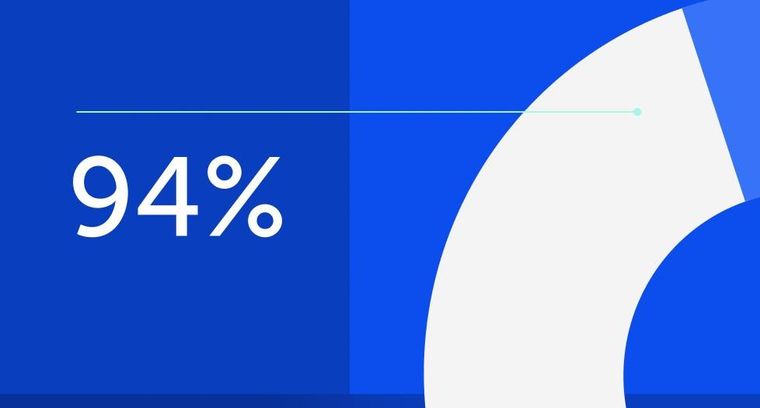
94% of researchers rate our articles as excellent or good
Learn more about the work of our research integrity team to safeguard the quality of each article we publish.
Find out more
MINI REVIEW article
Front. Immunol., 04 June 2019
Sec. Vaccines and Molecular Therapeutics
Volume 10 - 2019 | https://doi.org/10.3389/fimmu.2019.01278
This article is part of the Research TopicTargeting Angiogenesis to Treat Autoimmune Diseases and Cancer View all 11 articles
Angiogenesis is facilitated by the proteolytic activities of members of the matrix metalloproteinase (MMP) family. More specifically, MMP-9 and MT1-MMP directly regulate angiogenesis, while several studies indicate a role for MMP-2 as well. The correlation of MMP activity to tumor angiogenesis has instigated numerous drug development programs. However, broad-based and Zn2+-chelating MMP inhibitors have fared poorly in the clinic. Selective MMP inhibition by antibodies, biologicals, and small molecules has utilized unique modes of action, such as (a) binding to protease secondary binding sites (exosites), (b) allosterically blocking the protease active site, or (c) preventing proMMP activation. Clinical trials have been undertaken with several of these inhibitors, while others are in advanced pre-clinical stages. The mechanistically non-traditional MMP inhibitors offer treatment strategies for tumor angiogenesis that avoid the off-target toxicities and lack of specificity that plagued Zn2+-chelating inhibitors.
During the process of angiogenesis (the development of new blood vessels), the extracellular matrix (ECM) is degraded by matrix metalloproteinases (MMPs), facilitating endothelial cell invasion and leading to sprouting of new vessels (1–3). The MMP family (Figure 1) has fairly conserved sequences between species, indicating that they are part of essential biological processes. The domain organization of MMPs is also fairly conserved, as all contain a signal peptide, a pro-domain, and a catalytic (CAT) domain with a Zn2+ binding His-Glu-X-X-His-X-X-Gly-X-X-His motif (Figure 1). Most MMPs contain a linker region and a hemopexin-like (HPX) domain (Figure 1). In addition, some harbor specific features such as a furin activation domain (MMP-14/MT1-MMP, MMP-15/MT2-MMP, MMP-16/MT3-MMP, MMP-21, MMP-24/MT5-MMP, MMP-23, and MMP-28), fibronectin type II middle inserts (MMP-2 and MMP-9), and/or a transmembrane domain (MMP-14/MT1-MMP, MMP-15/MT2-MMP, MMP-16/MT3-MMP, and MMP-24/MT5-MMP) (Figure 1).
MMP-9 and MT1-MMP directly regulate angiogenesis, while some studies indicate a role for MMP-2 as well (1, 4). Tumor angiogenesis and growth is reduced in MMP-2 knockout mice (1). MMP-9 has been well-documented as a key contributor to the “angiogenic switch” in cancer progression (5–8). The roles of MMP-9 in angiogenesis include the release of vascular endothelial growth factor (VEGF) and/or basic fibroblast growth factor (FGF-2) (5, 7). Tumor-associated macrophages, once polarized into the M2 phenotype, release VEGF and MMP-9 (9). MT1-MMP contributes to blood vessel invasion, FGF-2-induced corneal angiogenesis, endothelial cell migration and tubulogenesis in three-dimensional collagen matrices, and vascular lumen formation (10–15).
Inhibiting enzymes involved in tumor-driven angiogenesis has been recognized as a potential anticancer therapy (16). Broad spectrum and moderately selective MMP inhibitors have been recognized as possessing antiangiogenic activity (17–19). The majority of MMP inhibitors contain a hydroxamic acid group which chelates the active site Zn2+ (20–24). Problems with hydroxamic acid-based metalloprotease inhibitors include the tendency of hydroxamic acids to chelate zinc in a non-selective fashion (25). An often observed side effect of hydroxamic acid-based MMP inhibitors has been musculoskeletal syndrome (MSS). MSS has been attributed to combined inhibition of MMP-1 and a disintegrin and metalloproteinase 17 (ADAM17) (26). A pyrimidine-2,4,6-trione derivative that selectively inhibits MT1-MMP, MMP-2, and MMP-9 is not associated with MSS (27). Recent advances in the development of selective MMP inhibitors have included unique modes of action for inhibiting MMPs implicated in angiogenesis (MMP-2, MMP-9, and MT1-MMP).
Mechanism-based inhibitors selective for MMP-2 and MMP-9 were developed based on the thiirane moiety (Figure 2A) (28). Although it was initially proposed that the thiirane would be activated via coordination with the active site Zn2+, allowing for covalent modification by an active site nucleophile (28), subsequent studies revealed a mechanism by which deprotonation at the methylene adjacent to the sulfone occurred, initiating ring opening of the thiirane and formation of a stable Zn2+-thiolate complex (31). The thiirane-based inhibitor SB-3CT (Figure 2A) exhibited antiangiogenic and antimetastatic behaviors (32, 33). In vivo, SB-3CT was found to be metabolized by several routes, including p-hydroxylation, hydroxylation at the methylene adjacent to the sulfone leading to sulfinic acid formation, and glutathione-based Cys conjugation of the thiirane ring (34). α-Methyl variants of SB-3CT had improved metabolic profiles, as only oxidation of the thiirane sulfur was observed (35). Unfortunately, SB-3CT was poorly water soluble. Thiirane-based inhibitors with improved water solubility were subsequently developed (36). ND-322 (which was selective for MMP-2 and MT1-MMP) was found to have antimetastatic activity (37), while the O-phosphate prodrug form of SB-3CT crossed the blood-brain barrier (38).
Figure 2. Structures of MMP small molecule inhibitors (A) thiiranes (where n = 1 for SB-3CT), (B) N-[4-(difluoromethoxy)phenyl]-2-[(4-oxo-6-propyl-1 H-pyrimidin-2-yl)sulfanyl]-acetamide, (C) N-(4-fluorophenyl)-4-(4-oxo-3,4,5,6,7,8-hexahydroquinazolin-2-ylthio)butanamide, (D) JNJ0966 [N-(2-((2-methoxyphenyl) amino)-4′-methyl-[4,5′-bithiazol]-2′-yl)acetamide], and (E) NSC405020 [3,4-dichloro-N-(1-methylbutyl)benzamide], and (bottom) MMP inhibitory antibodies (IgG) and antibody fragments. Illustrations reprinted with permission from Brown et al. (28), Alford et al. (29), and Santamaria and de Groot (30). Copyright 2000 and 2017 American Chemical Society and 2018 John Wiley and Sons.
Targeting antibodies (Abs) (Figure 2, bottom) directly to the Zn2+ complex in the MMP active site (designated metallobodies) could have superior properties over classical Abs by mimicking the molecular recognition offered by the endogenous inhibitors of MMPs, tissue inhibitor of metalloproteinases (TIMPs), while providing better selectivity (39). Mice were immunized with synthetic organic ligands bound to a metal ion (Zinc-Tripod), which mimicked the MMP catalytic Zn2+ complex. This was followed by immunization with the full-length MMP. The immunization procedure yielded function blocking metallobodies (SDS3 and SDS4) directed at the catalytic Zn2+ and enzyme surface epitopes in activated MMP-9 (39). Metallobodies SDS3 and SDS4 bound and inhibited MMP-9 with KD = 200 and 20 nM, respectively, and Ki = 1 μM and 54 nM, respectively. SDS3 and SDS4 also effectively inhibited MMP-2, but had no inhibitory activity toward MMP-1, MMP-7, MMP-12, or ADAM17, and more than an order of magnitude lower activity toward MT1-MMP. SDS3 was shown, in both prophylactic and therapeutic applications, to protect mice from dextran sodium sulfate-induced colitis (39).
