- 1Department of Radiation Oncology, University Hospital Tuebingen, Tuebingen, Germany
- 2Department of General Pediatrics/Pediatric Oncology, University Hospital Tuebingen, Tuebingen, Germany
- 3Dr. Margarete Fischer-Bosch-Institute of Clinical Pharmacology, Stuttgart, Germany
- 4Department of Radiation Oncology, University of Brescia, Brescia, Italy
- 5Departments of Clinical Pharmacology, Pharmacy and Biochemistry, University Hospital and University Tuebingen, Tuebingen, Germany
Cancer immunotherapy has been established as standard of care in different tumor entities. After the first reports on synergistic effects with radiotherapy and the induction of abscopal effects—tumor shrinkage outside the irradiated volume attributed to immunological effects of radiotherapy—several treatment combinations have been evaluated. Different immunotherapy strategies (e.g., immune checkpoint inhibition, vaccination, cytokine based therapies) have been combined with local tumor irradiation in preclinical models. Clinical trials are ongoing in different cancer entities with a broad range of immunotherapeutics and radiation schedules. SDF-1 (CXCL12)/CXCR4 signaling has been described to play a major role in tumor biology, especially in hypoxia adaptation, metastasis and migration. Local tumor irradiation is a known inducer of SDF-1 expression and release. CXCR4 also plays a major role in immunological processes. CXCR4 antagonists have been approved for the use of hematopoietic stem cell mobilization from the bone marrow. In addition, several groups reported an influence of the SDF-1/CXCR4 axis on intratumoral immune cell subsets and anti-tumor immune response. The aim of this review is to merge the knowledge on the role of SDF-1/CXCR4 in tumor biology, radiotherapy and immunotherapy of cancer and in combinatorial approaches.
Introduction
In radiation oncology, chemokine receptor CXCR4 and its ligand SDF-1 (stromal cell derived factor-1, CXCL12) have been described as prognostic factor for head and neck squamous cell carcinoma [e.g., (1)]. Functional data in glioblastoma models point to a role in migration and invasion of cancer cells (2). These and other observations strongly suggest SDF-1/CXCR4 signaling as promising target in anti-cancer therapy, in particular, in combination with radiation therapy (3). However, clinical development of CXCR4 antagonists has mainly focussed on mobilization of hematopoietic stem cells from the bone marrow to peripheral blood (4).
Radiation therapy has proven to elicit both pro-inflammatory, immunostimulatory activities, and anti-inflammatory, immunosuppressive mechanisms. These effects are dependent on radiation dose, tumor biology and the host predisposition (5). As immunotherapy for cancer has been established as standard of care for several cancer entities, such as melanoma (6) and lung cancer (7), the immunologic effects of standard anti-cancer treatment, such as radiation therapy and targeted therapies are of major interest. Radiation-induced immune modulation has been described as direct effects on irradiated tumor cells (“on-target” immunogenic effects) as well as indirect effects in the tumor immune microenvironment (“off-target” effects) (8). Remarkably, recent data also link CXCR4 blockade with antitumor immunity in the tumor immune microenvironment suggesting SDF-1/CXCR4-targeting as a therapeutic tool to interfere with the immune system.
The present article, therefore, aims to give an overview about the plethora of functions of SDF-1/CXCR4 signaling in tumor biology and immune responses in the context of combined radiotherapy and immunotherapy. The knowledge about these functions is indispensable for developing new concepts of anti-cancer therapy that comprise radiotherapy, immunomodulation and SDF-1/CXCR4 targeting.
Interference of Ionizing Radiation With Immune Responses
Radiotherapy effects on cancer had been mostly attributed to direct cytotoxic effects on cancer cells (especially DNA damage) (9). With the advance of cancer immunotherapies preclinical and clinical observations pointed toward synergistic effects. The so-called “abscopal effect” describes responses to radiotherapy (mostly in combination with immunotherapy) outside the irradiated volume and has been linked to immune mechanisms (10). The combination of immune checkpoint inhibitors with local tumor irradiation seems to induce synergistic effects and is currently tested in multiple clinical trials (11, 12). In addition, theoretical rationales and preclinical data provide the basis for also combining radiotherapy with other immunotherapy strategies, such as anticancer-vaccines and cytokine-based therapies (13).
Immune Effects of Tumor Irradiation
The mechanisms of radiation-induced immune effects have been summarized as immune-stimulating and immunosuppressive either directly in tumor cells or in the microenvironment (8). Radiation triggers anti-tumor immune responses directly in the cancer cells by upregulation of MHC-I molecules (14, 15) and possible induction of new tumor associated antigens (14). Cell death mechanisms induced by tumor irradiation lead to “immunogenic cell death” (ICD) (16, 17) characterized by the ability to stimulate the innate immune system and thus indirectly also adaptive immune responses (18, 19). ICD is characterized by the release of danger associated molecular patterns (DAMPs), such as calreticulin (20), high-mobility-group-box 1 (HMGB1) (21) and extracellular adenosine-tri-phosphate (ATP) (22). Additional mechanisms include cytokine release, such as CXCL10 (23), and type-1 interferon (24). Indirect immune stimulation has been attributed to increase and activation of tumor-infiltrating lymphocytes (25, 26), as well as maturation of dendritic cells (DCs) (27). The fact that clinically relevant anti-tumor responses (e.g., abscopal effects after palliative radiotherapy in metastatic cancer patients) are rare despite of these strong immune-stimulating effects is most probably due to simultaneously induced immunosuppression by tumor irradiation. Irradiated tumor cells upregulate immune checkpoint molecules, such as PD-L1 (28, 29) and release immunosuppressive cytokines, such as TGFβ (30, 31). Immunosuppressive cells increased in the tumor immune microenvironment upon local irradiation include regulatory T cells (32, 33) and myeloid-derived suppressor cells (MDSC) (34–36).
Combination Therapies of Irradiation and Immunotherapy for Cancer
These mechanisms have become the basis for combining radiotherapy and immunotherapy in order to exploit pro-immunogenic properties of irradiation and block immunosuppressive actions. Clinical trials ongoing with combinatorial approaches include immune checkpoint inhibition, cytokine based therapies and vaccination strategies (37, 38). Combination of radiotherapy with immune checkpoint inhibition has been recently summarized (39). Besides its use in metastatic cancer patients, durvalumab as adjuvant treatment after definitive radiochemotherapy for non-small cell lung cancer has shown significantly improved disease free survival (40). Vaccination strategies used in combination with radiotherapy include peptide and mRNA based approaches (41–43) which showed promising results in syngeneic mouse xenograft models. IL2 and IL12 as tumor targeted immunocytokines have been tested in combination with tumor irradiation in in vivo models showing promising results (44–47).
In conclusion, the strong rationale and promising results led to an increasing use of immunotherapeutics in combination with local tumor irradiation in standard of care treatment of palliative cancer patients as well as in numerous clinical trials with high expectations of the oncological field to improve survival and prognosis of cancer patients.
SDF-1/CXCR4 Function In Tumor Biology
SDF-1/CXCR4 signaling has been shown to contribute to virtually all processes in tumor biology. As described in this section, SDF-1/CXCR4 signaling reportedly contributes to neoplastic transformation, malignant tumor progression, infiltration, metastasis, angiogenesis and vasculogenesis, and consequently therapy resistance of many different tumor entities.
CXCR4, a Marker of Cancer Stem(-Like) Cells or Tumor-Initiating Cells
CXCR4 chemokine receptors are expressed by hematopoietic stem cells and are required for the trapping of these cells within the stem cell niches of the bone marrow. CXCR4 antagonists, such as AMD3100 (Plerixafor), therefore, can be used to mobilize stem cells into the peripheral blood for hematopoietic stem cell donation (see below). Beyond that, SDF-1/CXCR4 signaling has been shown to be functional in neural progenitor cells and to direct neural cell migration during embryogenesis (48). Notably, CXCR4 expression is further upregulated when neural progenitor cells differentiate into neuronal precursors whereas SDF-1 is upregulated during maturation of neural progenitor cells into astrocytes. While CXCR4 is localized in the cell body of neuronal precursors, expression is primarily restricted to axons and dendrites in mature neurons (49). In addition, SDF-1/CXCR4 signaling has been reported to contribute to chemotaxis and differentiation into oligodendrocytes of engrafted neural stem cells resulting in axonal remyelination in a mouse model of multiple sclerosis (50). Together this suggests that neurogenesis requires functional SDF-1/CXCR4 signaling and CXCR4 as marker of especially the neuronal lineage of neural stem cells.
Primary glioblastoma multiforme (GBM) develops directly by neoplastic transformation of neural stem cells and not by malignant progression from astrocytic gliomas or oligodendroglomas (the latter two are characterized by mutations in the IDH genes). Not unexpectedly, stem(-like) subpopulations of GBM functionally express SDF-1/CXCR4 signaling (51–56). Notably, auto-/paracrine SDF-1/CXCR4 signaling is required for maintenance of stemness and self-renewal capacity (57–59) since SDF-1/CXCR4 targeting leads to loss of stem cell markers and differentiation of stem(-like) cells into “differentiated” tumor bulk.
Besides glioblastoma, SDF-1/CXCR4 signaling has been shown to be functional in stem(-like) subpopulations of retinoblastoma (60), melanoma (61), pancreatic ductal adenocarcinoma (62), non-small cell lung cancer (63), cervical carcinoma (64), prostate cancer (65), head and neck squamous cell carcinoma (66), rhabdomyosarcoma (67, 68), synovial sarcoma (56), and leukemia (69). In summary, these data might hint to an ontogenetically early onset of SDF-1/CXCR4 signaling in mesenchymal and epithelial primordia of the different organs which might be the reason for SDF-1/CXCR4 expression in stem(-like) subpopulations of many different tumor entities.
Transition of stem(-like) cells and differentiated tumor bulk and vice versa seems to be highly dynamic and regulated by the reciprocal crosstalk with untransformed stroma cells of the tumor microenvironment (70–72). Beyond that, this crosstalk seems to induce phenotypical changes of cancer stem(-like) cells as deduced from the following observation. Sorted CD133+ stem(-like) cells and CD133− differentiated bulk cells of GBM did not differ in repair of radiation-induced DNA double strand breaks in vitro. Upon orthotopic transplantation in immunocompromized mice, however, CD133+ cells repaired more efficiently than CD133− cells indicating tumor-microenvironment-mediated upregulation of DNA repair selectively in CD133+ GBM cells (73). The next paragraph introduces the impact of SDF-1/CXCR4 signaling on the crosstalk of tumor cells with non-transformed stroma cells and its function for the cancer stem(-like) cell phenotype.
SDF-1/CXCR4 Signaling in the Crosstalk of Cancer Stem(-Like) Cells With Non-transformed Stroma Cells
The functional significance of SDF-1/CXR4 signaling between tumor cells and the tumor stroma is suggested by a retrospective analysis of genetic SDF-1 variants in patients with colorectal cancer where a certain SDF-1 polymorphism in fibroblasts is associated with higher stromal SDF-1 expression and increased risk for lymph node metastases in stage T3 colorectal cancer (74). Moreover, diffuse-type gastric cancer probably develops from quiescent Mist1+ stem cells upon Kras and APC mutation and loss of E-cadherin. Most importantly, this seems to be dependent on inflammatory processes triggered by SDF-1-expressing endothelial cells and CXR4-expressing gastric innate lymphoid cells that form the perivascular gastric stem cell niche (75).
Likewise, GBM cells co-opt vessels and home to perivascular stem cell niches. Reciprocal signaling between endothelial and GBM cells within these niches has been shown to induce and maintain a stem(-like) cell phenotype of GBM cells on the one hand and to promote angiogenesis on the other [for review see (76)]. Moreover, trans-differentiation of GBM stem-like cells into endothelial cells (77, 78) and pericytes (79) contributes to the adaptation of the tumor microvasculature to the needs of the GBM cells. SDF-1/CXCR4 signaling reportedly exerts pivotal functions in these processes. In particular, CXCR4 on GBM cells and SDF-1 produced by endothelial cells direct perivascular invasion as demonstrated in vitro and in orthotopic glioma mouse models (79–81). Accordingly, SDF-1-degradation by the cysteine protease cathepsin K facilitates evasion of GBM cells out of the niches (82). In addition to chemotaxis, CXCR4 stimulation by SDF-1 induces the production of vascular endothelial growth factor (VEGF) in GBM (83) and especially in CD133+ GBM stem-like cells (84). VEGF, in turn, stimulates beyond angiogenesis upregulation of CXCR4 (85) and SDF-1 (86) in microvascular endothelial cells. Moreover, VEGF is required for trans-differentiation of GBM-derived progenitor cells into endothelial cells (77). The significance of targeting VEGF and SDF-1/CXCR4 signaling for stem cell niche formation can be deduced from the observation that targeting of both, VEGF and CXCR4, decreases the number of perivascular GBM cells expressing stem cell markers in an orthotopic glioma mouse model, which was associated with improved survival of the tumor-bearing mice (87).
