- 1Department of Immunology, Faculty of Medicine, Kindai University, Osaka, Japan
- 2Department of Microbiology and Immunology, Emory University School of Medicine, Atlanta, GA, United States
Antigen-specific CD8+ tissue-resident memory T cells (TRM cells) persist in the lung following resolution of a respiratory virus infection and provide first-line defense against reinfection. In contrast to other memory T cell populations, such as central memory T cells that circulate between lymph and blood, and effector memory T cells (TEM cells) that circulate between blood and peripheral tissues, TRM cells are best defined by their permanent residency in the tissues and their independence from circulatory T cell populations. Consistent with this, we recently demonstrated that CD8+ TRM cells primarily reside within specific niches in the lung (Repair-Associated Memory Depots; RAMD) that normally exclude CD8+ TEM cells. However, it has also been reported that circulating CD8+ TEM cells continuously convert into CD8+ TRM cells in the lung interstitium, helping to sustain TRM numbers. The relative contributions of these two mechanisms of CD8+ TRM cells maintenance in the lung has been the source of vigorous debate. Here we propose a model in which the majority of CD8+ TRM cells are maintained within RAMD (conventional TRM) whereas a small fraction of TRM are derived from circulating CD8+ TEM cells and maintained in the interstitium. The numbers of both types of TRM cells wane over time due to declines in both RAMD availability and the overall number of TEM in the circulation. This model is consistent with most published reports and has important implications for the development of vaccines designed to elicit protective T cell memory in the lung.
Introduction
Memory CD8+ T cells in non-lymphoid tissues are optimally positioned to mediate rapid responses to invading pathogens. They comprise at least two distinct subpopulations: tissue-resident memory T cells (TRM cells) and effector memory T cells (TEM cells). TRM cells are a non-circulating population that typically, but not exclusively, expresses a specific array of surface markers (e.g., CD69, CD103, and CD49a) and possess gene-expression profiles that are associated with tissue retention (1). In contrast, TEM cells lack the expression of these molecules and continuously circulate between blood and non-lymphoid tissues (2). The vast majority of memory CD8+ T cells in most non-lymphoid tissues are TRM and play the predominant role in protective immunity (3, 4). In contrast, memory CD8+ T cells in the circulation have minimal, if any, impact on immediate local protection (3, 5). However, it is possible that the small numbers of CD8+ TEM cells that transit through the tissues at the time of reinfection may contribute to protection.
The lung appears to differ from other non-lymphoid tissues in that it harbors relatively large numbers of both tissue-circulating TEM and TRM cells in a number of distinct niches (3, 6). Furthermore, these memory CD8+ T cell subpopulations alter their phenotypes and functions in response to environmental factors present in distinct compartments of the lung (7, 8). Thus, a complete understanding of the phenotypic and functional features of these memory T cell populations in each of these lung compartment has been hampered by the challenges of isolating pure populations for analysis. This has resulted in confusion in the field. In this perspective, we attempt to resolve these issues and outline a model that explains the generation and maintenance of diverse populations of memory CD8+ T cells in the lung.
Memory CD8+ T Cells in the Lung
The tissues that comprise the barrier surfaces of the body typically consist of an epithelial layer that overlays a stromal layer, such as the epidermis and dermis in the skin and the epithelium and lamina propria in the intestine. These tissues differ considerably and provide distinct anatomical and biological niches for the maintenance of memory CD8+ T cells (9). Consistent with other barrier tissues, the lung airways (epithelium) and the lung interstitium (stroma) host phenotypically and functionally distinct memory CD8+ T cell populations.
Memory CD8+ T cells in the lung airways are localized primarily in the epithelial layers of the bronchiole and are readily isolated by bronchoalveolar lavage (BAL) (10–12). Since the lung airways are anatomically separated from blood vessels, there are few, if any, blood cell contaminants in BAL samples (unless the blood vessels are damaged by poor technique or infection). Consequently, it is possible to interpret the data on T cells isolated by BAL without using intravascular (i.v.) staining to distinguish contaminating cells from the blood (13). Such BAL data indicate that large numbers of antigen-specific memory CD8+ T cells are present in the lung airways for several months following recovery from a respiratory virus infection (14, 15). These airway T cells do not return to the circulation or the lung interstitium under steady-state conditions (12), suggesting that they are TRM. However, since they have relatively short lifespans (presumably due to cell-extrinsic factors, such as their biophysical removal by the barrier function of airway mucosa) (16), their maintenance depends on continual influx of memory cells from the interstitium (16, 17). This continual replenishment of memory pool does not fit with the definition of TRM and, as such, is a unique feature memory CD8+ T cells in the lung airways. Upon recruitment to the airways, the cells receive antigen-independent local environmental cues to acquire an activation phenotype (e.g., upregulation of CD69) and to completely downregulate the integrin LFA-1 (CD11a) (7, 16). As a result, memory CD8+ T cells in the airways lose cell contact-mediated cytolytic activity (11). Nevertheless, these cells can confer antigen-specific protection by rapidly secreting interferon (IFN)-γ in the face of antigenic challenge (18, 19).
Memory CD8+ T cells in the lung interstitium can be purified by enzymatic digestion of lung tissues after removal of the BAL. However, cells prepared this way are contaminated with small numbers of memory CD8+ T cells that had been trapped in the airways, although a certain fraction of these cells (i.e., cells that are exposed in the airway environment more than 48 h) can be distinguished by their reduced expression of CD11a (16). Interstitial cells prepared by enzymatic digestion are also contaminated with blood derived T cells from the capillaries. Therefore, prior i.v. staining is necessary to discriminate cells in the interstitium from those in the pulmonary capillary bed (13). It is important to point out two things here. First, data regarding parenchymal cells that have been isolated without i.v. staining must be cautiously interpreted given the significant degree of blood cell contamination. For example, before researchers began discriminating cells in the lung tissue and the lung vasculature, lung interstitium had erroneously been considered to be a “permissive tissue” that was readily accessible to memory CD8+ T cells in the circulation (20–22). However, a more detailed analysis has revealed that, as with other mucosal tissues, the migration of circulating memory CD8+ T cells into the lung interstitium is minimal in uninfected lung interstitium (6, 23, 24). Second, because memory CD8+ T cells in the lung interstitium (i.e., negative for i.v.-injected antibody) include both TRM and small numbers of tissue-circulating TEM, parabiosis approaches are necessary to distinguish these populations. Using these approaches, we and others have formally demonstrated that a large proportion of memory CD8+ T cells in the lung interstitium are TRM cells (Figure 1) (3, 6). It has also become evident that CD8+ TRM and TEM cell populations are maintained in distinct compartments of the lung interstitium: the former is predominantly localized within the site of tissue repair and regeneration around the bronchiole (we termed these Repair-Associated Memory Depots: RAMD), while the latter are widely and sparsely distributed in unaffected areas of the interstitium (6). Unlike memory CD8+ T cells in the airways, CD8+ TRM cells in the lung interstitium are a stable population (6). Hence, memory CD8+ T cells in the lung interstitium comprise a mixture of stable (TRM) and dynamic (TEM) memory populations that are maintained independently.