In general, metalloproteinases use the nucleophilic attack of a water molecule as one of the steps of amide bond hydrolysis (40). Water addition to the amide carbonyl results in a tetrahedral transition state. Phosphinic peptides [Ψ{PO2H-CH2}] are analogs of this transition state and behave as inhibitors of MMPs (41). Phosphinate triple-helical (collagen mimic) MMP inhibitors allow incorporation of specificity elements for both the S and S' subsites of the enzyme. Although binding to the non-primed region of the active site is generally weaker than the primed site to prevent product inhibition (40), it does add sequence diversity and potential selectivity. Triple-helical structure allows for interaction with both the active site and secondary binding sites (exosites) of collagenolytic MMPs (42–44), which include MMP-2, MMP-9, and MT1-MMP (45).
Our laboratory produced a series of triple-helical peptide inhibitors (THPIs) based on GlyΨ{PO2H-CH2}Leu, GlyΨ{PO2H-CH2}Val, and GlyΨ{PO2H-CH2}Ile transition state analogs (42, 46–51). The α1(V)GlyΨ{PO2H-CH2}Val THPI [C6-(Gly-Pro-Hyp)4-Gly-Pro-Pro-GlyΨ{PO2H-CH2}(R,S)Val- Val-Gly-Glu-Gln-Gly-Glu-Gln-Gly-Pro-Pro-(Gly-Pro-Hyp)4-NH2], based on the cleavage site in type V collagen by MMP-9 (52), was a selective inhibitor for MMP-2 and MMP-9 (46). The thermal stability of the α1(V)GlyΨ{PO2H-CH2}Val THPI was greatly reduced compared to the parent substrate (46, 53). We synthesized a stabilized version of the α1(V)GlyΨ{PO2H-CH2}Val THPI, designated α1(V)GlyΨ{PO2H-CH2}Val [mep14, 32,Flp15, 33] THPI, where mep was (2S,4R)-4-methylproline and Flp was (2S,4R)-4-fluoroproline (51). α1(V)GlyΨ{PO2H-CH2}Val [mep14, 32,Flp15, 33] THPI had a melting point (Tm value) 18 °C higher than α1(V)GlyΨ{PO2H-CH2}Val THPI (51). α1(V)GlyΨ{PO2H-CH2}Val [mep14, 32,Flp15, 33] THPI exhibited Ki values against MMP-2 and MMP-9 of 189.1 and 90.6 nM, respectively, at 25°C, and 2.24 and 0.98 nM, respectively, at 37°C (51).
Triple-helical peptides (THPs) have been found to be reasonably stable to general proteolysis, as observed in vitro in mouse, rat, and human serum and/or plasma and in vivo in rats (54–58). The stability of THPs has allowed for their administration orally (59). The α1(V)GlyΨ{PO2H-CH2}Val [mep14, 32,Flp15, 33] THPI was effective in vivo in a mouse model of multiple sclerosis, reducing clinical severity and weight loss (51).
Chlorotoxin (ClTx) is 36-residue peptide isolated from the venom of the Israeli Yellow scorpion Leiurus quinquestriatus (60). ClTx preferentially binds neuroectodermal tumors and exhibits antiangiogenic and anti-invasion activity (61–65). ClTx selectively inhibits MMP-2 in a dose-dependent manner (KD ~ 115 nM) (62). The ClTx interaction with a membrane complex of chloride channel-3 (ClC-3) and MMP-2 (66) has been used to create numerous cancer imaging agents (63, 65, 67–69). ClTx can pass through the blood-brain barrier (65), and has yielded promising preclinical and clinical results in the treatment of glioblastoma (64, 68).
Mouse mAb REGA-3G12, a selective inhibitor of MMP-9, was prepared using MMP-9 as antigen (70). REGA-3G12 recognized the MMP-9 Trp116 to Lys214 region, located in the CAT domain but not part of the Zn2+ binding site (71). REGA-3G12 bound to MMP-9 with KD = 2.1 nM (70). REGA-3G12 prevented interleukin-8-induced mobilization of hematopoietic progenitor cells in rhesus monkeys (72). A single chain variable fragment (scFv) (Figure 2, bottom) derived from REGA-3G12 selectively inhibited MMP-9 compared to MMP-2 (73). Gelatin hydrolysis was inhibited 44% at a scFv concentration of 5 μM (73).
Two monoclonal anti-MMP-9 antibodies, AB0041 and AB0046, were shown to inhibit tumor growth and metastasis in a surgical orthotopic xenograft model of colorectal carcinoma (74). AB0046 improved immune responses to tumors, as the inhibition of MMP-9 reversed MMP-9 inactivation of T-cell chemoattractant CXCR3 ligands (CXCL9, CXCL10, and CXCL11) (75). A humanized version of AB0041, GS-5745 (Andecaliximab), was generated for use in clinical trials (74). GS-5745 was found to bind to MMP-9 near the junction between the pro-domain and CAT domain, distal to the active site, and (a) inhibited proMMP-9 activation and (b) non-competitively inhibited MMP-9 activity (76). GS-5745 bound to MMP-9 with ~150-400-fold weaker affinity compared with proMMP-9 (KD = 2.0–6.6 vs. 0.008–0.043 nM) (76). GS-5745/Andecaliximab has been evaluated under several clinical trials. A randomized placebo controlled phase 1b single and multiple ascending dose-ranging clinical trial on 72 patients diagnosed with moderately to severely active ulcerative colitis (UC) showed that GS-5745 was safe, well-tolerated, and could be used as a potential therapeutic agent for UC (77). A phase 2/3 UC study with 165 patients treated over 8 weeks further indicated that GS-5745 was well-tolerated (78). A phase 1b trial investigating the safety, pharmacokinetics, and disease-related outcomes for 15 rheumatoid arthritis patients (ClinicalTrials.gov Identifier NCT02176876) demonstrated that GS-5745 was safe, with adverse events that were only grade 1 or 2 in severity and no indication of MSS (79).
Several non-active site small molecule MMP-9 inhibitors have been described. N-[4-(difluoromethoxy)phenyl]-2-[(4-oxo-6-propyl-1H-pyrimidin-2-yl)sulfanyl]-acetamide (Figure 2B) bound selectively to the MMP-9 HPX domain with KD = 2.1 μM and inhibited tumor growth and lung metastasis in MDA-MB-435 mouse models (80). Based on this lead compound a library of analogs was generated, and N-(4-fluorophenyl)-4-(4-oxo-3,4,5,6,7,8-hexahydroquinazolin-2-ylthio)butanamide (Figure 2C) emerged as a more potent inhibitor (KD = 320 nM) (29). This compound prevented association of proMMP-9 with the α4β1 integrin and CD44, resulting in the dissociation of epidermal growth factor receptor (EGFR) from the β1 integrin subunit and CD44 (29). High-throughput screening led to the identification of compound JNJ0966 [N-(2-((2-methoxyphenyl)amino)-4′-methyl-[4,5′-bithiazol]-2′-yl)acetamide] (Figure 2D), which bound selectively to proMMP-9 with KD = 5.0 μM (81). JNJ0966 inhibited the activation of proMMP-9 and the migration of HT1080 cells, and was able to penetrate the blood-brain barrier (81).
Several selective MT1-MMP inhibitory antibodies and antibody fragments have been described (27, 30, 82–84). Screening a human Fab display phage library resulted in the development of DX-2400, a selective, fully human MT1-MMP inhibitory antibody (Ki = 0.8 nM) (27, 85). DX-2400 was a competitive inhibitor of MT1-MMP (85). DX-2400 inhibited tumor MT1-MMP activity, resulting in the inhibition of MDA-MB-231 primary tumor growth but not MCF-7 tumor growth in xenograft mouse models (85). DX-2400 also inhibited metastasis (85), and enhanced tumor response to radiation therapy (86).