A further example of up-regulation of a stem(-like) cell phenotype mediated by SDF-1 signaling was reported for breast cancer cells where SDF-1 release from tumor-associated fibroblasts is required for the maintenance of tumor initiation capability (88). Finally, leukemia cells have been demonstrated to be trapped in stem cell niches of the bone marrow (89–91), and follicular lymphoma stem(-like) cells to follicular DCs in the germinal center of lymph nodes (92) by SDF-1/CXCR4 signaling. Combined, these data suggest that SDF-1 directed chemotaxis to certain microenvironmental stem cell niches is a general phenomenon of CXCR4-expressing hematopoietic and non-hematopoietic cancer cells. Of utmost importance, interactions with stromal cells within these niches contribute to a malignant and therapy-resistant phenotype of niche-residing cancer cells as outlined in the next paragraph.
SDF-1/CXCR4 Signaling in Tumor Microenvironment-Induced Therapy Resistance of Cancer Stem(-Like) Cells
Subventricular zones (SVZs) of the brain accommodate neural stem cells and have been shown to attract human GBM stem(-like) cells through SDF-1/CXCR4 signaling in an orthotopic glioma mouse model (93). Importantly, SVZ residence induces radioresistance of GBM stem(-like) cells in direct dependence on SDF-1 release by the SVZ stromal cells (94). Evidence for radioresistance conferred by SDF-1/CXCR4-dependent residency in perivascular niches was further provided by the observation that CXCR4 knockdown in mouse GBM cells resulted in both, diminished perivascular invasion and increased radiosensitivity (81).
Likewise, in mouse models of acute myeloid leukemia CXCR4 antagonism mobilized leukemia cells out of the bone marrow niches and, at the same time, enhanced chemosensitivity (90, 91). Mechanistically, bone marrow mesenchymal cells have been demonstrated to upregulate a signaling complex in the leukemia cells comprising CXCR4 and activating pro-survival signals via extracellular signal-related kinase 1/2 (ERK1/2) and the phosphoinositide 3-kinase (PI3K)/Akt pathway (95). Moreover, bone marrow disseminated xenografted head and neck squamous cell carcinoma (HNSCC) exhibits a higher cisplatin resistance than lung metastases ex vivo. This difference critically depends on TGF-β-triggered SDF-1/CXCR4 signaling (96). In summary, these preclinical in vivo and ex vivo data strongly suggest that SDF-1/CXCR4-mediated residency of tumor cells in stem cell niches induces resistance to chemo- and/or radiation therapy probably by inducing expression of a therapy-resistant cancer stem(-like) cell phenotype. The maintenance of the latter—as discussed above and impressively demonstrated by the ex vivo comparison of bone marrow and lung disseminated HNSCC—itself crucially depends on SDF-1/CXCR4. Beyond cancer stem(-like) cell induction, SDF-1/CXCR4 signaling has been demonstrated to trigger tumor invasion and metastasis as discussed in the next chapter.
SDF-1/CXCR4 Signaling in Triggering Tumor Invasion and Distant Metastasis
Associations between SDF-1/CXCR4 polymorphisms or SDF-1/CXCR4 abundance in tumor specimens and clinical outcome in several but not all studies might suggest a role of SDF-1/CXCR4 signaling in metastatic progression in a variety of tumor entities, such as renal cell carcinoma (97), prostate cancer (98), HNSCC (99–102), esophagogastric cancer (103), colorectal cancer (74), hepatocellular carcinoma (104), or osteosarcoma (105). In preclinical studies, overexpression of CXCR4 has been demonstrated to dramatically increase lung and liver metastases of murine pancreatic cancer in tail vein metastasis assays in nude mice (106). Consistently, antagonizing CXCR4 inhibited lung metastasis of human tongue squamous cell carcinoma (107), esophageal cancer (108), breast cancer (109) in immunocompromized mice. Intra-arterially injected circulating CXCR4-expressing melanoma cells require SDF-1 signaling by mesenchymal stem cells that act as pericytes for extravasation to bone and liver and perivascular niche formation as demonstrated by humanized heterotopic bone formation assay (110). Combined, these examples suggest that CXCR4 expression by cancer cells contribute to their tropism to metastatic niches.
Along those lines, CXCR4 downregulation by overexpression of miR-613 reportedly inhibits lung metastasis of osteosarcoma orthotopically xenografted in nude mice (105). Notably, epigenetic downregulation of SDF-1 has been demonstrated to boost metastases of CXCR4-expressing sarcoma in mouse models (111). Likewise, a SDF-1 polymorphism with low SDF-1 expression in breast cancer has been proposed to associate with susceptibility to metastases (112). It is, therefore, tempting to speculate that SDF-1−/CXCR4+ tumor cells are particularly prone to metastasize. As a matter of fact, high CXCR4 and low SDF-1 expression by the tumor has been associated with poor overall survival in osteosarcoma (111) and metastasis-free survival in head and neck squamous cell carcinoma (113). The latter association, however, was not confirmed by a recent study (114). Nevertheless, these reports strongly suggest a pro-metastatic function of SDF-1/CXCR4 signaling in several cancer entities.
In GBM which usually does not metastasize outside the central nervous system, SDF-1/CXCR4 signaling has been demonstrated in vitro to exert pivotal function in cell migration and chemotaxis (115–117). Most probably, SDF-1/CXCR4-dependent migration/chemotaxis does not only contribute to the above discussed homing of GBM cells to protective perivascular stem cell niches (see above) but also to deep infiltration of the brain parenchyma by highly migratory GBM stem(-like) cells. One driver of glioblastoma dissemination might be hypoxia through HIF-1α mediated up-regulation of SDF-1 and CXCR4 in GBM cells (85, 86). Unexpectedly, VEGF- or VEGF-R-targeting has been demonstrated in vitro to directly up-regulate CXCR4 expression and chemotaxis toward SDF-1 in VEGF-R-expressing GBM cells in a TGFβ-dependent manner (118). In accordance with these observations, anti-angiogenic therapy of orthotopic mouse glioma promotes GBM invasion by CXCR4 upregulation. Additional CXCR4-targeting blunts this effect (119). Consistently, combined VEGF- or VEGF-R- and CXCR4 antagonism prolongs survival of mice bearing orthotopically xenografted GBM as compared to only VEGF/VEGF-R-targeted mice (87, 118, 120). Also along those lines, anti-angiogenic therapy, such as Bevacizumab which has been demonstrated in large clinical trials not to improve overall survival of GBM patients is under suspicion to foster distant spread of the tumor at recurrence (121). Even if the tumor spread-promoting effect of Bevacizumab is under debate (122), nevertheless, these data bear witness to a close interaction between tumor hypoxia and SDF-1/CXCR4 signaling as introduced in more detail in the next chapter.
SDF-1/CXCR4-Signaling and its Function for Vasculogenesis
Hypoxia-induced up-regulation of SDF-1 secretion in tumors reportedly stimulates homing and engraftment of bone marrow-derived myeloid cells, as well as mesenchymal stem cell-derived endothelial and pericyte progenitor cells. This recruitment promotes neovascularization of the hypoxic tumor by transition of the myeloid and progenitor cells into endothelium and pericytes. Such SDF-1/CXCR4-dependent vasculogenesis has been demonstrated in mouse models of several tumor entities, such as GBM (123–127), HNSCC (128), lung adenocarcinoma (129), hepatocellular carcinoma (130) or breast cancer (131). Importantly, irradiation has been shown to induce SDF-1 expression and thus may boost vasculogenesis and tumor re-growth after end of therapy (125, 131–133) suggesting a radioresistance-conferring action of SDF-1/CXCR4 signaling as discussed in the next paragraph.
SDF-1/CXCR4-Signaling and Radioresistance
In many tumor entities radiation therapy is part of standard of care. Ionizing radiation has been demonstrated in vitro as well as in vivo to stimulate SDF-1/CXCR4 signaling in different human and mouse tumor entities either directly by S-nitrosylation and stabilization of HIF-1α (134) or indirectly via radiation-induced endothelial cell killing and resulting hypoxia (135) or HIF-1α-independent mechanisms (136). Radiation-induced modifications in SDF-1/CXCR4 signaling, in turn, have been reported in gliomas (116, 125, 127, 137), mesotheliomas (138), prostate (139), cervical (140), lung (131, 141) and breast cancer (131). Aside from the direct effect on cancer cells, radiation-induced SDF-1 secretion is also observed in different normal tissues/cells (94, 136, 142–147) or cancer-associated fibroblasts (144).
Importantly, radiation-modulated SDF-1/CXCR4 signaling has been shown to stimulate tumor re-growth (142, 148), EMT (144), migration (116), invasiveness (81, 127, 138, 141, 144) and metastases (138, 145), as well as homing of hematopoietic progenitor cells and accelerated vasculogenesis (125, 127, 131–133, 136, 137, 142). Thus, radiation-induced SDF-1/CXCR4 signaling may foster radioresistance, malignant progression and recurrence of tumors (94, 125, 139, 149–151). Preclinical evidence shows reduced metastases in orthotopic murine models of cervical cancer with Cisplatin-based radiochemotherapy and AMD3100 (152).
Thus, as CXCR4 is a prognostic marker for local control after curative radiotherapy and irradiation interferes with SDF-1/CXCR4 signaling, there is a strong rationale to develop translational and clinical interventional studies combining CXCR4 targeting with curative radio(chemo)therapy. The roles of SDF-1/CXCR4 signaling in tumor biology are summarized in Table 1.
SDF-1/CXCR4 Signaling as Druggable Target in Anti-cancer Therapy
As already touched upon, retrospective clinical data might hint to associations between SDF-1 polymorphisms or SDF-1/CXCR4 expression levels with susceptibility to neoplastic transformation, malignant progression or therapy response in a variety of tumor entities, such as renal cell carcinoma (97), prostate cancer (98), HNSCC (1, 100, 102, 113, 114), esophagogastric cancer (103), hepatocellular carcinoma (104), colorectal cancer (74), breast cancer (153), osteosarcoma (111), low grade glioma (154, 155), or GBM (156, 157). Beyond cancer SDF-1 genetics has been associated with e.g., the pathogenesis of multiple sclerosis (158) or prognosis in patients with cardiovascular disease (159).
Apart from genetic variants, SDF-1 as well as CXCR4 were shown to be regulated epigenetically by DNA methylation. DNA methylation status of the genes was suggested as prognostic biomarkers for e.g., breast or pancreatic cancer and GBM (160–162). Such prognostic or predictive value of SDF-1/CXCR4 might be expected from the plethora of SDF-1/CXCR4 functions in tumor biology mentioned above. These functions contribute to malignancy, progression and therapy resistance of the tumors and render SDF-1/CXCR4 signal to an ideal target in anti-cancer therapy. In particular, a combination of SDF-1/CXCR4-targeting and radiotherapy seems to be promising given the above mentioned radioprotective functions of SDF-1/CXCR4 signaling (Figure 1). Moreover, combinatorial treatment of conventional chemotherapy with CXCR4 inhibitors might be an approach to overcome cancer therapy resistance (163).
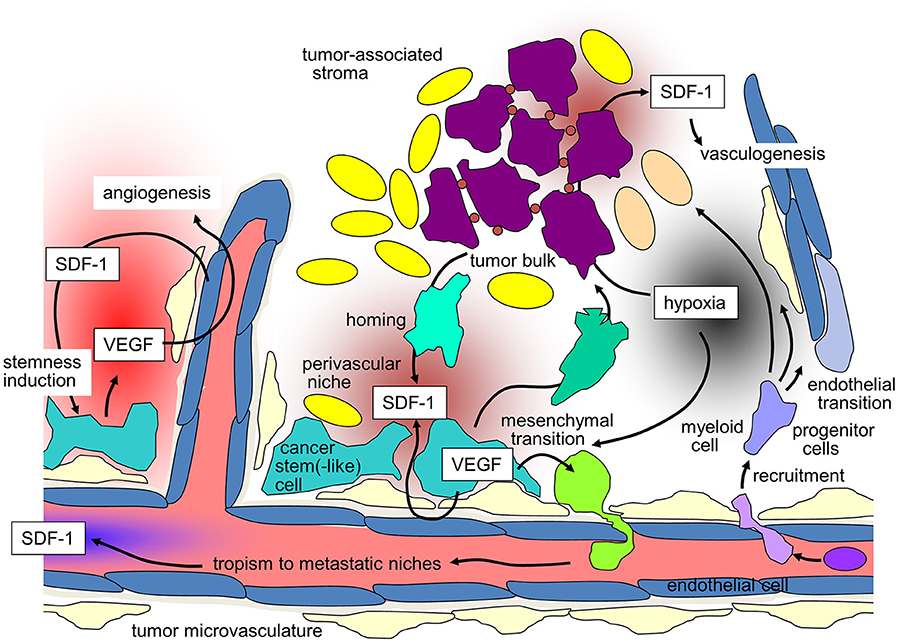
Figure 1. SDF-1/CXCR-4 signaling in tumors and its contribution to maintenance of tumor stemness, recruiting of distant stroma cells, angio- and vasculogenesis, and metastasis (for details see text).