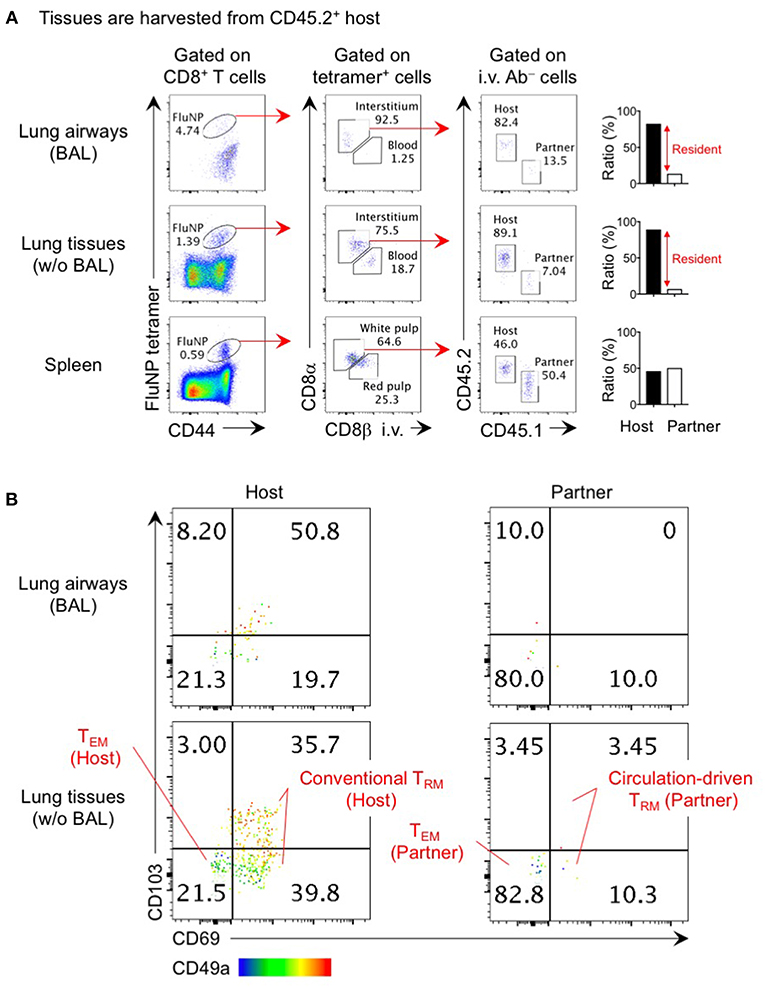
Figure 1. Analysis of lung TRM and TEM by parabiosis experiment. Congenically distinct mice (CD45.1+ and CD45.2+) were infected i.n. with influenza virus x31 (300 EID50) and subjected to parabiotic surgery 35 days later. Day 21 after the surgery, mice were injected i.v. with 1 μg anti-CD8β 3 min prior to tissue harvest. Cells in the lung airways were recovered by BAL. Lung tissues were digested by collagenase D, and enriched by centrifugation in 40/80% Percoll gradient. Cells were stained with influenza NP366−374/Db tetramer and fluorescent-conjugated antibodies. Data shown are derived from a CD45.2+ parabiont. Plots shown in (A) indicate the gating strategy of host- and partner-derived antigen-specific CD8+ T cells in the spleen, lung interstitium and airways. Bar graphs show ratio of host and partner cells among i.v. antibody negative cells in individual mouse. Plots shown in (B) indicate the expression of CD69, CD103, and CD49a on host- and partner-derived NP366−374/Db tetramer+ CD8+ T cells in the lung airways and interstitium. Host cells are the mixture of a large proportion of TRM (CD69+ CD49a+ CD103+ and CD69+ CD49a+ CD103−) and a minor population of TEM (CD69− CD49a− CD103−). The former population may include a small number of circulation-driven TRM converted from host TEM. The data also show how circulation-driven TRM cells are a relatively small population and are difficult to identify in individual animals.
The true phenotypes of memory T cells in the lung interstitium are best revealed through parabiosis studies in which a pair of influenza virus infected mice are surgically joined after memory has been established and rested until leucocytes in the blood of each mouse are equilibrated. Non-circulating host CD8+ T cells in the lung predominantly, but not exclusively, express TRM markers CD69, CD103, and CD49a that facilitate tissue-retention while partner-derived TEM cells are mostly negative for these markers (Figure 1) (6). The small fraction of host CD69− CD103− CD49a− cells likely represent the host-derived TEM population. It is interesting that a sizable fraction of host CD69+ CD49a+ cells in both the lung interstitium and airways lack the expression of another TRM marker, CD103 (Figure 1) (6, 25). The lack of CD103 on some TRM is consistent with subpopulation of TRM found in the intestinal lamina propria, brain, and liver (26–28). In this regard, i.n. infection of CD103 knockout mice with influenza virus resulted in partial, but not complete, loss of CD8+ TRM in these tissues (29). These data also indicate that the CD103 marker does not efficiently discriminate TRM from TEM in the lung. Given the diversity of memory CD8+ T cell populations in the lung, it is critical to precisely identify each population to avoid misinterpretation and confusion.