Recombinant human scFv antibodies (Figure 2, bottom) were generated against the MT1-MMP HPX domain (87). Two scFv antibodies, CHA and CHL (KD = 10.7 and 169 nM, respectively), were found to have differing activities. CHL inhibited MT1-MMP binding to collagen, while CHA had the opposite effect, yet both scFv antibodies inhibited HT1080 invasion of type I collagen. CHA inhibited CD44 shedding and endothelial cell sprouting from endothelial cell/fibroblast co-cultures in type I collagen, while CHL had no effect on either activity (87).
Monoclonal antibody (mAb) 9E8 (KD = 0.6 nM) inhibited MT1-MMP activation of proMMP-2, but not other MT1-MMP catalytic activities (88). mAb 9E8 bound to the Pro163 to Gln174 loop in the MT1-MMP CAT domain (89). This loop region is present in the CAT domain of MT1-MT6-MMPs, but is not found in all other MMPs. mAb 9E8 prevented formation of the MT1-MMP•TIMP-2•proMMP-2 complex required for proMMP-2 activation by interfering with TIMP-2 binding (89). Another antibody raised against the loop region, LOOPAb, also inhibited MT1-MMP activation of proMMP-2, but not MT1-MMP collagenolysis (90).
The LEM-2/15 antibody was generated using a cyclic peptide mimicking the MT1-MMP CAT domain V-B loop (residues 218-233) (91). A minimized Fab fragment (Figure 2, bottom) of LEM-2/15 was designed, and possessed a reasonable binding affinity compared to the intact antibody (KD = 2.3 vs. 0.4 nM, respectively) (92). The Fab fragment was a non-competitive inhibitor of MT1-MMP activities, including collagenolysis (92). The Fab fragment of LEM-2/15 induced a conformational change in MT1-MMP by destabilizing the exposed region of the V-B loop, ultimately narrowing the substrate binding cleft (30, 84, 92). Treatment with the Fab fragment of LEM-2/15 significantly increased the ability of virally infected mice to fight off secondary Strep. pneumoniae bacterial infection (93). Treatment with the Fab fragment of LEM-2/15, before or after infection, helped to maintain tissue integrity (93).
Human scFv-Fc (Figure 2, bottom) antibody E3 bound to the MT1-MMP CAT domain and inhibited type I collagen binding (94). A second generation E3 clone (E2_C6, KD = 0.11 nM) inhibited tumor growth and metastasis (94).
Human antibody Fab libraries were synthesized where the Peptide G sequence (Phe-Ser-Ile-Ala-His-Glu) (95) was incorporated into complementarity determining region (CDR)-H3 (96). Fab 1F8 exhibited EC50 = 8.3 nM against the MT1-MMP CAT domain, and inhibited MT1-MMP CAT domain activity with Ki = 110 nM (96).
Screening of a phage displayed synthetic humanized Fab library led to the identification of Fab 3369 (97). Fab 3369 inhibited the activity of the MT1-MMP CAT domain with IC50 = 62 nM (97). IgG 3369 treatment of MDA-MB-231 mammary orthotopic xenograft mice reduced lung metastases, collagen processing, and tumor density of CD31+ blood vessels (97).
It has been noted that antibody antigen binding sites are not complimentary to the concave shape of catalytic clefts, as antigen binding sites are planar or concave (84). To overcome this, the convex-shaped paratope of camelid antibodies was incorporated into the human antibody scaffold (98). Fab 3A2 bound selectively to MT1-MMP CAT domain outside of the active site cavity with KD = 4.8 nM, and was a competitive inhibitor with Ki = 9.7 nM (98, 99). Fab 3A2 inhibited MT1-MMP collagenolysis and reduced metastasis in a melanoma mouse model (99).
Virtual ligand screening of the NCI/NIH Developmental Therapeutics Program ~275,000 compound library resulted in the identification of compound NSC405020 [3,4-dichloro-N-(1-methylbutyl)benzamide] (Figure 2E), a small molecule MT1-MMP HPX domain inhibitor (100). NSC405020 inhibited MT1-MMP homodimerization but not proMMP-2 activation or catalytic activity toward a peptide substrate. NSC405020 reduced the collagenolytic activity of MCF7-β3/MT1-MMP cells and was effective in vivo, as intratumoral injections reduced tumor size significantly (100).
Tumor growth is limited without the ability of the tumor to create its own blood supply (101). The use of antiangiogenic therapeutic agents is viewed as beneficial due to (a) the prevention of new blood vessel formation and/or (b) the normalization of tumor-associated vasculature (102). Normalizing the tumor-associated vasculature can enhance the penetration of therapeutic agents (102, 103). Clinically utilized antiangiogenic agents typically target VEGF or the VEGF receptor (VEGFR), or are multikinase inhibitors (102). Significant improvement in overall survival and prolonged progression-free survival was observed when angiogenesis inhibitors were applied in gastric cancer (104). Anti-VEGFR-2 and multikinase inhibitor treatments were more efficacious than anti-VEGF treatment (104). This was suggested to be due to blocking only VEGF-A in the latter treatment (104). Thus, angiogenesis targeting via MMP inhibition could be very efficacious based on the potential broader impact than just VEGF-A inhibition (as discussed in the Introduction). The ability of the combination of angiogenesis inhibition and chemotherapy to prolong progression-free survival in patients with gastric cancer was dependent upon the antiangiogenic agent used (104).
Antiangiogenic therapies can have serious side effects, such as bleeding, venous or arterial thromboembolisms, proteinuria, and hypertension, and can also increase drug resistance, cancer invasion, and metastasis (102, 104–106). An obvious concern is that antiangiogenic approaches can negatively impact capillaries and blood flow in healthy tissues (104). Additionally, targeting VEGF can lead to upregulation of other pro-angiogenic factors (107, 108). All in all, side effects from the use of angiogenesis inhibitors are often viewed as manageable (104, 105, 109).
Unique modes of action have been used to develop antibody-based, triple-helical peptide, and small molecule inhibitors of MMPs implicated in angiogenesis. The selective, small molecule MMP-9 and MT1-MMP inhibitors do not yet have preferred affinities, but represent a promising start based on their novel mechanisms of inhibition. Clinical trials utilizing antibodies have provided evidence that selective MMP inhibitors do not induce MSS. Unfortunately, antibodies are subject to proteolysis, may be removed from circulation rapidly, and are costly. Nonetheless, antibodies have provided truly selective, high affinity MMP inhibitors. Selective, high affinity inhibitors can be developed for MMPs based on triple-helical structure. THPIs have excellent pharmacokinetic properties compared with other peptide-based therapeutics. The mechanistically non-traditional MMP inhibitors offer treatment strategies for tumor angiogenesis that avoid the off-target toxicities and lack of specificity that plagued Zn2+-chelating inhibitors.
One must consider that when applied as antiangiogenic agents, MMP inhibitors may have the undesired effect of (a) limiting turnover of already existing tumor vessels and (b) disrupting vascular homoeostatis, where normal vessel turnover and other related activities are needed. This would be dependent upon which MMP was targeted. For example, MT1-MMP has been shown to contribute to both angiogenesis and vascular regression in an aortic ring model (110). Inhibition of MT1-MMP catalytic activity following the vessel growth phase resulted in reduced vascular regression due to inhibition of collagenolysis (110). Vessels are destabilized by MT1-MMP shedding of Tie-2 from endothelial cells (111), and thus enzyme inhibition could stabilize tumor vessels (103). In similar fashion, TIMP-2 and TIMP-3 were found to stabilize newly formed vascular networks by (a) inhibiting regression and (b) preventing further endothelial cell tube morphogenesis (112). The action of TIMP-2 and TIMP-3 was correlated to MT1-MMP activity, and thus inhibition of MT1-MMP could stabilize vascular networks (112). Deletion of MT1-MMP or inhibition of MT1-MMP activity resulted in increased vascular leakage (103). In this latter case, MT1-MMP was proposed to modulate TGFβ availability, with decreased TGFβ levels impacting vascular homoeostatsis (103). MT1-MMP shedding of endoglin (CD105) results in the release of sEndoglin, which inhibits angiogenesis (113). MMP-9 contributes to edema prevention, which is a component of vascular homoeostasis (103). MMP-2 cleavage of ECM biomolecules leads to disruption of endothelial cell β1 integrin binding and subsequent signaling (114, 115). In turn, disruption of signaling leads to a decrease in MT1-MMP production (114).
