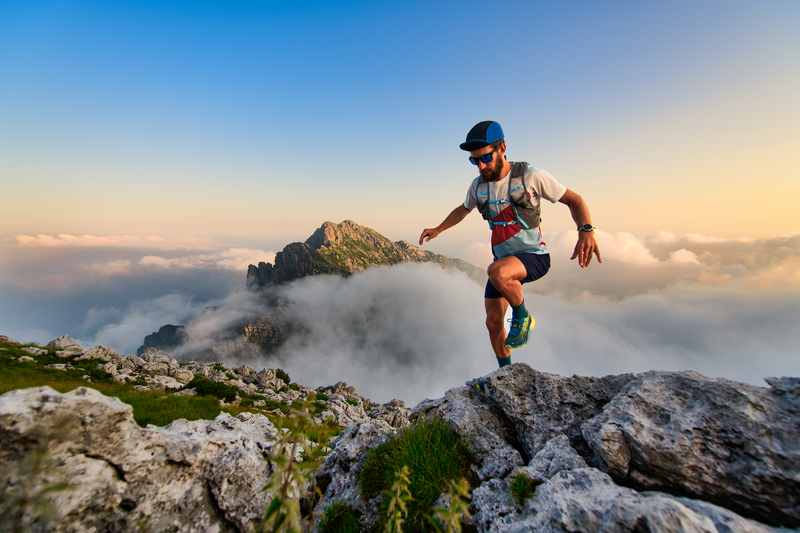
95% of researchers rate our articles as excellent or good
Learn more about the work of our research integrity team to safeguard the quality of each article we publish.
Find out more
REVIEW article
Front. Immunol. , 14 January 2019
Sec. Antigen Presenting Cell Biology
Volume 9 - 2018 | https://doi.org/10.3389/fimmu.2018.03145
This article is part of the Research Topic Looking Beyond Pattern Recognition: Perturbations in Cellular Homeostasis and Metabolism as Emerging Regulators of Dendritic Cell Function View all 11 articles
Dendritic cell (DC) activation is characterized by an acute increase in glucose metabolic flux that is required to fuel the high anabolic rates associated with DC activation. Inhibition of glycolysis significantly attenuates most aspects of DC immune effector function including antigen presentation, inflammatory cytokine production, and T cell stimulatory capacity. The cellular nutrient sensor mammalian/mechanistic Target of Rapamycin (mTOR) is an important upstream regulator of glycolytic metabolism and plays a central role in coordinating DC metabolic changes and immune responses. Because mTOR signaling can be activated by a variety of immunological stimuli, including signaling through the Toll-like Receptor (TLR) family of receptors, mTOR is involved in orchestrating many aspects of the DC metabolic response to microbial stimuli. It has become increasingly clear that mTOR's role in promoting or attenuating inflammatory processes in DCs is highly context-dependent and varies according to specific cellular subsets and the immunological conditions being studied. This review will address key aspects of the complex role of mTOR in regulating DC metabolism and effector function.
As the quintessential professional antigen presenting cells of the immune system, dendritic cells (DCs) play a central role in coordinating both innate and adaptive immune responses through efficient recognition and uptake of extracellular material and the potent ability to provide both presented antigen and costimulatory signals required for proper T lymphocyte activation (1). DC activation is typically initiated by Pattern Recognition Receptor (PRR) interactions with microbe-associated ligands, as has been exhaustively characterized for the Toll-like receptor (TLR) family of innate immune receptors (2–4). Signaling downstream of these receptors induces important transcription and translation programs in DCs that are essential for the induction of their immune effector function. While DCs share many of the innate immune features of other myeloid cells of the mononuclear phagocyte lineage such as the expression of PRRs, efficient endocytic, and phagocytic clearance of extracellular matter, and robust induction of cytokine-driven inflammatory response upon activation, DCs undergo a distinct cellular program of maturation that is affiliated with their important contributions to T cell activation. These latter functions include the processing and presentation of antigens on MHC molecules, the upregulation of co-stimulatory molecule expression, and the induction of chemokine receptor expression that drives DC migration to secondary lymphoid organs where DCs encounter and activate T lymphocytes through cognate antigen interactions (1, 5, 6).
While historically the field of immunology has focused on immune cell regulation at the transcriptional and translational levels, the recent emergence of the field of “immunometabolism” has provided the scientific community with a new framework for thinking about immune cell activation; specifically, how nutrient availability and usage controls the cellular effector functions important for immunological protection. One of the cornerstone findings of recent advances in the field is the observation that immune cell activation, in both the lymphoid and myeloid lineages, is broadly characterized by an increased flux of glucose metabolism, often termed in the literature as “aerobic glycolysis” as both a historical nod to the analogous “Warburg metabolism” described in cancer cells [reviewed in Potter et al. (7)] and to emphasize that the increase in glucose metabolism is not systemically induced by hypoxic conditions (8–18). Analogous to lymphocyte dependence on glucose metabolism for activation [reviewed in (17, 19)], TLR stimulation of DCs induces significant upregulation of aerobic glycolysis that is required for the survival and immune effector function of both human and mouse DCs (10–12, 15, 20, 21). As a cellular nutrient sensor and important upstream regulator of glycolytic metabolism, Mammalian Target of Rapamycin (mTOR) plays a central role in coordinating DC metabolic changes and immune responses. mTOR's role in cell biology is far-reaching and highly complex, regulating a diverse network of cellular responses including cell metabolism, energy homeostasis, protein translation, cellular differentiation, and proliferation, autophagy, and cell survival. Despite this complexity, the existence of highly selective, non-toxic, and FDA-approved mTOR inhibitors such as rapamycin has allowed the research community to broadly interrogate the role of mTOR function in DC biology at both the cellular and organism/patient level. As the role of mTOR in immune cell development and autophagy regulation have been covered comprehensively by previous reviews [reviewed in (22–24)], these aspects of mTOR biology will not be covered in depth. Instead, the focus of this review will be to highlight and discuss the current understanding of mTOR-dependent metabolic regulation of DC function.
The hierarchical regulation of cellular metabolism and energy homeostasis can be functionally partitioned into two opposing “programs,” anabolism and catabolism, each governed by a distinct central upstream regulator. Energetic anabolism, generically characterized by reduced metabolic activity coupled to energy conservation and production, is controlled by AMP-activated protein kinase (AMPK) in response to low cellular ATP levels or nutrient starvation [reviewed in (25)]. Catabolism, contrastingly comprised by high rates of energy expenditure for nutrient breakdown and molecular biosynthesis, is controlled by mTOR complex activity [reviewed in (26)]. Not surprisingly, these two processes cross-regulate each other, most notably by AMPK inhibition of mTOR activation. While AMPK has been implicated in important aspects of DC biology (10, 27, 28), it is a notably understudied aspect of DC metabolic biology and this review will focus primarily on mTOR-mediated metabolic regulation of DCs.