Generation and Maintenance of Canonical TRM Cells in the Lung
Following initial priming in the draining lymph nodes (LN), effector CD8+ T cells migrate to the inflamed tissues where they receive local instructive signals that promote their subsequent differentiation into TRM (9, 30). Transforming growth factor-β1 (TGF-β) is a common factor in most non-lymphoid tissues that drives T cell expression of CD103 and thereby promotes integrin αEβ7-mediated adhesion to E-cadherin on epithelial cells. A variety of cells, such as macrophages and stromal cells in the interstitium, and epithelial cells, are known to produce the latent form of TGF-β in the lung during early phases of an influenza virus infection (25, 31). As with the intestine (32–34), CD103+ dendritic cells (DC) in the lung interstitium may play a role in the local conversion of TGF-β into the active form through integrin αvβ8, and promote the establishment of CD103+ CD8+ TRM cells in the lung (35). In the absence of TGF-β signaling, CD8+ TRM cells in the whole lung (i.e., a mixture of cells in the airway and interstitium) completely lack the expression of CD103 (35, 36), although the number of antigen-specific CD8+ T cells in the whole lung is not affected (36). This suggests that the establishment of CD103− CD8+ TRM cells in the lung interstitium and airways is not dependent of TGF-β.
Since there is limited space for cells to inhabit in normal lung tissue, newly created anatomical niches are required for the establishment and long-term maintenance of CD8+ TRM cells in the lung (6, 9, 37). Upon respiratory virus infection, infection-induced cytolysis and disruption of infected cells by antigen-specific effector CD8+ T cells both contribute to tissue damage. A broad spectrum of cells including immune cells as well as basal cells (e.g., distal airway stem cells) accumulate at sites of damage to mediate the repair process which can be virtually observed as cytokeratin-expressing cell aggregates (Krt-pods) (38), thereby providing special niches for the establishment of CD8+ TRM cells in the lung interstitium (6, 37). Thus, lung CD8+ TRM cells may be specifically committed to protect weak spots (tissues undergoing repair) in the lung against reinfection (24). The structural characteristics of these TRM depots (RAMD) differ from inducible bronchus-associated lymphoid tissue (iBALT) as most CD8+ TRM cells in the RAMD do not form organized lymphoid structures (iBALT consists of CD4+ T cell cluster that surround B cell follicles) (6). This is consistent with the fact that unlike CD4+ T cells and B cells that act cooperatively, CD8+ TRM cells can act alone upon recall. Furthermore, our timed parabiosis approach (joining pairs of mice at various time points before and after infection) clearly demonstrated that CD8+ T cells recruited to the lung later than the peak of T cell response in the lung (around day 10 post influenza virus infection) failed to from TRM (6). This indicates that lung TRM niches are occupied at the peak of tissue damage and are no longer available for latecomer CD8+ T cells including TEM cells. It is well known that CD8+ TRM cells in the lung display relatively shorter longevity relative to TRM in other tissues as TRM cell-mediated heterosubtypic immunity to influenza virus lost at 4–6 months post-infection (5, 8). The decline in the size of the RAMDs overtime as tissue repair proceeds would explain the limited longevity of lung CD8+ TRM cells as compared to CD8+ TRM cells in other non-lymphoid tissues (6, 37). Similarly, the elevated proapoptotic activities of CD8+ TRM cells in the whole lung can be attributed to the concomitant loss of environmental factors that potentially support the homeostasis of TRM (8).
It has been established that concurrent CD4+ T cell responses also contribute to the establishment of CD8+ TRM cells in the lung (39). In contrast to other mucosa (female reproductive tract) where CD4+ T cells play an indirect role in promoting optimal positioning of CD8+ TRM cells by triggering the local production of inflammatory chemokines (40), CD4+ T cell help in the lung confers prolonged survival and improved functionality of CD8+ T cells by transcriptionally modulating the metabolism to maintain higher spare respiratory capacity (41), a hallmark of T cell memory (42). CD4+ T cell-derived IFN-γ also acts directly on CD8+ T cells to downregulate the expression of T-bet. This leads to memory CD8+ T cell rescue from T-bet-mediated repression of CD103, thereby promoting TRM formation (43). Given the differential distribution of CD8+ and CD4+ TRM (RAMD and iBALT, respectively), it seems likely that the primary involvement of CD4+ T cell help during CD8+ TRM formation is exerted during the acute phase of infection (41).
A recent study has shown that cell-intrinsic factors also contribute to the durability of TRM in the lung. CD8+ TRM cells generated from memory CD8+ T cells that had previously experienced multiple antigen encounters exhibit superior longevity compared to these generated from naïve CD8+ T cells (44). Reciprocal adoptive transfer approaches using a mixture of memory and naïve T cell receptor (TCR) transgenic T cells revealed that TRM cells derived from memory cells preferentially occupy lung TRM niches compared to TRM cells derived from naïve cells (44). This suggests that there may be increased frequencies of TRM precursors (KLRG1lo effector cells) among memory-derived CD8+ T cells, compared to naïve CD8+ T cells following activation in the draining LN. It is also possible that memory-derived CD8+ T cells may be capable of receiving additional instructive signals, such as 4-1BB signals for up-regulation of pro-survival factors, when cells are recruited to the RAMD and acquire resultant longevity (45).
Cognate antigen-driven local reactivation is also indispensable for the establishment of lung CD8+ TRM cells. The best example for this is the impact of route of infection/vaccination on the establishment of CD8+ TRM cells in the lung. Intranasal (i.n.) infection elicits robust populations of CD8+ TRM cells in the lung interstitium and airways, whereas non-pulmonary route of infection do not (5, 6, 19, 23, 24, 35, 46–49). In the case of the skin and genital tract, forced recruitment of circulating CD8+ T cells to the mucosa using inflammatory stimuli or topical administration of chemokines is sufficient to establish local TRM, an approach referred to as “prime and pull” (50, 51). However, we and others have shown that the exposure of CD8+ T cells to the lung environment is insufficient to promote subsequent differentiation of these into long-lived lung TRM (6, 23, 35). Instead, local reactivation induced by pulmonary administration of trace amount of antigen during the process of “prime and pull” is necessary for converting circulating CD8+ T cells into lung TRM cells (6, 23). Thus, both cell-intrinsic and extrinsic factors are necessary for complete conversion of these cells to TRM. First, pulmonary administration of antigen generates antigen-bearing target cells that are eliminated by antigen-specific CD8+ T cells, leading to the creation of damage and repair-associated TRM niches (6). Second, local reactivation provides cell-intrinsic effects such as prolonged expression of CD69 and CD49a necessary for retention (6, 23), and upregulation of interferon-induced transmembrane protein 3 (IFITM3) for survival (52). Furthermore, TCR signaling may protect TRM cells from a damage/danger-associated molecular pattern (DAMP)-induced cell death (53). Interestingly, there is differential expression of CD103 on distinct epitope-specific CD8+ TRM cells in the lung, irrespective of their localization, suggesting that difference in the extent of antigen presentation or subset of antigen presenting cells (APC) involved may also influence lung TRM biology (25).
