- 1Wake Forest Institute for Regenerative Medicine, Winston-Salem, NC, United States
- 2Wake Forest Center for Integrative Medicine, Wake Forest School of Medicine, Winston-Salem, NC, United States
- 3Department of Rheumatology and Immunology, Wake Forest School of Medicine, Winston-Salem, NC, United States
Rheumatoid arthritis (RA) is a systemic autoimmune disease characterized by chronic inflammation of synovium (synovitis), with inflammatory/immune cells and resident fibroblast-like synoviocytes (FLS) acting as major players in the pathogenesis of this disease. The resulting inflammatory response poses considerable risks as loss of bone and cartilage progresses, destroying the joint surface, causing joint damage, joint failure, articular dysfunction, and pre-mature death if left untreated. At the cellular level, early changes in RA synovium include inflammatory cell infiltration, synovial hyperplasia, and stimulation of angiogenesis to the site of injury. Different angiogenic factors promote this disease, making the role of anti-angiogenic therapy a focus of RA treatment. To control angiogenesis, mesenchymal stromal cells/pericytes (MSCs) in synovial tissue play a vital role in tissue repair. While recent evidence reports that MSCs found in joint tissues can differentiate to repair damaged tissue, this repair function can be repressed by the inflammatory milieu. Extremely-low frequency pulsed electromagnetic field (PEMF), a biophysical form of stimulation, has an anti-inflammatory effect by causing differentiation of MSCs. PEMF has also been reported to increase the functional activity of MSCs to improve differentiation to chondrocytes and osteocytes. Moreover, PEMF has been demonstrated to accelerate cell differentiation, increase deposition of collagen, and potentially return vascular dysfunction back to homeostasis. The aim of this report is to review the effects of PEMF on MSC modulation of cytokines, growth factors, and angiogenesis, and describe its effect on MSC regeneration of synovial tissue to further understand its potential role in the treatment of RA.
Introduction
Rheumatoid arthritis (RA) is a systemic autoimmune disease affecting over 1.3 million Americans, and as much as 1% of the population worldwide (1). Although RA predominantly affects large and small joints, it can affect other organs in the body, including those of the cardiovascular, pulmonary, and ophthalmologic systems (2). The pathophysiology of RA includes abnormal activation of blood cells, namely macrophages, T-cells, and B-cells, which produce pro-inflammatory mediators (e.g., cytokines and growth factors) that initiate an inflammatory cascade that leads to joint damage (i.e., bone erosions) and systemic complications (3). Current treatments include corticosteroids, traditional disease-modifying anti-rheumatic drugs (DMARDs), and anti-cytokines (biologics); however, these drugs have adverse effects which can be severe, including osteoporosis, alterations of metabolism, infection, bone marrow suppression, hepatitis, and an increased risk of malignancies (4–6). As the disease progresses, joints are damaged resulting in impaired range of motion, joint deformity, and dysfunction (7). Although the currently approved drugs are known to prevent further joint damage, the effect of these drugs in repairing bone erosions has yet to be demonstrated, and pro-anabolic agents are needed to promote bone formation at the erosion sites (8). Therefore, innovative and safe strategies aimed at both reducing inflammation and promoting tissue regeneration are urgently needed to inhibit the progression of RA.
A promising novel strategy for the treatment of RA is the local or systemic delivery of extremely low frequency pulsed electromagnetic fields (PEMF) to target mesenchymal stromal cells/pericytes (MSCs) to improve their ability to modulate immune responses and repair tissue. PEMF are physical stimuli that affect biological systems through the production of coherent or interfering fields that modify fundamental electromagnetic frequencies generated by living organisms (9, 10). PEMF activate multiple intracellular pathways, including numerous processes and biochemical mechanisms within both the immune and microvascular systems. There are two methods in which PEMF can be applied to biological tissues: capacitive or inductive coupling. In direct capacitive coupling, an electrode must be placed on the tissue (11); however, in non-direct capacitive coupling/inductive coupling, electrodes do not have to be in direct contact with the tissue because the electric field produces a magnetic field that, in turn, produces a current in the conductive tissues of the body (11–13). PEMF therapy is based on Faraday's law, a basic law of electromagnetism that predicts how a magnetic field will interact with an electric circuit to produce an electromotive force known as electromagnetic induction. This law dictates the more charge that is needed, the higher the intensity of the PEMF signal needs to be. This is represented by the equation dB/dT, where B is peak magnetic intensity, T is time, and d is the derivative (or change) in these units. Since the PEMF signal needs to be able to pass deep enough through the tissue to produce healing results, field intensity, frequency, and time of exposure are all important components in the dosimetry. PEMF follows the inverse square law, so it drops off exponentially from the distance of the surface of the coil; therefore, the closest tissue to the coil (applicator) gets the maximum intensity, and furthest tissue from the coil gets the least intensity.
PEMF can alter cell function by triggering the forced vibration of free ions on the surface of the plasma membrane, causing external oscillating field disruptions in the electrochemical balance of transmembrane proteins (ion channels) (9, 14). It has been suggested that PEMF may be propagated and effectively amplified along the entire signal transduction pathway, thereby modifying cell behavior (15–17). Indeed, several studies have reported that PEMF can modulate both cell surface receptor expression/activation, and downstream signal transduction pathways, thereby restoring homeostatic cell functions such as viability, proliferation, differentiation, communication with neighboring cells, and interaction with components of the extracellular matrix (ECM) (18–23).
By modulating the expression of various signaling cascades and cellular information processing networks to potentially restore them to homeostatic (healthy) production levels, PEMF is showing promise as a treatment for autoimmune diseases such as RA (24–27). Changes in the cells' microenvironment are integrated into a survival response by complex signal transduction mechanisms (28). Lipid nanopores forming stable, ion channel conduction pathways in the plasma membrane of cells (29), explain the conduction of ions into the cell from the extracellular space, specifically calcium (Ca2+) ion flux (17, 30, 31). It has been postulated that a direct effect of PEMF on phospholipids within the plasma membrane stimulates the production of second messengers, initiating multiple intracellular signal transduction pathways (32–34).
PEMF intensity is dependent upon wave amplitude/field strength measured in units of Tesla (T), or Gauss (10,000 T). In order to deliver a therapeutic PEMF, it is necessary to optimize three important parameters: frequency, intensity, and duration/time of exposure (9). Previous studies have conclusively shown that optimization of the frequency, intensity, and time of exposure is helpful in attaining consistent beneficial results in experimental arthritis in rats (35–37). A 5 Hz frequency, 4 microT (μT) intensity, applied for 90 min to the rat paw was reported to be the optimal dosimetry for lowering edema, and reducing swelling, inflammatory cell infiltration, hyperplasia, and hypertrophy of cells lining the synovial membrane (37). Preliminary studies in humans have also reported that PEMF can reduce chronic joint swelling and pain in patients with RA (25). Further, the beneficial effects of PEMF have been reported to last up to 3 months or longer in human patients with chronic inflammatory/autoimmune disorders (38) with no evidence of adverse effects (39).
PEMF Modulates RA Tissue Pathogenesis via Modulation of MSCs and FLS
Normal synovium composition consists of a well-organized matrix of fibroblast-like cells (FLS) and macrophage-like cells known as synovial cells or synoviocytes. The joint-lining synovial membrane consists of a layer of macrophage-like (type a) synoviocytes, fibroblast-like synoviocytes (FLS–type b), and mesenchymal stromal cells (MSCs) (40). In RA, the synovium becomes infiltrated by cells of lympho-hematopoietic origin, namely T-helper cells, B cells, and macrophages, which cause synovial hyperplasia and neoangiogenesis (7, 41, 42). The resulting inflammatory response poses considerable risks for joint damage, and articular dysfunction if left untreated (43). Type A synoviocytes are CD163+, CD68+, CD14+/lo cells that localize to the intima and the subintimal layers of the synovial membrane and proliferate in response to inflammatory conditions. Under pathological conditions, Type A (macrophage-like) synoviocytes contribute to cartilage destruction by producing pro-inflammatory cytokines. They originate in the bone marrow, like other mononuclear phagocytes, and are constantly replaced via the circulation. In rheumatoid synovium sections, 80–100% of the synovial lining cells are macrophage-like cells functioning as antigen processing- and antigen-presenting cells to T lymphocytes (44). Type A synoviocytes also induce the formation of osteophytes through the release of transforming growth factor-beta (TGF-β) 3 and bone morphogenetic proteins (BMP)-2 and BMP-4 (45).
FLS, a heterogeneous population of fibroblastic cells, express CD55 and also play a central role in the maintenance of joint inflammation and the destruction of cartilage (8, 46). RA joint pathology is characterized by chronic inflammation of the synovium (synovitis), which causes cartilage and bone erosion between inflammatory/immune cells and resident FLSs (47). Under healthy conditions, these cells contribute to the homeostasis of normal joints by synthesizing extracellular matrix (ECM) molecules and secreting specific components of synovial fluid (48). Synovial Fibroblasts respond to inflammatory cytokines, mainly TNF-α, by producing a large variety of inflammatory mediators along with tissue destruction (49, 50).