The mTOR protein itself functions as a required component of two major signaling complexes, mTOR complex 1 (mTORC1) and mTOR complex 2 (mTORC2). While mTORC1 is primarily responsible for cellular energy expenditure and protein translation, mTORC2 serves an important role as a positive regulator of mTORC1. For the purposes of this review, “mTOR activity” will refer to mTORC1 functions unless otherwise noted. It is notable that while mTOR promotes sustained catabolism of carbohydrates, it concurrently supports the de novo synthesis of lipids, proteins, and amino acids, serving as an important checkpoint in converting increased cellular fuel consumption into processes such as cell division and protein production that have obvious implications for broad physiological responses, including those carried out by immune cells (29). As a downstream target of the PI3K/Akt signaling axis, mTOR activation in DCs can be initiated by a number of immunologically relevant factors, including cytokine signaling, growth factor signaling, and PRR signaling. In light of this, mTOR is positioned as a critical molecule integrating immunological stimuli into changes in cellular metabolism that regulate protein translation events required for the immunological function of these cells. The role of mTOR in governing immune cell homeostasis and the use of mTOR inhibitors as viable immunoregulatory strategies continue to be of intense interest to the field (23).
Activation of DCs via TLRs promotes significant upregulation of aerobic glycolysis, which regulates the immune function of both human and mouse DCs (10–12, 18, 20, 21, 30, 31). To date, ligands for both MyD88 -dependent and -independent TLR members have been shown to result in an acute upregulation of glycolysis (20), as well as ligands for the C-type Lectin Receptors Dectin-1/2 (21), suggesting that this metabolic reprogramming may be a broadly conserved feature of PRR signaling. A wide variety of approaches, including inhibition of glycolysis through culture with 2-deoxy-glucose (2DG), pharmacological inhibition of glycolysis-regulating signaling pathways, and genetic silencing of rate-limiting glycolysis enzymes, have demonstrated that loss of glycolytic capability significantly impairs DC effector functions, including antigen presentation, co-stimulatory molecule expression, chemotaxis, cytokine secretion, and T lymphocyte stimulatory capacity (10–12, 18, 20, 21, 30, 31). The prevailing consensus has emerged that acute, and in some cases sustained, metabolic commitment to elevated rates of glucose catabolism are an essential metabolic requirement for proper DC activation. We have previously argued that DC metabolic reprogramming can be functionally partitioned into two temporal phases governed by distinct signaling events (32): (1) an acute induction of glycolysis occurring within minutes of TLR activation that supports the high biosynthetic demand associated with early DC maturation for several hours (20); (2) a long-term commitment to glycolysis in subsets of nitric oxide (NO) -producing DCs that is required for their metabolic adaptation to NO-mediated mitochondrial toxicity (12, 15).
Rapid induction of glycolysis in DCs, occurring within minutes of TLR stimulation, is controlled by a PI3K/TBK1/IKKε/Akt signaling axis that promotes the rapid translocation of Hexokinase 2 (HK2) to the mitochondria which supports the rapid flux of glucose catabolism associated with DC maturation (20). Glucose is rapidly consumed by activated DCs and glucose-derived carbons are primarily invested in pentose phosphate pathway (PPP) metabolism, lactate production, and citrate synthesis via the mitochondrial citrate shuttle, the latter presumably supporting fatty acid synthesis associated with endoplasmic reticulum, and Golgi body -dependent translation and secretory pathways that control inflammatory cytokine production (16, 20). The source of glucose that fuels this early activation comes from both the import of extracellular glucose, and the catabolism of intracellular glycogen pools that these cells possess in the resting state (18). The acute induction of glycolysis mediated by the PI3K/TBK1/IKKε/Akt signaling axis, conserved in multiple DC subsets in both mouse and human systems (20, 21, 31), is transient (lasting approximately 6–8 h) after which glycolysis levels gradually wane close to their pre-activation levels (20). The inability of mTOR inhibitors to attenuate this early wave of glycolysis indicates that mTOR activation is positioned downstream of early glycolysis commitment in DCs (12, 15, 20).
While a number of studies have concluded that that mTOR, and one of its downstream transcription factors HIF1α, are required for DC glycolytic reprogramming (9, 30, 33–35), we and others have shown that this is primarily the case for the long-term commitment to glycolysis observed in NO-producing DCs that express inducible nitric oxide synthase (iNOS) and is independent of the acute glycolytic reprogramming events described above (12, 15). In iNOS-expressing DCs, largely restricted to inflammatory monocyte-derived DCs in the mouse and minor subsets of human DCs [previously reviewed in (32)], mTOR–dependent HIF1α activity promotes iNOS expression in TLR-activated DCs (30, 36). iNOS protein expression becomes detectable just as the acute induction of glycolysis begins to wane (12, 15), followed by NO-mediated suppression of DC mitochondrial activity (12, 15) through reversible inhibition of mitochondrial cytochrome c oxidase function (37, 38). Through its regulation of iNOS expression, mTOR regulates the long-term commitment of these cells to glycolytic metabolism in a NO-dependent manner (12, 15). Notably, mTOR inhibition decreases NO production and restores mitochondrial function in iNOS-expressing DCs which leads to increased metabolic flexibility and enhanced inflammatory activity in these cells (11). While the multifaceted and highly complex NO-independent impacts of mTOR on DC function are discussed in more detail below, it is impossible to ignore the important role of mTOR in regulating DC iNOS expression and the implications of this on DC metabolism.
Metabolite tracing studies have shown that the rapid catabolism of glucose in TLR-stimulated DCs is closely linked with a number of biosynthetic pathways including the preferential generation of citrate through the TCA cycle (16, 20). Citrate production is understood to support fatty acid synthesis required for the expansion of endoplasmic reticulum and Golgi body cellular structures associated with DC activation (16, 20, 39, 40). While mTOR signaling is known to promote lipid biosynthesis (29), the explicit role of mTOR in regulating the citrate and fatty acid biosynthesis in stimulated DCs remains poorly defined. Nevertheless, the regulation of lipid metabolism in DCs has clear immunological relevance as there are notable instances where parasite infection of DCs leads to significant changes in lipid metabolism (41). In addition, LPS stimulation leads to specific increases in cellular ceramide concentration in DCs and immunogenic DCs and tolerogenic DCs display unique intracellular lipid profiles (42). With respect to cholesterol lipid metabolism, it is clear that this too is a highly important process in DCs. Liver X receptor, an important regulator of cholesterol metabolism, is implicated in promoting both DC differentiation and immune activation (43). Cholesterol hydroxylase activity is specifically upregulated by Type-I interferon signaling in macrophages and DCs (44), and both PRR and MHC molecules are associated with cholesterol-enriched lipid raft microdomains in the plasma membrane of DCs (45, 46). While it is logical that mTOR activity is involved in regulating these processes based on its role in other cell types, further work delineating mTOR's role in DC lipid metabolism is an important area for future investigation.
Because pharmacological inhibitors of mTOR function attenuate lymphocyte proliferation, mTOR signaling has classically been considered to play a broadly pro-inflammatory role in the immune system and has been used extensively for its systemic tolerogenic properties in the clinic. Recent studies at the cellular level have revealed that mTOR can exert both inflammatory and anti-inflammatory effects depending on the physiological context and cellular subsets in question. A summary of these findings for mTOR's documented impact on various aspects of DC effector function are delineated below.