While it is unclear which APC provide local antigen signaling, the delivery of antigen to pulmonary DC by antibody-targeted vaccination (conjugate of antigen and antibody specific for DC) significantly facilitates the establishment of CD103+ CD8+ TRM cells in the lung (35). Furthermore, CD103+ respiratory DC are known to continually carry residual antigen from the lung to the draining LN, suggesting that respiratory DC are the primary source of local antigen signaling (54). Given the unique ability of CD103+ respiratory DC to provide strong stimulatory signals in the draining LN, thereby generating effector CD8+ T cells that preferentially home back to the lung (55), local reactivation by respiratory DC may promote terminal effector maturation rather than memory differentiation (56–58). Thus, other APC subsets, such as pulmonary macrophages, that accumulate in the RAMD during the early phase of infection may be necessary to provide the optimal antigen signaling required for TRM development (59, 60).
Conversion From TEM to TRM: A Minor Pathway of TRM Development in the Lung
Despite the inefficiency of the non-pulmonary route of infection/immunization in establishing lung CD8+ TRM cells, several studies have nevertheless reported the deposition of CD8+ TRM cells in the lung following systemic infections (3, 61–63). Such blood-borne TRM are derived from effector cells that have undergone less differentiation (defined as null to intermediate expression of CX3CR1 and lack of KLRG1 expression and including exKLRG1 cells that have downregulated this molecule during the contraction phase) (64, 65). Adoptive transfer of splenic memory clearly revealed the emergence of a small fraction of CD103+ CD69+ CD8+ T cells in the whole lung (8). The appearance of CD8+ T cells exhibiting TRM phenotypes was also evident in our parabiosis experiments (Figure 1) (6), indicating that some levels of TEM to TRM conversion occurs in the lung. These cells exhibited a TRM gene-expression signature and their tissue-residency was also confirmed by parabiosis (3, 61). Since several cytokines, such as TGF-β, IL-33, and tumor necrosis factor (TNF)-α, are reported to drive TEM to TRM conversion (66), the formation of blood-borne CD8+ TRM cells in the lung likely depends on TNF, and its effect is prominent in previously infected lung tissues as compared to naive lung tissues (8). Because partner cells are also detected in the lung airways after parabiotic surgery (Figure 1) (6), circulating memory CD8+ T cells can reach to this tissue at basal levels, and CXCR3 plays a role in this recruitment (67). Treatment with pertussis toxin (PTx), which inhibits G protein-coupled chemokine receptors, significantly reduced the number of whole lung CD8+ TRM cells (including the dynamic population in the airways), suggesting that not only migration from the lung interstitium to the airway, but also the entrance of circulating CD8+ TEM cells into the lung depends on chemokine signaling (8). Despite their relatively low numbers, blood-borne lung CD8+ TRM cells confer some extent of protection against respiratory virus challenge (61–63). It should be emphasized, however, that this protection is far inferior to that mediated by bona fide lung CD8+ TRM cells generated by intranasal infection/immunization (5, 19, 23, 24, 48, 49). It is well known that the phenotype and function of memory CD8+ T cells in the circulation continues to change over time after infection, with central memory T cells (TCM cells) emerging as the predominant subset (64, 68–70). This leads to reduced numbers of memory CD8+ TEM that can be recruited to the lung and the eventual loss of a dynamic population of memory CD8+ T cells in the lung (8).
Future Perspective
In Figure 2, we suggest a model by which the diverse populations of memory CD8+ T cells are generated and maintained in the distinct compartments of the lung. Although the ontogeny of lung TRM and TEM differs, some levels of conversion from TEM to TRM occurs within the lung interstitium and also following recruitment to the airways. Furthermore, although lung airway memory CD8+ T cells are a non-circulating population, the maintenance of their numbers depends on the continual influx of new cells from the lung interstitium. Thus, precise discrimination of each population is critical for future studies to avoid confusion in the field (2). Based on the model, it is likely that the limited longevity of conventional lung CD8+ TRM cells and eventual loss of blood-borne lung CD8+ TRM cells both contribute the rapid decay of total CD8+ TRM cells in this tissue (Figure 2). In other words, such a short-lived nature of lung memory CD8+ T cells may, in a sense, be programed to avoid unnecessary pathogenesis in this tissue (71). Hence, multiple combinations of strategies to extend the longevity of both TRM and TEM should be considered for the development of vaccines against respiratory infectious pathogens. Since additional tissue damage is required to create new TRM niches, strategies that enable the effective establishment of TRM (including conversion from TEM to TRM) without the induction of undesirable pathogenesis should be considered in the future.