In fact, several SDF-1- or CXCR4-targeting drugs have been applied in preclinical models [e.g., Ulocuplumab (164), ALT-1188 (165), POL5551 (166), PRX177561 (167)], were well-tolerated in clinical trials [e.g., AMD070 (168), Balixafortide (POL6326, Polyphor) (169)] or are FDA-approved [AMD3100, Plerixafor (170)] indicating that SDF-1/CXCR4 targeting is clinically feasible. Overall, Plerixafor used for stem cell mobilization does not induce severe side effects (171, 172). A randomized phase 3 trial comparing G-CSF with plerixafor vs. placebo reported mainly fatigue, gastrointestinal side effects like nausea and diarrhea and injection site reactions (173).
CXCR4, however, is expressed on immune cells suggesting that SDF-1/CXCR4-targeted anti-cancer therapy at the same time interferes with the immune response to cancers and cancer cells in e.g., circulation or micrometastases. In order to explore these functions and develop a rationale if trimodal therapy combining CXCR4 targeting with immunotherapy and radiotherapy might be of benefit, it is crucial to understand the function of SDF-1/CXCR4 signaling in immune cells and the effects of CXCR4 inhibition on the immune response to cancer.
Physiologic Role of Cxcr4 in the Immune System
In addition to its function in tumor biology, SDF-1/CXCR4 signaling controls multiple physiological processes in hematopoiesis, T, B and NK cell development and the organization of the immune system. Ablation of either of the components of the SDF-1/CXCR4 axis generates a similar phenotype of deficient B lymphopoiesis and myelopoiesis, disturbed immune responses leading to cancers, autoimmunity and inflammatory diseases (174–176). Recently a 16 amino acid fragment of serum albumin (EPI-X4) was identified as an effective and highly specific endogenous CXCR4 antagonist (177). Peptide EPI-X4 is evolutionarily conserved and generated from the highly abundant albumin precursor by pH-regulated proteases. It antagonizes SDF-1-induced tumor cell migration and suppresses inflammatory responses in mice. In human the peptide is abundant in the urine of patients with inflammatory diseases. EPI-X4 mobilizes stem cells, which explains in part why stem cells can directly respond to inflammation with their migration into the periphery.
Hematopoietic Stem Cell Niche
HSCs (Hematopoietic stem cells) are a rare cell population that can give rise to all lineages of the immune system. HSCs reside in the undifferentiated state in the bone marrow, where the binding of their CXCR4 receptor to its ligand SDF-1—constitutively provided by the bone marrow (BM) niche—promotes their survival (178, 179) while negatively regulating their proliferation (180–182). In addition to direct effects on HSCs, SDF-1/CXCR4 signaling also promotes survival and growth of human bone marrow stromal stem cells (183). Inhibiting the interaction between CXCR4 receptor and SDF-1 leads to the migration of hematopoietic stem and progenitor cells (HSPC) into the periphery, a process termed mobilization, which is required for harvesting stem cells for transplantation [either autologous from the patient (184) or in healthy donors (185)]. A dramatic increase in mobilization efficiency and yields of progenitor cells compared to standard G-CSF is achieved when using CXCR4 antagonists, such as AMD3100, Mozobil® (184, 186, 187). CXCR4 antagonist BL-8040 in a recent phase I clinical study (NCT02073019) besides highly efficient mobilization of pluripotent hematopoietic progenitors showed also superior yields of CD4+ and CD8+ T cells, NKT, NK, and DCs, suggesting increased engraftment ability of CXCR4 mobilized populations, a higher anti-tumor effect and faster immune reconstitution potential. Moreover, mobilization as a 1-day procedure is less wearing for the donor and allows faster access to the stem cells (188). Since HSCs maintain hematopoiesis throughout life, qualitative and quantitative effects through prolonged pharmacologic blockade of the SDF-1/CXCR4 axis need to be investigated. Concerns that the HSC pool in the bone marrow would decrease were not confirmed, as CXCR4-blockade led to higher repopulating capacity and exceptional mobilization along with an expansion of the BM HSC pool, which unexpectedly suggests reversible inhibition of the SDF-1/CXCR4 axis also as a novel strategy to restore damaged BM (189). BM HSCs during reversible long-term CXCR4/SDF1 long term blockade increase their cycling activity 2- to 3-fold [only 10–20% of Lin-Sca1+Kit- (LSK) and 30–40% of LSK SLAM cells being quiescent (G0 phase)] compared to 50–60% of LSK and 70% of LSK SLAM under homeostatic conditions (190, 191). Thus, these findings together with mounting evidence for direct cytolytic and specific anti-leukemic effects of CXCR4 inhibition (192–194) suggests prolonged CXCR4 blockade as a novel safe therapeutic scheme for treatment of (hematologic) malignancies either alone or in conjunction with chemotherapy.
Dendritic Cells
The priming of naïve T cells is dependent on efficient antigen presentation and a strong costimulatory signal both provided by dendritic cells during Th1 polarized immune responses. Th1 polarization is thought to be critical for immune rejection of tumors, while activated T cells polarized to Th2 cytokine and cell profiles might induce even tumor immune evasion (195). Plasmacytoid DCs (pDCs) as type I interferon (IFN)-producing cells play a central role in antiviral and anti-tumor immunity. pDCs are produced from hematopoietic stem cells in the bone marrow where they nestle down with reticular cells in the intersinal space which abundantly provides SDF-1. Concordantly, the number of pDCs and their earliest progenitors is severely reduced in the absence of CXCR4 in vitro and in vivo, underlining the function of SDF-1/CXCR4 axis as a key regulator of pDC development and the importance of provision of SDF-1 by cellular niches (196, 197). Upon activation, CXCR4 expressing DCs migrate into SDF-1 expressing lymphatic vessels where they initiate immune responses, a process that is severely blocked by systemic CXCR4 antagonist application (198). Since the dysregulated expression of SDF-1/CXCR4 is associated with the pathology of various autoimmune diseases, including rheumatoid arthritis, systemic lupus erythematosus, and multiple sclerosis, targeting SDF-1/CXCR4 axis with 4-F-Benzoyl-TN14003 may be beneficial for prevention of autoimmune disease (198–201). It is not clear, whether these effects on DCs might decrease the efficacy of anti-cancer immune responses upon CXCR4 inhibition.
Myeloid Derived Suppressor Cells
As reviewed in (202), MDSCs are highly immunosuppressive cells in the tumor microenvironment and mainly suppress intratumoral T cells. SDF-1 secreted by tumor associated fibroblasts induces MDSCs and impairs anti-tumor immune responses as shown in a hepatic carcinoma model (203). Another liver cancer model (metastases of colorectal carcinoma) showed less MDSC infiltrates in the metastases after treatment with AMD3100, accompanied by reduced metastases growth (204). Patient samples of ascites also showed that CXCR4 signaling is involved in MDSC recruitment. SDF-1 release of cancer cells as well as CXCR4 signaling in MDSCs could be abrogated by COX2 inhibition.
Regulatory T Cells (nTreg and iTreg)
Regulatory T cells (Tregs) constitute 5–10% of peripheral CD4+ T cells in humans (205, 206). Tregs maintain immune homeostasis, peripheral tolerance and prevent autoimmunity by suppressing and terminating immune responses. They constitute a major barrier for an effective antitumor immunity, and the number of peripheral and intratumoral Treg cells is an independent prognostic factor in malignancies (207). Cancer cell- and M2 macrophages derived SDF-1 attracts Treg cells into the tumor lesion where they robustly induce FOXP3 and other Treg signature molecules in human naïve CD4+ T cells which display enhanced FOXP3 stability and low expression of pro-inflammatory cytokines (208). Treg cells limit immune effector cell function via cytokines (209–212), direct lysis (213), inhibitory receptors on their cell surface (214–217), via metabolic disruption (218), by starving effector cells via depletion of local IL-2 (219) or indirectly by turning secondary cell types into suppressive ones i.e., IDO (220) and tolerogenic cytokine producing DCs with low costimulatory potential (221). Treg depletion dramatically reduced tumor growth or induced full remission (222–224). In contrast to conventional chemotherapeutic agents which also deplete T effector cells and may induce autoimmunity due to the systemic elimination of T-regs (225), CXCR4 targeting allows the specific targeting of Tregs, since intratumoral Tregs consistently express higher CXCR4 levels than CD4+CD25− and CD8+ cells (226). In intraperitoneal papillary epithelial ovarian cancer, CXCR4 antagonism increased tumor cell apoptosis and necrosis, reduced intraperitoneal dissemination, and selectively reduced intratumoral FoxP3 Tregs (226). Superior immune responses as shown for CXCR4 antagonist BL-8040 is not solely owing to a selectively reduced recruitment of Treg cells into the tumor, and an increase in the number of immune and progenitor cells (227). CXCR4 antagonists have shown to also reverse Tregs' suppressive activity. Plerixafor and the antagonistic CXCR4 peptide R29 (228) inhibited Treg-suppressive activity significantly (by 10-fold) in Tregs from primary renal cancer specimens in which high numbers of activated Tregs (expressing CTLA-4/CXCR-4/PD-1/ICOS) were detected. A possible mechanistic explanation involves the demethylation of Treg-FOXP3 promoter (229). Thus, inhibition of Tregs by blocking SDF-1/CXCR4 is one of the major rationales for a better anti-tumor immune response via CXCR4 inhibition.
Effector Cells
T effector (Teff) cells also constitutively express the chemokine receptor CXCR4. Besides T cell migration along SDF-1 gradients, CXCR4 after T cell receptor crosslinking is recruited to and accumulates at the immunological synapse, resulting in stronger T cell-APC interaction, shutdown of T cell responsiveness to chemotactic gradients, and in higher levels of T cell proliferation and IFN-γ production (230). Vice versa, the presence of competing external chemokine signals has been shown to disrupt the stability of T-APC conjugates as a result of impaired recruitment of the receptor to the immunologic synapse (231). CXCR4 confers the homing of antiviral T cell responses to bone marrow. Ablation of CXCR4 thus impairs memory cell maintenance due to defective homeostatic proliferation in the bone marrow niche, yet allows fully functional asymmetric cell fates after antigenic rechallenge in CD8+ T cells (232). Antitumoral activity was shown for CXCR4 antagonist BL-8040 in tumor bearing mice, where it induced robust mobilization of CD4+ and CD8+ T lymphocytes and DC in numbers that were significantly higher compared to tumor free naïve counterparts. The authors did not discriminate the lymphocytic population with respect of Teff/Treg ratio or CD8+ content though (233), yet showed in pre-clinical in vivo pancreatic cancer models, immune cells mobilized from the bone marrow into the circulation accumulate within the tumor lesion where they inhibit tumor growth. Such a dramatic effect on the intratumoral T cell compartment function is reflected in a study by Elda Righi (226) where CXCR4 antagonist AMD3100 by favorably modulating the intratumoral Teff/Treg ratio 6-fold, created a phenotype reminiscent of two studies that—although in different contexts—depleted intratumoral T-regs which highly significantly improved cytotoxic T-cell function in the tumor tissue and prolonged survival (234, 235). Along those lines, epigenetic down-regulation of SDF-1 expression in osteosarcoma has been demonstrated to impair cytotoxic T-cell homing to the tumor site (111). In contrast, SDF-1 overexpression by melanoma cells in the B16-ova melanoma model has been shown to chemo-repel antigen-specific cytotoxic T cells (236) suggesting a complex and fine-tuned control of Teff infiltration by SDF-1/CXCR4 signaling.
Chemokines control also the trafficking of developing and mature natural killer cells (NK) in the bone marrow (237). While several CCRs are expressed during progression from precursor to immature and mature NK cells CXCR4 was only detected on immature NK cells. Administration of the CXCR4 antagonist, AMD3100, induced strong reduction of mature NK and immature NK cells in the BM in a murine model and increased their number in blood and spleen, which suggests that this chemokine axis also regulates NK cell subsets localization in the bone marrow niche and their migration to the periphery for their maturation (238). Notably, genetic deletion of CXCR4 in myeloid cells in a melanoma mouse model fostered NK cell-mediated antitumor immunity suggesting indirect suppression of NK cell activity by CXCR4 signaling (239).