While some studies have concluded that mTOR inhibition has minimal or contrasting effects on aspects of DC activation (47–49), other studies have argued that mTOR inhibition dramatically influences DC maturation in either a positive or negative direction. Many studies in both the mouse and human system have shown a negative impact on DC maturation (47, 50–52), while others have shown that mTOR inhibition can actually augment DC activation (10, 11, 15, 50). In one study, treatment with 1,25-dihydroxyvitamin D3 was shown to induce an mTOR-dependent tolerogenic phenotype in monocyte-derived human DCs (moDCs) that was characterized by decreased surface expression of CD80, HLA-DR, and CD86, and increased production of IL-10 (53). In mouse bone marrow -derived DCs (BMDCs), shRNA knocking down AMPK, a negative regulator of mTOR activity, increased costimulatory molecule expression while AMPK agonists decreased DC maturation (10). These studies show a rapid de-phosphorylation of AMPK upon LPS stimulation and that IL-10 attenuates LPS-mediated AMPK de-phosphorylation (10). Taken together, these findings are consistent with a model whereby reduced AMPK activity and concomitant mTOR induction serve as a “master switch” to promote DC maturation and immune function. Consistent with this, we and others have shown that CD40 and CD86 expression on mouse BMDCs and subsets of human DCs can be enhanced by mTOR inhibition during TLR stimulation of these cells (11, 15, 50). One of the most informative studies for resolving the published discrepancies on the role of mTOR in DC maturation, published by Haidinger et al. showed that mTOR inhibitors negatively regulate IL-4/GM-CSF -differentiated moDC activation, but augment maturation of freshly isolated myeloid DCs from human peripheral blood (50). In accordance with this, multiple studies have reported that the requirement for mTOR signaling in DC development and function varies with respect to the DC subsets in question (50, 54, 55). This intriguing idea, that mTOR exhibits disparate roles in unique DC subsets is further supported by the finding that different DC subsets engage distinct metabolic signatures to support their specialized function (56, 57). To this point, tolerogenic DCs are reported to exhibit an increased dependence on mitochondrial metabolism, in contrast to the glycolysis-centric phenotype observed for many inflammatory DC subsets (56, 57). The fact that mTOR activator is typically considered an upstream promoter of protein translation, it is interesting that mTOR inhibitors can actually augment proinflammatory molecule production in certain DC subsets (11, 15, 50). This phenomenon is not restricted to the role of mTOR in regulating iNOS expression as it has also been reported for circulating human DCs that do not produce NO upon activation (11, 50).
The production of cytokines has been the most widely studied DC effector function with respect to the role of mTOR in these cells. Freshly isolated mouse splenic pDCs exhibit an mTOR -activated phenotype, and rapamycin-treated mouse and human pDCs show impaired secretion of multiple cytokines including Type I interferons, TNF-alpha, and IL-6 (58). In L. monocytogenes infected mice, rapamycin treatment protected animals from lethal challenge, and led to increased serum concentrations of IL-12p70, IFN-γ, and IL-6 (59). Treatment of human moDCs with the phytochemical cytopiloyne, which is reported to preferentially target mTORC2 signaling, lowers LPS-driven costimulatory molecules expression and inflammatory cytokine production (60). Another study identified a beta-Catenin/mTOR signaling axis as a primary driver of DC IL-10 production responsible for CD8+ T lymphocyte activation (61). Multiple studies have shown that inhibition of mTOR leads to decreased IL-10 production, often concomitant with increased production of inflammatory cytokines such as IL-12 and IL-6 (50, 62). Consistent with this, mTORC1 signaling by intestinal DCs has been shown to be required for IL-10 production and tolerance homeostasis in the gut (62). The connection between mTOR signaling and DC IL-10 production is particularly noteworthy because IL-10 has been shown to antagonize long-term glycolysis commitment in BMDCs (10). Upon LPS stimulation of whole blood from kidney transplant patients treated with rapamycin, IL-12p40, IL-6, TNF-α, and IL-1β levels were increased while IL-10 levels were decreased, compared to patient controls (63). Furthermore, rapamycin treatment of human CD14+ monocytes was shown to enhance IL-12p40, IL-12p70, and TNF-α production, and decrease IL-10 production, upon LPS stimulation (63). Interestingly, in a murine sepsis model, while dexamethasone treatment led to 100% survival, rapamycin treatment led to ~40% survival, and combined treatment led to ~50% survival, providing in vivo evidence that the pro-inflammatory impact of mTOR inhibition by rapamycin supersedes the anti-inflammatory impact of dexamethasone stimulation of the glucocorticoid receptor (63). With respect to cytokine production, there is a fair amount of consistency regarding the role of mTOR-dependent promotion of IL-10 production as an important brake on the inflammatory cytokine output by DCs.
An important prerequisite for a DC's ability to stimulate T cells in vivo is its capacity to traffic to secondary lymphoid organs upon activation. While one study has shown that rapamycin limits DC lymph node trafficking in a mouse psoriasis model (64), other studies have shown no impact of rapamycin treatment on CCR7 expression or in vivo migratory capacity (47). In general, further studies are needed to better define the role of mTOR in regulating DC chemotaxis and migration, particularly given the high metabolic demand that these processes likely require. With regard to mTOR's impact on DC T cell stimulatory capacity, the published literature indicates that this phenotype is highly dependent on the DC subset in question. One study reported that rapamycin treatment enhanced the ability of TLR7-stimulated human pDCs to promote both the proliferation of CD4+ T cells and the induction of T regulatory cells (48), while other studies support the idea that rapamycin treatment globally suppresses DC capacity to stimulate T lymphocytes (49, 53, 58, 65). A more nuanced look at mTOR's role in T lymphocyte activation has shown an important role for mTOR-mediated Th1/Th2 skewing of CD4+ cells, with the majority of studies demonstrating that mTOR preferentially supports Th2 lymphocyte activation, presumably through its function in promoting IL-10 production by DCs (62, 66, 67). Additionally, mTOR inhibition by rapamycin has been shown to promote T regulatory cell induction both in vitro and in vivo through DC-dependent action (68).
In contrast to the studies above, we and other have shown that mTOR inhibition can enhance T cell stimulatory capacity in certain contexts (11, 15, 50, 69). mTORC2 deficiency has been documented to augment CD8+ lymphocyte -mediated graft rejection in mice (70), while mice given autologous DCs simulated with LPS in the presences of rapamycin led to a negative impact on T lymphocyte activation and improved graft vs. host survival (71). In GM-CSF -differentiated BMDCs, both mTOR inhibitors enhance LPS-driven DC activation and T cell stimulatory capacity, at least in part through attenuation of mTOR-dependent nitric oxide generation (11, 15). In multiple mouse models of autologous DC vaccination, rapamycin conditioning of DCs enhanced vaccine efficacy to both melanoma tumor challenge (11) and tuberculosis infection (69). This phenotype is not restricted to mouse cells as freshly isolated CD1c+ human myeloid DCs have also been shown to exhibit enhanced T cell proliferation with rapamycin conditioning (50). Nevertheless, whether or not rapamycin treatment can enhance DC autologous vaccination regimens in humans remains to be determined.