MSCs are also shown to be present in various areas of the joint (51). Immunoregulatory function of MSCs can be modulated by proinflammatory cytokines such as IFN-γ, TNF-α, and IL-1α or β (52). Synovial MSCs express CD44, CD90, CD271, and UDPGD, required for hyaluronan synthesis, and possess high chondrogenic potential (53). Synovial MSCs, which when healthy, maintain tissues and facilitate the repair process. While both FLSs and MSCs are part of the synovium, their functional specialization and diversification may be dependent on their positional information and environmental cues (54); however the relationship between MSCs and FLSs remains unclear. MSCs in the synovial lining could be perhaps stem cells interspersed between the FLSs and synovial macrophages. Alternatively, the FLSs could be a stage of differentiation of the MSC lineage, taking on FLS-specific properties, but still maintaining their MSC lineage (54).
While immune cells have been extensively investigated in the pathogenesis of RA, little is known about the in vivo functions of FLSs/MSCs in the regulation of immune homeostasis in physiology and their contribution to immune regulation in RA. Under normal conditions, FLSs/MSCs would control the degree of immune responses; however, the inflammatory environmental signals cue inflammatory cells, unsettling the immunomodulatory functions of FLSs/MSCs, damaging the pannus, contributing to chronic disease maintenance and progression (55). Aberrant cross-talk between FLSs/MSCs and immune cells (T-cells, B cells and macrophages) could be a vicious cycle of chronic RA progression (54). This could be due to MSCs ability to express inflammatory mediators such as prostaglandin E2 and IL-6. Also enzymatic production of arachidonic acid enhanced in MSCs by TNF-α or IFN-γ have a deleterious effect on immune cells in the RA microenvironment (56). Thus, heterogeneity of MSCs in terms of immune and hematopoietic function can either maintain immune homeostasis or promote RA pathogenesis.
Healthy MSC function has been shown to inhibit inflammatory responses and improve regeneration (57, 58) by: (a) inhibiting inflammatory cell infiltration and inflammatory cytokine release (59); (b) activating regulatory T-cells (Tregs) (60); and (c) influencing the transition from Th1 cells toward Th2 cells (61). MSCs exert their regulatory activities through the release of immunomodulatory molecules such as IL-10, TGF-β, PGE2, and indoleamine 2,3-dioxygenase (IDO) (62, 63). In addition, MSCs are able to polarize macrophage differentiation toward the anti-inflammatory M2 phenotype in vitro and in vivo (64, 65); inhibit T-cell proliferation (61, 66); and induce the formation of Tregs (67, 68). As such, MSCs are an attractive target for immunomodulation, particularly in the treatment of cartilage injuries and diseases such as RA (54), as modulation of resident synovial MSCs could lead to the control of the inflammatory immune response (57) and ultimately decrease the RA-associated angiogenesis processes.
Stimulation of resident MSCs, or other tissue specific cells to improve inflammation and/or tissue regeneration, is a relatively new concept in medicine that could potentially be achieved by the use of PEMF (10, 69–72). PEMF has the potential to prevent aberrant and promote healthy MSC function. PEMF has been shown to induce differentiation of MSCs to promote immunomodulation and improve cartilage and bone regeneration in vitro (10) and in vivo (73). Stimulation of chondrogenesis in situ through PEMF could lead to an increase of cartilage matrix and collagen levels in RA damaged joints (24, 26, 27, 30, 74, 75). In addition, PEMF promotes proliferation of endogenous chondroblasts (73), supports the enhancement of cartilage regeneration (76), and potentiates MSCs' anti-inflammatory responses. In RA, PEMF also upregulates adenosine receptors to increase anti-inflammatory effects on both chondrocytes and FLS and reduces levels of enzymes produced by FLS and osteoclasts that lead to bone destruction (24, 27, 77) (Table 1).
PEMF as an Alternative to Biologics in the Treatment of RA
The cytokine network in RA is complex and involves an interplay of both pro-inflammatory and anti-inflammatory cytokines. Regulating this cellular microenvironment is essential to maintaining healthy MSC phenotype. In RA, the macrophage-mediated inflammatory response is the main source of proinflammatory cytokines, including TNF-α, IL-1β, IL-6, C-X-C motif chemokine ligand 4 (CXCL4), and CXCL7 (83). While data from clinical trials show some efficacy using biologic drugs, the blockade of these cytokines does not fully control RA in all patients (84, 85). Interleukin-4 (IL-4) and−10 (IL-10) are pleiotropic cytokines considered to be promising modulators to control RA, as these regulatory mediators may have a direct inhibitory effect on the macrophage activity in the synovium (86, 87). While the targeted suppression of key inflammatory pathways involved in joint inflammation and destruction allows better disease control, it comes at the price of elevated infection risk, since blockade of these pathways can lead to broad immunosuppression (88, 89). In addition, these drugs are expensive, costing around $1,000–$3000 US per month, and the risks of prolonged treatment remain uncertain (87). While biologic drugs for RA work by halting the progression of joint damage, and sometimes pushing RA into remission, preliminary evidence shows loss of efficacy over time; therefore, rotation between available biological drugs is often necessary to maintain a good clinical response (89). Another unknown is the appropriate treatment duration for biologic medications. Once remission of the disease is achieved, it is unclear whether the drugs need to be maintained, or if they can safely be suspended (87, 90).
The pro-inflammatory transcription factor nuclear factor kappa B (NF-kB) plays crucial roles in the regulation of inflammation and immune responses by controlling the transcription of multiple cytokine genes (e.g., TNF-α, IL-1, IL-6, and INF-γ), as well as genes involved in cell survival. Given its central role in the control of inflammation and immunity, it is not surprising that inappropriate NF-kB activity has been linked to many autoimmune and inflammatory diseases, including RA (91–93). Exposure to PEMF induces early upregulation of adenosine receptors A2A and A3 that reduce PGE2 and pro-inflammatory cytokines such as TNF-α, which combine to inhibit the activation of transcription factor NF-kB (94, 95). Specifically, at 5 Hz, 0.04 mT, a 1 h exposure to PEMF has been shown to down-regulate both NF-kB and TNF-α in murine macrophages (75). By inhibiting NF-kB activation (94), exposure to PEMF led to decreased production of TNF-α, IL-1β, IL-6, and PGE2 in human chondrocytes, osteoblasts, and synovial fibroblasts (94, 96).
It is important to note inflammatory cytokines can prevent MSCs differentiation, repressing their stem cell function. Cytokines, ions, growth factors, and chemokines modulate physiological processes of MSCs through their microenvironment (97). In both animal and clinical trials, TNF-α, IL-1β, IL-6, PGE2, and the anti-inflammatory cytokine IL-10 have all been shown to be modulated by PEMF (98–101). Exposure to PEMF has also been shown to stabilize plasma membrane Ca2+ ATPase (PMCA) activity (35). PMCA is a transport protein that removes Ca2+ from the cell, and thereby regulates the intracellular concentration of Ca2+ in all eukaryotic cells (102). These extremely low frequencies have a documented record of long-term safety, and their anti-inflammatory properties are well-established in animal arthritis models (35, 37). In double-blind clinical trials in which the knees and spine of RA patients were exposed to 5 Hz, 10–20 Gauss PEMF exposure for 10–30 min/day, 3–5x/ week for 1 month, up to a 47% improvement was documented in various clinical measures such as pain severity, joint tenderness and range of motion (24, 103). These beneficial clinical effects were attributed to PEMF's ability to significantly reduce the production of the RA-associated inflammatory cytokines IL-1β, IL-6, TNF-α, and PGE2, while increasing the levels of the anti-inflammatory cytokine IL-10 in peripheral blood mononuclear cells (PBMCs) such as T-cells and macrophages (26, 96, 104).
Table 2 provides a summary of the various parameters with which PEMF has been explored to-date for its ability to modulate cytokines and growth factors.
Ability of ELF-PEMF to Potentially Restore Angiogenic Homeostasis
Angiogenesis is the formation of new capillaries from pre-existing vasculature, and this process plays a critical role in the pathogenesis of several inflammatory autoimmune diseases such as RA (106). In RA, excessive infiltration of circulating leukocytes into the inflamed joint induces synovial tissue macrophages and fibroblasts to produce inflammatory and proangiogenic factors, such as TNF-α, IL-1β, IL-6, IL-17, and TGF-β that trigger neoangiogenesis (95, 106, 107). This inappropriate neoangiogenesis is also known to play a key role in the abnormal tissue growth, disordered tissue perfusion, abnormal ossification, enhanced responses to normal or pathological stimuli (108), and the development of the hyperplasic proliferative pathologic synovium (7). This area, called “pannus,” destroys articular cartilage, subchondral bone, and periarticular soft tissue, further increasing the density of synovial blood vessels required to develop the hyperplasic and invasive nature of the RA synovium (41). Although these newly formed blood vessels deliver oxygen to the augmented inflammatory cell mass, the neovascular network is dysfunctional and thus fails to restore tissue oxygen homeostasis. As a result, the rheumatoid joint remains in a markedly hypoxic environment (109). Hypoxia has been shown to activate NF-kB, which in turn activates macrophages, fibroblasts, and endothelial cells (107), stimulating further release of proinflammatory cytokines and growth factors (110–112) that directly or indirectly mediate inflammatory angiogenesis (113, 114). Repetitive cycles of hypoxia and reoxygenation, together with oxidants produced by phagocytic cells, promote a state of chronic oxidative stress within the microenvironment of the affected joint, leading to the generation of reactive oxygen species (ROS), which can further contribute to tissue damage. Given the central role neoangiogenesis plays in the pathogenesis of RA, anti-angiogenic therapy appears ideal.