In summary, SDF-1/CXCR4 signaling affects most subsets of immune cells, the most prominent and clinically applied effect being the mobilization of HSCs by blocking CXCR4 as summarized in Table 2.
Combined Immunotherapy and CXCR4 Targeting
The promotion of antitumor immunity by CXCR4-antagonists was reported for a mouse model of ovarian cancer (226) and in an orthotopic preclinical hepatocellular carcinoma (HCC) model where a CXCR4 antagonist was combined with a checkpoint inhibitor. In this HCC model multi-kinase-inhibitor sorafenib treatment-induced hypoxia fostered SDF-1 production, leading to the recruitment of immunosuppressive tumor-associated macrophages, myeloid-derived suppressive cells, and Tregs all with increased PD-L1 expression. CXCR4 antagonist plerixafor combined with anti-PD-1 therapy showed the most pronounced tumor growth delay, and was associated with increased intratumoral penetration and activation of CD8+ T lymphocytes (241). A novel strategy for the treatment of drug-resistant ovarian cancer combines chemotherapy to increase immunogenic cell death and virally delivered CXCR4 to reverse the immunosuppressive tumor microenvironment (242). Ovarian cancer of murine and human ovarian tumor variants resistant to paclitaxel and carboplatin were infected with oncolytic vaccinia virus expressing a CXCR4 antagonist and were +/– treated in combination with doxorubicin. The chemo-resistant variants' augmented expression of CXCR4 was associated with an increased susceptibility to viral infection and doxorubicin-mediated killing compared to parental counterparts in vitro and in tumor-challenged mice. Antitumor immune responses in this model culminated in the control of metastatic tumor growth and tumor-free survival. Mechanistically, the authors showed combination treatment increased apoptosis and phagocytosis of tumor material by DCs which efficiently induced adaptive antitumor immunity, reflected by increased intratumoral infiltration of antitumor CD8+ T cells and reduced immunosuppressive Tregs (242). Based on these results (Figure 2), the MORPHEUS clinical trials were started including treatment arms combining immune-checkpoint inhibitors with CXCR4 inhibition (NCT03193190, NCT03281369 and NCT03337698 for pancreatic cancer, gastric cancer and non-small cell lung cancer, respectively).
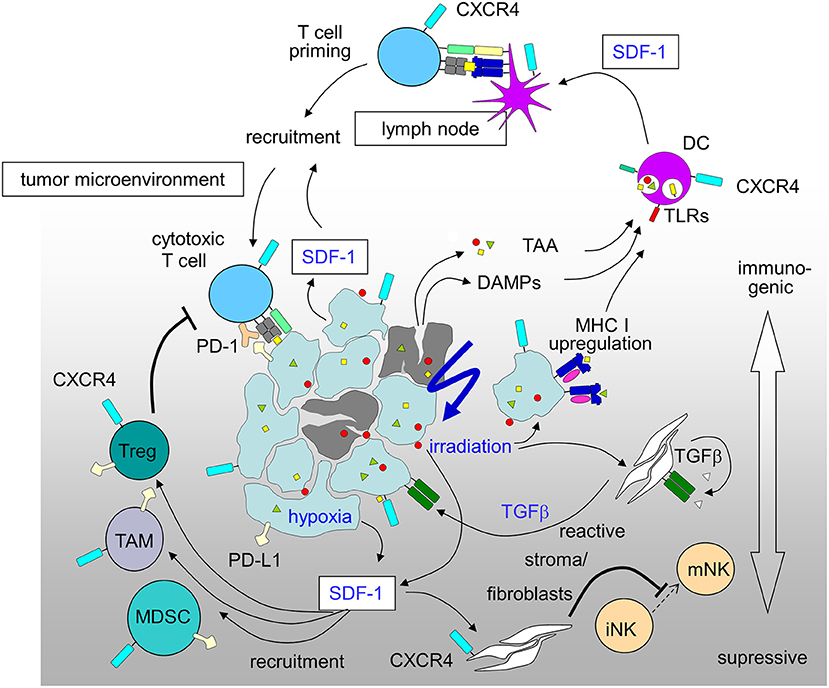
Figure 2. Immunosuppressive and immunostimulatory action of SDF-1/CXCR4 signaling in tumors induced by radiation-therapy and hypoxia (for details see text; DAMPs, danger-associated molecular patterns; DC, dendritic cells; MDSC, myeloid derived suppressor cell; PD-1, programmed cell death protein-1; PD-L1, PD-1 ligand; TAA, tumor-associated antigens; TAM, tumor-associated macrophage; Treg, regulatory T-cell).
Concluding Remarks
With combinatorial approaches of radiotherapy and immunotherapy on the rise, it is important to evaluate novel treatment strategies in radiation oncology with respect to tumor and radiation biology as well as immunologic effects. For SDF-1/CXCR4 targeting both perspectives provide a strong rationale for combination therapies. The SDF-1/CXCR4 axis plays pivotal roles in various aspects of tumor biology, and in particular in the stress response of tumors to ionizing radiation. In preclinical in vivo models CXCR4 targeting increases the efficacy of radiation therapy and blunts adverse effects, such as radiation-stimulated metastases and vasculogenesis. Mobilization of HSCs, a significant increase of immune and progenitor cells in the periphery that are able to migrate into the tumor and the selective targeting of Treg cells in the tumor lesion provide the rationale for an increased anti-tumor immune response upon CXCR4 inhibition. Preclinical mechanistic studies as well as translational and clinical evaluation of the role of the SDF-1/CXCR4 axis in the context of cancer radiotherapy and immunotherapy might lead to novel treatment strategies implementing SDF-1/CXCR4 targeting in this context using the small molecule inhibitors already approved for the use in patients and healthy donors for HSC mobilization.
Author Contributions
FE and SH designed the concept and wrote the manuscript. LK wrote chapter SDF-1/CXCR4-Signaling and Radioresistance. MS wrote chapter SDF-1/CXCR4 Signaling as Druggable Target in Anti-cancer Therapy. LB wrote chapter Interference of Ionizing Radiation With Immune Responses. ES contributed to chapter SDF-1/CXCR4 Function in Tumor Biology. KS wrote chapter Physiologic Role of CXCR4 in the Immune System. DZ and all authors read and approved the manuscript.
Conflict of Interest Statement
FE has a research collaboration with Merck KgAa. SH has a research collaboration with Novocure. DZ, FE have research and educational grants from Elekta, Philips, Siemens, Sennewald.
The remaining authors declare that the research was conducted in the absence of any commercial or financial relationships that could be construed as a potential conflict of interest
Funding
FE was partly funded by the Else-Kroener-Fresenius Research Foundation under Grant 2015_Kolleg.14. LK was supported by the ICEPHA program of the University of Tübingen and the Robert Bosch Stiftung, Bosch-Gesellschaft für Medizinische Forschung, Stuttgart, MS was partly supported by a grant from the German Cancer Aid (70112564) and by the Robert Bosch Stiftung, Stuttgart and ES by a scholarship from the DAAD. SH was partly funded by grants from the German Cancer Aid (70112872, 70113144). We acknowledge support by Deutsche Forschungsgemeinschaft and Open Access Publishing Fund of University of Tübingen.
References
1. De-Colle C, Menegakis A, Monnich D, Welz S, Boeke S, Sipos B, et al. SDF-1/CXCR4 expression is an independent negative prognostic biomarker in patients with head and neck cancer after primary radiochemotherapy. Radiother Oncol. (2018) 126:125–31. doi: 10.1016/j.radonc.2017.10.008
2. Richardson PJ. CXCR4 and glioblastoma. Anticancer Agents Med Chem. (2016) 16:59–74. doi: 10.2174/1871520615666150824153032
3. Duda DG, Kozin SV, Kirkpatrick ND, Xu L, Fukumura D, Jain RK. CXCL12 (SDF1alpha)-CXCR4/CXCR7 pathway inhibition: an emerging sensitizer for anticancer therapies? Clin Cancer Res. (2011) 17:2074–80. doi: 10.1158/1078-0432.ccr-10-2636
4. Hartmann T, Hubel K, Monsef I, Engert A, Skoetz N. Additional plerixafor to granulocyte colony-stimulating factors for haematopoietic stem cell mobilisation for autologous transplantation in people with malignant lymphoma or multiple myeloma. Cochrane Database Syst Rev. (2015) Cd010615. doi: 10.1002/14651858.CD010615.pub2
5. Demaria S, Formenti SC. Radiation as an immunological adjuvant: current evidence on dose and fractionation. Front Oncol. (2012) 2:153. doi: 10.3389/fonc.2012.00153
6. Sullivan RJ, Atkins MB, Kirkwood JM, Agarwala SS, Clark JI, Ernstoff MS, et al. An update on the Society for Immunotherapy of Cancer consensus statement on tumor immunotherapy for the treatment of cutaneous melanoma: version 2.0. J Immunother Cancer. (2018) 6:44. doi: 10.1186/s40425-018-0362-6
7. Chae YK, Arya A, Iams W, Cruz MR, Chandra S, Choi J, et al. Current landscape and future of dual anti-CTLA4 and PD-1/PD-L1 blockade immunotherapy in cancer; lessons learned from clinical trials with melanoma and non-small cell lung cancer (NSCLC). J Immunother Cancer. (2018) 6:39. doi: 10.1186/s40425-018-0349-3
8. Wennerberg E, Vanpouille-Box C, Bornstein S, Yamazaki T, Demaria S, Galluzzi L. Immune recognition of irradiated cancer cells. Immunol Rev. (2017) 280:220–30. doi: 10.1111/imr.12568
9. Kavanagh JN, Redmond KM, Schettino G, Prise KM. DNA double strand break repair: a radiation perspective. Antioxid Redox Signal. (2013) 18:2458–72. doi: 10.1089/ars.2012.5151
10. Postow MA, Callahan MK, Barker CA, Yamada Y, Yuan J, Kitano S, et al. Immunologic correlates of the abscopal effect in a patient with melanoma. N Engl J Med. (2012) 366:925–31. doi: 10.1056/NEJMoa1112824
11. Pilones KA, Vanpouille-Box C, Demaria S. Combination of radiotherapy and immune checkpoint inhibitors. Semin Radiat Oncol. (2015) 25:28–33. doi: 10.1016/j.semradonc.2014.07.004
12. Teng F, Kong L, Meng X, Yang J, Yu J. Radiotherapy combined with immune checkpoint blockade immunotherapy: achievements and challenges. Cancer Lett. (2015) 365:23–9. doi: 10.1016/j.canlet.2015.05.012
13. Eckert F, Gaipl US, Niedermann G, Hettich M, Schilbach K, Huber SM, et al. Beyond checkpoint inhibition–immunotherapeutical strategies in combination with radiation. Clin Transl Radiat Oncol. (2017) 2:29–35. doi: 10.1016/j.ctro.2016.12.006
14. Reits EA, Hodge JW, Herberts CA, Groothuis TA, Chakraborty M, Wansley EK, et al. Radiation modulates the peptide repertoire, enhances MHC class I expression, and induces successful antitumor immunotherapy. J Exp Med. (2006) 203:1259–71. doi: 10.1084/jem.20052494
15. Santin AD, Hermonat PL, Hiserodt JC, Chiriva-Internati M, Woodliff J, Theus JW, et al. Effects of irradiation on the expression of major histocompatibility complex class I antigen and adhesion costimulation molecules ICAM-1 in human cervical cancer. Int J Radiat Oncol Biol Phys. (1997) 39:737–42.