We have previously reviewed the profound impact that DC iNOS expression and NO production has on the metabolism, survival, and immune function of these cells (32). TLR activation induces iNOS expression in mouse BMDCs, and the long-term metabolic commitment to glycolysis in these cells is driven by reversible NO-mediated inhibition of mitochondrial respiration in these cells (12, 15). Furthermore, iNOS inhibition or deletion leads to prolonged post-activation survival and enhanced immunostimulatory capacity in mouse BMDCs (11, 12, 15). Interestingly, several recent studies have shown that mTOR promotion or Leishmania infection can downregulate iNOS expression in macrophages in vivo, suggesting that regulation of iNOS expression may depend critically on complex factors in situ (72, 73). A recent study, investigating the role of innate immune receptor signal strength in modulating DC metabolism and function, showed that stronger stimuli induce higher iNOS expression, NO production, and more dramatic inhibition of mitochondrial respiration (21). In these studies, even though early activation is associated with mTORC1 activity, only strong inflammatory stimuli induce sustained mTORC1 and mTORC2 activation (21). Other studies have suggested that mTOR-driven glycolysis regulates iNOS expression itself (30). These studies showed that both glucose depletion and rapamycin inhibition led to decreased HIF1α and iNOS expression, and promotion of HIF1α activity induced Nos2 (iNOS) mRNA expression (30). Interestingly, a reciprocal relationship between iNOS and HIF1α was observed as iNOS inhibition or deletion also led to diminished HIF1α expression (30). These studies showed that the relationship between mTOR -mediated metabolic changes and iNOS activity is complicated, but defined an anti-inflammatory effect of glucose metabolism on DC immune function that is dependent on an mTOR/HIF1α/iNOS signaling circuit (30). Furthermore, there is evidence to suggest that T cell depletion of local glucose levels in the tissue microenvironment can impact mTOR/HIF1α/iNOS activity (30). While the contribution of mTOR-mediated iNOS expression and function to DC metabolism is striking, it is noteworthy that even in DC subsets that do not produce NO, mTOR inhibition can augment DC immune function (11, 15, 50).
It noteworthy to consider that only specific subsets of DC populations express iNOS in both mice and humans. GM-CSF -differentiated mouse BMDCs classically induce iNOS expression when stimulated by LPS and IFN-γ (74). From an in vivo perspective, monocyte-derived inflammatory DCs (originally termed TNF-a/iNOS-producing-DCs, or “TipDCs”) are potent NO producers and are required to control a number of different types of both bacterial and viral infections (75–77). However, conventional tissue-resident DC subsets in secondary lymphoid organs rarely express iNOS in mice (12, 78, 79), and GM-CSF/IL-4—cultured monocyte-derived human DCs (moDCs) also do not express iNOS (12, 80). Despite these differences, it is clear that human DC populations can express iNOS in vivo including blood circulating CD1a+ DCs (81) and DCs found in psoriatic skin lesions (82–84). Given the heterogeneity of iNOS expression in DC subsets discussed above, it is clearly important to consider the subsets of DCs under investigation when interpreting the literature and this heterogeneity alone may explain key discrepancies among various studies. Nevertheless, even as a relatively rare subset, iNOS-expressing DCs have important immunological and metabolic consequences in the inflammatory tissue microenvironment that is important to consider (32).
While mTOR -dependent NO production has been strongly implicated in promoting DC cell death in iNOS-expressing DC subsets (11, 15), there is significant evidence to suggest that mTOR also plays a role in promoting DC survival in other contexts. Treatment of C57BL/6 splenic CD11c+ mature dendritic cells with AMPK activators, which directly antagonize mTOR activity, leads to increased pro-apoptotic molecule expression (85). In addition, rapamycin treatment of splenic CD11c+ mature dendritic cells increased apoptosis, indicating that mTOR promotes cell survival in this system (85). Interestingly, CCR7 expression induced by DC activation leads to inhibitory phosphorylation of AMPK and subsequent activation of mTOR signaling, which supports the post-activation survival of these cells (85). In further support of mTOR serving as a survival promoter in DCs, rapamycin-treated CD34+ hematopoietic progenitor cells resulted in impaired interstitial DC development, indicating that PI3K/mTOR regulates proliferation and survival (86). In addition, human moDCs treated with rapamycin induces decreased expression of anti-apoptotic protein mcl-1 and drives moDC apoptosis (87).
The process of autophagy, whereby cytoplasmic components are ingested, degraded, and their molecular components recycled, plays important roles in DC antigen presentation [recently reviewed in (24)]. It has been elegantly shown that autophagy is a constitutive process in DCs that actively contributes endogenous peptide antigens to MHC-II complexes in the resting state (88). Upon TLR stimulation, the increase in mTOR activity inhibits the formation of the autophagy initiation complex, thereby restricting autophagy rates from the basal state (89). Experts in the field have argued that mTOR-dependent autophagy inhibition leads to a decreased emphasis on endogenous antigen presentation and increased presentation of exogenous antigen (22, 89). In support of this model, IL-4 -mediated induction of autophagy has been shown to augment endogenous antigen presentation on MHC-II molecules (90). Interestingly, while mTOR attenuates autophagy-dependent contributions to antigen presentation, it concomitantly promotes the presentation of exogenously acquired antigens by supporting lysosome acidification and endolysosomal trafficking of MHC-II/peptide complexes to the cell surface (91–93). These findings have clear clinical significance as kidney transplant patients on mTOR-inhibitor therapy exhibit higher levels of alloreactive T cells, possibly due to enhanced autophagy-dependent presentation of donor-endogenous antigen (94). While it seems clear that activated DCs downregulate autophagy in an mTOR -dependent manner, the contribution of autophagy to the nutrient compartment of resting DCs and its counter-regulation by AMPK remains an underexplored topic.
Given the importance of metabolic changes in supporting the immune activation of DCs, it is not surprising that the central metabolic regulator mTOR plays a critical role in coordinating activation-associated changes in DC metabolism and function (Figure 1). While mTOR has well-documented impacts on DC development, immune effector function, and survival, the challenge in the field rests in understanding the complex and nuanced role that mTOR plays in distinct DC subsets and specific immunological contexts. To this point, it is evident that mTOR can influence DC biology in either a pro-inflammatory or anti-inflammatory direction, which can complicate the interpretation of data where global inhibition of mTOR is employed. Significant aspects of mTOR-mediated regulation of DC biology that would benefit from further investigation include the role of mTOR in nutrient flux in both basal and activated conditions, the cross-regulation of these processes by AMPK, the contribution of mTOR signaling to lipid metabolism, and a further delineation of differential mTOR signaling in distinct DC subsets in both mouse and human cells. We look forward with interest to the ongoing work in the field that will help resolve some of these discrepancies and better clarify the distinct contribution of mTOR signaling to the heterogeneous family of DCs in the mammalian immune system.
Figure 1. Model highlighting major pathways reported to be regulated either directly or indirectly by mTOR in DC biology.
All authors listed have made a substantial, direct and intellectual contribution to the work, and approved it for publication.