While angiogenesis forms from new capillaries from pre-existing vessels, vasculogenesis is established capillarity formation from endothelial precursor cells (EPCs). Current understanding of the role of angiogensis and vasculogensis in RA is a focus of therapeutic intervention (115). Angiogenesis is profuse in RA and causes defective EPC function, leading to atherosclerosis and vascular disease in arthritis (115). Angiogenesis is essential for the expansion of synovial tissue in RA: pre-existing vessels facilitate the entry of blood-derived leukocytes into the synovial sublining, to generate and potentiate inflammation. Several steps are involved in angiogenesis, each of which is modulated by specific factors (10). The process starts with growth factors such as vascular endothelial growth factor (VEGF) and fibroblast growth factor (FGF) binding to their cognate receptors on endothelial cells (ECs) and activation of these cells to produce proteolytic enzymes (116). Recent evidence has emerged that implicates VEGF to be one of the key players in RA pathogenesis and vascular abnormalities (7, 41). For example, VEGF expression levels in synovial fluid and tissues have been shown to correlate with the clinical severity of RA, and with the degree of joint destruction (117). Proangiogenic factors such as VEGF are modulators of change in vascular permeability, and studies suggest that capillaries are more deeply distributed in the RA synovium, compared with normal tissue (118, 119). The synthesis of VEGF is induced by cytokines and growth factors (e.g., TNF-α), and through oxidative stress, and hypoxia (117, 120). Overexpression of VEGF-C in FLS by stimulation with TNF-alpha may play an important role in the progression of synovial inflammation and hyperplasia in RA by contributing to local lymphangiogenesis and angiogenesis (121). Both oxidative stress and hypoxia are present within the joints of RA patients (117). TNF-α has also been reported to induce the release of VEGF from endothelial cells (122), which can lead to an imbalance between endothelial cells (EC) tube formation and the parallel development of MSCs/pericytes and thereby altering angiogenesis and vasculogenesis (107).
MSCs are perivascular cells that are precursors of pericytes and adventitial cells that envelop microvessels and surround larger arteries and veins, as well as the myriad of other stromal cells that act in concert to maintain/restore tissue homeostasis (123, 124). Aberrant MSCs can release various inflammatory cytokines and VEGF (85), enhancing tissue inflammation (108), and promoting angiogenesis, both of which are of direct relevance to the pathogenesis of RA (125). Pericytes have been shown to possess stem-like qualities, and have been hypothesized to be the in vivo counterparts, or precursors, of MSCs (126–128). MSC/pericytes are recognized for their central role in blood vessel formation, and they act as a repair system in response to injury by maintaining the structural integrity of blood vessels (129). Pericytes have been shown to both stabilize and promote capillary sprouting (130). Perivascular pericytes envelop the vascular tube surface of the inner EC layer that lines the blood vessel wall (131). Because of their close anatomical and functional association with ECs, pericytes are thought to regulate capillary diameter and physically influence EC behavior (132) via contraction in response to electrical or neurotransmitter stimulation (133). Homing of endothelial progenitor cells (EPCs) to an RA injury site is important for repair of vasculature and angiogenesis. Applied direct current (DC) electric fields has been reported to guide EPC migration through VEGF receptor signaling in vitro, controlling EPC behavior to heal injury sites in the vascular (134). PEMF has also been reported to increase the number and function of circulating EPCs in treating myocardial ischemia/reperfusion (I/R) injury in rats (135).
Collectively, these data point to EPCs and MSCs as highly localized modulators of blood flow (130). It has also been found that MSCs can stabilize blood vessels and contribute to tissue and immune system homeostasis under physiological conditions by assuming a more active role in tissue repair in response to injury (136). As such, MSCs/pericytes represent a logical target for new in vivo therapeutic approaches to treating the vascular abnormalities present in RA and halting disease progression to restore homeostasis (136). Since PEMF have been shown to stimulate the production of MSCs (137), and MSCs can stabilize blood vessels and contribute to immune system homeostasis, the possibility exists that PEMF could provide a therapeutic application to restore immune balance and bringing hypoxic conditions and synovial angiogenesis back to a state of homeostasis.
MSCs represent an ideal target on which PEMF can initiate their effects on the aberrant immune response that drives the pathogenesis of RA. MSCs/pericytes down-modulate the production of synovial macrophages, which trigger production of cytokines, such as IL-4, that initiate the proliferation of synovial fibroblasts, promoting the expression of growth factors such as VEGF and TGF-β (138, 139). Exposure of MSCs/pericytes to PEMF appears to trigger a cascade of downstream effects on multiple pathways, affecting macrophages, T-cells, and B cells, and the cytokines that are produced. The cumulative result of these varied effects is modulation of VEGF and TGF-β, which ultimately curtails the production of synovial fibroblasts and osteoclasts and halts bone resorption, while promoting the production of chondrocytes and osteoblasts to restore cartilage and bone health/integrity (Figure 1).
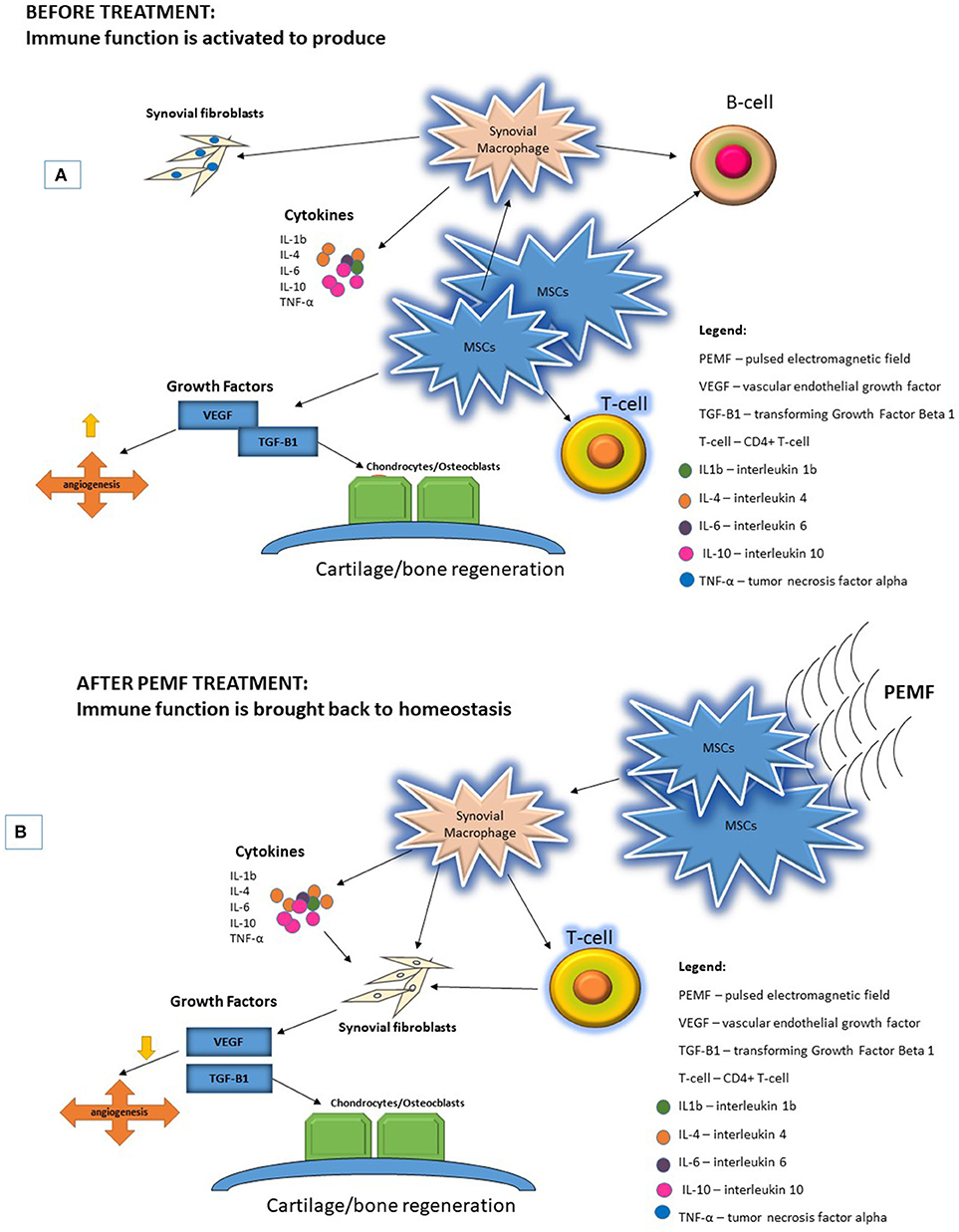
Figure 1. PEMF are physical stimuli that produce membrane activations of multiple cellular pathways. (A) RA pathogenesis begins with activation of immune function increasing proinflammatory cytokines and upregulating growth factors to increase FLS proliferation and bone resorption. (B) Application of PEMF could potentially bring immune function back to homeostasis.
The effects of PEMF on vessel growth and development, both in vitro and in vivo, support the use of this approach to therapeutically modulate the aberrant angiogenesis present in RA, (140–142). PEMF has been reported to improve osteochondral ossification, and modulate nociception (143–146) through the down-regulation of neovascularization (15, 147, 148) in both animals and humans with RA (9, 24, 25, 27, 149). It has also been reported to significantly reduce activation levels of VEGF (15), to inhibit the proliferative ability of human umbilical vein endothelial cells (HUVECs) (148), and to reduce the extent of vascularization in diseased tissue (142). Approximately half of the cited studies of PEMF application indicate a vasodilatory effect, the magnitude of which is dependent upon the initial vessel tone. The remaining half indicates that PEMF has the potential to trigger vasoconstriction. The ultimate outcome of PEMF application thus appears to depend on the cellular/mechanistic basis of the disease in question (140). A summary of some of the studies that have explored the use of various regimens of PEMF to potentially restore angiogenic homeostasis appear in Table 3.