Another consideration for MMP inhibition is the effect on the production of antiangiogenic agents, such as angiostatin (from plasminogen), endostatin (from type XVIII collagen), arresten (from the α1(IV) collagen chain), canstatin (from the α2(IV) collagen chain), and tumstatin (from the α3(IV) collagen chain). MMP-9 is capable of generating angiostatin (116, 117), endostatin (118, 119), arresten (120), canstatin (120), and tumstatin (120, 121). However, the redundancy of proteases capable of generating these agents (116, 118, 120) suggests that inhibiting one (such as MMP-9) may have little effect on these particular antiangiogenic activities.
While selective MMP inhibitors are greatly needed, often overlooked is that the timing of MMP inhibitor application is also critical (see above). Application of a broad spectrum MMP inhibitor (marimostat) in combination with gemcitabine significantly improved survival in pancreatic cancer patients with disease confined to the pancreas (122). Presurgical treatment with an oral MMP inhibitor improved survival from 67 to 92% in a mouse breast cancer model (123). As discussed earlier, MMP-9 is a key contributor to the angiogenic switch during carcinogenesis of pancreatic islets (5). However, MMP-9 deficiency in pancreatic ductal adenocarcinoma (PDAC) mouse models resulted in more invasive tumors and an increase in desmoplastic stroma (124). The absence of MMP-9 led to increased interleukin 6 levels in the bone marrow, which activated tumor cell STAT3 signaling and promoted PDAC invasion and metastasis (124). Thus, MMP-9 represents an anti-target in the later stage of pancreatic cancer. The “window of opportunity” for MMP inhibitor application is often in premetastatic disease (125).
The author confirms being the sole contributor of this work and has approved it for publication.
Work on MMP inhibitors in my laboratory has been supported by the National Institutes of Health (CA098799, AR063795, CA239214, and NHLBI contract 268201000036C), the James and Esther King Biomedical Research Program, the US-Israel Binational Science Foundation (BSF), the Center for Molecular Biology and Biotechnology at Florida Atlantic University, and the State of Florida, Executive Office of the Governor's Department of Economic Opportunity.
The author declares that the research was conducted in the absence of any commercial or financial relationships that could be construed as a potential conflict of interest.
Abs, antibodies; ADAM, a disintegrin and metalloproteinase; CAT, catalytic; ClC-3, chloride channel-3; ClTx, chlorotoxin; ECM, extracellular matrix; EGFR, epidermal growth factor receptor; FGF-2, basic fibroblast growth factor 2; Flp, (2S, 4R)-4-fluoroproline; HPX, hemopexin-like; mep, (2S, 4R)-4-methylproline; MMP, matrix metalloproteinase; MSS, musculoskeletal syndrome; PDAC, pancreatic ductal adenocarcinoma; scFv, single chain variable fragment; THP, triple-helical peptide; THPI, triple-helical peptide inhibitor; TIMP, tissue inhibitor of metalloproteinase; UC, ulcerative colitis; VEGF, vascular endothelial growth factor.
1. Egeblad M, Werb Z. New functions for the matrix metalloproteinases in cancer progression. Nat Rev Cancer. (2002) 2:161–74. doi: 10.1038/nrc745
2. Senger DR, Davis GE. Angiogenesis. Cold Spring Harb Perspect Biol. (2011) 3:1–19. doi: 10.1101/cshperspect.a005090
3. Kang H, Hong Z, Zhong M, Klomp J, Bayless KJ, Mehta D, et al. Piezo1 mediates angiogenesis through activation of MT1-MMP signaling. Am J Physiol Cell Physiol. (2019) 316:C92–C103. doi: 10.1152/ajpcell.00346.2018
4. Deryugina EI, Quigley JP. Pleiotropic roles of matrix metalloproteinases in tumor angiogenesis: Contrasting, overlapping and compensatory functions. Biochim Biophys Acta. (2010) 1803:103–20. doi: 10.1016/j.bbamcr.2009.09.017
5. Bergers G, Brekken RA, McMahon G, Vu TH, Itoh T, Tamaki K, et al. Matrix metalloproteinase-9 triggers the angiogenic switch during carcinogenesis. Nat Cell Biol. (2000) 2:737–44. doi: 10.1038/35036374
6. Ardi VC, Kupriyanova TA, Deryugina EI, Quigley JP. Human neutrophils uniquely release TIMP-free MMP-9 to provide a potent catalytic stimulator of angiogenesis. Proc Natl Acad Sci USA. (2007) 104:20262–7. doi: 10.1073/pnas.0706438104
7. Ardi VC, Van den Steen PE, Opdenakker G, Schweighofer B, Deryugina EI, Quigley JP. Neutrophil MMP-9 proenzyme, unencumbered by TIMP-1, undergoes efficient activation in vivo and catalytically induces angiogenesis via a basic fibroblast growth factor (FGF-2)/FGFR-2 pathway. J Biol Chem. (2009) 284:25854–66. doi: 10.1074/jbc.M109.033472
8. Bekes EM, Schweighofer B, Kupriyanova TA, Zajac E, Ardi VC, Quigley JP, et al. Tumor-recruited neutrophils and neutrophil TIMP-free MMP-9 regulate coordinately the levels of tumor angiogenesis and efficiency of malignant cell intravasation. Am J Pathol. (2011) 179:1455–70. doi: 10.1016/j.ajpath.2011.05.031
9. Riabov V, Gudima A, Wang N, Mickley A, Orekhov A, Kzhyshkowska J. Role of tumor associated macrophages in tumor angiogenesis and lymphangiogenesis. Front Physiol. (2014) 5:75. doi: 10.3389/fphys.2014.00075
10. Zhou Z, Apte SS, Soininen R, Cao R, Baaklini GY, Rauser RW, et al. Impaired endochondral ossification and angiogenesis in mice deficient in membrane-type matrix metalloproteinase 1. Proc Natl Acad Sci USA. (2000) 97:4052–7. doi: 10.1073/pnas.060037197
11. Koike T, Vernon RB, Hamner MA, Sadoun E, Reed MJ. MT1-MMP, but not secreted MMPs, influences the migration of human microvascular endothelial cells in 3-dimensional collagen gels. J Cell Biochem. (2002) 86:748–58. doi: 10.1002/jcb.10257
12. Chun T-H, Sabeh F, Ota I, Murphy H, McDonagh KT, Holmbeck K, et al. MT1-MMP-dependent neovessel formation within the confines of the three-dimensional extracellular matrix. J Cell Biol. (2004) 167:757–67. doi: 10.1083/jcb.200405001
13. Genís L, Gálvez BG, Gonzalo P, Arroyo AG. MT1-MMP: universal or particular player in angiogenesis? Cancer Metastasis Rev. (2006) 25:77–86. doi: 10.1007/s10555-006-7891-z
14. Stratman AN, Saunders WB, Sacharidou A, Koh W, Fisher KE, Zawieja DC, et al. Endothelial cell lumen and vascular guidance tunnel formatinon requires MT1-MMP-dependent proteolysis in 3-dimensional collagen matrices. Blood. (2009) 114:237–47. doi: 10.1182/blood-2008-12-196451
15. Sacharidou A, Koh W, Stratman AN, Mayo AM, Fisher KE, Davis GE. Endothelial lumen signaling complexes control 3D matrix-specific tubulogenesis through interdependent Cdc42- and MT1-MMP-mediated events. Blood. (2010) 115:5259–69. doi: 10.1182/blood-2009-11-252692
16. Ricciuti B, Foglietta J, Bianconi V, Sahebkar A, Pirro M. Enzymes involved in tumor-driven angiogenesis: A valuable target for anticancer therapy. Semin Cancer Biol. (2017). doi: 10.1016/j.semcancer.2017.11.005. [Epub ahead of print].
17. Gatto C, Rieppi M, Borsotti P, Innocenti S, Ceruti R, Drudis T, et al. BAY 12-9566, a novel inhibitor of matrix metalloproteinases with antiangiogenic activity. Clin Cancer Res. (1999) 5:3603–7.
