.03.001
3. Greenfeld M, Tauman R, DeRowe A, Sivan Y. Obstructive sleep apnea syndrome due to adenotonsillar hypertrophy in infants. Int J Pediatr Otorhinolaryngol. (2003) 67:1055–60. doi: 10.1016/S0165-5876(03)00182-4
4. Leonardis RL, Robison JG, Otteson TD. Evaluating the management of obstructive sleep apnea in neonates and infants. JAMA Otolaryngol Neck Surg. (2013) 139:139–46. doi: 10.1001/jamaoto.2013.1331
5. Sharma PB, Baroody F, Gozal D, Lester LA. Obstructive sleep apnea in the formerly preterm infant: an overlooked diagnosis. Front Neurol. (2011) 2:73. doi: 10.3389/fneur.2011.00073
6. Trosman I, Trosman SJ. Cognitive and behavioral consequences of sleep disordered breathing in children. Med Sci. (2017) 5:E30. doi: 10.3390/medsci5040030
7. Smitthimedhin A, Whitehead MT, Bigdeli M, Nino G, Perez G, Otero HJ. MRI determination of volumes for the upper airway and pharyngeal lymphoid tissue in preterm and term infants. Clin Imaging. (2018) 50:51–6. doi: 10.1016/j.clinimag.2017.12.010
8. Hornik CP, Fort P, Clark RH, Watt K, Benjamin DK, Smith PB, et al. Early and late onset sepsis in very-low-birth-weight infants from a large group of neonatal intensive care units. Early Hum Dev. (2012) 88(Suppl. 2):S69–74. doi: 10.1016/S0378-3782(12)70019-1
9. Shah A, Diculescu VC, Qureshi R, Oliveira-Brett AM. Electrochemical behaviour of dimethyl-2-oxoglutarate on glassy carbon electrode. Bioelectrochemistry. (2010) 77:145–50. doi: 10.1016/j.bioelechem.2009.07.012
10. Kheirandish L, Gozal D, Pequignot J-M, Pequignot J, Row BW. Intermittent hypoxia during development induces long-term alterations in spatial working memory, monoamines, and dendritic branching in rat frontal cortex. Pediatr Res. (2005) 58:594–9. doi: 10.1203/01.pdr.0000176915.19287.e2
11. Smith SMC, Friedle SA, Watters JJ. Chronic intermittent hypoxia exerts CNS region-specific effects on rat microglial inflammatory and TLR4 gene expression. PLoS ONE. (2013) 8:e81584. doi: 10.1371/journal.pone.0081584
12. Snyder B, Shell B, Cunningham JT, Cunningham RL. Chronic intermittent hypoxia induces oxidative stress and inflammation in brain regions associated with early-stage neurodegeneration. Physiol Rep. (2017) 5:e13258. doi: 10.14814/phy2.13258
13. Li RC, Row BW, Gozal E, Kheirandish L, Fan Q, Brittian KR, et al. Cyclooxygenase 2 and intermittent hypoxia-induced spatial deficits in the rat. Am J Respir Crit Care Med. (2003) 168:469–75. doi: 10.1164/rccm.200211-1264OC
14. Pozo ME, Cave A, Köroglu OA, Litvin DG, Martin RJ, Di Fiore J, et al. Effect of postnatal intermittent hypoxia on growth and cardiovascular regulation of rat pups. Neonatology. (2012) 102:107–13. doi: 10.1159/000338096
15. Gozal E, Row BW, Schurr A, Gozal D. Developmental differences in cortical and hippocampal vulnerability to intermittent hypoxia in the rat. Neurosci Lett. (2001) 305:197–201. doi: 10.1016/S0304-3940(01)01853-5
16. Cai J, Tuong CM, Gozal D. A neonatal mouse model of intermittent hypoxia associated with features of apnea in premature infants. Respir Physiol Neurobiol. (2011) 178:210–7. doi: 10.1016/j.resp.2011.06.003
17. Soukhova-O'Hare GK, Cheng ZJ, Roberts AM, Gozal D. Postnatal intermittent hypoxia alters baroreflex function in adult rats. Am J Physiol Circ Physiol. (2006) 290:H1157–64. doi: 10.1152/ajpheart.00767.2005