Conclusion
Under normal physiological conditions, MSCs in the joint are believed to contribute to the maintenance and repair of joint tissues. In RA, however, the repair function of MSCs appears to be repressed by the inflammatory milieu. In addition to being passive targets, MSCs could interact with the immune system and play an active role in the perpetuation of arthritis and progression of joint damage (54). Achieving homeostasis in the face of acute inflammatory/immune challenges in the human body involves maintaining a balance of highly complex biochemical and cellular interactions. When this delicate balance is upset, acute inflammatory and immune responses designed to quickly eliminate a transient threat become chronic, and inflammatory/autoimmune disease sets in. RA is a paradigmatic autoimmune disease, and current RA therapies target inflammatory molecules involved in autoimmune activation. Despite the therapeutic improvements in RA, there are still a substantial number of patients who respond only transiently to these approaches, and others who do not respond at all. As such, there is an urgent unmet need to identify complementary and innovative therapies for the treatment of RA.
PEMF is emerging as a novel and highly promising means of treating chronic inflammation and aberrant immunity that exists in diseases such as RA. It can be used to target aberrant MSCs to potentially bring the inflammatory milieu back to homeostasis. Cellular electrical properties such as membrane surface charge and membrane potential can be readily influenced by PEMF (151–153), which can affect oscillatory frequencies of the myriad of enzymes present within the cells. PEMF can also influence cell membranes, nucleic acids, and bioelectrical phenomena generated by coherent groups of cells that are essential to cell-to-cell communication processes (154, 155). PEMF appears to exert its effects on cellular function and differentiation by altering the spatial and temporal patterns of intracellular calcium (Ca2+) concentration (10) and restoring levels/activity of potassium (K+) channels (17, 156, 157). By restoring normal Ca2+ ion flux and Na+/K+ balance, the cell can begin the process of down-regulating inflammatory cytokines, heat-shock proteins, and proangiogenic molecules such as VEGF (157), making it possible for the body to commence rebuilding healthy cartilage. Using PEMF to modulate inflammation and immune function is relatively safe in contrast to the broad immunosuppression currently in clinical favor (39, 158). An alternative to immunosuppression–healthy immunomodulation and tissue repair–can be achieved by targeting MSCs with PEMF. While traditional approaches target individual molecules or signaling pathways, PEMF works on all cellular/organismal systems in a holistic and integrative manner by potentially bringing the transmission and flow of information (signal transduction) back to a state of homeostasis via coherence of sinusoidal pulses (159). There are other potential advantages of PEMF including low-cost, easy-to-use at-home, without adverse effects. While cell therapies or biologics suffer from the possibility of loss of efficacy over time (87), preliminary clinical studies with PEMF have shown no loss of efficacy even after exposure to the field has ended (160). Another key unsolved problem in the treatment/management of RA is determining the optimal duration of therapy, and the lack of data to inform clinicians whether drugs should be suspended once remission of the disease is obtained (87). PEMF has the advantage of use without concerns regarding global immunosuppression until the desired clinical outcome is obtained (87). Since MSCs are ubiquitous, targeting their regenerative, and anti-inflammatory capacities would be an optimal combination of exogenous (PEMF), and endogenous (MSC) therapies. Clinical applications include whole-body mats for systemic approach (161), and hand-held devices for localized therapy (149). For localized applications, direct capacitive coupling mechanisms such as electrodes adhere to the site of inflammation/tissue degeneration. For non-direct capacitive/inductive coupling, mats can be used for full body applications. Current research shows optimal frequency <75 Hz, with optimal intensity (field strength) <5 mT, and optimal time courses ranging between 15 and 90 min, with longer duration most effective for severe symptoms.
Author Contributions
GA-P provided expertise and contributed editorial and written content on mesenchymal stromal cells (MSCs). DA provided expertise on RA and contributed editorial and written content on RA pathology. CR wrote the manuscript and provided expertise on the therapeutic effects of pulsed electromagnetic field for the treatment of RA.
Conflict of Interest Statement
The authors declare that the research was conducted in the absence of any commercial or financial relationships that could be construed as a potential conflict of interest.
Acknowledgments
We wish to acknowledge the Guth Family Fund WFBHA-63313-740-120330-740196 for their continued support.
References
1. Network RAS. Rheumatoid Arthritis Facts and Statistics. (2016) Available online at: https://www.rheumatoidarthritis.org/ra/facts-and-statistics/ (Accessed August 31, 2017).
2. Isaacs J. The changing face of rheumatoid arthritis: sustained remission for all? Nat Rev Immunol. (2010) 10:605–11. doi: 10.1038/nri2804
3. Smolen J, Aletaha D, Redlich K. The pathogenesis of rheumatoid arthritis: new insights from old clinical data? Nat Rev Rheumatol. (2012) 8:235–43. doi: 10.1038/nrrheum.2012.23
4. Center JHA. Rheumatoid Arthritis Treatment. (2017) Available online at: https://www.hopkinsarthritis.org/arthritis-info/rheumatoid-arthritis/ra-treatment/ (Accessed September 11, 2017).
5. Ethgen O, de Lemos Esteves F, Bruyere O, Reginster JY. What do we know about the safety of corticosteroids in rheumatoid arthritis? Curr Med Res Opin. (2013) 29:1147–60. doi: 10.1185/03007995.2013.818531
6. Ramiro S, Gaujoux-Viala C, Nam JL, Smolen JS, Buch M, Gossec L, et al. Safety of synthetic and biological DMARDs: a systematic literature review informing the 2013 update of the EULAR recommendations for management of rheumatoid arthritis. Ann Rheum Dis. (2014) 73:529–35. doi: 10.1136/annrheumdis-2013-204575
7. Paleolog E. The vasculature in rheumatoid arthritis: cause or consequence? Int J Exp Path. (2009) 90:249–61. doi: 10.1111/j.1365-2613.2009.00640.x
8. Fu H, Hu D, Zhang L, Tang P. Role of extracellular vesicles in rheumatoid arthritis. Mol Immunol. (2018) 93:125–32. doi: 10.1016/j.molimm.2017.11.016
9. Ganesan K, Gengadharan A, Balachandran C, Manohar B. Low frequency pulsed electromagnetic field - a viable alternative for arthritis. Indian J Exp Biol. (2009) 47:939–48. doi: 10.1002/bem.20535
10. Ross C, Siriwardane ML, Almeida-Porada G, Proada CD, Brink P, Christ GJ, et al. The effect of low-frequency electromagnetic field on human bone-marrow derived mesenchymal stem/progenitor cell differentiation. Stem Cell Res. (2015) 15:96–108. doi: 10.1016/j.scr.2015.04.009
11. Trock D. Electromagnetic fields and magnets: investigational treatment for musculoskeletal disorders. Rheum Dis Clin North Am. (2000) 26:51–62. doi: 10.1016/S0889-857X(05)70119-8
12. Stiller M, Pak GH, Shupack JL, Thaler S, Kenny C, Jondreau L. A portable pulsed electromagnetic field (PEMF) device to enhance healing of recalcitrant venous ulcers: a double-blind, placebo-controlled clinical trial. Br J Dermatol. (1992) 127:47–54. doi: 10.1111/j.1365-2133.1992.tb08047.x
13. Cohen D, Palti Y, Cuffin BN, Schmid SJ. Magnetic fields produced by steady currents in the body. Proc Natl Acad Sci USA. (1980) 77:1447–51.
14. Liboff A, McLeod BR. Kinetics of channelized membrane ions in magnetic field. Bioelectromagnetics. (1988) 9:39–51.
15. Delle-Monache S, Angelucci A, Sanità P, Iorio R, Bennato F, Mancini F, et al. Inhibition of angiogenesis mediated by extremely low-frequency magnetic fields (ELF-MFs). PLoS ONE. (2013) 8:e79309. doi: 10.1371/journal.pone.0079309
16. Gordon G. Designed electromagnetic pulsed therapy: clinical applications. J Cell Physiol. (2007) 212:579–82. doi: 10.1002/jcp.21025
17. Ross C. The use of electric, magnetic, and electromagnetic field for directed cell migration and adhesion in regenerative medicine. Biotechnol Prog. (2016) 33:5–16. doi: 10.1002/btpr.2371
18. Chen C, Lin YS, Fu YC, Wang CK, Wu SC, Wang GJ, et al. Electromagnetic fields enhance chondrogenesis of human adipose-derived stem cells in a chondrogenic microenvironment in vitro. J Appl Physiol. (2013) 114:647–55. doi: 10.1152/japplphysiol.01216.2012
19. Sun W, Gan Y, Fu Y, Lu D, Chiang H. An incoherent magnetic field inhibited EFG receptor clustering and phosphorylation induced by a 50 Hz magnetic field in cultured FL cells. Cell Physiol Biochem. (2008) 33:508–14. doi: 10.1159/000185524
20. Nie K, Henderson A. MAP kinase activation in cells exposed to a 60 Hz electromagnetic field. J Cell Biochem. (2003) 90:1197–206. doi: 10.1002/jcb.10704
21. Goodman R, Lin-Ye A, Geddis MS, Wickramaratne PJ, Hodge SE, Pantazatos SP, et al. Extremely low frequency electromagnetic fields activate the ERK cascade, increase hsp70 protein levels and promote regeneration in planaria. Int J Radiat Biol. (2009) 85:851–9. doi: 10.1080/09553000903072488
22. Bekhite M, Finkensieper A, Abou-Zaid FA, El-Shourbagy IK, Omar KM, Figulla HR, et al. Static electromagnetic fields induce vasculogenesis and chondro-osteogenesis of mouse embryonic stem cells by reactive oxygen species-mediated up-regulation of vascular endothelial growth factor. Stem Cells Dev. (2010) 19:731–43. doi: 10.1089/scd.2008.0266
23. Li X, Zhang M, Bai L, Bai W, Xu W, Zhu H. Effects of 50 Hz pulsed electromagnetic fields on the growth and cell cycle arrest of mesenchymal stem cells: an in vitro study. Electromagn Biol Med. (2012) 31:356–64. doi: 10.3109/15368378.2012.662194
24. Ganguly K, Sarkar AK, Datta AK, Rakshit A. A study of the effects of pulsed electromagnetic field therapy with respect to serological grouping in rheumatoid arthritis. J Indian Med Assoc. (1998) 96:272–5.