18. Shalinsky DR, Brekken J, Zou H, McDermott CD, Forsyth P, Edwards D, et al. Broad antitumor and antiangiogenic activities of AG3340, a potent and selective MMP inhibitor undergoing advanced oncology clinical trials. Ann N Y Acad Sci. (1999) 878:236–70. doi: 10.1111/j.1749-6632.1999.tb07689.x
19. Lockhart AC, Braun RD, Yu D, Ross JR, Dewhirst MW, Humphrey JS, et al. Reduction of wound angiogenesis in patients treated with BMS-275291, a broad spectrum matrix metalloproteinase inhibitor. Clin Cancer Res. (2003) 9:586–93.
20. Beckett RP. Recent advances in the field of matrix metalloproteinase inhibitors. Exp Opin Ther Patents. (1996) 6:1305–15. doi: 10.1517/13543776.6.12.1305
21. Beckett RP, Whittaker M. Matrix metalloproteinase inhibitors 1998. Exp Opin Ther Patents. (1998) 8:259–82. doi: 10.1517/13543776.8.3.259
22. Whittaker M, Floyd CD, Brown P, Gearing AJH. Design and therapeutic application of matrix metalloproteinase inhibitors. Chem Rev. (1999) 99:2735–76. doi: 10.1021/cr9804543
23. Lauer-Fields JL, Fields GB. Matrix metalloproteinase inhibitors and cancer. Exp Opin Ther Patents. (2000) 10:1873–84. doi: 10.1517/13543776.10.12.1873
24. Rao BG. Recent developments in the design of specific matrix metalloproteinase inhibitors aided by structural and computational studies. Curr Pharm Design. (2005) 11:295–322. doi: 10.2174/1381612053382115
25. Saghatelian A, Jessani N, Joseph A, Humphrey M, Cravatt BF. Activity-based probes for the proteomic profiling of metalloproteases. Proc Natl Acad Sci USA. (2004) 101:10000–5. doi: 10.1073/pnas.0402784101
26. Becker DP, Barta TE, Bedell LJ, Boehm TL, Bond BR, Carroll J, et al. Orally active MMP-1 sparing α-tetrahydropyranyl and α-piperidinyl sulfone matrix metalloproteinase (MMP) inhibitors with efficacy in cancer, arthritis, and cardiovascular disease. J Med Chem. (2010) 53:6653–80. doi: 10.1021/jm100669j
27. Devy L, Dransfield DT. New strategies for the next generation of matrix-metalloproteinase inhibitors: selectively targeting membrane-anchored MMPs with therapeutic antibodies. Biochem Res Int. (2011) 2011:191670. doi: 10.1155/2011/191670
28. Brown S, Bernardo MM, Li Z-H, Kotra LP, Tanaka Y, Fridman R, et al. Potent and selective mechanism-based inhibition of gelatinases. J Am Chem Soc. (2000) 122:6799–800. doi: 10.1021/ja001461n
29. Alford VM, Kamath A, Ren X, Kumar K, Gan Q, Awwa M, et al. Targeting the hemopexin-like domain of latent matrix metalloproteinase-9 (proMMP-9) with a small molecule inhibitor prevents the formation of focal adhesion junctions. ACS Chem Biol. (2017) 12:2788–803. doi: 10.1021/acschembio.7b00758
30. Santamaria S, de Groot R. Monoclonal antibodies against metzincin targets. Br J Pharmacol. (2019) 176:52–66. doi: 10.1111/bph.14186
31. Forbes C, Shi Q, Fisher JF, Lee M, Hesek D, Llarrull LI, et al. Active site ring-opening of a thiirane moiety and picomolar inhibition of gelatinases. Chem Biol Drug Des. (2009) 74:527–34. doi: 10.1111/j.1747-0285.2009.00881.x
32. Krüger A, Arlt MJE, Gerg M, Kopitz C, Bernardo MM, Chang M, et al. Antimetastatic activity of a novel mechanism-based gelatinase inhibitor. Cancer Res. (2005) 65:3523–6. doi: 10.1158/0008-5472.CAN-04-3570
33. Bonfil RD, Sabbota A, Nabha S, Bernardo MM, Dong Z, Meng H, et al. Inhibition of human prostate cancer growth, osteolysis and angiogenesis in a bone metastasis model by a novel mechanism-based selective gelatinase inhibitor. Int J Cancer. (2006) 118:2721–6. doi: 10.1002/ijc.21645
34. Celenza G, Villegas-Estrada A, Lee M, Boggess B, Forbes C, Wolter WR, et al. Metabolism of (4-phenoxyphenylsulfonyl) methylthiirane, a selective gelatinase inhibitor. Chem Biol Drug Des. (2008) 71:187–96. doi: 10.1111/j.1747-0285.2008.00632.x
35. Gooyit M, Lee M, Hesek D, Boggess B, Oliver AG, Fridman R, et al. Synthesis, kinetic characterization and metabolism of diastereomeric 2-(1-(4-phenoxyphenylsulfonyl)ethyl)thiiranes as potent gelatinase and MT1-MMP inhibitors. Chem Biol Drug Des. (2009) 74:535–46. doi: 10.1111/j.1747-0285.2009.00898.x
36. Gooyit M, Lee M, Schroeder VA, Ikejiri M, Suckow MA, Mobashery S, et al. Selective water-soluble gelatinase inhibitor prodrugs. J Med Chem. (2011) 54:6676–90. doi: 10.1021/jm200566e
37. Marusak C, Bayles I, Ma J, Gooyit M, Gao M, Chang M, et al. The thiirane-based selective MT1-MMP/MMP2 inhibitor ND-322 reduces melanoma tumor growth and delays metastatic dissemination. Pharmacol Res. (2016) 113:515–20. doi: 10.1016/j.phrs.2016.09.033
38. Lee M, Chen Z, Tomlinson BN, Gooyit M, Hesek D, Juárez MR, et al. Water-soluble MMP-9 inhibitor reduces lesion volume after severe traumatic brain injury. ACS Chem Neurosci. (2015) 6:1658–64. doi: 10.1021/acschemneuro.5b00140
39. Sela-Passwell N, Kikkeri R, Dym O, Rozenberg H, Margalit R, Arad-Yellin R, et al. Antibodies targeting the catalytic zinc complex of activated matrix metalloproteinases show therapeutic potential. Nat Med. (2011) 18:143–7. doi: 10.1038/nm.2582
40. Babine RE, Bender SL. Molecular recognition of protein-ligand complexes: applications to drug design. Chem Rev. (1997) 97:1359–472. doi: 10.1021/cr960370z
41. Gall AL, Ruff M, Kannan R, Cuniasse P, Yiotakis A, Dive V, et al. Crystal structure of the stromelysin-3 (MMP-11) catalytic domain complexed with a phosphinic inhibitor mimicking the transition-state. J Mol Biol. (2001) 307:577–86. doi: 10.1006/jmbi.2001.4493
42. Lauer-Fields JL, Chalmers MJ, Busby SA, Minond D, Griffin PR, Fields GB. Identification of specific hemopexin-like domain residues that facilitate matrix metalloproteinase collagenolytic activity. J Biol Chem. (2009) 284:24017–24. doi: 10.1074/jbc.M109.016873
43. Bertini I, Fragai F, Luchinat C, Melikian M, Toccafondi M, Lauer JL, et al. Structural basis for matrix metalloproteinase 1 catalyzed collagenolysis. J Am Chem Soc. (2012) 134:2100–10. doi: 10.1021/ja208338j
44. Manka SW, Carafoli F, Visse R, Bihan D, Raynal N, Farndale RW, et al. Structural insights into triple-helical collagen cleavage by matrix metalloproteinase 1. Proc Natl Acad Sci USA. (2012) 109:12461–6. doi: 10.1073/pnas.1204991109
45. Amar S, Smith L, Fields GB. Matrix metalloproteinase collagenolysis in health and disease. Biochim Biophys Acta Mol Cell Res. (2017) 1864:1940–51. doi: 10.1016/j.bbamcr.2017.04.015
46. Lauer-Fields JL, Brew K, Whitehead JK, Li S, Hammer RP, Fields GB. Triple-helical transition-state analogs: a new class of selective matrix metalloproteinase inhibitors. J Am Chem Soc. (2007) 129:10408–17. doi: 10.1021/ja0715849