16. Golden EB, Apetoh L. Radiotherapy and immunogenic cell death. Semin Radiat Oncol. (2015) 25:11–7. doi: 10.1016/j.semradonc.2014.07.005
17. Golden EB, Frances D, Pellicciotta I, Demaria S, Helen Barcellos-Hoff M, Formenti SC. Radiation fosters dose-dependent and chemotherapy-induced immunogenic cell death. Oncoimmunology (2014) 3:e28518. doi: 10.4161/onci.28518
18. Garg AD, Dudek-Peric AM, Romano E, Agostinis P. Immunogenic cell death. Int J Dev Biol. (2015) 59:131–40. doi: 10.1387/ijdb.150061pa
19. Gameiro SR, Jammeh ML, Wattenberg MM, Tsang KY, Ferrone S, Hodge JW. Radiation-induced immunogenic modulation of tumor enhances antigen processing and calreticulin exposure, resulting in enhanced T-cell killing. Oncotarget (2014) 5:403–16. doi: 10.18632/oncotarget.1719
20. Panaretakis T, Kepp O, Brockmeier U, Tesniere A, Bjorklund AC, Chapman DC, et al. Mechanisms of pre-apoptotic calreticulin exposure in immunogenic cell death. Embo J. (2009) 28:578–90. doi: 10.1038/emboj.2009.1
21. Suzuki Y, Mimura K, Yoshimoto Y, Watanabe M, Ohkubo Y, Izawa S, et al. Immunogenic tumor cell death induced by chemoradiotherapy in patients with esophageal squamous cell carcinoma. Cancer Res. (2012) 72:3967–76. doi: 10.1158/0008-5472.can-12-0851
22. Martins I, Wang Y, Michaud M, Ma Y, Sukkurwala AQ, Shen S, et al. Molecular mechanisms of ATP secretion during immunogenic cell death. Cell Death Differ. (2014) 21:79–91. doi: 10.1038/cdd.2013.75
23. Sistigu A, Yamazaki T, Vacchelli E, Chaba K, Enot DP, Adam J, et al. Cancer cell-autonomous contribution of type I interferon signaling to the efficacy of chemotherapy. Nat Med. (2014) 20:1301–9. doi: 10.1038/nm.3708
24. Vanpouille-Box C, Formenti SC, Demaria S. TREX1 dictates the immune fate of irradiated cancer cells. Oncoimmunology (2017) 6:e1339857. doi: 10.1080/2162402x.2017.1339857
25. Ganss R, Ryschich E, Klar E, Arnold B, Hammerling GJ. Combination of T-cell therapy and trigger of inflammation induces remodeling of the vasculature and tumor eradication. Cancer Res. (2002) 62:1462–70.
26. Rodriguez-Ruiz ME, Garasa S, Rodriguez I, Solorzano JL, Barbes B, Yanguas A, et al. Intercellular adhesion molecule-1 and vascular cell adhesion molecule are induced by ionizing radiation on lymphatic endothelium. Int J Radiat Oncol Biol Phys. (2017) 97:389–400. doi: 10.1016/j.ijrobp.2016.10.043
27. Kulzer L, Rubner Y, Deloch L, Allgauer A, Frey B, Fietkau R, et al. Norm- and hypo-fractionated radiotherapy is capable of activating human dendritic cells. J Immunotoxicol. (2014) 11:328–36. doi: 10.3109/1547691x.2014.880533
28. Deng L, Liang H, Burnette B, Beckett M, Darga T, Weichselbaum RR, et al. Irradiation and anti-PD-L1 treatment synergistically promote antitumor immunity in mice. J Clin Invest. (2014) 124:687–95. doi: 10.1172/jci67313
29. Twyman-Saint Victor C, Rech AJ, Maity A, Rengan R, Pauken KE, Stelekati E, et al. Radiation and dual checkpoint blockade activate non-redundant immune mechanisms in cancer. Nature (2015) 520:373–7. doi: 10.1038/nature14292
30. Dancea HC, Shareef MM, Ahmed MM. Role of Radiation-induced TGF-beta signaling in cancer therapy. Mol Cell Pharmacol. (2009) 1:44–56.
31. Vanpouille-Box C, Diamond JM, Pilones KA, Zavadil J, Babb JS, Formenti SC, et al. TGFbeta is a master regulator of radiation therapy-induced antitumor immunity. Cancer Res. (2015) 75:2232–42. doi: 10.1158/0008-5472.can-14-3511
32. Liu S, Sun X, Luo J, Zhu H, Yang X, Guo Q, et al. Effects of radiation on T regulatory cells in normal states and cancer: mechanisms and clinical implications. Am J Cancer Res. (2015) 5:3276–85.
33. Price JG, Idoyaga J, Salmon H, Hogstad B, Bigarella CL, Ghaffari S, et al. CDKN1A regulates Langerhans cell survival and promotes Treg cell generation upon exposure to ionizing irradiation. Nat Immunol. (2015) 16:1060–8. doi: 10.1038/ni.3270
34. Meirow Y, Kanterman J, Baniyash M. Paving the road to tumor development and spreading: myeloid-derived suppressor cells are ruling the fate. Front Immunol. (2015) 6:523. doi: 10.3389/fimmu.2015.00523
35. Sampath S, Won H, Massarelli E, Li M, Frankel P, Vora N, et al. Combined modality radiation therapy promotes tolerogenic myeloid cell populations and STAT3-related gene expression in head and neck cancer patients. Oncotarget (2018) 9:11279–90. doi: 10.18632/oncotarget.24397
36. Wang X, Yang X, Tsai Y, Yang L, Chuang KH, Keng PC, et al. IL-6 mediates macrophage infiltration after irradiation via up-regulation of CCL2/CCL5 in non-small cell lung cancer. Radiat Res. (2017) 187:50–59. doi: 10.1667/rr14503.1
37. Vacchelli E, Bloy N, Aranda F, Buque A, Cremer I, Demaria S, et al. Trial watch: immunotherapy plus radiation therapy for oncological indications. Oncoimmunology (2016) 5:e1214790. doi: 10.1080/2162402x.2016.1214790
38. Basler L, Kowalczyk A, Heidenreich R, Fotin-Mleczek M, Tsitsekidis S, Zips D, et al. Abscopal effects of radiotherapy and combined mRNA-based immunotherapy in a syngeneic, OVA-expressing thymoma mouse model. Cancer Immunol Immunother. (2018) 67:653–62. doi: 10.1007/s00262-018-2117-0
39. Patel SA, Minn AJ. Combination cancer therapy with immune checkpoint blockade: mechanisms and strategies. Immunity (2018) 48:417–33. doi: 10.1016/j.immuni.2018.03.007
40. Antonia SJ, Villegas A, Daniel D, Vicente D, Murakami S, Hui R, et al. Durvalumab after chemoradiotherapy in stage III non-small-cell lung cancer. N Engl J Med. (2017) 377:1919–29. doi: 10.1056/NEJMoa1709937
41. Fotin-Mleczek M, Zanzinger K, Heidenreich R, Lorenz C, Kowalczyk A, Kallen KJ, et al. mRNA-based vaccines synergize with radiation therapy to eradicate established tumors. Radiat Oncol. (2014) 9:180. doi: 10.1186/1748-717x-9-180
42. Mondini M, Nizard M, Tran T, Mauge L, Loi M, Clemenson C, et al. Synergy of radiotherapy and a cancer vaccine for the treatment of HPV-associated head and neck cancer. Mol Cancer Ther. (2015) 14:1336–45. doi: 10.1158/1535-7163.mct-14-1015
43. Sebastian M, Papachristofilou A, Weiss C, Fruh M, Cathomas R, Hilbe W, et al. Phase Ib study evaluating a self-adjuvanted mRNA cancer vaccine (RNActive(R)) combined with local radiation as consolidation and maintenance treatment for patients with stage IV non-small cell lung cancer. BMC Cancer (2014) 14:748. doi: 10.1186/1471-2407-14-748
44. Eckert F, Jelas I, Oehme M, Huber SM, Sonntag K, Welker C, et al. Tumor-targeted IL-12 combined with local irradiation leads to systemic tumor control via abscopal effects in vivo. Oncoimmunology (2017) 6:e1323161. doi: 10.1080/2162402x.2017.1323161
45. Eckert F, Schmitt J, Zips D, Krueger MA, Pichler BJ, Gillies SD, et al. Enhanced binding of necrosis-targeting immunocytokine NHS-IL12 after local tumour irradiation in murine xenograft models. Cancer Immunol Immunother. (2016) 65:1003–13. doi: 10.1007/s00262-016-1863-0
46. Schilbach K, Alkhaled M, Welker C, Eckert F, Blank G, Ziegler H, et al. Cancer-targeted IL-12 controls human rhabdomyosarcoma by senescence induction and myogenic differentiation. Oncoimmunology (2015) 4:e1014760. doi: 10.1080/2162402x.2015.1014760
47. Zegers CM, Rekers NH, Quaden DH, Lieuwes NG, Yaromina A, Germeraad WT, et al. Radiotherapy combined with the immunocytokine L19-IL2 provides long-lasting antitumor effects. Clin Cancer Res. (2015) 21:1151–60. doi: 10.1158/1078-0432.ccr-14-2676
48. Peng H, Huang Y, Rose J, Erichsen D, Herek S, Fujii N, et al. Stromal cell-derived factor 1-mediated CXCR4 signaling in rat and human cortical neural progenitor cells. J Neurosci Res. (2004) 76:35–50. doi: 10.1002/jnr.20045
49. Peng H, Kolb R, Kennedy JE, Zheng J. Differential expression of CXCL12 and CXCR4 during human fetal neural progenitor cell differentiation. J Neuroimmune Pharmacol. (2007) 2:251–8. doi: 10.1007/s11481-007-9081-3
50. Carbajal KS, Schaumburg C, Strieter R, Kane J, Lane TE. Migration of engrafted neural stem cells is mediated by CXCL12 signaling through CXCR4 in a viral model of multiple sclerosis. Proc Natl Acad Sci USA. (2010) 107:11068–73. doi: 10.1073/pnas.1006375107
51. Liu G, Yuan X, Zeng Z, Tunici P, Ng H, Abdulkadir IR, et al. Analysis of gene expression and chemoresistance of CD133+ cancer stem cells in glioblastoma. Mol Cancer (2006) 5:67. doi: 10.1186/1476-4598-5-67
52. Salmaggi A, Boiardi A, Gelati M, Russo A, Calatozzolo C, Ciusani E, et al. Glioblastoma-derived tumorospheres identify a population of tumor stem-like cells with angiogenic potential and enhanced multidrug resistance phenotype. Glia (2006) 54:850–60. doi: 10.1002/glia.20414
53. Hattermann K, Held-Feindt J, Lucius R, Muerkoster SS, Penfold ME, Schall TJ, et al. The chemokine receptor CXCR7 is highly expressed in human glioma cells and mediates antiapoptotic effects. Cancer Res. (2010) 70:3299–308. doi: 10.1158/0008-5472.CAN-09-3642
54. Zheng X, Xie Q, Li S, Zhang W. CXCR4-positive subset of glioma is enriched for cancer stem cells. Oncol Res. (2011) 19:555–61.