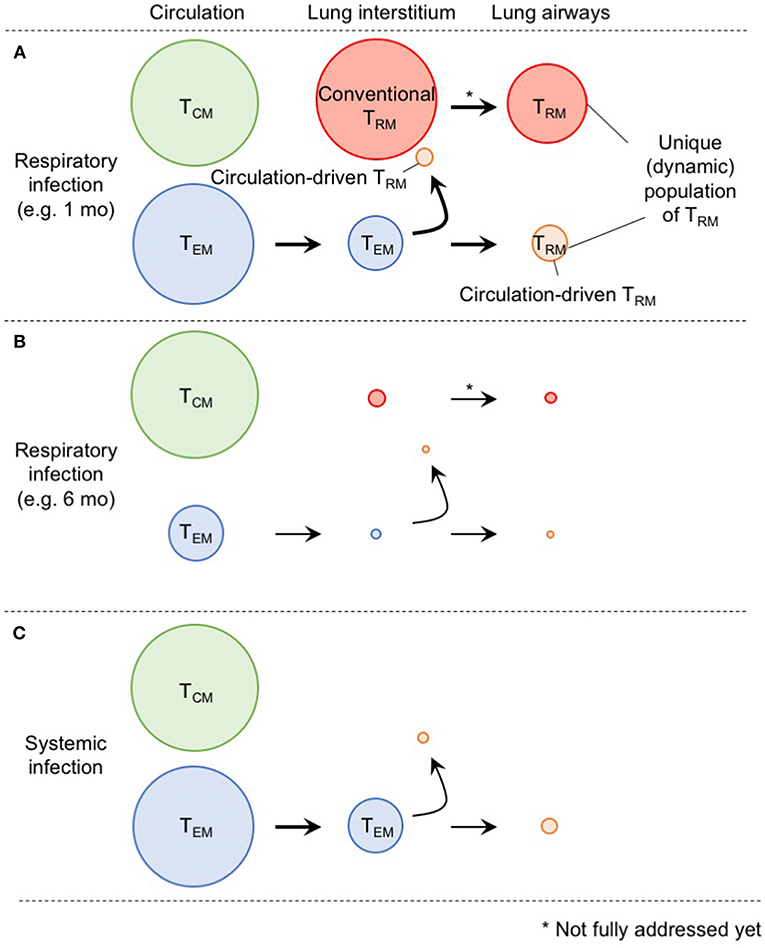
Figure 2. A comprehensive picture of memory CD8+ T cell populations in the lung. (A) Memory CD8+ T cells in the lung interstitium comprise a major population of conventional TRM and a smaller population of TEM. Some of the latter also give rise to TRM in response to TNF secreted in the conditioned lung that experience prior virus infection. Both host and partner cells in the interstitium are likely recruited to the lung airways and undergo phenotypic changes induced by environmental factors in this tissue. Although lung airway memory CD8+ T cells represent non-circulating population, and thus, are recognized as TRM, continual replacement is required for their maintenance. The size of the circles indicates the relative sizes of the respective populations in the lung. (B) As TEM cells in the circulation decrease overtime after infection, input of TEM to the lung interstitium and airways also decrease. Full recovery from the tissue damage, and resultant decrease of the size of RAMDs leads to reduction in the number of host CD8+ TRM cells in the lung interstitium and airways. Consequently, the animals lost CD8+ T cell-mediated protective immunity in the lung. (C) Because of the lack of local antigen, bona fide CD8+ TRM cells are not generated in the lung interstitium and airways. Although some TEM cells give rise to TRM in the lung, the extent is less than infection-experienced lung.
Ethics Statement
The studies utilizing laboratory animals were carried out in strict accordance with the Act on Welfare and Management of Animals of the government of Japan and the Regulations for the Care and Use of Laboratory Animals of Kinki University. The protocol for the present study was approved by the Institutional Animal Experimentation Committee of Kinki University Faculty of Medicine (Permit Number: KAME-26-025). All surgery was performed under anesthesia, and all efforts were made to minimize suffering.
Author Contributions
All authors listed have made a substantial, direct and intellectual contribution to the work, and approved it for publication.
Funding
This work is supported by Grant-in-Aid for Young Scientists (A) 24689043, and Grant-in-Aid for Scientific Research (C) 16K08850 from the Ministry of Education, Culture, Sports, Science and Technology of Japan, and grants from Takeda Science Foundation, Daiichi-Sankyo Foundation of Life Science, Uehara Memorial Foundation, Kanae Foundation for the Promotion of Medical Science, The Waksman Foundation of Japan, Kato Memorial Bioscience Foundation, Mochida Memorial Foundation for Medical and Pharmaceutical Research, Life Science Foundation of Japan, Japan Foundation for Pediatric Research, The Naito Foundation, and SENSHIN Medical Research Foundation (All to ST).
Conflict of Interest Statement
The authors declare that the research was conducted in the absence of any commercial or financial relationships that could be construed as a potential conflict of interest.
Acknowledgments
We thank Dr. David L. Woodland for editing the manuscript.
References
1. Mueller SN, Mackay LK. Tissue-resident memory T cells: local specialists in immune defence. Nat Rev Immunol. (2016) 16:79–89. doi: 10.1038/nri.2015.3
2. Jameson SC, Masopust D. Understanding subset diversity in T cell memory. Immunity. (2018) 48:214–26. doi: 10.1016/j.immuni.2018.02.010
3. Steinert EM, Schenkel JM, Fraser KA, Beura LK, Manlove LS, Igyarto BZ, et al. Quantifying memory CD8 T cells reveals regionalization of immunosurveillance. Cell. (2015) 161:737–49. doi: 10.1016/j.cell.2015.03.031
4. Thome JJ, Yudanin N, Ohmura Y, Kubota M, Grinshpun B, Sathaliyawala T, et al. Spatial map of human T cell compartmentalization and maintenance over decades of life. Cell. (2014) 159:814–28. doi: 10.1016/j.cell.2014.10.026
5. Wu T, Hu Y, Lee YT, Bouchard KR, Benechet A, Khanna K, et al. Lung-resident memory CD8 T cells (TRM) are indispensable for optimal cross-protection against pulmonary virus infection. J Leukoc Biol. (2014) 95:215–24. doi: 10.1189/jlb.0313180
6. Takamura S, Yagi H, Hakata Y, Motozono C, McMaster SR, Masumoto T, et al. Specific niches for lung-resident memory CD8+ T cells at the site of tissue regeneration enable CD69-independent maintenance. J Exp Med. (2016) 213:3057–73. doi: 10.1084/jem.20160938
7. Kohlmeier JE, Miller SC, Woodland DL. Cutting edge: antigen is not required for the activation and maintenance of virus-specific memory CD8+ T cells in the lung airways. J Immunol. (2007) 178:4721–5. doi: 10.4049/jimmunol.178.8.4721
8. Slütter B, Van Braeckel-Budimir N, Abboud G, Varga SM, Salek-Ardakani S, Harty JT. Dynamics of influenza-induced lung-resident memory T cells underlie waning heterosubtypic immunity. Sci Immunol. (2017) 2:eaag2031. doi: 10.1126/sciimmunol.aag2031
9. Takamura S. Niches for the long-term maintenance of tissue-resident memory T cells. Front Immunol. (2018) 9:1214. doi: 10.3389/fimmu.2018.01214
10. Ely KH, Roberts AD, Woodland DL. Cutting edge: effector memory CD8+ T cells in the lung airways retain the potential to mediate recall responses. J Immunol. (2003) 171:3338–42. doi: 10.4049/jimmunol.171.7.3338