47. Lauer-Fields JL, Whitehead JK, Li S, Hammer RP, Brew K, Fields GB. Selective modulation of matrix metalloproteinase 9 (MMP-9) functions via exosite inhibition. J Biol Chem. (2008) 283:20087–95. doi: 10.1074/jbc.M801438200
48. Bhowmick M, Sappidi RR, Fields GB, Lepore SD. Efficient synthesis of fmoc-protected phosphinic pseuedodipeptides: building blocks for the synthesis of matrix metalloproteinase inhibitors (MMPIs). Biopolymers. (2011) 96:1–3. doi: 10.1002/bip.21425
49. Bhowmick M, Fields GB. Synthesis of Fmoc-Gly-Ile phosphinic pseudodipeptide: residue specific conditions for construction of matrix metalloproteinase inhibitor building blocks. Int J Pept Res Ther. (2012) 18:335–9. doi: 10.1007/s10989-012-9307-y
50. Bhowmick M, Stawikowska R, Tokmina-Roszyk D, Fields GB. Matrix metalloproteinase inhibition by heterotrimeric triple-helical peptide transition state analogs. ChemBioChem. (2015) 16:1084–92. doi: 10.1002/cbic.201402716
51. Bhowmick M, Tokmina-Roszyk D, Onwuha-Ekpete L, Harmon K, Robichaud T, Fuerst R, et al. Second generation triple-helical peptide transition state analog matrix metalloproteinase inhibitors. J Med Chem. (2017) 60:3814–27. doi: 10.1021/acs.jmedchem.7b00018
52. Niyibizi C, Chan R, Wu J-J, Eyre D. A 92 kDa gelatinase (MMP-9) cleavage site in native type V collagen. Biochem Biophys Res Commun. (1994) 202:328–33. doi: 10.1006/bbrc.1994.1931
53. Lauer-Fields JL, Sritharan T, Stack MS, Nagase H, Fields GB. Selective hydrolysis of triple-helical substrates by matrix metalloproteinase-2 and−9. J Biol Chem. (2003) 278:18140–5. doi: 10.1074/jbc.M211330200
54. Fan C-Y, Huang C-C, Chiu W-C, Lai C-C, Liou G-G, Li H-C, et al. Production of multivalent protein binders using a self-trimerizing collagen-like peptide scaffold. FASEB J. (2008) 22:3795–804. doi: 10.1096/fj.08-111484
55. Ndinguri MW, Zheleznyak A, Lauer JL, Anderson CJ, Fields GB. Application of collagen-model triple-helical peptide-amphiphiles for CD44 targeted drug delivery systems. J. Drug Deliv. (2012). 2012:592602. doi: 10.1155/2012/592602
56. Yamazaki CM, Nakase I, Endo H, Kishimoto S, Mashiyama Y, Masuda R, et al. Collagen-like cell-penetrating peptides. Angew Chem Int Ed Engl. (2013) 52:5497–500. doi: 10.1002/anie.201301266
57. Yasui H, Yamazaki CM, Nose H, Awada C, Takao T, Koide T. Potential of collagen-like triple helical peptides as drug carriers: their in vivo distribution, metabolism, and excretion profiles in rodents. Biopolymers. (2013) 100:705–13. doi: 10.1002/bip.22234
58. Shinde A, Feher KM, Hu C, Slowinska K. Peptide internalization enabled by folding: triple-helical cell-penetrating peptides. J Pept Sci. (2015) 21:77–84. doi: 10.1002/psc.2725
59. Koide T, Yamamoto N, Taira KB, Yasui H. Fecal excretion of orally administered collagen-like peptides in rats: contribution of the triple-helical conformation to their stability. Biol Pharm Bull. (2016) 39:135–7. doi: 10.1248/bpb.b15-00561
60. DeBin JA, Strichartz GR. Chloride channel inhibition by the venom of the scorpion Leiurus quinquestriatus. Toxicon. (1991) 29:1403–8. doi: 10.1016/0041-0101(91)90128-E
61. Soroceanu L, Gillespie Y, Khazaeli MB, Sontheimer H. Use of chlorotoxin for targeting of primary brain tumors. Cancer Res. (1998) 58:4871–9.
62. Deshane J, Garner CC, Sontheimer H. Chlorotoxin inhibits glioma cell invasion via matrix metalloproteinase-2. J Biol Chem. (2003) 278:4135–44. doi: 10.1074/jbc.M205662200
63. Mamelak AN. Targeted antitumor therapy with the scorpion venom chlorotoxin. Drugs Future. (2011) 36:615–25. doi: 10.1358/dof.2011.36.8.1656504
64. Cohen-Inbar O, Zaaroor M. Glioblastoma multiforme targeted therapy: the chlorotoxin story. J Clin Neurosci. (2016) 33:52–8. doi: 10.1016/j.jocn.2016.04.012
65. Cohen G, Burks SR, Frank JA. Chlorotoxin-A multimodal imaging platform for targeting glioma tumors. Toxins. (2018) 10:E496. doi: 10.3390/toxins10120496
66. Qin C, He B, Dai W, Lin Z, Zhang H, Wang X, et al. The impact of a chlorotoxin-modified liposome system on receptor MMP-2 and the receptor-associated protein ClC-3. Biomaterials. (2014) 35:5908–20. doi: 10.1016/j.biomaterials.2014.03.077
67. Veiseh M, Gabikian P, Bahrami SB, Veiseh O, Zhang M, Hackman RC, et al. Tumor paint: a chlorotoxin:Cy5.5 bioconjugate for intraoperative visualization of cancer foci. Cancer Res. (2007) 67:6882–8. doi: 10.1158/0008-5472.CAN-06-3948
68. Staderini M, Megia-Fernandez A, Dhaliwal K, Bradley M. Peptides for optical medical imaging and steps towards therapy. Bioorg Med Chem. (2018) 26:2816–26. doi: 10.1016/j.bmc.2017.09.039
69. Zhao J, Wang YL, Li XB, Gao SY, Liu SY, Song YK, et al. Radiosynthesis and preliminary biological evaluation of 18F-fluoropropionyl-chlorotoxin as a potential PET tracer for glioma imaging. Contrast Media Mol Imag. (2018) 2018:8439162. doi: 10.1155/2018/8439162
70. Paemen L, Martens E, Masure S, Opdenakker G. Monoclonal antibodies specific for natural human neutrophil gelatinase B used for affinity purification, quantitation by two-site ELISA and inhibition of enzymatic activity. Eur J Biochem. (1995) 234:759–65. doi: 10.1111/j.1432-1033.1995.759_a.x
71. Martens E, Leyssen A, Van Aelst I, Fiten P, Piccard H, Hu J, et al. A monoclonal antibody inhibits gelatinase B/MMP-9 by selective binding to part of the catalytic domain and not to the fibronectin or zinc binding domains. Biochim Biophys Acta. (2007) 1770:178–86. doi: 10.1016/j.bbagen.2006.10.012
72. Pruijt JF, Fibbe WE, Laterveer L, Pieters RA, Lindley IJ, Paemen L, et al. Prevention of interleukin-8-induced mobilization of hematopoietic progenitor cells in rhesus monkeys by inhibitory antibodies against the metalloproteinase gelatinase B (MMP-9). Proc Natl Acad Sci USA. (1999) 96:10863–8. doi: 10.1073/pnas.96.19.10863
73. Hu J, Van den Steen PE, Houde M, Ilenchuk TT, Opdenakker G. Inhibitors of gelatinase B/matrix metalloproteinase-9 activity comparison of a peptidomimetic and polyhistidine with single-chain derivatives of a neutralizing monoclonal antibody. Biochem Pharmacol. (2004) 67:1001–9. doi: 10.1016/j.bcp.2003.10.030
74. Marshall DC, Lyman SK, McCauley S, Kovalenko M, Spangler R, Liu C, et al. Selective allosteric inhibition of MMP9 is efficacious in preclinical models of ulcerative colitis and colorectal cancer. PLoS ONE. (2015) 10:e0127063. doi: 10.1371/journal.pone.0127063
75. Juric V, O'Sullivan C, Stefanutti E, Kovalenko M, Greenstein A, Barry-Hamilton V, et al. MMP-9 inhibition promotes anti-tumor immunity through disruption of biochemical and physical barriers to T-cell trafficking to tumors. PLoS ONE. (2018) 13:e0207255. doi: 10.1371/journal.pone.0207255