18. Soukhova-O'Hare GK, Roberts AM, Gozal D. Impaired control of renal sympathetic nerve activity following neonatal intermittent hypoxia in rats. Neurosci Lett. (2006) 399:181–5. doi: 10.1016/j.neulet.2006.01.054
19. Soukhova-O'Hare GK, Ortines RV, Gu Y, Nozdrachev AD, Prabhu SD, Gozal D. Postnatal intermittent hypoxia and developmental programming of hypertension in spontaneously hypertensive rats: the role of reactive oxygen species and L-Ca2+ channels. Hypertension. (2008) 52:156–62. doi: 10.1161/HYPERTENSIONAHA.108.110296
20. Julien C, Bairam A, Joseph V. Chronic intermittent hypoxia reduces ventilatory long-term facilitation and enhances apnea frequency in newborn rats. Am J Physiol Regul Integr Comp Physiol. (2008) 294:R1356–66. doi: 10.1152/ajpregu.00884.2007
21. Ingemarsson I. Gender aspects of preterm birth. BJOG. (2003) 110(Suppl. 20):34–8. doi: 10.1046/j.1471-0528.2003.00022.x
22. Cunningham CL, Martínez-Cerdeño V, Noctor SC. Microglia regulate the number of neural precursor cells in the developing cerebral cortex. J Neurosci. (2013) 33:4216–33. doi: 10.1523/JNEUROSCI.3441-12.2013
23. Miyamoto A, Wake H, Ishikawa AW, Eto K, Shibata K, Murakoshi H, et al. Microglia contact induces synapse formation in developing somatosensory cortex. Nat Commun. (2016) 7:12540. doi: 10.1038/ncomms12540
24. Mosser C-A, Baptista S, Arnoux I, Audinat E. Microglia in CNS development: shaping the brain for the future. Prog Neurobiol. (2017) 149–150:1–20. doi: 10.1016/j.pneurobio.2017.01.002
25. Paolicelli RC, Bolasco G, Pagani F, Maggi L, Scianni M, Panzanelli P, et al. Synaptic pruning by microglia is necessary for normal brain development. Science. (2011) 333:1456–8. doi: 10.1126/science.1202529
26. Tremblay M-È, Lowery RL, Majewska AK. Microglial interactions with synapses are modulated by visual experience. PLoS Biol. (2010) 8:e1000527. doi: 10.1371/journal.pbio.1000527
27. Ueno M, Fujita Y, Tanaka T, Nakamura Y, Kikuta J, Ishii M, et al. Layer V cortical neurons require microglial support for survival during postnatal development. Nat Neurosci. (2013) 16:543–51. doi: 10.1038/nn.3358
28. Wakselman S, Béchade C, Roumier A, Bernard D, Triller A, Bessis A. Development/plasticity/repair developmental neuronal death in hippocampus requires the microglial CD11b integrin and DAP12 immunoreceptor. J Neurosci. (2008) 28:8138–43. doi: 10.1523/JNEUROSCI.1006-08.2008
29. Parkhurst CN, Yang G, Ninan I, Savas JN, Yates JR, Lafaille JJ, et al. Microglia promote learning-dependent synapse formation through brain-derived neurotrophic factor. Cell. (2013) 155:1596–609. doi: 10.1016/j.cell.2013.11.030
30. Derecki NC, Cronk JC, Lu Z, Xu E, Abbott SBG, Guyenet PG, et al. Wild-type microglia arrest pathology in a mouse model of Rett syndrome. Nature. (2012) 484:105–9. doi: 10.1038/nature10907
31. Schuitemaker A, van der Doef TF, Boellaard R, van der Flier WM, Yaqub M, Windhorst AD, et al. Microglial activation in healthy aging. Neurobiol Aging. (2012) 33:1067–72. doi: 10.1016/j.neurobiolaging.2010.09.016