25. Shupak N, McKay JC, Nielson WR, Rollman GB, Prato FS, Thomas AW. Exposure to a specific pulsed low-frequency magnetic field: a double-blind placebo-controlled study of effects on pain ratings in rheumatoid arthritis and fibromyalgia patients. Pain Res Manag. (2006) 11:85–90. doi: 10.1155/2006/842162
26. Gómez-Ochoa I, Gómez-Ochoa P, Gómez-Casal F, Cativiela E, Larrad-Mur L. Pulsed electromagnetic fields decrease proinflammatory cytokine secretion (IL-1β and TNF-α) on human fibroblast-like cell culture. Rheumatol Int. (2011) 31:1283–9. doi: 10.1007/s00296-010-1488-0
27. Gajewski M, Rzodkiewicz P, Maśliński S, Wojtecka-Łukasik E. The role of physiological elements in future therapies of rheumatoid arthritis. III The role of the electromagnetic field in regulation of redox potential and life cycle of inflammatory cells. Rheumatol Clin. (2015) 53:219–24. doi: 10.5114/reum.2015.54000
28. Ladoux B, Mège RM. Mechanobiology of collective cell behaviours. Nat Rev Mol Cell Biol. (2017) 18:743–57. doi: 10.1038/nrm.2017.98
29. Pakhomov A, Bowman AM, Ibey BL, Andre FM, Pakhomova ON, Schoenbach KH. Lipid nanopores can form a stable, ion channel-like conduction pathway in cell membrane. Biochem Biophys Res Commun. (2009) 385:181–6. doi: 10.1016/j.bbrc.2009.05.035
30. Ross C, Harrison BS. The use of magnetic field for the reduction of inflammation: a review of the history and therapeutic results. Altern Ther Health Med. (2013) 19:47–54.
31. Panagopoulos D, Karabarbounis A, Margaritis LH. Mechanism for action of electromagnetic fields on cells. Biochem Biophys Res Commun. (2002) 298:95–102. doi: 10.1016/S0006-291X(02)02393-8
32. Semenov I, Xiao S, Pakhomov AG. Primary pathways of intracellular Ca(2+) mobilization by nanosecond pulsed electric field. Biochim Biophys Acta (2013) 1828:981–9. doi: 10.1016/j.bbamem.2012.11.032
33. Tolstykh G, Beier HT, Roth CC, Thompson GL, Payne JA, Kuipers MA, et al. Activation of intracellular phosphoinositide signaling after a single 600 nanosecond electric pulse. Bioelectrochemistry (2013) 94:23–9. doi: 10.1016/j.bioelechem.2013.05.002
34. Pilla A, Fitzsimmons R, Muehsam D, Wu J, Rohde C, Casper D. Electromagnetic fields as first messenger in biological signaling: application to calmodulin-dependent signaling in tissue repair. Biochim Biophys Acta. (2011) 1810:1236–45. doi: 10.1016/j.bbagen.2011.10.001
35. Selvam R, Ganesan K, Narayana Raju KV, Gangadharan AC, Manohar BM, Puvanakrishnan R. Low frequency and low intensity pulsed electromagnetic field exerts its antiinflammatory effect through restoration of plasma membrane calcium ATPase activity. Life Sci. (2007) 80:2403–10. doi: 10.1016/j.lfs.2007.03.019
36. Poornapriya T, Meera R, Devadas S, Puvanakrishnan R. Preliminary studies on the effect of electromagnetic field in adjuvant induced arthritis in rats. Med Sci Res. (1998) 26:467–9.
37. Kumar V, Kumar DA, Kalaivani K, Gangadharan AC, Narayana Raju KVS, Thejomoorthy P, et al. Optimization of pulsed electromagnetic field therapy for management of arthritis in rats. Bioelectromagnetics. (2005) 26:431–9. doi: 10.1002/bem.20100
38. Sanseverino E, Vannini A, Castellacci P. Therapeutic effects of pulsed magnetic fields on joint diseases. Panminerva Med. (1992) 34:187–96.
39. Ohtani S, Ushiyama A, Maeda A, Ogasawara Y, Wang J, Kunugita N, et al. The effects of ratio-frequency electromagnetic fields on T cell function during development. J Rad Res. (2015) 56:467–74. doi: 10.1093/jrr/rru126
40. De Bari C, Dell'Accio F, Tylzanowski P, Luyten FP. Multipotent mesenchymal stem cells from adult human synovial membrane. Arthritis Rheum. (2001) 44:1928–42. doi: 10.1002/1529-0131(200108)44%3A8<1928%3A%3AAID-ART331>3.0.CO%3B2-P
41. Paleolog E. Angiogenesis in rheumatoid arthritis. Arthritis Res Ther. (2002) 4:S81–90. doi: 10.1186/ar575
42. McInnes I, Schett G. Cytokines in the pathogenesis of rheumatoid arthritis. Nat Rev Immunol. (2007) 7:429–42. doi: 10.1038/nri2094
43. McGonagle D, McDermott MF. A proposed classification of the immunological diseases. PLoS Med. (2006) 3:e297. doi: 10.1371/journal.pmed.0030297
44. Cutolo M, Sulli A, Barone A, Seriolo B, Accardo S. Macrophages, synovial tissue and rheumatoid arthritis. Clin Exp Rheumatol. (1993) 11:331–9.
45. Blom A, van Lent PL, Holthuysen AE, van der Kraan PM, Roth J, van Rooijen N, et al. Synovial lining macrophages mediate osteophyte formation during experimental osteoarthritis. Osteoarthritis Cartil. (2004) 12:627–35. doi: 10.1016/j.joca.2004.03.003
46. Del Rey M, Faré R, Usategui A, Cañete JD, Bravo B, Galindo M, et al. CD271(+) stromal cells expand in arthritic synovium and exhibit a proinflammatory phenotype. Arthritis Res Ther. (2016) 18:66. doi: 10.1186/s13075-016-0966-5
47. Tu J, Hong W, Zhang P, Wang X, Körner H, Wei W. Ontology and function of fibroblast-like and macrophage-like synoviocytes: how do they talk to each other and can they be targeted for rheumatoid arthritis therapy? Front Immunol. (2018) 9:1467. doi: 10.3389/fimmu.2018.01467
48. Uccelli A, Moretta L, Pistoia V. Mesenchymal stem cells in health and disease. Nat Rev Immunol. (2008) 8:726–36. doi: 10.1038/nri2395
49. Shi C. Recent progress toward understanding the physiological function of bone marrow mesenchymal stem cells. Immunol Infect Dis. (2012) 136:133–8. doi: 10.1111/j.1365-2567.2012.03567.x
50. Markides H, Kehoe O, Morris RH, El Haj AJ. Whole body tracking of superparamagnetic iron oxide nanoparticle-labelled cells–a rheumatoid arthritis mouse model. Stem Cell Res Ther. (2013) 4:126. doi: 10.1186/scrt337
51. El-Jawhari J, El-Sherbiny YM, Jones EA, McGonagle D. Mesenchymal stem cells, autoimmunity and rheumatoid arthritis. QJM. (2014) 107:505–14. doi: 10.1093/qjmed/hcu033
52. Krampera M, Cosmi L, Angeli R, Pasini A, Liotta F, Andreini A, et al. Mesenchymal stromal cell 'licensing': a multi-step process. Leukemia (2011) 25:1408–14. doi: 10.1038/leu.2011.108
53. de Sousa E, Casado PL, Neto VM, Duarte MEL, Aguiar DP. Synovial fluid and synovial membrane mesenchymal stem cells: latest discoveries and therapeutic perspectives. Stem Cell Res Ther. (2014) 5:112. doi: 10.1186/scrt501
54. De Bari C. Are mesenchymal stem cells in rheumatoid arthritis the good or bad guys? Arthritis Res Ther. (2015) 17:113. doi: 10.1186/s13075-015-0634-1
55. Vandenabeele F, De Bari C, Moreels M, Lambrichts I, Dell'Accio F, Lippens PL, et al. Morphological and immunocytochemical characterization of cultured fibroblast-like cells derived from adult human synovial membrane. Arch. Histol. Cytol. (2003) 66:145–53. doi: 10.1679/aohc.66.145
56. Jorgensen C. Mesenchymal stem cells in arthritis: role of bone marrow microenvironment. Arthritis Res Ther. (2010) 12:135–6. doi: 10.1186/ar3105
57. Li R, Zhao SZ. Control and cross talk between angiogenesis and inflammation by mesenchymal stem cells for the treatment of ocular surface diseases. Stem Cell Int. (2016) 2016:7961816. doi: 10.1155/2016/2470351
58. Phinney D, Prockop DJ. Mesenchymal stem/multipotent stromal cells: the state of transdifferentiation and modes of tissue repair–current views. Stem Cells. (2007) 25:2896–902. doi: 10.1634/stemcells.2007-0637