11. Hogan RJ, Usherwood EJ, Zhong W, Roberts AA, Dutton RW, Harmsen AG, et al. Activated antigen-specific CD8+ T cells persist in the lungs following recovery from respiratory virus infections. J Immunol. (2001) 166:1813–22. doi: 10.4049/jimmunol.166.3.1813
12. Hogan RJ, Cauley LS, Ely KH, Cookenham T, Roberts AD, Brennan JW, et al. Long-term maintenance of virus-specific effector memory CD8+ T cells in the lung airways depends on proliferation. J Immunol. (2002) 169:4976–81. doi: 10.4049/jimmunol.169.9.4976
13. Anderson KG, Sung H, Skon CN, Lefrancois L, Deisinger A, Vezys V, et al. Cutting edge: intravascular staining redefines lung CD8 T cell responses. J Immunol. (2012) 189:2702–6. doi: 10.4049/jimmunol.1201682
14. Woodland DL, Kohlmeier JE. Migration, maintenance and recall of memory T cells in peripheral tissues. Nat Rev Immunol. (2009) 9:153–61. doi: 10.1038/nri2496
15. Hikono H, Kohlmeier JE, Ely KH, Scott I, Roberts AD, Blackman MA, et al. T-cell memory and recall responses to respiratory virus infections. Immunol Rev. (2006) 211:119–32. doi: 10.1111/j.0105-2896.2006.00385.x
16. Ely KH, Cookenham T, Roberts AD, Woodland DL. Memory T cell populations in the lung airways are maintained by continual recruitment. J Immunol. (2006) 176:537–43. doi: 10.4049/jimmunol.176.1.537
17. Zammit DJ, Turner DL, Klonowski KD, Lefrancois L, Cauley LS. Residual antigen presentation after influenza virus infection affects CD8 T cell activation and migration. Immunity. (2006) 24:439–49. doi: 10.1016/j.immuni.2006.01.015
18. McMaster SR, Wilson JJ, Wang H, Kohlmeier JE. Airway-resident memory CD8 T cells provide antigen-specific protection against respiratory virus challenge through rapid IFN-gamma production. J Immunol. (2015) 195:203–9. doi: 10.4049/jimmunol.1402975
19. Kinnear E, Lambert L, McDonald JU, Cheeseman HM, Caproni LJ, Tregoning JS. Airway T cells protect against RSV infection in the absence of antibody. Mucosal Immunol. (2018) 11:249–56. doi: 10.1038/mi.2017.46
20. Masopust D, Vezys V, Usherwood EJ, Cauley LS, Olson S, Marzo AL, et al. Activated primary and memory CD8 T cells migrate to nonlymphoid tissues regardless of site of activation or tissue of origin. J Immunol. (2004) 172:4875–82. doi: 10.4049/jimmunol.172.8.4875
21. Klonowski KD, Williams KJ, Marzo AL, Blair DA, Lingenheld EG, Lefrancois L. Dynamics of blood-borne CD8 memory T cell migration in vivo. Immunity. (2004) 20:551–62. doi: 10.1016/S1074-7613(04)00103-7
22. Shin H, Iwasaki A. Tissue-resident memory T cells. Immunol Rev. (2013) 255:165–81. doi: 10.1111/imr.12087
23. McMaster SR, Wein AN, Dunbar PR, Hayward SL, Cartwright EK, Denning TL, et al. Pulmonary antigen encounter regulates the establishment of tissue-resident CD8 memory T cells in the lung airways and parenchyma. Mucosal Immunol. (2018) 11:1071–8. doi: 10.1038/s41385-018-0003-x
24. Gilchuk P, Hill TM, Guy C, McMaster SR, Boyd KL, Rabacal WA, et al. A distinct lung-interstitium-resident memory CD8 + T cell subset confers enhanced protection to lower respiratory tract infection. Cell Rep. (2016) 16:1800–9. doi: 10.1016/j.celrep.2016.07.037
25. Yoshizawa A, Bi K, Keskin DB, Zhang G, Reinhold B, Reinherz EL. TCR-pMHC encounter differentially regulates transcriptomes of tissue-resident CD8 T cells. Eur J Immunol. (2018) 48:128–50. doi: 10.1002/eji.201747174
26. Bergsbaken T, Bevan MJ. Proinflammatory microenvironments within the intestine regulate the differentiation of tissue-resident CD8(+) T cells responding to infection. Nat Immunol. (2015) 16:406–14. doi: 10.1038/ni.3108
27. Wakim LM, Woodward-Davis A, Bevan MJ. Memory T cells persisting within the brain after local infection show functional adaptations to their tissue of residence. Proc Natl Acad Sci USA. (2010) 107:17872–9. doi: 10.1073/pnas.1010201107
28. Fernandez-Ruiz D, Ng WY, Holz LE, Ma JZ, Zaid A, Wong YC, et al. Liver-resident memory CD8(+) T cells form a front-line defense against malaria liver-stage infection. Immunity. (2016) 45:889–902. doi: 10.1016/j.immuni.2016.08.011
29. Lee YT, Suarez-Ramirez JE, Wu T, Redman JM, Bouchard K, Hadley GA, et al. Environmental and antigen receptor-derived signals support sustained surveillance of the lungs by pathogen-specific cytotoxic T lymphocytes. J Virol. (2011) 85:4085–94. doi: 10.1128/JVI.02493-10
30. Iijima N, Iwasaki A. Tissue instruction for migration and retention of TRM cells. Trends Immunol. (2015) 36:556–64. doi: 10.1016/j.it.2015.07.002
31. Denney L, Branchett W, Gregory LG, Oliver RA, Lloyd CM. Epithelial-derived TGF-beta1 acts as a pro-viral factor in the lung during influenza A infection. Mucosal Immunol. (2018) 11:523–35. doi: 10.1038/mi.2017.77
32. Travis MA, Reizis B, Melton AC, Masteller E, Tang Q, Proctor JM, et al. Loss of integrin alpha(v)beta8 on dendritic cells causes autoimmunity and colitis in mice. Nature. (2007) 449:361–5. doi: 10.1038/nature06110
33. Luda KM, Joeris T, Persson EK, Rivollier A, Demiri M, Sitnik KM, et al. IRF8 transcription-factor-dependent classical dendritic cells are essential for intestinal T cell homeostasis. Immunity. (2016) 44:860–74. doi: 10.1016/j.immuni.2016.02.008