32. Zhan Y, Paolicelli RC, Sforazzini F, Weinhard L, Bolasco G, Pagani F, et al. Deficient neuron-microglia signaling results in impaired functional brain connectivity and social behavior. Nat Neurosci. (2014) 17:400–6. doi: 10.1038/nn.3641
33. Caravale B, Tozzi C, Albino G, Vicari S. Cognitive development in low risk preterm infants at 3-4 years of life. Arch Dis Child Fetal Neonatal Ed. (2005) 90:F474–9. doi: 10.1136/adc.2004.070284
34. Verney C, Monier A, Fallet-Bianco C, Gressens P. Early microglial colonization of the human forebrain and possible involvement in periventricular white-matter injury of preterm infants. J Anat. (2010) 217:436–48. doi: 10.1111/j.1469-7580.2010.01245.x
35. Zhan G, Fenik P, Pratico D, Veasey SC. Inducible nitric oxide synthase in long-term intermittent hypoxia: hypersomnolence and brain injury. Am J Respir Crit Care Med. (2005) 171:1414–20. doi: 10.1164/rccm.200411-1564OC
36. Huang Y-S, Guilleminault C, Hwang F-M, Cheng C, Lin C-H, Li H-Y, et al. Inflammatory cytokines in pediatric obstructive sleep apnea. Medicine. (2016) 95:e4944. doi: 10.1097/MD.0000000000004944
37. Nanduri J, Prabhakar NR. Developmental programming of O(2) sensing by neonatal intermittent hypoxia via epigenetic mechanisms. Respir Physiol Neurobiol. (2013) 185:105–9. doi: 10.1016/j.resp.2012.07.016
38. Pawar A, Nanduri J, Yuan G, Khan SA, Wang N, Kumar GK, et al. Reactive oxygen species-dependent endothelin signaling is required for augmented hypoxic sensory response of the neonatal carotid body by intermittent hypoxia. Am J Physiol Regul Integr Comp Physiol. (2009) 296:R735–42. doi: 10.1152/ajpregu.90490.2008
39. Schwarz JM, Sholar PW, Bilbo SD. Sex differences in microglial colonization of the developing rat brain. J Neurochem. (2012) 120:948–63. doi: 10.1111/j.1471-4159.2011.07630.x
40. Weinhard L, Neniskyte U, Vadisiute A, di Bartolomei G, Aygün N, Riviere L, et al. Sexual dimorphism of microglia and synapses during mouse postnatal development. Dev Neurobiol. (2018) 78:618–26. doi: 10.1002/dneu.22568
41. McGrath J, Saha S, Welham J, El Saadi O, MacCauley C, Chant D. A systematic review of the incidence of schizophrenia: the distribution of rates and the influence of sex, urbanicity, migrant status and methodology. BMC Med. (2004) 2:13. doi: 10.1186/1741-7015-2-13
42. Werling DM, Geschwind DH. Sex differences in autism spectrum disorders. Curr Opin Neurol. (2013) 26:146–53. doi: 10.1097/WCO.0b013e32835ee548
43. Nelson LH, Warden S, Lenz KM. Sex differences in microglial phagocytosis in the neonatal hippocampus. Brain Behav Immun. (2017) 64:11–22. doi: 10.1016/j.bbi.2017.03.010
44. Johnson SM, Randhawa KS, Epstein JJ, Gustafson E, Hocker AD, Huxtable AG, et al. Gestational intermittent hypoxia increases susceptibility to neuroinflammation and alters respiratory motor control in neonatal rats. Respir Physiol Neurobiol. (2017) 256:128–42. doi: 10.1016/j.resp.2017.11.007
45. Brockmann PE, Damiani F, Nuñez F, Moya A, Pincheira E, Paul MA, et al. Sleep-disordered breathing in children with Down syndrome: usefulness of home polysomnography. Int J Pediatr Otorhinolaryngol. (2016) 83:47–50. doi: 10.1016/j.ijporl.2016.01.030
46. Smith SMC, Kimyon RS, Watters JJ. Cell-type-specific Jumonji histone demethylase gene expression in the healthy rat CNS: detection by a novel flow cytometry method. ASN Neuro. (2014) 6:193–207. doi: 10.1042/AN20130050