59. Tu X, Huang SX, Li WS, Song JX, Yang XL. Mesenchymal stem cells improve intestinal integrity during severe acute pancreatitis. Mol Med Rep. (2014) 10:1813–20. doi: 10.3892/mmr.2014.2453
60. Baharlou R, Ahmadi-Vasmehjani A, Faraji F, Atashzar MR, Khoubyari M, Ahi S, et al. Human adipose tissue-derived mesenchymal stem cells in rheumatoid arthritis: regulatory effects on peripheral blood mononuclear cell activation. Int Immunopharmacol. (2017) 47:59–69. doi: 10.1016/j.intimp.2017.03.016
61. Aggarwal S, Pittenger MF. Human mesenchymal stem cells modulate allogeneic immune cell responses. Blood. (2005) 105:1815–22. doi: 10.1182/blood-2004-04-1559
62. Kyurkchiev D, Bochev I, Ivanova-Todorova E, Mourdjeva M, Oreshkova T, Belemezova K, et al. Secretion of immunoregulatory cytokines by mesenchymal stem cells. World J Stem Cells. (2014) 6:552–70. doi: 10.4252/wjsc.v6.i5.552
63. Otto W, Wright NA. Mesenchymal stem cells: from experiment to clinic. Fibrogenesis Tissue Repair. (2011) 4:20. doi: 10.1186/1755-1536-4-20
64. Németh K, Leelahavanichkul A, Yuen PS, Mayer B, Parmelee A, Doi K, et al. Bone marrow stromal cells attenuate sepsis via prostaglandin E(2)-dependent reprogramming of host macrophages to increase their interleukin-10 production. Nat Med. (2009) 15:42–9. doi: 10.1038/nm.1905
65. François M, Romieu-Mourez R, Li M, Galipeau J. Human MSC suppression correlates with cytokine induction of indoleamine 2,3-dioxygenase and bystander M2 macrophage differentiation. Mol Ther. (2012) 20:187–95. doi: 10.1038/mt.2011.189
66. Zappia E, Casazza S, Pedemonte E, Benvenuto F, Bonanni I, Gerdoni E, et al. Mesenchymal stem cells ameliorate experimental autoimmune encephalomyelitis inducing T-cell anergy. Blood. (2005) 106:1755–61. doi: 10.1182/blood-2005-04-1496
67. Prevosto C, Zancolli M, Canevali P, Zocchi MR, Poggi A. Generation of CD4+ or CD8+ regulatory T cells upon mesenchymal stem cell-lymphocyte interaction. Haematol Lat. (2007) 92:881–8. doi: 10.3324/haematol.11240
68. Augello A, Tasso R, Negrini SM, Cancedda R, Pennesi G. Cell therapy using allogeneic bone marrow mesenchymal stem cells prevents tissue damage in collagen-induced arthritis. Arthritis Rheum. (2007) 56:1175–86. doi: 10.1002/art.22511
69. Mayer-Wagner S, Passberger A, Sievers B, Aigner J, Summer B, Schiergens TS, et al. Effects of low frequency electromagnetic fields on the chondrogenic differentiation of human mesenchymal stem cells. Bioelectromagnetics. (2011) 32:283–90. doi: 10.1002/bem.20633
70. Ross C. Optimal Time of Efficacy for Using Bone Tissue Engineered Cell Therapies and Pulsed Electromagnetic Field [PEMF] for the Treatment of Osteoporosis. Cell Stem Cells Regen Med. (2017) 3:1–6. doi: 10.16966/2472-6990.116
71. Urnukhsaikhan E, Cho H, Mishig-Ochir T, Seo YK, Park JK. Pulsed electromagnetic fields promote survival and neuronal differentiation of human BM-MSCs. Life Sci. (2016) 151:130–8. doi: 10.1016/j.lfs.2016.02.066
72. Viganò M, Sansone V, d'Agostino MC, Romeo P, Perucca Orfei C, de Girolamo L. Mesenchymal stem cells as therapeutic target of biophysical stimulation for the treatment of musculoskeletal disorders. J Orthop Surg Res. (2016) 11:163. doi: 10.1186/s13018-016-0496-5
73. Fitzsimmons R, Gordon SL, Kronberg J, Ganey T, Pilla AA. A pulsing electric field (PEF) increases human chondrocyte proliferation through a transduction pathway involving nitric oxide signaling. J Orthop Res. (2008) 26:854–9. doi: 10.1002/jor.20590
74. Ross C, Harrison BS. An introduction to electromagnetic field therapy and immune function: a brief history and current status. J Sci Appl Biosci. (2015) 3:17–28.
75. Ross C, Harrison BS. Effect of pulsed electromagnetic field on inflammatory pathway markers in RAW 264.7 murine macrophages. J Inflamm Res. (2013) 6:45–51. doi: 10.2147/JIR.S40269
76. Fini M, Pagaini S, Giavaresi G, De Mattei M, Ongaro N, Varani K, et al. Functional tissue engineering in articular cartilage repair: is there a role for electromagnetic biophysical stimulation? Tissue Eng Part B Rev. (2013) 19:353–67. doi: 10.1089/ten.teb.2012.0501
77. Hong J, Kang KS, Yi HG, Kim SY, Cho DW. Electromagnetically controllable osteoclast activity. Bone. (2014) 62:99–107. doi: 10.1016/j.bone.2014.02.005
78. Chen C, Lin YS, Fu YC, Wang CK, Wu SC, Wang GJ, et al. Electromagnetic fields enhance chondrogenesis of human adipose-derived stem cells in a chondrogenic microenvironment in vitro. Bioelectromagnetics. (2013) 23:283–90.
79. De Mattei M, Caruso A, Pezzetti F, Pellati A, Stabellini G, Sollazzo V, et al. Effects of pulsed electromagnetic fields on human articular chondrocyte proliferation. Connect Tissue Res. (2001) 42:269–79. doi: 10.3109/03008200109016841
80. Esposito M, Lucariello A, Costanzo C, Fiumarella A, Giannini A, Riccardi G, et al. Differentiation of human umbilical cord-derived mesenchymal stem cells, WJ-MSCs, into chondrogenic cells in the presence of pulsed electromagnetic fields. In Vivo. (2013) 27:495–500.
81. Parate D, Franco-Obregon A. Enhancement of mesenchymal stem cell chondrogenesis with short-term low intensity pulsed electromagnetic field. Nat Sci Rep. (2017) 7:9421. doi: 10.1038/s41598-017-09892-w
82. Varani K, De Mattei M, Vincenzi F, Gessi S, Merighi S, Pellati A, et al. Characterization of adenosine receptors in bovine chondrocytes and fibroblast-like synoviocytes exposed to low frequency low energy pulsed electromagnetic fields. Osteoarthritis Cartil. (2008) 16:292–304. doi: 10.1016/j.joca.2007.07.004
83. Yi Y. Role of inflammasomes in inflammatory autoimmune rheumatic diseases. Korean J Physiol Pharmacol. (2018) 22:1–15. doi: 10.4196/kjpp.2018.22.1.1
84. Tikiz C, Utuk O, Pirildar T, Bayturan O, Bayindir P, Taneli F, et al. Effects of Angiotensin-converting enzyme inhibition and statin treatment on inflammatory markers and endothelial functions in patients with long term rheumatoid arthritis. J Rheumatol. (2005) 32:2095–101.
85. Lubberts E, van den Berg WB. Cytokines in the Pathogenesis of Rheumatoid Arthritis and Collagen-Induced Arthritis. Mol Immunol. (2000) 2000–13.
86. Venkatesha S, Dudics S, Acharya B, Moudgil KD. Cytokine-modulating strategies and newer cytokine targets for arthritis therapy. Int J Mol Sci. (2014) 16:887–906. doi: 10.3390/ijms16010887
87. Martin-Martin L, Giovannangeli F, Bizzi E, Massafra U, Ballanti E, Cassol M, et al. An open randomized active-controlled clinical trial with low-dose SKA cytokines versus DMARDs evaluating low disease activity maintenance in patients with rheumatoid arthritis. Drug Des Devel Ther. (2017) 11:985–94. doi: 10.2147/DDDT.S118298
88. De Keyser F. Choice of Biologic Therapy for Patients with Rheumatoid Arthritis: The Infection Perspective. Curr Rheumatol Rev. (2011) 7:77–87. doi: 10.2174/157339711794474620
89. Strehblow C, Haberhauer G, Fasching P. Comparison of different biologic agents in patients with rheumatoid arthritis after failure of the first biologic therapy. Wien Med Wochenschr. (2010) 160:225–9. doi: 10.1007/s10354-010-0796-z
90. Metzger G. Biologics for RA. (2017) Available online at: https://www.webmd.com/rheumatoid-arthritis/features/ra-biologics-cost#1 (Accessed March 16, 2018).