76. Appleby TC, Greenstein AE, Hung M, Liclican A, Velasquez M, Villaseñor AG, et al. Biochemical characterization and structure determination of a potent, selective antibody inhibitor of human MMP9. J Biol Chem. (2017) 292:6810–20. doi: 10.1074/jbc.M116.760579
77. Sandborn WJ, Bhandari BR, Fogel R, Onken J, Yen E, Zhao X, et al. Randomised clinical trial: a phase 1, dose-ranging study of the anti-matrix metalloproteinase-9 monoclonal antibody GS-5745 versus placebo for ulcerative colitis. Aliment Pharmacol Ther. (2016) 44:157–69. doi: 10.1111/apt.13653
78. Sandborn WJ, Bhandari BR, Randall C, Younes ZH, Romanczyk T, Xin Y, et al. Andecaliximab [anti-matrix metalloproteinase-9] induction therapy for ulcerative colitis: a randomised, double-blind, placebo-controlled, phase 2/3 study in patients with moderate to severe disease. J Crohns Colitis. (2018) 12:1021–9. doi: 10.1093/ecco-jcc/jjy049
79. Gossage DL, Cieslarová B, Ap S, Zheng H, Xin Y, Lal P, et al. Phase 1b Study of the safety, pharmacokinetics, and disease-related outcomes of the matrix metalloproteinase-9 inhibitor andecaliximab in patients with rheumatoid arthritis. Clin. Ther. (2018) 40:156–65.e155. doi: 10.1016/j.clinthera.2017.11.011
80. Dufour A, Sampson NS, Li J, Kuscu C, Rizzo RC, DeLeon JL, et al. Small molecule anti-cancer compounds selectively target the hemopexin domain of matrix metalloproteinase-9 (MMP-9). Cancer Res. (2011) 71:4977–88. doi: 10.1158/0008-5472.CAN-10-4552
81. Scannevin RH, Alexander R, Haarlander TM, Burke SL, Singer M, Huo C, et al. Discovery of a highly selective chemical inhibitor of matrix metalloproteinase-9 (MMP-9) that allosterically inhibits zymogen activation. J Biol Chem. (2017) 292:17963–74. doi: 10.1074/jbc.M117.806075
82. Zucker S, Cao J. Selective matrix metalloproteinase (MMP) inhibitors in cancer therapy: ready for prime time? Cancer Biol Ther. (2009) 8:1–3. doi: 10.4161/cbt.8.24.10353
83. Sela-Passwell N, Rosenblum G, Shoham T, Sagi I. Structural and functional bases for allosteric control of MMP activities: can it pave the path for selective inhibition? Biochim Biophys Acta. (2010) 1803:29–38. doi: 10.1016/j.bbamcr.2009.04.010
84. Levin M, Udi Y, Solomonov I, Sagi I. Next generation matrix metalloproteinase inhibitors - Novel strategies bring new prospects. Biochim Biophys Acta. (2017) 1864:1927–39. doi: 10.1016/j.bbamcr.2017.06.009
85. Devy L, Huang L, Naa L, Yanamandra N, Pieters H, Frans N, et al. Selective inhibition of matrix metalloproteinase-14 blocks tumor growth, invasion, and angiogenesis. Cancer Res. (2009) 69:1517–26. doi: 10.1158/0008-5472.CAN-08-3255
86. Ager EI, Kozin SV, Kirkpatrick ND, Seano G, Kodack DP, Askoxylakis V, et al. Blockade of MMP14 activity in murine breast carcinomas: implications for macrophages, vessels, and radiotherapy. J Natl Cancer Inst. (2015)107:djv017. doi: 10.1093/jnci/djv017
87. Basu B, Correa de Sampaio P, Mohammed H, Fogarasi M, Corrie P, Watkins NA, et al. Inhibition of MT1-MMP activity using functional antibody fragments selected against its hemopexin domain. Int J Biochem Cell Biol. (2012) 44:393–403. doi: 10.1016/j.biocel.2011.11.015
88. Ingvarsen S, Porse A, Erpicum C, Maertens L, Jürgensen HJ, Madsen DH, et al. Targeting a single function of the multifunctional matrix metalloproteinase MT1-MMP: impact on lymphangiogenesis. J Biol Chem. (2013) 288:10195–204. doi: 10.1074/jbc.M112.447169
89. Shiryaev SA, Remacle AG, Golubkov VS, Ingvarsen S, Porse A, Behrendt N, et al. A monoclonal antibody interferes with TIMP-2 binding and incapacitates the MMP-2-activating function of multifunctional, pro-tumorigenic MMP-14/MT1-MMP. Oncogenesis. (2013) 2:e80. doi: 10.1038/oncsis.2013.44
90. Woskowicz AM, Weaver SA, Shitomi Y, Ito N, Itoh Y. MT-LOOP-dependent localization of membrane type I matrix metalloproteinase (MT1-MMP) to the cell adhesion complexes promotes cancer cell invasion. J Biol Chem. (2013) 288:35126–37. doi: 10.1074/jbc.M113.496067
91. Gálvez BG, Matías-Román S, Albar JP, Sánchez-Madrid F, Arroyo AG. Membrane type 1-matrix metalloproteinase is activated during migration of human endothelial cells and modulates endothelial motility and matrix remodeling. J Biol Chem. (2001) 276:37491–500. doi: 10.1074/jbc.M104094200
92. Udi Y, Grossman M, Solomonov I, Dym O, Rozenberg H, Moreno V, et al. Inhibition mechanism of membrane metalloprotease by an exosite-swiveling conformational antibody. Structure. (2015) 23:104–15. doi: 10.1016/j.str.2014.10.012
93. Talmi-Frank D, Altboum Z, Solomonov I, Udi Y, Jaitin DA, Klepfish M, et al. Extracellular matrix proteolysis by MT1-MMP contributes to influenza-related tissue damage and mortality. Cell Host Microbe. (2016) 20:458–70. doi: 10.1016/j.chom.2016.09.005
94. Botkjaer KA, Kwok HF, Terp MG, Karatt-Vellatt A, Santamaria S, McCafferty J, et al. Development of a specific affinity-matured exosite inhibitor to MT1-MMP that efficiently inhibits tumor cell invasion in vitro and metastasis in vivo. Oncotarget. (2016) 7:16773–92. doi: 10.18632/oncotarget.7780
95. Suojanen J, Salo T, Koivunen E, Sorsa T, Pirilä E. A novel and selective membrane type-1 matrix metalloproteinase (MT1-MMP) inhibitor reduces cancer cell motility and tumor growth. Cancer Biol Ther. (2009) 8:2362–70. doi: 10.4161/cbt.8.24.10139
96. Nam DH, Rodriguez C, Remacle AG, Strongin AY, Ge X. Active-site MMP-selective antibody inhibitors discovered from convex paratope synthetic libraries. Proc Natl Acad Sci USA. (2016) 113:14970–5. doi: 10.1073/pnas.1609375114
97. Ling B, Watt K, Banerjee S, Newsted D, Truesdell P, Adams J, et al. A novel immunotherapy targeting MMP-14 limits hypoxia, immune suppression and metastasis in triple-negative breast cancer models. Oncotarget. (2017) 8:58372–85. doi: 10.18632/oncotarget.17702
98. Nam DH, Fang K, Rodriguez C, Lopez T, Ge X. Generation of inhibitory monoclonal antibodies targeting matrix metalloproteinase-14 by motif grafting and CDR optimization. Protein Eng Des Sel. (2017) 30:113–8. doi: 10.1093/protein/gzw070
99. Remacle AG, Cieplak P, Nam DH, Shiryaev SA, Ge X, Strongin AY. Selective function-blocking monoclonal human antibody highlights the important role of membrane type-1 matrix metalloproteinase (MT1-MMP) in metastasis. Oncotarget. (2017) 8:2781–99. doi: 10.18632/oncotarget.13157
100. Remacle AG, Golubkov VS, Shiryaev SA, Dahl R, Stebbins JL, Chernov AV, et al. Novel MT1-MMP small-molecule inhibitors based on insights into hemopexin domain function in tumor growth. Cancer Res. (2012) 72:2339–49. doi: 10.1158/0008-5472.CAN-11-4149
101. Folkman J. Tumour Angiogenesis. In: Mendelsohn J, Howley PM, Israel MA, Liotta LA. The Molecular Basis of Cancer. Philadelphia, PA: WB Saunders (1995). p. 206–32.