Funding from the NIH NIAID 1R21AI135385-01A1 grant (EA) supported this work.
The authors declare that the research was conducted in the absence of any commercial or financial relationships that could be construed as a potential conflict of interest.
1. Banchereau J, Steinman RM. Dendritic cells and the control of immunity. Nature (1998) 392:245–52. doi: 10.1038/32588
2. Barton GM, Medzhitov R. Control of adaptive immune responses by Toll-like receptors. Curr Opin Immunol. (2002) 14:380–3. doi: 10.1016/S0952-7915(02)00343-6
3. Akira S, Takeda K. Toll-like receptor signalling. Nat Rev Immunol. (2004) 4:499–511. doi: 10.1038/nri1391
4. Amati L, Pepe M, Passeri ME, Mastronardi ML, Jirillo E, Covelli V. Toll-like receptor signaling mechanisms involved in dendritic cell activation: potential therapeutic control of T cell polarization. Curr Pharm Design (2006) 12:4247–54. doi: 10.2174/138161206778743583
5. Lipscomb MF, Masten BJ. Dendritic cells: immune regulators in health and disease. Physiol Rev. (2002) 82:97–130.
6. Lee HK, Iwasaki A. Innate control of adaptive immunity: dendritic cells and beyond. Semin Immunol. (2007) 19:48–55. doi: 10.1016/j.smim.2006.12.001
7. Potter M, Newport E, Morten KJ. The Warburg effect: 80 years on. Biochem Soc Trans. (2016) 44:1499–505. doi: 10.1042/BST20160094
8. Fox CJ, Hammerman PS, Thompson CB. Fuel feeds function: energy metabolism and the T-cell response. Nat Rev Immunol. (2005) 5:844–52. doi: 10.1038/nri1710
9. Jantsch J, Chakravortty D, Turza N, Prechtel AT, Buchholz B, Gerlach RG, et al. Hypoxia and hypoxia-inducible factor-1 alpha modulate lipopolysaccharide-induced dendritic cell activation and function. J Immunol. (2008) 180:4697–705. doi: 10.4049/jimmunol.180.7.4697
10. Krawczyk CM, Holowka T, Sun J, Blagih J, Amiel E, DeBerardinis RJ, et al. Toll-like receptor-induced changes in glycolytic metabolism regulate dendritic cell activation. Blood (2010) 115:4742–9. doi: 10.1182/blood-2009-10-249540
11. Amiel E, Everts B, Freitas TC, King IL, Curtis JD, Pearce EL, et al. Inhibition of mechanistic target of rapamycin promotes dendritic cell activation and enhances therapeutic autologous vaccination in mice. J Immunol. (2012) 189:2151–8. doi: 10.4049/jimmunol.1103741
12. Everts B, Amiel E, van der Windt GJ, Freitas TC, Chott R, Yarasheski KE, et al. Commitment to glycolysis sustains survival of NO-producing inflammatory dendritic cells. Blood (2012) 120:1422–31. doi: 10.1182/blood-2012-03-419747
13. Delmastro-Greenwood MM, Piganelli JD. Changing the energy of an immune response. Am J Clin Exp Immunol. (2013) 2:30–54.
14. Pearce EL, Pearce EJ. Metabolic pathways in immune cell activation and quiescence. Immunity (2013) 38:633–43. doi: 10.1016/j.immuni.2013.04.005
15. Amiel E, Everts B, Fritz D, Beauchamp S, Ge B, Pearce EL, et al. Mechanistic target of rapamycin inhibition extends cellular lifespan in dendritic cells by preserving mitochondrial function. J Immunol. (2014) 193:2821–30. doi: 10.4049/jimmunol.1302498
16. Everts B, Pearce EJ. Metabolic control of dendritic cell activation and function: recent advances and clinical implications. Front Immunol. (2014) 5:203. doi: 10.3389/fimmu.2014.00203
17. Pearce EJ, Everts B. Dendritic cell metabolism. Nat Rev Immunol. (2015) 15:18–29. doi: 10.1038/nri3771
18. Thwe PM, Pelgrom L, Cooper R, Beauchamp S, Reisz JA, D'Alessandro A, et al. Cell-intrinsic glycogen metabolism supports early glycolytic reprogramming required for dendritic cell immune responses. Cell Metab. (2017) 26:558–67 e555. doi: 10.1016/j.cmet.2017.08.012
19. van der Windt GJ, Pearce EL. Metabolic switching and fuel choice during T-cell differentiation and memory development. Immunol Rev. (2012) 249:27–42. doi: 10.1111/j.1600-065X.2012.01150.x
20. Everts B, Amiel E, Huang SC, Smith AM, Chang CH, Lam WY, et al. TLR-driven early glycolytic reprogramming via the kinases TBK1-IKKvarepsilon supports the anabolic demands of dendritic cell activation. Nat Immunol. (2014) 15:323–32. doi: 10.1038/ni.2833
21. Guak H, Al Habyan S, Ma EH, Aldossary H, Al-Masri M, Won SY, et al. Glycolytic metabolism is essential for CCR7 oligomerization and dendritic cell migration. Nat Commun. (2018) 9:2463. doi: 10.1038/s41467-018-04804-6
22. Sukhbaatar N, Hengstschlager M, Weichhart T. mTOR-mediated regulation of dendritic cell differentiation and function. Trends Immunol. (2016) 37:778–89. doi: 10.1016/j.it.2016.08.009
23. Jones RG, Pearce EJ. MenTORing Immunity: mTOR signaling in the development and function of tissue-resident immune cells. Immunity (2017) 46:730–42. doi: 10.1016/j.immuni.2017.04.028
24. Ghislat G, Lawrence T. Autophagy in dendritic cells. Cell Mol Immunol. (2018) 15:944–52. doi: 10.1038/cmi.2018.2
25. Herzig S, Shaw RJ. AMPK: guardian of metabolism and mitochondrial homeostasis. Nat Rev Mol Cell Biol. (2018) 19:121–35. doi: 10.1038/nrm.2017.95
26. Sengupta S, Peterson TR, Sabatini DM. Regulation of the mTOR complex 1 pathway by nutrients, growth factors, and stress. Mol Cell (2010) 40:310–22. doi: 10.1016/j.molcel.2010.09.026
27. Nurbaeva MK, Schmid E, Szteyn K, Yang W, Viollet B, Shumilina E, et al. Enhanced Ca(2)(+) entry and Na+/Ca(2)(+) exchanger activity in dendritic cells from AMP-activated protein kinase-deficient mice. Faseb J. (2012) 26:3049–58. doi: 10.1096/fj.12-204024
28. Nieves W, Hung LY, Oniskey TK, Boon L, Foretz M, Viollet B, et al. Myeloid-restricted AMPKalpha1 promotes host immunity and protects against IL-12/23p40-dependent lung injury during hookworm infection. J Immunol. (2016) 196:4632–40. doi: 10.4049/jimmunol.1502218
29. Saxton RA, Sabatini DM. mTOR signaling in growth, metabolism, and disease. Cell (2017) 169:361–71. doi: 10.1016/j.cell.2017.03.035
30. Lawless SJ, Kedia-Mehta N, Walls JF, McGarrigle R, Convery O, Sinclair LV, et al. Glucose represses dendritic cell-induced T cell responses. Nat Commun. (2017) 8:15620. doi: 10.1038/ncomms15620