91. Roman-Blas J, Jimenez SA. NF-kappaB as a potential therapeutic target in osteoarthritis and rheumatoid arthritis. Osteoarthritis Cartil. (2006) 14:839–48. doi: 10.1016/j.joca.2006.04.008
92. Lawrence T. The nuclear factor NF-κB pathway in inflammation. Cold Spring Harb Perspect Biol. (2009) 1:a001651. doi: 10.1101/cshperspect.a001651
93. van Loo GBR. Negative regulation of NF-κB and its involvement in rheumatoid arthritis. Arthritis Res Ther. (2011) 13:221. doi: 10.1186/ar3324
94. Vincenzi F, Targa M, Corciulo C, Gessi S, Merighi S, Setti S, et al. Pulsed electromagnetic fields increased the anti-inflammatory effect of A2A and A3 adenosine receptors in human T/C-28a2 chondrocytes and hFOB 1.19 osteoblasts. PLoS ONE. (2013) 8:e65561. doi: 10.1371/journal.pone.0065561
95. Simmonds R, Foxwell BM. Signalling, inflammation and arthritis: NF-kappaB and its relevance to arthritis and inflammation. Rheumatology (Oxford). (2008) 47:584–90. doi: 10.1093/rheumatology/kem298
96. Ongaro A, Varani K, Masieri F, Pellati A, Massari L, Cadossi R, et al. Electromagnetic fields (EMFs) and adenosine receptors modulate prostaglandin E(2) and cytokine release in human osteoarthritic synovial fibroblasts. J Cell Physiol. (2012) 227:2461–9. doi: 10.1002/jcp.22981
97. Wang M, Yuan Q, Xie L. Mesenchymal stem cell-based immunomodulation: properties and clinical application. Stem Cells Int. 2018:3057624. doi: 10.1155/2018/3057624
98. Jasti A, Wetzel BJ, Aviles H, Vesper DN, Nindl G, Johnson MT. Effect of a wound healing electromagnetic field on inflammatory cytokine gene expression in rats. Biomed Sci Instrum. (2001) 37:209–14.
99. Nelson F, Zvirbulis R, Pilla AA. Non-invasive electromagnetic field therapy produces rapid and substantial pain reduction in early knee osteoarthritis: a randomized double-blind pilot study. Rheumatol Int. (2013) 33:2169–73. doi: 10.1007/s00296-012-2366-8
100. Rasouli J, Lekhraj R, White NM, Flamm ES, Pilla AA, Strauch B, et al. Attenuation of interleukin-1beta by pulsed electromagnetic fields after traumatic brain injury. Neurosci Lett. (2012) 519:4–8. doi: 10.1016/j.neulet.2012.03.089
101. He Y, Liu DD, Fang YJ, Zhan XQ, Yao JJ, Mei YA. Exposure to extremely low-frequency electromagnetic fields modulates Na+ currents in rat cerebellar granule cells through increase of AA/PGE2 and EP receptor-mediated cAMP/PKA pathway. PLoS ONE. (2013) 8:e54376. doi: 10.1371/journal.pone.0054376
102. Di Leva F, Domi T, Fedrizzi L, Lim D, Carafoli E. The plasma membrane Ca2+ ATPase of animal cells: structure, function and regulation. Arch Biochem Biophys. (2008) 476:65–74. doi: 10.1016/j.abb.2008.02.026
103. Segal N, Toda Y, Huston J, Saeki Y, Shimizu M, Fuchs H, et al. Two configurations of static magnetic fields for treating rheumatoid arthritis of the knee: a double-blind clinical trial. Arch Phys Med Rehabil. (2001) 82:1453–60. doi: 10.1053/apmr.2001.24309
104. de Girolamo L, Viganò M, Galliera E, Stanco D, Setti S, Marazzi MG, et al. In vitro functional response of human tendon cells to different dosages of low-frequency pulsed electromagnetic field. Knee Surg Sports Traumatol Arthrosc. (2015) 23:3443–53. doi: 10.1007/s00167-014-3143-x
105. Tang X, Alliston T, Goughlin D, Miller S, Zhang N, Waldorff EI, et al. Dynamic imaging demonstrates that pulsed electromagnetic fields (PEMF) suppress IL-6 transcription in bovine nucleus pulposus cells. J Orthop Res. 36:778–87. doi: 10.1002/jor.23713
106. Elshabrawy H, Chen Z, Volin MV, Shalini Ravella S, Virupannavar S, Shahrara S. The pathogenic role of angiogenesis in rheumatoid arthritis. Angiogenesis. (2015) 18:433–48. doi: 10.1007/s10456-015-9477-2
107. Leblond A, Allanore Y, Avouac J. Targeting synovial neoangiogenesis in rheumatoid arthritis. Autoimmun Rev. (2017) 16:594–601. doi: 10.1016/j.autrev.2017.04.005
108. Walsh D, Pearson CI. Angiogenesis in the pathogenesis of inflammatory joint and lung diseases. Arthritis Res. (2001) 3:147–53. doi: 10.1186/ar292
109. Taylor P, Sivakumar B. Hypoxia and angiogenesis in rheumatoid arthritis. Curr Opin Rheumatol. (2005) 17:293–8. doi: 10.1097/01.bor.0000155361.83990.5b
110. Quiñonez-Flores C, González-Chávez SA, Pacheco-Tena C. Hypoxia and its implications in rheumatoid arthritis. J Biomed Sci. (2016) 23:62. doi: 10.1186/s12929-016-0281-0
111. Konisti S, Kiriakidis S, Paleolog EM. Hypoxia–a key regulator of angiogenesis and inflammation in rheumatoid arthritis. Nat Rev Rheumatol. (2012) 8:153–62. doi: 10.1038/nrrheum.2011.205
112. Sun X, Zhang H. NFKB and NFKBI polymorphisms in relation to susceptibility of tumour and other diseases. Histol Histopathol. 22:1387–98. doi: 10.14670/HH-22.1387
113. Koch A, Harlow LA, Haines GK, Amento EP, Unemori EN, Wong WL, et al. Vascular endothelial growth factor. A cytokine modulating endothelial function in rheumatoid arthritis. J Immunol. (1994) 152:4149–56.
114. Szekanecz Z, Koch AE. Mechanisms of Disease: angiogenesis in inflammatory diseases. Nat Clin Pract Rheumatol. (2007) 3:635–43. doi: 10.1038/ncprheum0647
115. Szekanecz Z, Besenyei T, Szentpétery A, Koch AE. Angiogenesis and vasculogenesis in rheumatoid arthritis. Curr Opin Rheumatol. (2010) 22:299–306. doi: 10.1097/BOR.0b013e328337c95a
116. MacDonald IL, Li SC, Su CM, Wang YH, Tsai CH, Tang C. Implications of angiogenesis involvement in arthritis. Int J Mol Sci. (2018) 19:E2012. doi: 10.3390/ijms19072012
117. Berse B, Hunt JA, Diegel RJ, Morganelli P, Yeo K, Brown F, et al. Hypoxia augments cytokine (transforming growth factor-beta (TGF-beta) and IL-1)-induced vascular endothelial growth factor secretion by human synovial fibroblasts. Clin Exp Immunol. (1999) 115:176–82. doi: 10.1046/j.1365-2249.1999.00775.x
118. Stevens C, Blake DR, Merry P, Revell PA, Levick JR. A comparative study by morphometry of the microvasculature in normal and rheumatoid synovium. Arthritis Rheum. (1991) 34:1508–13. doi: 10.1002/art.1780341206
119. Stevens C, Williams RB, Farrell AJ, Blake DR. Hypoxia and inflammatory synotitis: observations and speculation. Ann Rheum Dis. (1991) 50:124–32. doi: 10.1136/ard.50.2.124
120. Cho C, Cho ML, Min SY, Kim WU, Min DJ, Lee SS, et al. CD40 engagement on synovial fibroblast up-regulates production of vascular endothelial growth factor. J Immunol. (2000) 164:5055–61. doi: 10.4049/jimmunol.164.10.5055
121. Cha H, Bae EK, Koh JH, Chai JY, Jeon CH, Ahn KS, et al. Tumor necrosis factor-alpha induces vascular endothelial growth factor-C expression in rheumatoid synoviocytes. J Rheumatol. (2007) 34:16–9.