47. Nikodemova M, Watters JJ. Efficient isolation of live microglia with preserved phenotypes from adult mouse brain. J Neuroinflammation. (2012) 9:147. doi: 10.1186/1742-2094-9-147
48. Crain JM, Nikodemova M, Watters JJ. Microglia express distinct M1 and M2 phenotypic markers in the postnatal and adult central nervous system in male and female mice. J Neurosci Res. (2013) 91:1143–51. doi: 10.1002/jnr.23242
49. Livak KJ, Schmittgen TD. Analysis of relative gene expression data using real-time quantitative PCR and the 2–ΔΔCT method. Methods. (2001) 25:402–8. doi: 10.1006/meth.2001.1262
50. McCarthy DJ, Chen Y, Smyth GK. Differential expression analysis of multifactor RNA-Seq experiments with respect to biological variation. Nucleic Acids Res. (2012) 40:4288–97. doi: 10.1093/nar/gks042
51. Robinson MD, McCarthy DJ, Smyth GK. edgeR: a Bioconductor package for differential expression analysis of digital gene expression data. Bioinformatics. (2010) 26:139–40. doi: 10.1093/bioinformatics/btp616
52. Huang DW, Sherman BT, Lempicki RA. Systematic and integrative analysis of large gene lists using DAVID bioinformatics resources. Nat Protoc. (2009) 4:44–57. doi: 10.1038/nprot.2008.211
53. Huang DW, Sherman BT, Lempicki RA. Bioinformatics enrichment tools: paths toward the comprehensive functional analysis of large gene lists. Nucleic Acids Res. (2009) 37:1–13. doi: 10.1093/nar/gkn923
54. Thomas PD, Campbell MJ, Kejariwal A, Mi H, Karlak B, Daverman R, et al. PANTHER: a library of protein families and subfamilies indexed by function. Genome Res. (2003) 13:2129–41. doi: 10.1101/gr.772403
55. Heinz S, Benner C, Spann N, Bertolino E, Lin YC, Laslo P, et al. Simple combinations of lineage-determining transcription factors prime cis-regulatory elements required for macrophage and B cell identities. Mol Cell. (2010) 38:576–89. doi: 10.1016/j.molcel.2010.05.004
56. Szklarczyk D, Franceschini A, Wyder S, Forslund K, Heller D, Huerta-Cepas J, et al. STRING v10: protein-protein interaction networks, integrated over the tree of life. Nucleic Acids Res. (2015) 43:D447–52. doi: 10.1093/nar/gku1003
57. Cahill ME, Xie Z, Day M, Photowala H, Barbolina MV, Miller CA, et al. Kalirin regulates cortical spine morphogenesis and disease-related behavioral phenotypes. Proc Natl Acad Sci USA. (2009) 106:13058–63. doi: 10.1073/pnas.0904636106
58. Cahill ME, Walker DM, Gancarz AM, Wang ZJ, Lardner CK, Bagot RC, et al. The dendritic spine morphogenic effects of repeated cocaine use occur through the regulation of serum response factor signaling. Mol Psychiatry. (2018) 23:1474–86. doi: 10.1038/mp.2017.116
59. Cote-Sierra J, Foucras G, Guo L, Chiodetti L, Young HA, Hu-Li J, et al. Interleukin 2 plays a central role in Th2 differentiation. Proc Natl Acad Sci USA. (2004) 101:3880–5. doi: 10.1073/pnas.0400339101
60. Teige I, Liu Y, Issazadeh-Navikas S. IFN-β inhibits T cell activation capacity of central nervous system APCs. J Immunol. (2006) 177:3542–53. doi: 10.4049/jimmunol.177.6.3542
61. Perry VH, Gordon S. Modulation of CD4 antigen on macrophages and microglia in rat brain. J Exp Med. (1987) 166:1138–43.