34. Boucard-Jourdin M, Kugler D, Endale Ahanda ML, This S, De Calisto J, Zhang A, et al. beta8 integrin expression and activation of TGF-beta by intestinal dendritic cells are determined by both tissue microenvironment and cell lineage. J Immunol. (2016) 197:1968–78. doi: 10.4049/jimmunol.1600244
35. Wakim LM, Smith J, Caminschi I, Lahoud MH, Villadangos JA. Antibody-targeted vaccination to lung dendritic cells generates tissue-resident memory CD8 T cells that are highly protective against influenza virus infection. Mucosal Immunol. (2015) 8:1060–71. doi: 10.1038/mi.2014.133
36. Hu Y, Lee YT, Kaech SM, Garvy B, Cauley LS. Smad4 promotes differentiation of effector and circulating memory CD8 T cells but is dispensable for tissue-resident memory CD8 T cells. J Immunol. (2015) 194:2407–14. doi: 10.4049/jimmunol.1402369
37. Takamura S. Persistence in temporary lung niches: a survival strategy of lung-resident memory CD8(+) T cells. Viral Immunol. (2017) 30:438–50. doi: 10.1089/vim.2017.0016
38. Kumar PA, Hu Y, Yamamoto Y, Hoe NB, Wei TS, Mu D, et al. Distal airway stem cells yield alveoli in vitro and during lung regeneration following H1N1 influenza infection. Cell. (2011) 147:525–38. doi: 10.1016/j.cell.2011.10.001
39. Belz GT, Wodarz D, Diaz G, Nowak MA, Doherty PC. Compromised influenza virus-specific CD8(+)-T-cell memory in CD4(+)-T-cell-deficient mice. J Virol. (2002) 76:12388–93. doi: 10.1128/JVI.76.23.12388-12393.2002
40. Nakanishi Y, Lu B, Gerard C, Iwasaki A. CD8(+) T lymphocyte mobilization to virus-infected tissue requires CD4(+) T-cell help. Nature. (2009) 462:510–3. doi: 10.1038/nature08511
41. Cullen JG, McQuilten HA, Quinn KM, Olshansky M, Russ BE, Morey A, et al. CD4(+) T help promotes influenza virus-specific CD8(+) T cell memory by limiting metabolic dysfunction. Proc Natl Acad Sci USA. (2019) 116:4481–8. doi: 10.1073/pnas.1808849116
42. van der Windt GJ, Everts B, Chang CH, Curtis JD, Freitas TC, Amiel E, et al. Mitochondrial respiratory capacity is a critical regulator of CD8+ T cell memory development. Immunity. (2012) 36:68–78. doi: 10.1016/j.immuni.2011.12.007
43. Laidlaw BJ, Zhang N, Marshall HD, Staron MM, Guan T, Hu Y, et al. CD4+ T cell help guides formation of CD103+ lung-resident memory CD8+ T cells during influenza viral infection. Immunity. (2014) 41:633–45. doi: 10.1016/j.immuni.2014.09.007
44. Van Braeckel-Budimir N, Varga SM, Badovinac VP, Harty JT. Repeated antigen exposure extends the durability of influenza-specific lung-resident memory CD8(+) T cells and heterosubtypic immunity. Cell Rep. (2018) 24:3374–82 e3. doi: 10.1016/j.celrep.2018.08.073
45. Zhou AC, Wagar LE, Wortzman ME, Watts TH. Intrinsic 4–1BB signals are indispensable for the establishment of an influenza-specific tissue-resident memory CD8 T-cell population in the lung. Mucosal Immunol. (2017) 10:1294–309. doi: 10.1038/mi.2016.124
46. Takamura S, Roberts AD, Jelley-Gibbs DM, Wittmer ST, Kohlmeier JE, Woodland DL. The route of priming influences the ability of respiratory virus-specific memory CD8+ T cells to be activated by residual antigen. J Exp Med. (2010) 207:1153–60. doi: 10.1084/jem.20090283
47. Nizard M, Roussel H, Diniz MO, Karaki S, Tran T, Voron T, et al. Induction of resident memory T cells enhances the efficacy of cancer vaccine. Nat Commun. (2017) 8:15221. doi: 10.1038/ncomms15221
48. Morabito KM, Ruckwardt TR, Redwood AJ, Moin SM, Price DA, Graham BS. Intranasal administration of RSV antigen-expressing MCMV elicits robust tissue-resident effector and effector memory CD8+ T cells in the lung. Mucosal Immunol. (2017) 10:545–54. doi: 10.1038/mi.2016.48
49. Zens KD, Chen JK, Farber DL. Vaccine-generated lung tissue-resident memory T cells provide heterosubtypic protection to influenza infection. JCI Insight. (2016) 1:85832. doi: 10.1172/jci.insight.85832
50. Shin H, Iwasaki AA vaccine strategy that protects against genital herpes by establishing local memory T cells. Nature. (2012) 491:463–7. doi: 10.1038/nature11522
51. Mackay LK, Stock AT, Ma JZ, Jones CM, Kent SJ, Mueller SN, et al. Long-lived epithelial immunity by tissue-resident memory T (TRM) cells in the absence of persisting local antigen presentation. Proc Natl Acad Sci USA. (2012) 109:7037–42. doi: 10.1073/pnas.1202288109
52. Wakim LM, Gupta N, Mintern JD, Villadangos JA. Enhanced survival of lung tissue-resident memory CD8(+) T cells during infection with influenza virus due to selective expression of IFITM3. Nat Immunol. (2013) 14:238–45. doi: 10.1038/ni.2525
53. Stark R, Wesselink TH, Behr FM, Kragten NAM, Arens R, Koch-Nolte F, et al. T RM maintenance is regulated by tissue damage via P2RX7. Sci Immunol. (2018) 3:eaau1022. doi: 10.1126/sciimmunol.aau1022
54. Kim TS, Hufford MM, Sun J, Fu YX, Braciale TJ. Antigen persistence and the control of local T cell memory by migrant respiratory dendritic cells after acute virus infection. J Exp Med. (2010) 207:1161–72. doi: 10.1084/jem.20092017