31. Perrin-Cocon L, Aublin-Gex A, Diaz O, Ramière C, Peri F, André P, et al. Toll-like receptor 4–induced glycolytic burst in human monocyte-derived dendritic cells results from p38-dependent stabilization of HIF-1α and increased hexokinase II expression. J Immunol. (2018) 201:1510–21. doi: 10.4049/jimmunol.1701522
32. Thwe PM, Amiel E. The role of nitric oxide in metabolic regulation of Dendritic cell immune function. Cancer Lett. (2018) 412:236–42. doi: 10.1016/j.canlet.2017.10.032
33. Jantsch J, Wiese M, Schodel J, Castiglione K, Glasner J, Kolbe S, et al. Toll-like receptor activation and hypoxia use distinct signaling pathways to stabilize hypoxia-inducible factor 1alpha (HIF1A) and result in differential HIF1A-dependent gene expression. J Leukoc Biol. (2011) 90:551–62. doi: 10.1189/jlb.1210683
34. Fliesser M, Morton CO, Bonin M, Ebel F, Hunniger K, Kurzai O, et al. Hypoxia-inducible factor 1alpha modulates metabolic activity and cytokine release in anti-Aspergillus fumigatus immune responses initiated by human dendritic cells. Int J Med Microbiol. (2015) 305:865–73. doi: 10.1016/j.ijmm.2015.08.036
35. Linke M, Fritsch SD, Sukhbaatar N, Hengstschlager M, Weichhart T. mTORC1 and mTORC2 as regulators of cell metabolism in immunity. FEBS Lett. (2017) 591:3089–103. doi: 10.1002/1873-3468.12711
36. Harris AJ, Thompson AR, Whyte MK, Walmsley SR. HIF-mediated innate immune responses: cell signaling and therapeutic implications. Hypoxia (2014) 2:47–58. doi: 10.2147/HP.S50269
37. Clementi E, Brown GC, Feelisch M, Moncada S. Persistent inhibition of cell respiration by nitric oxide: crucial role of S-nitrosylation of mitochondrial complex I and protective action of glutathione. Proc Natl Acad Sci USA. (1998) 95:7631–6.
38. Barone MC, Darley-Usmar VM, Brookes PS. Reversible inhibition of cytochrome c oxidase by peroxynitrite proceeds through ascorbate-dependent generation of nitric oxide. J Biol Chem. (2003) 278:27520–4. doi: 10.1074/jbc.M304129200
39. Rehman A, Hemmert KC, Ochi A, Jamal M, Henning JR, Barilla R, et al. Role of fatty-acid synthesis in dendritic cell generation and function. J Immunol. (2013) 190:4640–9. doi: 10.4049/jimmunol.1202312
40. O'Neill LA, Pearce EJ. Immunometabolism governs dendritic cell and macrophage function. J Exp Med. (2016) 213:15–23. doi: 10.1084/jem.20151570
41. Lecoeur H, Giraud E, Prevost MC, Milon G, Lang T. Reprogramming neutral lipid metabolism in mouse dendritic leucocytes hosting live Leishmania amazonensis amastigotes. PLoS Negl Trop Dis. (2013) 7:e2276. doi: 10.1371/journal.pntd.0002276
42. Ocana-Morgner C, Sales S, Rothe M, Shevchenko A, Jessberger R. Tolerogenic versus immunogenic lipidomic profiles of CD11c(+) immune cells and control of immunogenic dendritic cell ceramide dynamics. J Immunol. (2017) 198:4360–72. doi: 10.4049/jimmunol.1601928
43. Zhong L, Yang Q, Xie W, Zhou J. Liver X receptor regulates mouse GM-CSF-derived dendritic cell differentiation in vitro. Mol Immunol. (2014) 60:32–43. doi: 10.1016/j.molimm.2014.03.006
44. Park K, Scott AL. Cholesterol 25-hydroxylase production by dendritic cells and macrophages is regulated by type I interferons. J Leukoc Biol. (2010) 88:1081–7. doi: 10.1189/jlb.0610318
45. Xu S, Huo J, Gunawan M, Su IH, Lam KP. Activated dectin-1 localizes to lipid raft microdomains for signaling and activation of phagocytosis and cytokine production in dendritic cells. J Biol Chem. (2009) 284:22005–11. doi: 10.1074/jbc.M109.009076
46. Bosch B, Heipertz EL, Drake JR, Roche PA. Major histocompatibility complex (MHC) class II-peptide complexes arrive at the plasma membrane in cholesterol-rich microclusters. J Biol Chem. (2013) 288:13236–42. doi: 10.1074/jbc.M112.442640
47. Reichardt W, Durr C, von Elverfeldt D, Juttner E, Gerlach UV, Yamada M, et al. Impact of mammalian target of rapamycin inhibition on lymphoid homing and tolerogenic function of nanoparticle-labeled dendritic cells following allogeneic hematopoietic cell transplantation. J Immunol. (2008) 181:4770–9. doi: 10.4049/jimmunol.181.7.4770
48. Boor PP, Metselaar HJ, Mancham S, van der Laan LJ, Kwekkeboom J. Rapamycin has suppressive and stimulatory effects on human plasmacytoid dendritic cell functions. Clin Exp Immunol. (2013) 174:389–401. doi: 10.1111/cei.12191
49. do Nascimento de Freitas D, Gassen RB, Fazolo T, Souza APD. Rapamycin increases RSV RNA levels and survival of RSV-infected dendritic cell depending on T cell contact. Toxicol In Vitro (2016) 36:114–9. doi: 10.1016/j.tiv.2016.07.016
50. Haidinger M, Poglitsch M, Geyeregger R, Kasturi S, Zeyda M, Zlabinger GJ, et al. A versatile role of mammalian target of rapamycin in human dendritic cell function and differentiation. J Immunol. (2010) 185:3919–31. doi: 10.4049/jimmunol.1000296
51. Raich-Regue D, Rosborough BR, Watson AR, McGeachy MJ, Turnquist HR, Thomson AW. mTORC2 deficiency in myeloid dendritic cells enhances their allogeneic Th1 and Th17 stimulatory ability after TLR4 ligation in vitro and in vivo. J Immunol. (2015) 194:4767–76. doi: 10.4049/jimmunol.1402551
52. Cheng M, Hu S, Wang Z, Pei Y, Fan R, Liu X, et al. Inhibition of neddylation regulates dendritic cell functions via Deptor accumulation driven mTOR inactivation. Oncotarget (2016) 7:35643–54. doi: 10.18632/oncotarget.9543
53. Ferreira GB, Vanherwegen AS, Eelen G, Gutierrez AC, Van Lommel L, Marchal K, et al. Vitamin D3 induces tolerance in human dendritic cells by activation of intracellular metabolic pathways. Cell Rep. (2015). 10:P711–25. doi: 10.1016/j.celrep.2015.01.013
54. Sathaliyawala T, O'Gorman WE, Greter M, Bogunovic M, Konjufca V, Hou ZE, et al. Mammalian target of rapamycin controls dendritic cell development downstream of Flt3 ligand signaling. Immunity (2010) 33:597–606. doi: 10.1016/j.immuni.2010.09.012
55. Biswas M, Sarkar D, Kumar SR, Nayak S, Rogers GL, Markusic DM, et al. Synergy between rapamycin and FLT3 ligand enhances plasmacytoid dendritic cell-dependent induction of CD4+CD25+FoxP3+ Treg. Blood (2015) 125:2937–47. doi: 10.1182/blood-2014-09-599266
56. Malinarich F, Duan K, Hamid RA, Bijin A, Lin WX, Poidinger M, et al. High mitochondrial respiration and glycolytic capacity represent a metabolic phenotype of human tolerogenic dendritic cells. J Immunol. (2015) 194:5174–86. doi: 10.4049/jimmunol.1303316