62. Baruch K, Ron-Harel N, Gal H, Deczkowska A, Shifrut E, Ndifon W, et al. CNS-specific immunity at the choroid plexus shifts toward destructive Th2 inflammation in brain aging. PNAS. (2013) 110:2264–9. doi: 10.1073/pnas.1211270110
63. Baruch K, Deczkowska A, David E, Castellano JM, Miller O, Kertser A, et al. Aging-induced type I interferon response at the choroid plexus negatively affects brain function. Science. (2014) 346:89–93. doi: 10.1126/science.1252945
64. Gadani SP, Cronk JC, Norris GT, Kipnis J. IL-4 in the brain: a cytokine to remember. J Immunol. (2012) 189:4213–9. doi: 10.4049/jimmunol.1202246
65. Lacy M, Hauser M, Pliskin N, Assuras S, Valentine MO, Reder A. The effects of long-term interferon-beta-1b treatment on cognitive functioning in multiple sclerosis: a 16-year longitudinal study. Mult Scler J. (2013) 19:1765–72. doi: 10.1177/1352458513485981
66. Cahill ME, Bagot RC, Gancarz AM, Walker DM, Sun H, Wang Z-J, et al. Bidirectional synaptic structural plasticity after chronic cocaine administration occurs through Rap1 small GTPase signaling. Neuron. (2016) 89:566–82. doi: 10.1016/j.neuron.2016.01.031
67. Elliott MR, Koster KM, Murphy PS. Efferocytosis signaling in the regulation of macrophage inflammatory responses. J Immunol. (2017) 198:1387–94. doi: 10.4049/jimmunol.1601520
68. Linton MF, Babaev VR, Huang J, Linton EF, Tao H, Yancey PG. Macrophage apoptosis and efferocytosis in the pathogenesis of atherosclerosis. Circ J. (2016) 80:2259–68. doi: 10.1253/circj.CJ-16-0924
69. Pujol-Autonell I, Ampudia R-M, Planas R, Marin-Gallen S, Carrascal J, Sanchez A, et al. Efferocytosis promotes suppressive effects on dendritic cells through prostaglandin E2 production in the context of autoimmunity. PLoS ONE. (2013) 8:e63296. doi: 10.1371/journal.pone.0063296
70. Ipseiz N, Uderhardt S, Scholtysek C, Steffen M, Schabbauer G, Bozec A, et al. The nuclear receptor Nr4a1 mediates anti-inflammatory effects of apoptotic cells. J Immunol. (2014) 192:4852–8. doi: 10.4049/jimmunol.1303377
71. Wlodarczyk A, Holtman IR, Krueger M, Yogev N, Bruttger J, Khorooshi R, et al. A novel microglial subset plays a key role in myelinogenesis in developing brain. EMBO J. (2017) 36:3292–308. doi: 10.15252/embj.201696056
72. Herz J, Filiano AJ, Smith A, Yogev N, Kipnis J. Myeloid cells in the central nervous system. Immunity. (2017) 46:943–56. doi: 10.1016/j.immuni.2017.06.007
73. Santini SM, Lapenta C, Logozzi M, Parlato S, Spada M, Di Pucchio T, et al. Type I interferon as a powerful adjuvant for monocyte-derived dendritic cell development and activity in vitro and in Hu-PBL-SCID mice. J Exp Med. (2000) 191:1777–88. doi: 10.1084/jem.191.10.1777
74. Montoya M, Schiavoni G, Mattei F, Gresser I, Belardelli F, Borrow P, et al. Type I interferons produced by dendritic cells promote their phenotypic and functional activation. Blood. (2002) 99:3263–71. doi: 10.1182/blood.V99.9.3263
75. Angele MK, Ayala A, Monfils BA, Cioffi WG, Bland KI, Chaudry IH. Testosterone and/or low estradiol: normally required but harmful immunologically for males after trauma-hemorrhage. J Trauma. (1998) 44:78–85. doi: 10.1097/00005373-199801000-00007
76. McCrohon JA, Jessup W, Handelsman DJ, Celermajer DS. Androgen exposure increases human monocyte adhesion to vascular endothelium and endothelial cell expression of vascular cell adhesion molecule-1. Circulation. (1999) 99:2317–22.