55. Kim TS, Gorski SA, Hahn S, Murphy KM, Braciale TJ. Distinct dendritic cell subsets dictate the fate decision between effector and memory CD8(+) T cell differentiation by a CD24-dependent mechanism. Immunity. (2014) 40:400–13. doi: 10.1016/j.immuni.2014.02.004
56. McGill J, Van Rooijen N, Legge KL. Protective influenza-specific CD8 T cell responses require interactions with dendritic cells in the lungs. J Exp Med. (2008) 205:1635–46. doi: 10.1084/jem.20080314
57. McGill J, Van Rooijen N, Legge KL. IL-15 trans-presentation by pulmonary dendritic cells promotes effector CD8 T cell survival during influenza virus infection. J Exp Med. (2010) 207:521–34. doi: 10.1084/jem.20091711
58. McGill J, Legge KL. Cutting edge: contribution of lung-resident T cell proliferation to the overall magnitude of the antigen-specific CD8 T cell response in the lungs following murine influenza virus infection. J Immunol. (2009) 183:4177–81. doi: 10.4049/jimmunol.0901109
59. Desai P, Tahiliani V, Stanfield J, Abboud G, Salek-Ardakani S. Inflammatory monocytes contribute to the persistence of CXCR3(hi) CX3CR1(lo) circulating and lung-resident memory CD8(+) T cells following respiratory virus infection. Immunol Cell Biol. (2018) 96:370–8. doi: 10.1111/imcb.12006
60. Chu KL, Batista NV, Wang KC, Zhou AC, Watts TH. GITRL on inflammatory antigen presenting cells in the lung parenchyma provides signal 4 for T-cell accumulation and tissue-resident memory T-cell formation. Mucosal Immunol. (2019) 12:363–77. doi: 10.1038/s41385-018-0105-5
61. Kadoki M, Patil A, Thaiss CC, Brooks DJ, Pandey S, Deep D, et al. Organism-level analysis of vaccination reveals networks of protection across tissues. Cell. (2017) 171:398–413 e21. doi: 10.1016/j.cell.2017.08.024
62. Pan Y, Tian T, Park CO, Lofftus SY, Mei S, Liu X, et al. Survival of tissue-resident memory T cells requires exogenous lipid uptake and metabolism. Nature. (2017) 543:252–6. doi: 10.1038/nature21379
63. Liu L, Zhong Q, Tian T, Dubin K, Athale SK, Kupper TS. Epidermal injury and infection during poxvirus immunization is crucial for the generation of highly protective T cell-mediated immunity. Nat Med. (2010) 16:224–7. doi: 10.1038/nm.2078
64. Gerlach C, Moseman EA, Loughhead SM, Alvarez D, Zwijnenburg AJ, Waanders L, et al. The chemokine receptor CX3CR1 defines three antigen-experienced CD8 T cell subsets with distinct roles in immune surveillance and homeostasis. Immunity. (2016) 45:1270–84. doi: 10.1016/j.immuni.2016.10.018
65. Herndler-Brandstetter D, Ishigame H, Shinnakasu R, Plajer V, Stecher C, Zhao J, et al. KLRG1(+) effector CD8(+) T cells lose KLRG1, differentiate into all memory T cell lineages, and convey enhanced protective immunity. Immunity. (2018) 48:716–29 e8. doi: 10.1016/j.immuni.2018.03.015
66. Skon CN, Lee JY, Anderson KG, Masopust D, Hogquist KA, Jameson SC. Transcriptional downregulation of S1pr1 is required for the establishment of resident memory CD8+ T cells. Nat Immunol. (2013) 14:1285–93. doi: 10.1038/ni.2745
67. Slütter B, Pewe LL, Kaech SM, Harty JT. Lung airway-surveilling CXCR3(hi) memory CD8(+) T cells are critical for protection against influenza A virus. Immunity. (2013) 39:939–48. doi: 10.1016/j.immuni.2013.09.013
68. Wherry EJ, Teichgraber V, Becker TC, Masopust D, Kaech SM, Antia R, et al. Lineage relationship and protective immunity of memory CD8 T cell subsets. Nat Immunol. (2003) 4:225–34. doi: 10.1038/ni889
69. Martin MD, Kim MT, Shan Q, Sompallae R, Xue HH, Harty JT, et al. Phenotypic and functional alterations in circulating memory CD8 T cells with time after primary infection. PLoS Pathog. (2015) 11:e1005219. doi: 10.1371/journal.ppat.1005219
70. Hikono H, Kohlmeier JE, Takamura S, Wittmer ST, Roberts AD, Woodland DL. Activation phenotype, rather than central- or effector-memory phenotype, predicts the recall efficacy of memory CD8+ T cells. J Exp Med. (2007) 204:1625–36. doi: 10.1084/jem.20070322
Keywords: tissue-resident memory, CD8+ T cells, memory T cell maintenance, lung, respiratory virus infections
Citation: Takamura S and Kohlmeier JE (2019) Establishment and Maintenance of Conventional and Circulation-Driven Lung-Resident Memory CD8+ T Cells Following Respiratory Virus Infections. Front. Immunol. 10:733. doi: 10.3389/fimmu.2019.00733
Received: 15 January 2019; Accepted: 19 March 2019;
Published: 05 April 2019.
Edited by:
Michael Vajdy, EpitoGenesis, United StatesReviewed by:
Jason Kyle Whitmire, University of North Carolina at Chapel Hill, United StatesBrian S. Sheridan, Stony Brook University, United States
Copyright © 2019 Takamura and Kohlmeier. This is an open-access article distributed under the terms of the Creative Commons Attribution License (CC BY). The use, distribution or reproduction in other forums is permitted, provided the original author(s) and the copyright owner(s) are credited and that the original publication in this journal is cited, in accordance with accepted academic practice. No use, distribution or reproduction is permitted which does not comply with these terms.
*Correspondence: Shiki Takamura, dGFrYW11cmFAbWVkLmtpbmRhaS5hYy5qcA==