57. Sim WJ, Ahl PJ, Connolly JE. Metabolism is central to tolerogenic dendritic cell function. Mediators Inflamm. (2016) 2016:2636701. doi: 10.1155/2016/2636701
58. Cao W, Manicassamy S, Tang H, Kasturi SP, Pirani A, Murthy N, et al. Toll-like receptor-mediated induction of type I interferon in plasmacytoid dendritic cells requires the rapamycin-sensitive PI(3)K-mTOR-p70S6K pathway. Nat Immunol. (2008) 9:1157–64. doi: 10.1038/ni.1645
59. Weichhart T, Costantino G, Poglitsch M, Rosner M, Zeyda M, Stuhlmeier KM, et al. The TSC-mTOR signaling pathway regulates the innate inflammatory response. Immunity (2008) 29:565–77. doi: 10.1016/j.immuni.2008.08.012
60. Wei WC, Liu CP, Yang WC, Shyur LF, Sheu JH, Chen SS, et al. Mammalian target of rapamycin complex 2 (mTORC2) regulates LPS-induced expression of IL-12 and IL-23 in human dendritic cells. J Leukoc Biol. (2015) 97:1071–80. doi: 10.1189/jlb.2A0414-206RR
61. Fu C, Liang X, Cui W, Ober-Blobaum JL, Vazzana J, Shrikant PA, et al. beta-Catenin in dendritic cells exerts opposite functions in cross-priming and maintenance of CD8+ T cells through regulation of IL-10. Proc Natl Acad Sci USA. (2015) 112:2823–8. doi: 10.1073/pnas.1414167112
62. Ohtani M, Hoshii T, Fujii H, Koyasu S, Hirao A, Matsuda S. Cutting edge: mTORC1 in intestinal CD11c+ CD11b+ dendritic cells regulates intestinal homeostasis by promoting IL-10 production. J Immunol. (2012) 188:4736–40. doi: 10.4049/jimmunol.1200069
63. Weichhart T, Haidinger M, Katholnig K, Kopecky C, Poglitsch M, Lassnig C, et al. Inhibition of mTOR blocks the anti-inflammatory effects of glucocorticoids in myeloid immune cells. Blood (2011) 117:4273–83. doi: 10.1182/blood-2010-09-310888
64. Burger C, Shirsath N, Lang V, Diehl S, Kaufmann R, Weigert A, et al. Blocking mTOR signalling with rapamycin ameliorates imiquimod-induced psoriasis in mice. Acta Derm Venereol. (2017) 97:1087–94. doi: 10.2340/00015555-2724
65. Turnquist HR, Cardinal J, Macedo C, Rosborough BR, Sumpter TL, Geller DA, et al. mTOR and GSK-3 shape the CD4+ T-cell stimulatory and differentiation capacity of myeloid DCs after exposure to LPS. Blood (2010) 115:4758–69. doi: 10.1182/blood-2009-10-251488
66. Hussaarts L, Smits HH, Schramm G, van der Ham AJ, van der Zon GC, Haas H, et al. Rapamycin and omega-1: mTOR-dependent and -independent Th2 skewing by human dendritic cells. Immunol Cell Biol. (2013) 91:486–9. doi: 10.1038/icb.2013.31
67. Mineharu Y, Kamran N, Lowenstein PR, Castro MG. Blockade of mTOR signaling via rapamycin combined with immunotherapy augments antiglioma cytotoxic and memory T-cell functions. Mol Cancer Ther. (2014) 13:3024–36. doi: 10.1158/1535-7163.Mct-14-0400
68. Pothoven KL, Kheradmand T, Yang Q, Houlihan JL, Zhang H, Degutes M, et al. Rapamycin-conditioned donor dendritic cells differentiate CD4CD25Foxp3 T cells in vitro with TGF-beta1 for islet transplantation. Am J Transpl. (2010) 10:1774–84. doi: 10.1111/j.1600-6143.2010.03199.x
69. Jagannath C, Bakhru P. Rapamycin-induced enhancement of vaccine efficacy in mice. Methods Mol Biol. (2012) 821:295–303. doi: 10.1007/978-1-61779-430-8_18
70. Watson AR, Dai H, Diaz-Perez JA, Killeen ME, Mathers AR, Thomson AW. mTORC2 deficiency in cutaneous dendritic cells potentiates CD8(+) effector T cell responses and accelerates skin graft rejection. Am J Transpl. (2018). doi: 10.1111/ajt.15083. [Epub ahead of print].
71. Stenger EO, Rosborough BR, Mathews LR, Ma H, Mapara MY, Thomson AW, et al. IL-12hi rapamycin-conditioned dendritic cells mediate IFN-gamma-dependent apoptosis of alloreactive CD4+ T cells in vitro and reduce lethal graft-versus-host disease. Biol Blood Marrow Transpl. (2014) 20:192–201. doi: 10.1016/j.bbmt.2013.11.007
72. Linke M, Pham HT, Katholnig K, Schnoller T, Miller A, Demel F, et al. Chronic signaling via the metabolic checkpoint kinase mTORC1 induces macrophage granuloma formation and marks sarcoidosis progression. Nat Immunol. (2017b) 18:293–302. doi: 10.1038/ni.3655
73. Kumar A, Das S, Mandal A, Verma S, Abhishek K, Kumar A, et al. Leishmania infection activates host mTOR for its survival by M2 macrophage polarization. Parasite Immunol. (2018) 40:e12586. doi: 10.1111/pim.12586
74. Lu L, Bonham CA, Chambers FG, Watkins SC, Hoffman RA, Simmons RL, et al. Induction of nitric oxide synthase in mouse dendritic cells by IFN-gamma, endotoxin, and interaction with allogeneic T cells: nitric oxide production is associated with dendritic cell apoptosis. J Immunol. (1996) 157:3577–86
75. Serbina NV, Salazar-Mather TP, Biron CA, Kuziel WA, Pamer EG. TNF/iNOS-producing dendritic cells mediate innate immune defense against bacterial infection. Immunity (2003) 19:59–70. doi: 10.1016/S1074-7613(03)00171-7
76. Aldridge JR Jr, Moseley CE, Boltz DA, Negovetich NJ, Reynolds C, Franks J, et al. TNF/iNOS-producing dendritic cells are the necessary evil of lethal influenza virus infection. Proc Natl Acad Sci USA (2009) 106:5306–11. doi: 10.1073/pnas.0900655106
77. De Trez C, Magez S, Akira S, Ryffel B, Carlier Y, Muraille E. iNOS-producing inflammatory dendritic cells constitute the major infected cell type during the chronic Leishmania major infection phase of C57BL/6 resistant mice. PLoS Pathog. (2009) 5:e1000494. doi: 10.1371/journal.ppat.1000494
78. Nishioka Y, Wen H, Mitani K, Robbins PD, Lotze MT, Sone S, et al. Differential effects of IL-12 on the generation of alloreactive CTL mediated by murine and human dendritic cells: a critical role for nitric oxide. J Leukoc Biol. (2003) 73:621–9. doi: 10.1189/jlb.0402205
79. Powell TJ, Jenkins CD, Hattori R, MacPherson GG. Rat bone marrow-derived dendritic cells, but not ex vivo dendritic cells, secrete nitric oxide and can inhibit T-cell proliferation. Immunology (2003) 109:197–208. doi: 10.1046/j.1365-2567.2003.01639.x
80. Padgett EL, Pruett SB. Evaluation of nitrite production by human monocyte-derived macrophages. Biochem Biophys Res Commun. (1992) 186:775–81.