122. Yoshida S, Ono M, Shono T, Izumi H, Ishibashi T, Suzuki H, et al. Involvement of interleukin-8, vascular endothelial growth factor, and basic fibroblast growth factor in tumor necrosis factor alpha-dependent angiogenesis. Mol Cell Biol. (1997) 17:4015–23. doi: 10.1128/MCB.17.7.4015
123. de Souza L, Malta TM, Kashima Haddad S, Covas DT. Mesenchymal stem cells and pericytes: to what extent are they related? Stem Cells Dev. (2016) 25:1843–52. doi: 10.1089/scd.2016.0109
124. Corselli M, Chen CW, Sun B, Yap S, Rubin JP, Péault B. The tunica adventitia of human arteries and veins as a source of mesenchymal stem cells. Stem Cells Dev. (2012) 21:1299–308. doi: 10.1089/scd.2011.0200
125. Fava R, Olsen NJ, Spencer-Green G, Yeo KT, Yeo TK, Berse B, et al. Vascular permeability factor/endothelial growth factor (VPF/VEGF): accumulation and expression in human synovial fluids and rheumatoid synovial tissue. J Exp Med. (1994) 180:341–6. doi: 10.1084/jem.180.1.341
126. Caplan A. All MSCs are pericytes? Cell Stem Cell. (2008) 3:229–30. doi: 10.1016/j.stem.2008.08.008
127. Feng J, Mantesso A, Sharpe PT. Perivascular cells as mesenchymal stem cells. Expert Opin Biol Ther. (2010) 10:1441–51. doi: 10.1517/14712598.2010.517191
128. Crisan M, Corselli M, Chen WC, Peault B. Perivascular cells for regenerative medicine. J Cell Mol Med. (2012) 16:2851–60. doi: 10.1111/j.1582-4934.2012.01617.x
129. Bergers G, Song S. The role of pericytes in blood-vessel formation and maintenance. Neuro Oncol. (2005) 7:452–64. doi: 10.1215/S1152851705000232
130. Stapor P, Sweat RS, Dashti DC, Betancourt AM, Murfee WL. Pericyte dynamics during angiogenesis: new insights from new identities. J Vasc Res. (2014) 51:163–74. doi: 10.1159/000362276
131. Maziarz A, Kocan B, Bester M, Budzik S, Cholewa M, Ochiya T, et al. How electromagnetic fields can influence adult stem cells: positive and negative impacts. Stem Cell Res Ther. (2016) 7:54. doi: 10.1186/s13287-016-0312-5
132. Gerhardt H, Betsholtz C. Endothelial-pericyte interaction in angiogensis. Cell Tissue Res. (2003) 314:15–23. doi: 10.1007/s00441-003-0745-x
133. Peppiatt C, Howarth C, Mobbs P, Attwell D. Bidirectional control of CNS capillary diameter by pericytes. Nature. (2006) 443:700–4. doi: 10.1038/nature05193
134. Abarbanell A, Coffey AC, Fehrenbacher JW, Beckman DJ, Herrmann JL, Weil B, et al. Directing migration of endothelial progenitor cells with applied DC electric fields. Stem Cell Res. (2012) 8:38–48. doi: 10.1016/j.scr.2011.08.001
135. Ma F, Li W, Li X, Tran BH, Suguro R, Guan R, et al. Novel protective effects of pulsed electromagnetic field ischemia/reperfusion injury rats. Biosci Rep. (2016) 36:e00420. doi: 10.1042/BSR20160082
136. Murray I, West CC, Hardy WR, James AW, Park TS, Nguyen A, et al. Natural history of mesenchymal stem cells, from vessel walls to culture vessels. Cell Mol Life Sci. (2014) 71:1353–74. doi: 10.1007/s00018-013-1462-6
137. Fan W, Qian F, Ma Q, Zhang P, Chen T, Chen C, et al. 50 Hz electromagnetic field exposure promotes proliferation and cytokine production of bone marrow mesenchymal stem cells. Int J Clin Exp Med. (2015) 8:7394–404.
138. Hong K, Cho ML, Min SY, Shin YJ, Yoo SA, Choi JJ, et al. Effect of interleukin-4 on vascular endothelial growth factor production in rheumatoid synovial fibroblasts. Clin Exp Immunol. (2007) 147:573–9. doi: 10.1111/j.1365-2249.2006.03295.x
139. Shin T, Kim HS, Kang TW, Lee BC, Lee HY, Kim YJ, et al. Human umbilical cord blood-stem cells direct macrophage polarization and block inflammasome activation to alleviate rheumatoid arthritis. Cell Death Dis. (2016) 7:e2524. doi: 10.1038/cddis.2016.442
140. McKay J, Prato FS, Thomas AW. The effects of magentic field exposure on blood flow and blood vessels in the microvasculature. bioelectromagnetics. (2007) 28:81–98.
141. Okana H, Tomita N, Ikada Y. Spatial gradient effects of 120 mT static magnetic field on endothelial tubular formation in vitro. Bioelectromagnetics. (2008) 29:233–6. doi: 10.1002/bem.20376
142. Williams C, Markov MS, Hardman WE, Cameron IL. Therapeutic electromagnetic field effects on angiogenesis and tumor growth. Anticancer Res. (2001) 21:3887–91.
143. Baker R. Human magnetoreception for navigation: electromagnetic fields and neurobehavioral function. Prog Clin Biol Res. (1988) 257:63–80.
144. Cook C, Thomas AW, Prato FS. Human electrophysiological and cognitive effects of exposure to ELF magnetic and ELF modulated RF and microwave fields: a review of recent studies. Bioelectromagnetics. (2002) 23:144–57. doi: 10.1002/bem.107
145. Sartucci F, Bonfiglio L, Del Seppia C, Luschi P, Ghione S, Murri L, et al. Changes in pain perception and pain-related somatosensory evoked potentials in humans produced by exposure to oscillating magnetic fields. Brain Res. (1997) 769:362–6. doi: 10.1016/S0006-8993(97)00755-5
146. Shupak N, Prato FS, Thomas AW. Human exposure to a specific pulsed magnetic field: Effects of thermal sensory and pain threshold. Neurosci Lett. (2004) 363:157–62. doi: 10.1016/j.neulet.2004.03.069
147. Li R, Huang JJ, Shi YQ, Hu A, Lu ZY, Weng L, et al. Pulsed electromagnetic field improves postnatal neovascularization in response to hindlimb ischemia. Am J Transl Res. (2015) 7:430–44. doi: 10.1136/heartjnl-2014-307109.20
148. Wang Z, Yang P, Xu H, Qian A, Hu L, Shang P. Inhibitory effects of a gradient static magnetic field on normal angiogenesis. Bioelectromagnetics. (2009) 30:446–53. doi: 10.1002/bem.20501
149. Ross C, Teli T, Harrison B. Electromagnetic field devices and their effect on nociception and peripheral inflammatory pain mechanisms. Altern Ther Health Med. (2016) 22:34–47.
150. Leoci R, Aiudi G, Silvestre F, Lissner E, Lacalandra GM. Effect of pulsed electromagnetic field therapy on prostate volume and vascularity in the treatment of benign prostatic hyperplasia: a pilot study in a canine model. Prostate Cancer Prostatic Dis. (2014) 74:1132–41. doi: 10.1002/pros.22829
151. Tsong T, Liu DS, Chauvin F, Astumian RD. Resonance electroconformational coupling: a proposed mechanism for energy and signal transductions by membrane proteins. Biosci Rep. (1989) 9:13–26. doi: 10.1007/BF01117508
152. Tsong T. Electrical modulation of membrane proteins: enforced conformational oscillations and biologial energy and signal transduction. Annu Rev Biophys Biophys Chem. (1990) 19:83–106. doi: 10.1146/annurev.bb.19.060190.000503
153. Tsong T. Molecular recognition and processing of periodic signals in cells: study of activation of membrane ATPases by alternating electric fields. Biochim Biophys Acta. (1992) 1113:53–70. doi: 10.1016/0304-4157(92)90034-8
154. Liboff A. Geomagnetic cyclotron resonance in living cells. J Biol Phys. (1985) 13:99–104. doi: 10.1007/BF01878387
155. De Ninno A, Pregnolato M. Electromagnetic homeostasis and the role of low-amplitude electromagnetic fields on life organization. Electromagn Biol Med. (2017) 36:115–22. doi: 10.1080/15368378.2016.1194293
156. Buckner C, Buckner AL, Koren SA, Persinger MA, Lafrenie RM. Inhibition of cancer cell growth by exposure to a specific time-varying electromagnetic field involves T-type calcium channels. PLoS ONE. (2015) 10:e0124136. doi: 10.1371/journal.pone.0124136
157. Ikehara T, Yamaguchi H, Miyamoto H. Effects of electromagnetic fields on membrane ion transport of cultured cells. J Med Invest. (1998) 45:47–56.
158. Ross C, Pettenati MJ, Procita J, Cathey L, George SK, Almeida-Porada G. Evaluation of cytotoxic and genotoxic effects of extremely low-frequency electromagnetic field on mesenchymal stromal cells. Glob Adv Health Med. (2018) 7:2164956118777472. doi: 10.1177/2164956118777472
159. Rubik B, Muehsam D, Hammerschlag R, Jain S. Biofield science and healing: history, terminology, and concepts. Glob Adv Health Med. (2015) 4:8–14. doi: 10.7453/gahmj.2015.038.suppl
160. Adravanti P, Nicoletti S, Setti S, Ampollini A, de Girolamo L. Effect of pulsed electromagnetic field therapy in patients undergoing total knee arthroplasty: a randomised controlled trial. Int Orthop. (2014) 38:397–403. doi: 10.1007/s00264-013-2216-7
Keywords: pulsed electromagnetic field (PEMF), rheumatoid arthritis (RA), mesenchymal stromal cells/pericytes (MSCs), osteogenesis, chondrogenesis, angiogenesis
Citation: Ross CL, Ang DC and Almeida-Porada G (2019) Targeting Mesenchymal Stromal Cells/Pericytes (MSCs) With Pulsed Electromagnetic Field (PEMF) Has the Potential to Treat Rheumatoid Arthritis. Front. Immunol. 10:266. doi: 10.3389/fimmu.2019.00266
Received: 27 August 2018; Accepted: 31 January 2019;
Published: 04 March 2019.
Edited by:
Guido Moll, Charité Medical University of Berlin, GermanyReviewed by:
Jérôme Avouac, Université Paris Descartes, FranceRita Consolini, University of Pisa, Italy
Copyright © 2019 Ross, Ang and Almeida-Porada. This is an open-access article distributed under the terms of the Creative Commons Attribution License (CC BY). The use, distribution or reproduction in other forums is permitted, provided the original author(s) and the copyright owner(s) are credited and that the original publication in this journal is cited, in accordance with accepted academic practice. No use, distribution or reproduction is permitted which does not comply with these terms.
*Correspondence: Christina L. Ross, Y2hycm9zc0B3YWtlaGVhbHRoLmVkdQ==