102. Abdalla AME, Xiao L, Ullah MW, Yu M, Ouyang C, Yang G. Current challenges of cancer anti-angiogenic therapy and the promise of nanotherapeutics. Theranostics. (2018) 8:533–48. doi: 10.7150/thno.21674
103. Sounni NE, Paye A, Host L, Noël A. MT-MMPs as regulators of vessel stability associated with angiogenesis. Front Pharmacol. (2011) 2:111. doi: 10.3389/fphar.2011.00111
104. Yu J, Zhang Y, Leung LH, Liu L, Yang F, Yao X. Efficacy and safety of angiogenesis inhibitors in advanced gastric cancer: a systematic review and meta-analysis. J Hematol Oncol. (2016) 9:111. doi: 10.1186/s13045-016-0340-8
105. Kamba T, McDonald DM. Mechanisms of adverse effects of anti-VEGF therapy for cancer. Br J Cancer. (2007) 96:1788–95. doi: 10.1038/sj.bjc.6603813
106. Ebos JM, Lee CR, Kerbel RS. Tumor and host-mediated pathways of resistance and disease progression in response to antiangiogenic therapy. Clin Cancer Res. (2009) 15:5020–5. doi: 10.1158/1078-0432.CCR-09-0095
107. Kopetz S, Hoff PM, Morris JS, Wolff RA, Eng C, Glover KY, et al. Phase II trial of infusional fluorouracil, irinotecan, and bevacizumab for metastatic colorectal cancer: efficacy and circulating angiogenic biomarkers associated with therapeutic resistance. J Clin Oncol. (2010) 28:453–9. doi: 10.1200/JCO.2009.24.8252
108. Gyanchandani R, Ortega Alves MV, Myers JN, Kim S. A proangiogenic signature is revealed in FGF-mediated bevacizumab-resistant head and neck squamous cell carcinoma. Mol Cancer Res. (2013) 11:1585–96. doi: 10.1158/1541-7786.MCR-13-0358
109. Li J, Zhao X, Chen L, Guo H, Lv F, Jia K, et al. Safety and pharmacokinetics of novel selective vascular endothelial growth factor receptor-2 inhibitor YN968D1 in patients with advanced malignancies. BMC Cancer. (2010) 10:529. doi: 10.1186/1471-2407-10-529
110. Aplin AC, Zhu WH, Fogel E, Nicosia RF. Vascular regression and survival are differentially regulated by MT1-MMP and TIMPs in the aortic ring model of angiogenesis. Am J Physiol Cell Physiol. (2009) 297:C471–C480. doi: 10.1152/ajpcell.00019.2009
111. Onimaru M, Yonemitsu Y, Suzuki H, Fujii T, Sueishi K. An autocrine linkage between matrix metalloproteinase-14 and Tie-2 via ectodomain shedding modulates angiopoietin-1-dependent function in endothelial cells. Arterioscler Thromb Vasc Biol. (2010) 30:818–26. doi: 10.1161/ATVBAHA.109.201111
112. Saunders WB, Bohnsack BL, Faske JB, Anthis NJ, Bayless KJ, Hirschi KK, et al. Coregulation of vascular tube stabilization by endothelial cell TIMP-2 and pericyte TIMP-3. J Cell Biol. (2006) 175:179–91. doi: 10.1083/jcb.200603176
113. Hawinkels LJAC, Kuiper P, Wiercinska E, Verspaget HW, Liu Z, Pardali E, et al. Matrix metalloproteinase-14 (MT1-MMP)-mediated endoglin shedding inhibits tumor angiogenesis. Cancer Res. (2010) 70:4141–50. doi: 10.1158/0008-5472.CAN-09-4466
114. Yan L, Moses MA, Huang S, Ingber DE. Adhesion-dependent control of matrix metalloproteinase-2 activation in human capillary endothelial cells. J Cell Sci. (2000) 113:3979–87.
115. Miki T, Shamma A, Kitajima S, Takegami Y, Noda M, Nakashima Y, et al. The beta1-integrin-dependent function of RECK in physiologic and tumor angiogenesis. Mol Cancer Res. (2010) 8:665–76. doi: 10.1158/1541-7786.MCR-09-0351
116. Cornelius LA, Nehring LC, Harding E, Bolanowski M, Welgus HG, Kobayashi DK, et al. Matrix metalloproteinases generate angiostatin: effects on neovascularization. J Immunol. (1998) 161:6845–52.
117. O'Reilly MS, Wiederschain D, Stetler-Stevenson WG, Folkman J, Moses MA. Regulation of angiostatin production by matrix metalloproteinase-2 in a model of concomitant resistance. J Biol Chem. (1999) 274:29568–71. doi: 10.1074/jbc.274.41.29568
118. Heljasvaara R, Nyberg P, Luostarinen J, Parikka M, Heikkilä P, Rehn M, et al. Generation of biologically active endostatin fragments from human collagen XVIII by distinct matrix metalloproteases. Exp Cell Res. (2005) 307:292–304. doi: 10.1016/j.yexcr.2005.03.021
119. Bendrik C, Robertson J, Gauldie J, Dabrosin C. Gene transfer of matrix metalloproteinase-9 induces tumor regression of breast cancer in vivo. Cancer Res. (2008) 68:3405–12. doi: 10.1158/0008-5472.CAN-08-0295
120. Ghajar CM, George SC, Putnam AJ. Matrix metalloproteinase control of capillary morphogenesis. Crit Rev Eukaryot Gene Expr. (2008) 18:251–78. doi: 10.1615/CritRevEukarGeneExpr.v18.i3.30
121. Hamano Y, Zeisberg M, Sugimoto H, Lively JC, Maeshima Y, Yang C, et al. Physiological levels of tumstatin, a fragment of collagen IV alpha3 chain, are generated by MMP-9 proteolysis and suppress angiogenesis via alphaV beta3 integrin. Cancer Cell. (2003) 3:589–601. doi: 10.1016/S1535-6108(03)00133-8
122. Bramhall SR, Schulz J, Nemunaitis J, Brown PD, Baillet M, Buckels JA. A double-blind placebo-controlled, randomised study comparing gemcitabine and marimastat with gemcitabine and placebo as first line therapy in patients with advanced pancreatic cancer. Br J Cancer. (2002) 87:161–7. doi: 10.1038/sj.bjc.6600446
123. Winer A, Janosky M, Harrison B, Zhong J, Moussai D, Siyah P, et al. Inhibition of breast cancer metastasis by presurgical treatment with an oral matrix metalloproteinase inhibitor: a preclinical proof-of-principle study. Mol Cancer Ther. (2016) 15:2370–7. doi: 10.1158/1535-7163.MCT-16-0194
124. Grünwald B, Vandooren J, Gerg M, Ahomaa K, Hunger A, Berchtold S, et al. Systemic ablation of MMP-9 triggers invasive growth and metastasis of pancreatic cancer via Deregulation of IL6 expression in the bone marrow. Mol Cancer Res. (2016) 14:1147–58. doi: 10.1158/1541-7786.MCR-16-0180
Keywords: protease inhibitor, clinical trial, metalloproteinase, cancer, angiogenesis, antibody, exosite
Citation: Fields GB (2019) Mechanisms of Action of Novel Drugs Targeting Angiogenesis-Promoting Matrix Metalloproteinases. Front. Immunol. 10:1278. doi: 10.3389/fimmu.2019.01278
Received: 11 December 2018; Accepted: 20 May 2019;
Published: 04 June 2019.
Edited by:
Julia Kzhyshkowska, Universität Heidelberg, GermanyReviewed by:
Lasse Dahl Ejby Jensen, Linköping University, SwedenCopyright © 2019 Fields. This is an open-access article distributed under the terms of the Creative Commons Attribution License (CC BY). The use, distribution or reproduction in other forums is permitted, provided the original author(s) and the copyright owner(s) are credited and that the original publication in this journal is cited, in accordance with accepted academic practice. No use, distribution or reproduction is permitted which does not comply with these terms.
*Correspondence: Gregg B. Fields, ZmllbGRzZ0BmYXUuZWR1
Disclaimer: All claims expressed in this article are solely those of the authors and do not necessarily represent those of their affiliated organizations, or those of the publisher, the editors and the reviewers. Any product that may be evaluated in this article or claim that may be made by its manufacturer is not guaranteed or endorsed by the publisher.
Research integrity at Frontiers
Learn more about the work of our research integrity team to safeguard the quality of each article we publish.