55. Klumpp L, Sezgin EC, Skardelly M, Eckert F, Huber SM. KCa3.1 channels and glioblastoma: in vitro studies. Curr Neuropharmacol (2017) 16:627–35. doi: 10.2174/1570159X15666170808115821
56. Guadagno E, Vitiello M, Francesca P, Cali G, Caponnetto F, Cesselli D, et al. PATZ1 is a new prognostic marker of glioblastoma associated with the stem-like phenotype and enriched in the proneural subtype. Oncotarget (2017) 8:59282–300. doi: 10.18632/oncotarget.19546
57. Lee CC, Lai JH, Hueng DY, Ma HI, Chung Y, Sun YY, et al. Disrupting the CXCL12/CXCR4 axis disturbs the characteristics of glioblastoma stem-like cells of rat RG2 glioblastoma. Cancer Cell Int. (2013) 13:85. doi: 10.1186/1475-2867-13-85
58. Gatti M, Pattarozzi A, Bajetto A, Wurth R, Daga A, Fiaschi P, et al. Inhibition of CXCL12/CXCR4 autocrine/paracrine loop reduces viability of human glioblastoma stem-like cells affecting self-renewal activity. Toxicology (2013) 314:209–20. doi: 10.1016/j.tox.2013.10.003
59. Gravina GL, Mancini A, Colapietro A, Vitale F, Vetuschi A, Pompili S, et al. The novel CXCR4 antagonist, PRX177561, reduces tumor cell proliferation and accelerates cancer stem cell differentiation in glioblastoma preclinical models. Tumour Biol. (2017) 39:1010428317695528. doi: 10.1177/1010428317695528
60. Nair RM, Balla MM, Khan I, Kalathur RKR, Kondaiah P, Vemuganti GK. In vitro characterization of CD133(lo) cancer stem cells in Retinoblastoma Y79 cell line. BMC Cancer (2017) 17:779. doi: 10.1186/s12885-017-3750-2
61. Mimeault M, Batra SK. Novel biomarkers and therapeutic targets for optimizing the therapeutic management of melanomas. World J Clin Oncol. (2012) 3:32–42. doi: 10.5306/wjco.v3.i3.32
62. Ishiwata T, Matsuda Y, Yoshimura H, Sasaki N, Ishiwata S, Ishikawa N, et al. Pancreatic cancer stem cells: features and detection methods. Pathol Oncol Res. (2018) 24:797–805. doi: 10.1007/s12253-018-0420-x
63. Jung MJ, Rho JK, Kim YM, Jung JE, Jin YB, Ko YG, et al. Upregulation of CXCR4 is functionally crucial for maintenance of stemness in drug-resistant non-small cell lung cancer cells. Oncogene (2013) 32:209–21. doi: 10.1038/onc.2012.37
64. Kumazawa S, Kajiyama H, Umezu T, Mizuno M, Suzuki S, Yamamoto E, et al. Possible association between stem-like hallmark and radioresistance in human cervical carcinoma cells. J Obstet Gynaecol Res. (2014) 40:1389–98. doi: 10.1111/jog.12357
65. Luo J, Ok S, Liang L, Huang CK, Li L, Wen S, et al. Infiltrating bone marrow mesenchymal stem cells increase prostate cancer stem cell population and metastatic ability via secreting cytokines to suppress androgen receptor signaling. Oncogene (2014) 33:2768–78. doi: 10.1038/onc.2013.233
66. Faber A, Hoermann K, Stern-Straeter J, Schultz DJ, Goessler UR. Functional effects of SDF-1alpha on a CD44(+) CXCR4(+) squamous cell carcinoma cell line as a model for interactions in the cancer stem cell niche. Oncol Rep. (2013) 29:579–84. doi: 10.3892/or.2012.2171
67. Ciccarelli C, Vulcano F, Milazzo L, Gravina GL, Marampon F, Macioce G, et al. Key role of MEK/ERK pathway in sustaining tumorigenicity and in vitro radioresistance of embryonal rhabdomyosarcoma stem-like cell population. Mol Cancer (2016) 15:16. doi: 10.1186/s12943-016-0501-y
68. Megiorni F, Gravina GL, Camero S, Ceccarelli S, Del Fattore A, Desiderio V, et al. Pharmacological targeting of the ephrin receptor kinase signalling by GLPG1790 in vitro and in vivo reverts oncophenotype, induces myogenic differentiation and radiosensitizes embryonal rhabdomyosarcoma cells. J Hematol Oncol. (2017) 10:161. doi: 10.1186/s13045-017-0530-z
69. Huang X, Xiong M, Jin Y, Deng C, Xu H, An C, et al. Evidence that high-migration drug-surviving MOLT4 leukemia cells exhibit cancer stem cell-like properties. Int J Oncol. (2016) 49:343–51. doi: 10.3892/ijo.2016.3526
70. Lagadec C, Vlashi E, Della Donna L, Dekmezian C, Pajonk F. Radiation-induced reprogramming of breast cancer cells. Stem Cells (2012) 30:833–44. doi: 10.1002/stem.1058
71. Mimeault M, Batra SK. Altered gene products involved in the malignant reprogramming of cancer stem/progenitor cells and multitargeted therapies. Mol Aspects Med. (2013) 39:3–32. doi: 10.1016/j.mam.2013.08.001
72. Milanovic M, Fan DNY, Belenki D, Dabritz JHM, Zhao Z, Yu Y, et al. Senescence-associated reprogramming promotes cancer stemness. Nature (2018) 553:96–100. doi: 10.1038/nature25167
73. Jamal M, Rath BH, Tsang PS, Camphausen K, Tofilon PJ. The brain microenvironment preferentially enhances the radioresistance of CD133(+) glioblastoma stem-like cells. Neoplasia (2012) 14:150–8. doi: 10.1593/neo.111794
74. Chang SC, Lin PC, Yang SH, Wang HS, Li AF, Lin JK. SDF-1alpha G801A polymorphism predicts lymph node metastasis in stage T3 colorectal cancer. Ann Surg Oncol. (2009) 16:2323–30. doi: 10.1245/s10434-009-0501-x
75. Hayakawa Y, Ariyama H, Stancikova J, Sakitani K, Asfaha S, Renz BW, et al. Mist1 expressing gastric stem cells maintain the normal and neoplastic gastric epithelium and are supported by a perivascular stem cell niche. Cancer Cell (2015) 28:800–14. doi: 10.1016/j.ccell.2015.10.003
76. Roos A, Ding Z, Loftus JC, Tran NL. Molecular and microenvironmental determinants of glioma stem-like cell survival and invasion. Front Oncol. (2017) 7:120. doi: 10.3389/fonc.2017.00120
77. Wang R, Chadalavada K, Wilshire J, Kowalik U, Hovinga KE, Geber A, et al. Glioblastoma stem-like cells give rise to tumour endothelium. Nature (2010) 468:829–33. doi: 10.1038/nature09624
78. Ricci-Vitiani L, Pallini R, Biffoni M, Todaro M, Invernici G, Cenci T, et al. Tumour vascularization via endothelial differentiation of glioblastoma stem-like cells. Nature (2010) 468:824–8. doi: 10.1038/nature09557
79. Cheng L, Huang Z, Zhou W, Wu Q, Donnola S, Liu JK, et al. Glioblastoma stem cells generate vascular pericytes to support vessel function and tumor growth. Cell (2013) 153:139–52. doi: 10.1016/j.cell.2013.02.021
80. Rao S, Sengupta R, Choe EJ, Woerner BM, Jackson E, Sun T, et al. CXCL12 mediates trophic interactions between endothelial and tumor cells in glioblastoma. PLoS ONE (2012) 7:e33005. doi: 10.1371/journal.pone.0033005
81. Yadav VN, Zamler D, Baker GJ, Kadiyala P, Erdreich-Epstein A, DeCarvalho AC, et al. CXCR4 increases in-vivo glioma perivascular invasion, and reduces radiation induced apoptosis: a genetic knockdown study. Oncotarget (2016) 7:83701–19. doi: 10.18632/oncotarget.13295
82. Hira VV, Verbovsek U, Breznik B, Srdic M, Novinec M, Kakar H, et al. Cathepsin K cleavage of SDF-1alpha inhibits its chemotactic activity towards glioblastoma stem-like cells. Biochim Biophys Acta (2017) 1864:594–603. doi: 10.1016/j.bbamcr.2016.12.021
83. Yang SX, Chen JH, Jiang XF, Wang QL, Chen ZQ, Zhao W, et al. Activation of chemokine receptor CXCR4 in malignant glioma cells promotes the production of vascular endothelial growth factor. Biochem Biophys Res Commun. (2005) 335:523–8. doi: 10.1016/j.bbrc.2005.07.113
84. Ping YF, Yao XH, Jiang JY, Zhao LT, Yu SC, Jiang T, et al. The chemokine CXCL12 and its receptor CXCR4 promote glioma stem cell-mediated VEGF production and tumour angiogenesis via PI3K/AKT signalling. J Pathol. (2011) 224:344–54. doi: 10.1002/path.2908
85. Zagzag D, Lukyanov Y, Lan L, Ali MA, Esencay M, Mendez O, et al. Hypoxia-inducible factor 1 and VEGF upregulate CXCR4 in glioblastoma: implications for angiogenesis and glioma cell invasion. Lab Invest. (2006) 86:1221–32. doi: 10.1038/labinvest.3700482
86. Zagzag D, Esencay M, Mendez O, Yee H, Smirnova I, Huang Y, et al. Hypoxia- and vascular endothelial growth factor-induced stromal cell-derived factor-1alpha/CXCR4 expression in glioblastomas: one plausible explanation of Scherer's structures. Am J Pathol. (2008) 173:545–60. doi: 10.2353/ajpath.2008.071197
87. Barone A, Sengupta R, Warrington NM, Smith E, Wen PY, Brekken RA, et al. Combined VEGF and CXCR4 antagonism targets the GBM stem cell population and synergistically improves survival in an intracranial mouse model of glioblastoma. Oncotarget (2014) 5:9811–22. doi: 10.18632/oncotarget.2443
88. Boesch M, Onder L, Cheng HW, Novkovic M, Morbe U, Sopper S, et al. Interleukin 7-expressing fibroblasts promote breast cancer growth through sustenance of tumor cell stemness. Oncoimmunology (2018) 7:e1414129. doi: 10.1080/2162402X.2017.1414129
89. Mohle R, Bautz F, Rafii S, Moore MA, Brugger W, Kanz L. The chemokine receptor CXCR-4 is expressed on CD34+ hematopoietic progenitors and leukemic cells and mediates transendothelial migration induced by stromal cell-derived factor-1. Blood (1998) 91:4523–30.
90. Zeng Z, Shi YX, Samudio IJ, Wang RY, Ling X, Frolova O, et al. Targeting the leukemia microenvironment by CXCR4 inhibition overcomes resistance to kinase inhibitors and chemotherapy in AML. Blood (2009) 113:6215–24. doi: 10.1182/blood-2008-05-158311
91. Nervi B, Ramirez P, Rettig MP, Uy GL, Holt MS, Ritchey JK, et al. Chemosensitization of acute myeloid leukemia (AML) following mobilization by the CXCR4 antagonist AMD3100. Blood (2009) 113:6206–14. doi: 10.1182/blood-2008-06-162123
92. Lee CG, Das B, Lin TL, Grimes C, Zhang X, Lavezzi T, et al. A rare fraction of drug-resistant follicular lymphoma cancer stem cells interacts with follicular dendritic cells to maintain tumourigenic potential. Br J Haematol. (2012) 158:79–90. doi: 10.1111/j.1365-2141.2012.09123.x
93. Goffart N, Kroonen J, Di Valentin E, Dedobbeleer M, Denne A, Martinive P, et al. Adult mouse subventricular zones stimulate glioblastoma stem cells specific invasion through CXCL12/CXCR4 signaling. Neuro Oncol. (2015) 17:81–94. doi: 10.1093/neuonc/nou144
94. Goffart N, Lombard A, Lallemand F, Kroonen J, Nassen J, Di Valentin E, et al. CXCL12 mediates glioblastoma resistance to radiotherapy in the subventricular zone. Neuro Oncol. (2017) 19:66–77. doi: 10.1093/neuonc/now136
95. Pillozzi S, Masselli M, De Lorenzo E, Accordi B, Cilia E, Crociani O, et al. Chemotherapy resistance in acute lymphoblastic leukemia requires hERG1 channels and is overcome by hERG1 blockers. Blood (2011) 117:902–14. doi: 10.1182/blood-2010-01-262691
96. Nakamura T, Shinriki S, Jono H, Guo J, Ueda M, Hayashi M, et al. Intrinsic TGF-beta2-triggered SDF-1-CXCR4 signaling axis is crucial for drug resistance and a slow-cycling state in bone marrow-disseminated tumor cells. Oncotarget (2015) 6:1008–19. doi: 10.18632/oncotarget.2826
97. Cai C, Wang LH, Dong Q, Wu ZJ, Li MY, Sun YH. Association of CXCL12 and CXCR4 gene polymorphisms with the susceptibility and prognosis of renal cell carcinoma. Tissue Antigens. (2013) 82:165–70. doi: 10.1111/tan.12170
98. Isman FK, Kucukgergin C, Dasdemir S, Cakmakoglu B, Sanli O, Seckin S. Association between SDF1-3'A or CXCR4 gene polymorphisms with predisposition to and clinicopathological characteristics of prostate cancer with or without metastases. Mol Biol Rep. (2012) 39:11073–9. doi: 10.1007/s11033-012-2010-4