81. Huang YM, Xiao BG, Westerlund I, Link H. Phenotypic and functional properties of dendritic cells isolated from human peripheral blood in comparison with mononuclear cells and T cells. Scand J Immunol. (1999) 49:177–83.
82. Lowes MA, Chamian F, Abello MV, Fuentes-Duculan J, Lin SL, Nussbaum R, et al. Increase in TNF-alpha and inducible nitric oxide synthase-expressing dendritic cells in psoriasis and reduction with efalizumab (anti-CD11a). Proc Natl Acad Sci USA. (2005) 102:19057–62. doi: 10.1073/pnas.0509736102
83. Zaba LC, Cardinale I, Gilleaudeau P, Sullivan-Whalen M, Suarez-Farinas M, Fuentes-Duculan J, et al. Amelioration of epidermal hyperplasia by TNF inhibition is associated with reduced Th17 responses. J Exp Med. (2007) 204:3183–94. doi: 10.1084/jem.20071094
84. Haider AS, Lowes MA, Suarez-Farinas M, Zaba LC, Cardinale I, Khatcherian A, et al. Identification of cellular pathways of “type 1,” Th17 T cells, and TNF- and inducible nitric oxide synthase-producing dendritic cells in autoimmune inflammation through pharmacogenomic study of cyclosporine A in psoriasis. J Immunol. (2008) 180:1913–20. doi: 10.4049/jimmunol.180.3.1913
85. Lopez-Cotarelo P, Escribano-Diaz C, Gonzalez-Bethencourt IL, Gomez-Moreira C, Deguiz ML, Torres-Bacete J, et al. A novel MEK-ERK-AMPK signaling axis controls chemokine receptor CCR7-dependent survival in human mature dendritic cells. J Biol Chem. (2015) 290:827–40. doi: 10.1074/jbc.M114.596551
86. van de Laar L, Buitenhuis M, Wensveen FM, Janssen HL, Coffer PJ, and Woltman AM. Human CD34-derived myeloid dendritic cell development requires intact phosphatidylinositol 3-kinase-protein kinase B-mammalian target of rapamycin signaling. J Immunol. (2010) 184:6600–11. doi: 10.4049/jimmunol.0903089
87. Woltman AM, van der Kooij SW, Coffer PJ, Offringa R, Daha MR, van Kooten C. Rapamycin specifically interferes with GM-CSF signaling in human dendritic cells, leading to apoptosis via increased p27KIP1 expression. Blood (2003) 101:1439–45. doi: 10.1182/blood-2002-06-1688
88. Schmid D, Pypaert M, Munz C. Antigen-loading compartments for major histocompatibility complex class II molecules continuously receive input from autophagosomes. Immunity (2007) 26:79–92. doi: 10.1016/j.immuni.2006.10.018
89. Deretic V. Autophagy as an innate immunity paradigm: expanding the scope and repertoire of pattern recognition receptors. Curr Opin Immunol. (2012) 24:21–31. doi: 10.1016/j.coi.2011.10.006
90. Terawaki S, Camosseto V, Prete F, Wenger T, Papadopoulos A, Rondeau C, et al. RUN and FYVE domain-containing protein 4 enhances autophagy and lysosome tethering in response to Interleukin-4. J Cell Biol. (2015) 210:1133–52. doi: 10.1083/jcb.201501059
91. Vyas JM, Kim YM, Artavanis-Tsakonas K, Love JC, Van der Veen AG, Ploegh HL. Tubulation of class II MHC compartments is microtubule dependent and involves multiple endolysosomal membrane proteins in primary dendritic cells. J Immunol. (2007) 178:7199–210. doi: 10.4049/jimmunol.178.11.7199
92. Liberman R, Bond S, Shainheit MG, Stadecker MJ, Forgac M. Regulated assembly of vacuolar ATPase is increased during cluster disruption-induced maturation of dendritic cells through a phosphatidylinositol 3-kinase/mTOR-dependent pathway. J Biol Chem. (2014) 289:1355–63. doi: 10.1074/jbc.M113.524561
93. Saric A, Hipolito VE, Kay JG, Canton J, Antonescu CN, Botelho RJ. mTOR controls lysosome tubulation and antigen presentation in macrophages and dendritic cells. Mol Biol Cell (2016) 27:321–33. doi: 10.1091/mbc.E15-05-0272
Keywords: dendritic cell (DC), mTOR, immune metabolism, glycolysis, metabolism regulation
Citation: Snyder JP and Amiel E (2019) Regulation of Dendritic Cell Immune Function and Metabolism by Cellular Nutrient Sensor Mammalian Target of Rapamycin (mTOR). Front. Immunol. 9:3145. doi: 10.3389/fimmu.2018.03145
Received: 14 October 2018; Accepted: 19 December 2018;
Published: 14 January 2019.
Edited by:
Bart Everts, Leiden University Medical Center, NetherlandsReviewed by:
Thomas Weichhart, Medical University of Vienna, AustriaCopyright © 2019 Snyder and Amiel. This is an open-access article distributed under the terms of the Creative Commons Attribution License (CC BY). The use, distribution or reproduction in other forums is permitted, provided the original author(s) and the copyright owner(s) are credited and that the original publication in this journal is cited, in accordance with accepted academic practice. No use, distribution or reproduction is permitted which does not comply with these terms.
*Correspondence: Eyal Amiel, RXlhbC5BbWllbEBtZWQudXZtLmVkdQ==
Disclaimer: All claims expressed in this article are solely those of the authors and do not necessarily represent those of their affiliated organizations, or those of the publisher, the editors and the reviewers. Any product that may be evaluated in this article or claim that may be made by its manufacturer is not guaranteed or endorsed by the publisher.
Research integrity at Frontiers
Learn more about the work of our research integrity team to safeguard the quality of each article we publish.