77. Ramien C, Taenzer A, Lupu A, Heckmann N, Engler JB, Patas K, et al. Sex effects on inflammatory and neurodegenerative processes in multiple sclerosis. Neurosci Biobehav Rev. (2016) 67:137–46. doi: 10.1016/j.neubiorev.2015.12.015
78. Mishra JS, Hankins GD, Kumar S. Testosterone downregulates angiotensin II type-2 receptor via androgen receptor-mediated ERK1/2 MAP kinase pathway in rat aorta. J Renin Angiotensin Aldosterone Syst. (2016) 17:1470320316674875. doi: 10.1177/1470320316674875
79. Nuñez JL, McCarthy MM. Androgens predispose males to GABAA-mediated excitotoxicity in the developing hippocampus. Exp Neurol. (2008) 210:699–708. doi: 10.1016/j.expneurol.2008.01.001
80. Orr B, Vanpoucke G, Grace OC, Smith L, Anderson RA, Riddick ACP, et al. Expression of pleiotrophin in the prostate is androgen regulated and it functions as an autocrine regulator of mesenchyme and cancer associated fibroblasts and as a paracrine regulator of epithelia. Prostate. (2011) 71:305–17. doi: 10.1002/pros.21244
81. Verhovshek T, Cai Y, Osborne MC, Sengelaub DR. Androgen regulates brain-derived neurotrophic factor in spinal motoneurons and their target musculature. Endocrinology. (2010) 151:253–61. doi: 10.1210/en.2009-1036
82. Nanduri J, Makarenko V, Reddy VD, Yuan G, Pawar A, Wang N, et al. Epigenetic regulation of hypoxic sensing disrupts cardiorespiratory homeostasis. Proc Natl Acad Sci USA. (2012) 109:2515–20. doi: 10.1073/pnas.1120600109
83. Mendoza-Fernández V, Andrew RD, Barajas-López C. Interferon-α inhibits long-term potentiation and unmasks a long-term depression in the rat hippocampus. Brain Res. (2000) 885:14–24. doi: 10.1016/S0006-8993(00)02877-8
84. Farré R, Montserrat JM, Gozal D, Almendros I, Navajas D. Intermittent hypoxia severity in animal models of sleep apnea. Front Physiol. (2018) 9:1556. doi: 10.3389/fphys.2018.01556
85. Schmidt-Neilsen K, Larimer JL. Oxygen dissociation curves of mammalian blood in relation to body size. Am J Physiol Content. (1958) 195:424–8. doi: 10.1152/ajplegacy.1958.195.2.424
86. Frank MG, Ruby NF, Heller HC, Franken P. Development of circadian sleep regulation in the rat: a longitudinal study under constant conditions. Sleep. (2017) 40:zsw077. doi: 10.1093/sleep/zsw077
Keywords: microglia, intermittent hypoxia, development, neonate, sex differences
Citation: Kiernan EA, Wang T, Vanderplow AM, Cherukuri S, Cahill ME and Watters JJ (2019) Neonatal Intermittent Hypoxia Induces Lasting Sex-Specific Augmentation of Rat Microglial Cytokine Expression. Front. Immunol. 10:1479. doi: 10.3389/fimmu.2019.01479
Received: 13 March 2019; Accepted: 13 June 2019;
Published: 02 July 2019.
Edited by:
Jorge Matias-Guiu, Complutense University of Madrid, SpainReviewed by:
Jose A. Martínez-Orgado, San Carlos University Clinical Hospital, SpainMaria F. Cano-Abad, Autonomous University of Madrid, Spain
Copyright © 2019 Kiernan, Wang, Vanderplow, Cherukuri, Cahill and Watters. This is an open-access article distributed under the terms of the Creative Commons Attribution License (CC BY). The use, distribution or reproduction in other forums is permitted, provided the original author(s) and the copyright owner(s) are credited and that the original publication in this journal is cited, in accordance with accepted academic practice. No use, distribution or reproduction is permitted which does not comply with these terms.
*Correspondence: Jyoti J. Watters, amp3YXR0ZXJzQHdpc2MuZWR1