99. Katayama A, Ogino T, Bandoh N, Nonaka S, Harabuchi Y. Expression of CXCR4 and its down-regulation by IFN-gamma in head and neck squamous cell carcinoma. Clin Cancer Res. (2005) 11:2937–46. doi: 10.1158/1078-0432.CCR-04-1470
100. Chen R, Xu Y, Du X, Liu N, Li Y, He Q, et al. CXCL12 genetic variants as prognostic markers in nasopharyngeal carcinoma. Oncotargets Ther. (2015) 8:2835–42. doi: 10.2147/OTT.S90430
101. Qiao N, Wang L, Wang T, Li H. Inflammatory CXCL12-CXCR4/CXCR7 axis mediates G-protein signaling pathway to influence the invasion and migration of nasopharyngeal carcinoma cells. Tumor Biol. (2016) 37:8169–79. doi: 10.1007/s13277-015-4686-2
102. Rave-Frank M, Tehrany N, Kitz J, Leu M, Weber HE, Burfeind P, et al. Prognostic value of CXCL12 and CXCR4 in inoperable head and neck squamous cell carcinoma. Strahlenther Onkol. (2016) 192:47–54. doi: 10.1007/s00066-015-0892-5
103. Schimanski CC, Jordan M, Schlaegel F, Schmidtmann I, Lang H, Galle PR, et al. SNP rs1801157 significantly correlates with distant metastasis in CXCL12 expressing esophagogastric cancer. Int J Oncol. (2011) 39:515–20. doi: 10.3892/ijo.2011.1044
104. Chang CC, Chen SC, Hsieh YH, Chen YC, Chen TY, Chu YH, et al. Stromal cell-derived factor-1 but not its receptor, CXCR4, gene variants increase susceptibility and pathological development of hepatocellular carcinoma. Clin Chem Lab Med. (2009) 47:412–8. doi: 10.1515/CCLM.2009.092
105. Zhu Y, Tang L, Zhao S, Sun B, Cheng L, Tang Y, et al. CXCR4-mediated osteosarcoma growth and pulmonary metastasis is suppressed by MicroRNA-613. Cancer Sci. (2018) 109:2412–22. doi: 10.1111/cas.13653
106. Saur D, Seidler B, Schneider G, Algul H, Beck R, Senekowitsch-Schmidtke R, et al. CXCR4 expression increases liver and lung metastasis in a mouse model of pancreatic cancer. Gastroenterology (2005) 129:1237–50. doi: 10.1053/j.gastro.2005.06.056
107. Uchida D, Kuribayashi N, Kinouchi M, Sawatani Y, Shimura M, Mori T, et al. Effect of a novel orally bioavailable CXCR4 inhibitor, AMD070, on the metastasis of oral cancer cells. Oncol Rep. (2018) 40:303–8. doi: 10.3892/or.2018.6400
108. Gros SJ, Kurschat N, Drenckhan A, Dohrmann T, Forberich E, Effenberger K, et al. Involvement of CXCR4 chemokine receptor in metastastic HER2-positive esophageal cancer. PLoS ONE (2012) 7:e47287. doi: 10.1371/journal.pone.0047287
109. Muller A, Homey B, Soto H, Ge N, Catron D, Buchanan ME, et al. Involvement of chemokine receptors in breast cancer metastasis. Nature (2001) 410:50–6. doi: 10.1038/35065016
110. Correa D, Somoza RA, Lin P, Schiemann WP, Caplan AI. Mesenchymal stem cells regulate melanoma cancer cells extravasation to bone and liver at their perivascular niche. Int J Cancer (2015) 138:417–27. doi: 10.1002/ijc.29709
111. Li B, Wang Z, Wu H, Xue M, Lin P, Wang S, et al. Epigenetic regulation of CXCL12 plays a critical role in mediating tumor progression and the immune response in osteosarcoma. Cancer Res. (2018) 78:3938–53. doi: 10.1158/0008-5472.CAN-17-3801
112. de Oliveira KB, Guembarovski RL, Guembarovski AM, da Silva do Amaral Herrera AC, Sobrinho WJ, Ariza CB, et al. CXCL12, CXCR4 and IFNgamma genes expression: implications for proinflammatory microenvironment of breast cancer. Clin Exp Med. (2013) 13:211–9. doi: 10.1007/s10238-012-0194-5
113. Clatot F, Picquenot JM, Choussy O, Gouerant S, Moldovan C, Schultheis D, et al. Intratumoural level of SDF-1 correlates with survival in head and neck squamous cell carcinoma. Oral Oncol. (2011) 47:1062–8. doi: 10.1016/j.oraloncology.2011.07.021
114. De-Colle C, Monnich D, Welz S, Boeke S, Sipos B, Fend F, et al. SDF-1/CXCR4 expression in head and neck cancer and outcome after postoperative radiochemotherapy. Clin Transl Radiat Oncol. (2017) 5:28–36. doi: 10.1016/j.ctro.2017.06.004
115. Sciaccaluga M, Fioretti B, Catacuzzeno L, Pagani F, Bertollini C, Rosito M, et al. CXCL12-induced glioblastoma cell migration requires intermediate conductance Ca2+-activated K+ channel activity. Am J Physiol Cell Physiol. (2010) 299:C175–84. doi: 10.1152/ajpcell.00344.2009
116. Edalat L, Stegen B, Klumpp L, Haehl E, Schilbach K, Lukowski R, et al. BK K+ channel blockade inhibits radiation-induced migration/brain infiltration of glioblastoma cells. Oncotarget (2016) 7:14259–78. doi: 10.18632/oncotarget.7423
117. Kingsmore KM, Logsdon DK, Floyd DH, Peirce SM, Purow BW, Munson JM. Interstitial flow differentially increases patient-derived glioblastoma stem cell invasion via CXCR4, CXCL12, and CD44-mediated mechanisms. Integr Biol (Camb). (2016) 8:1246–60. doi: 10.1039/c6ib00167j
118. Pham K, Luo D, Siemann DW, Law BK, Reynolds BA, Hothi P, et al. VEGFR inhibitors upregulate CXCR4 in VEGF receptor-expressing glioblastoma in a TGFbetaR signaling-dependent manner. Cancer Lett. (2015) 360:60–7. doi: 10.1016/j.canlet.2015.02.005
119. Gagner JP, Sarfraz Y, Ortenzi V, Alotaibi FM, Chiriboga LA, Tayyib AT, et al. Multifaceted C-X-C chemokine receptor 4 (CXCR4) inhibition interferes with anti-vascular endothelial growth factor therapy-induced glioma dissemination. Am J Pathol. (2017) 187:2080–94. doi: 10.1016/j.ajpath.2017.04.020
120. Deng L, Stafford JH, Liu SC, Chernikova SB, Merchant M, Recht L, et al. SDF-1 blockade enhances anti-VEGF therapy of glioblastoma and can be monitored by MRI. Neoplasia (2017) 19:1–7. doi: 10.1016/j.neo.2016.11.010
121. Lu KV, Bergers G. Mechanisms of evasive resistance to anti-VEGF therapy in glioblastoma. CNS Oncol. (2013) 2:49–65. doi: 10.2217/cns.12.36
122. Wick W, Wick A, Weiler M, Weller M. Patterns of progression in malignant glioma following anti-VEGF therapy: perceptions and evidence. Curr Neurol Neurosci Rep. (2011) 11:305–12. doi: 10.1007/s11910-011-0184-0
123. Aghi M, Cohen KS, Klein RJ, Scadden DT, Chiocca EA. Tumor stromal-derived factor-1 recruits vascular progenitors to mitotic neovasculature, where microenvironment influences their differentiated phenotypes. Cancer Res. (2006) 66:9054–64. doi: 10.1158/0008-5472.CAN-05-3759
124. Du R, Lu KV, Petritsch C, Liu P, Ganss R, Passegue E, et al. HIF1alpha induces the recruitment of bone marrow-derived vascular modulatory cells to regulate tumor angiogenesis and invasion. Cancer Cell (2008) 13:206–20. doi: 10.1016/j.ccr.2008.01.034
125. Kioi M, Vogel H, Schultz G, Hoffman RM, Harsh GR, Brown JM. Inhibition of vasculogenesis, but not angiogenesis, prevents the recurrence of glioblastoma after irradiation in mice. J Clin Invest. (2010) 120:694–705. doi: 10.1172/jci40283
126. Liu W, Zhu E, Wang R, Wang L, Liu T. CXCL12 G801A polymorphism is associated with an increased risk of benign salivary gland tumors in the Chinese population. Med Oncol. (2012) 29:677–81. doi: 10.1007/s12032-011-9838-7
127. Wang SC, Yu CF, Hong JH, Tsai CS, Chiang CS. Radiation therapy-induced tumor invasiveness is associated with SDF-1-regulated macrophage mobilization and vasculogenesis. PLoS ONE (2013) 8:e69182. doi: 10.1371/journal.pone.0069182
128. Zhu G, Tang Y, Geng N, Zheng M, Jiang J, Li L, et al. HIF-alpha/MIF and NF-kappaB/IL-6 axes contribute to the recruitment of CD11b+Gr-1+ myeloid cells in hypoxic microenvironment of HNSCC. Neoplasia (2014) 16:168–79. doi: 10.1593/neo.132034
129. Peng Q, Liu M, Song SM, Li XH, Du YH, Zhi Y, et al. The recruitment of exogenous endothelial progenitor cells in lung tumor model of nude mice. Chin J Cancer (2010) 29:952–8.
130. Wang Y, Yu H, Shan Y, Tao C, Wu F, Yu Z, et al. EphA1 activation promotes the homing of endothelial progenitor cells to hepatocellular carcinoma for tumor neovascularization through the SDF-1/CXCR4 signaling pathway. J Exp Clin Cancer Res. (2016) 35:65. doi: 10.1186/s13046-016-0339-6
131. Kozin SV, Kamoun WS, Huang Y, Dawson MR, Jain RK, Duda DG. Recruitment of myeloid but not endothelial precursor cells facilitates tumor regrowth after local irradiation. Cancer Res. (2010) 70:5679–85. doi: 10.1158/0008-5472.CAN-09-4446
132. Liu T, Xie C, Ma H, Zhang S, Liang Y, Shi L, et al. Gr-1+CD11b+ cells facilitate Lewis lung cancer recurrence by enhancing neovasculature after local irradiation. Sci Rep. (2014) 4:4833. doi: 10.1038/srep04833
133. Wang HH, Cui YL, Zaorsky NG, Lan J, Deng L, Zeng XL, et al. Mesenchymal stem cells generate pericytes to promote tumor recurrence via vasculogenesis after stereotactic body radiation therapy. Cancer Lett. (2016) 375:349–59. doi: 10.1016/j.canlet.2016.02.033
134. Li F, Sonveaux P, Rabbani ZN, Liu S, Yan B, Huang Q, et al. Regulation of HIF-1alpha stability through S-nitrosylation. Mol Cell. (2007) 26:63–74. doi: 10.1016/j.molcel.2007.02.024
135. Moeller BJ, Cao Y, Li CY, Dewhirst MW. Radiation activates HIF-1 to regulate vascular radiosensitivity in tumors: role of reoxygenation, free radicals, and stress granules. Cancer Cell (2004) 5:429–41. doi: 10.1016/S1535-6108(04)00115-1
136. Lerman OZ, Greives MR, Singh SP, Thanik VD, Chang CC, Seiser N, et al. Low-dose radiation augments vasculogenesis signaling through HIF-1-dependent and -independent SDF-1 induction. Blood (2010) 116:3669–76. doi: 10.1182/blood-2009-03-213629
137. Tabatabai G, Frank B, Mohle R, Weller M, Wick W. Irradiation and hypoxia promote homing of haematopoietic progenitor cells towards gliomas by TGF-beta-dependent HIF-1alpha-mediated induction of CXCL12. Brain (2006) 129(Pt 9):2426–35. doi: 10.1093/brain/awl173
138. Yamauchi Y, Safi S, Orschiedt L, Gardyan A, Brons S, Rieber J, et al. Low-dose photon irradiation induces invasiveness through the SDF-1alpha/CXCR4 pathway in malignant mesothelioma cells. Oncotarget (2017) 8:68001–11. doi: 10.18632/oncotarget.19134
139. Domanska UM, Boer JC, Timmer-Bosscha H, van Vugt MA, Hoving HD, Kliphuis NM, et al. CXCR4 inhibition enhances radiosensitivity, while inducing cancer cell mobilization in a prostate cancer mouse model. Clin Exp Metast. (2014) 31:829–39. doi: 10.1007/s10585-014-9673-2
140. Wang F, Fu Z, Cheng H. Differentially expressed genes in residual cervical cancer after radiotherapy screened by cDNA microarray. Chin Clin Oncol. (2016) 21:41–6. doi: 10.12659/MSM.893689
141. Gu Q, He Y, Ji J, Yao Y, Shen W, Luo J, et al. Hypoxia-inducible factor 1alpha (HIF-1alpha) and reactive oxygen species (ROS) mediates radiation-induced invasiveness through the SDF-1alpha/CXCR4 pathway in non-small cell lung carcinoma cells. Oncotarget (2015) 6:10893–907. doi: 10.18632/oncotarget.3535
142. Chen FH, Fu SY, Yang YC, Wang CC, Chiang CS, Hong JH. Combination of vessel-targeting agents and fractionated radiation therapy: the role of the SDF-1/CXCR4 pathway. Int J Radiat Oncol Biol Phys. (2013) 86:777–84. doi: 10.1016/j.ijrobp.2013.02.036
143. Kim JH, Kolozsvary A, Jenrow KA, Brown SL. Plerixafor, a CXCR4 antagonist, mitigates skin radiation-induced injury in mice. Radiat Res. (2012) 178:202–6. doi: 10.1667/RR2886.1
144. Li D, Qu C, Ning Z, Wang H, Zang K, Zhuang L, et al. Radiation promotes epithelial-to-mesenchymal transition and invasion of pancreatic cancer cell by activating carcinoma-associated fibroblasts. Am J Cancer Res. (2016) 6:2192–206.
145. Ratajczak MZ, Jadczyk T, Schneider G, Kakar SS, Kucia M. Induction of a tumor-metastasis-receptive microenvironment as an unwanted and underestimated side effect of treatment by chemotherapy or radiotherapy. J Ovarian Res. (2013) 6:95. doi: 10.1186/1757-2215-6-95
146. Shu HK, Yoon Y, Hong S, Xu K, Gao H, Hao C, et al. Inhibition of the CXCL12/CXCR4-axis as preventive therapy for radiation-induced pulmonary fibrosis. PLoS ONE (2013) 8:e79768. doi: 10.1371/journal.pone.0079768
147. Yang R, Duan C, Yuan L, Engelbach JA, Tsien CI, Beeman SC, et al. Inhibitors of HIF-1alpha and CXCR4 mitigate the development of radiation necrosis in mouse brain. Int J Radiat Oncol Biol Phys. (2018) 100:1016–25. doi: 10.1016/j.ijrobp.2017.12.257
148. Chaudary N, Pintilie M, Jelveh S, Lindsay P, Hill RP, Milosevic M. Plerixafor improves primary tumor response and reduces metastases in cervical cancer treated with radio-chemotherapy. Clin Cancer Res. (2017) 23:1242–9. doi: 10.1158/1078-0432.CCR-16-1730
149. Liu SC, Alomran R, Chernikova SB, Lartey F, Stafford J, Jang T, et al. Blockade of SDF-1 after irradiation inhibits tumor recurrences of autochthonous brain tumors in rats. Neuro Oncol. (2014) 16:21–8. doi: 10.1093/neuonc/not149
150. Trautmann F, Cojoc M, Kurth I, Melin N, Bouchez LC, Dubrovska A, et al. CXCR4 as biomarker for radioresistant cancer stem cells. Int J Radiat Biol. (2014) 90:687–99. doi: 10.3109/09553002.2014.906766
151. Zhou KX, Xie LH, Peng X, Guo QM, Wu QY, Wang WH, et al. CXCR4 antagonist AMD3100 enhances the response of MDA-MB-231 triple-negative breast cancer cells to ionizing radiation. Cancer Lett. (2018) 418:196–203. doi: 10.1016/j.canlet.2018.01.009
152. Lecavalier-Barsoum M, Chaudary N, Han K, Koritzinsky M, Hill R, Milosevic M. Targeting the CXCL12/CXCR4 pathway and myeloid cells to improve radiation treatment of locally advanced cervical cancer. Int J Cancer (2018) 143:1017–28. doi: 10.1002/ijc.31297
153. Hassan S, Baccarelli A, Salvucci O, Basik M. Plasma stromal cell-derived factor-1: host derived marker predictive of distant metastasis in breast cancer. Clin Cancer Res. (2008) 14:446–54. doi: 10.1158/1078-0432.CCR-07-1189
154. Salmaggi A, Gelati M, Pollo B, Marras C, Silvani A, Balestrini MR, et al. CXCL12 expression is predictive of a shorter time to tumor progression in low-grade glioma: a single-institution study in 50 patients. J Neurooncol. (2005) 74:287–93. doi: 10.1007/s11060-004-7327-y
155. Calatozzolo C, Maderna E, Pollo B, Gelati M, Marras C, Silvani A, et al. Prognostic value of CXCL12 expression in 40 low-grade oligodendrogliomas and oligoastrocytomas. Cancer Biol Ther. (2006) 5:827–32. doi: 10.4161/cbt.5.7.2838
156. Bian XW, Yang SX, Chen JH, Ping YF, Zhou XD, Wang QL, et al. Preferential expression of chemokine receptor CXCR4 by highly malignant human gliomas and its association with poor patient survival. Neurosurgery (2007) 61:570–8; discussion 578–9. doi: 10.1227/01.NEU.0000290905.53685.A2
157. Tang W, Wang X, Chen Y, Zhang J, Chen Y, Lin Z. CXCL12 and CXCR4 as predictive biomarkers of glioma recurrence pattern after total resection. Pathol Biol (Paris) (2015) 63:190–8. doi: 10.1016/j.patbio.2015.07.002
158. Noroozi Karimabad M, Khanamani Falahati-Pour S, Hassanshahi G. Significant role(s) of CXCL12 and the SDF-1 3'A genetic variant in the pathogenesis of multiple sclerosis. Neuroimmunomodulation (2016) 23:197–208. doi: 10.1159/000449427
159. Rath D, Schaeffeler E, Winter S, Hewer J, Muller K, Droppa M, et al. SDF1 polymorphisms influence outcome in patients with symptomatic cardiovascular disease. PLoS ONE (2016) 11:e0161933. doi: 10.1371/journal.pone.0161933
160. Ma X, Shang F, Zhu W, Lin Q. CXCR4 expression varies significantly among different subtypes of glioblastoma multiforme (GBM) and its low expression or hypermethylation might predict favorable overall survival. Expert Rev Neurother. (2017) 17:941–6. doi: 10.1080/14737175.2017.1351299
161. Ramos EA, Grochoski M, Braun-Prado K, Seniski GG, Cavalli IJ, Ribeiro EM, et al. Epigenetic changes of CXCR4 and its ligand CXCL12 as prognostic factors for sporadic breast cancer. PLoS ONE (2011) 6:e29461. doi: 10.1371/journal.pone.0029461
162. Sato N, Matsubayashi H, Fukushima N, Goggins M. The chemokine receptor CXCR4 is regulated by DNA methylation in pancreatic cancer. Cancer Biol Ther. (2005) 4:70–6. doi: 10.4161/cbt.4.1.1378
163. Domanska UM, Kruizinga RC, Nagengast WB, Timmer-Bosscha H, Huls G, de Vries EG, et al. A review on CXCR4/CXCL12 axis in oncology: no place to hide. Eur J Cancer (2013) 49:219–30. doi: 10.1016/j.ejca.2012.05.005
164. Kashyap MK, Kumar D, Jones H, Amaya-Chanaga CI, Choi MY, Melo-Cardenas J, et al. Ulocuplumab (BMS-936564/MDX1338): a fully human anti-CXCR4 antibody induces cell death in chronic lymphocytic leukemia mediated through a reactive oxygen species-dependent pathway. Oncotarget (2016) 7:2809–22. doi: 10.18632/oncotarget.6465
165. Ghobadi A, Holt M, Meier S, Ritchey J, Tahirovic Y, Bridges A, et al. ALT-1188: a new CXCR4 antagonist in development for mobilization of HSPCs. Blood (2013) 122:891.
166. Karpova D, Dauber K, Spohn G, Chudziak D, Wiercinska E, Schulz M, et al. The novel CXCR4 antagonist POL5551 mobilizes hematopoietic stem and progenitor cells with greater efficiency than Plerixafor. Leukemia (2013) 27:2322–31. doi: 10.1038/leu.2013.266
167. Gravina GL, Mancini A, Marampon F, Colapietro A, Delle Monache S, Sferra R, et al. The brain-penetrating CXCR4 antagonist, PRX177561, increases the antitumor effects of bevacizumab and sunitinib in preclinical models of human glioblastoma. J Hematol Oncol. (2017) 10:5. doi: 10.1186/s13045-016-0377-8
168. Stone ND, Dunaway SB, Flexner C, Tierney C, Calandra GB, Becker S, et al. Multiple-dose escalation study of the safety, pharmacokinetics, and biologic activity of oral AMD070, a selective CXCR4 receptor inhibitor, in human subjects. Antimicrob Agents Chemother. (2007) 51:2351–8. doi: 10.1128/AAC.00013-07
169. Karpova D, Brauninger S, Wiercinska E, Kramer A, Stock B, Graff J, et al. Mobilization of hematopoietic stem cells with the novel CXCR4 antagonist POL6326 (balixafortide) in healthy volunteers-results of a dose escalation trial. J Transl Med. (2017) 15:2. doi: 10.1186/s12967-016-1107-2
170. Fricker SP. Physiology and pharmacology of plerixafor. Transfus Med Hemother. (2013) 40:237–45. doi: 10.1159/000354132
171. Bilgin YM, de Greef GE. Plerixafor for stem cell mobilization: the current status. Curr Opin Hematol. (2016) 23:67–71. doi: 10.1097/moh.0000000000000200
172. Teusink A, Pinkard S, Davies S, Mueller M, Jodele S. Plerixafor is safe and efficacious for mobilization of peripheral blood stem cells in pediatric patients. Transfusion (2016) 56:1402–5. doi: 10.1111/trf.13599
173. DiPersio JF, Stadtmauer EA, Nademanee A, Micallef IN, Stiff PJ, Kaufman JL, et al. Plerixafor and G-CSF versus placebo and G-CSF to mobilize hematopoietic stem cells for autologous stem cell transplantation in patients with multiple myeloma. Blood (2009) 113:5720–6. doi: 10.1182/blood-2008-08-174946
174. Ma Q, Jones D, Borghesani PR, Segal RA, Nagasawa T, Kishimoto T, et al. Impaired B-lymphopoiesis, myelopoiesis, and derailed cerebellar neuron migration in CXCR4- and SDF-1-deficient mice. Proc Natl Acad Sci USA. (1998) 95:9448–53.
175. Nagasawa T, Hirota S, Tachibana K, Takakura N, Nishikawa S, Kitamura Y, et al. Defects of B-cell lymphopoiesis and bone-marrow myelopoiesis in mice lacking the CXC chemokine PBSF/SDF-1. Nature (1996) 382:635–8. doi: 10.1038/382635a0
176. Zou YR, Kottmann AH, Kuroda M, Taniuchi I, Littman DR. Function of the chemokine receptor CXCR4 in haematopoiesis and in cerebellar development. Nature (1998) 393:595–9. doi: 10.1038/31269
177. Zirafi O, Kim KA, Standker L, Mohr KB, Sauter D, Heigele A, et al. Discovery and characterization of an endogenous CXCR4 antagonist. Cell Rep. (2015) 11:737–47. doi: 10.1016/j.celrep.2015.03.061
178. Guo Y, Hangoc G, Bian H, Pelus LM, Broxmeyer HE. SDF-1/CXCL12 enhances survival and chemotaxis of murine embryonic stem cells and production of primitive and definitive hematopoietic progenitor cells. Stem Cells (2005) 23:1324–32. doi: 10.1634/stemcells.2005-0085
179. Sugiyama T, Kohara H, Noda M, Nagasawa T. Maintenance of the hematopoietic stem cell pool by CXCL12-CXCR4 chemokine signaling in bone marrow stromal cell niches. Immunity (2006) 25:977–88. doi: 10.1016/j.immuni.2006.10.016
180. Cashman J, Clark-Lewis I, Eaves A, Eaves C. Stromal-derived factor 1 inhibits the cycling of very primitive human hematopoietic cells in vitro and in NOD/SCID mice. Blood (2002) 99:792–9. doi: 10.1182/blood.V99.3.792
181. Nie Y, Han YC, Zou YR. CXCR4 is required for the quiescence of primitive hematopoietic cells. J Exp Med. (2008) 205:777–83. doi: 10.1084/jem.20072513
182. Tzeng YS, Li H, Kang YL, Chen WC, Cheng WC, Lai DM. Loss of Cxcl12/Sdf-1 in adult mice decreases the quiescent state of hematopoietic stem/progenitor cells and alters the pattern of hematopoietic regeneration after myelosuppression. Blood (2011) 117:429–39. doi: 10.1182/blood-2010-01-266833
183. Kortesidis A, Zannettino A, Isenmann S, Shi S, Lapidot T, Gronthos S. Stromal-derived factor-1 promotes the growth, survival, and development of human bone marrow stromal stem cells. Blood (2005) 105:3793–801. doi: 10.1182/blood-2004-11-4349
184. Devine SM, Flomenberg N, Vesole DH, Liesveld J, Weisdorf D, Badel K, et al. Rapid mobilization of CD34+ cells following administration of the CXCR4 antagonist AMD3100 to patients with multiple myeloma and non-Hodgkin's lymphoma. J Clin Oncol. (2004) 22:1095–102. doi: 10.1200/jco.2004.07.131
185. Liles WC, Broxmeyer HE, Rodger E, Wood B, Hubel K, Cooper S, et al. Mobilization of hematopoietic progenitor cells in healthy volunteers by AMD3100, a CXCR4 antagonist. Blood (2003) 102:2728–30. doi: 10.1182/blood-2003-02-0663
186. Broxmeyer HE, Orschell CM, Clapp DW, Hangoc G, Cooper S, Plett PA, et al. Rapid mobilization of murine and human hematopoietic stem and progenitor cells with AMD3100, a CXCR4 antagonist. J Exp Med. (2005) 201:1307–18. doi: 10.1084/jem.20041385
187. Devine SM, Vij R, Rettig M, Todt L, McGlauchlen K, Fisher N, et al. Rapid mobilization of functional donor hematopoietic cells without G-CSF using AMD3100, an antagonist of the CXCR4/SDF-1 interaction. Blood (2008) 112:990–8. doi: 10.1182/blood-2007-12-130179
188. Abraham M, Pereg Y, Bulvik B, Klein S, Mishalian I, Wald H, et al. Single dose of the CXCR4 antagonist BL-8040 induces rapid mobilization for the collection of human CD34(+) cells in healthy volunteers. Clin Cancer Res. (2017) 23:6790–801. doi: 10.1158/1078-0432.ccr-16-2919
189. Karpova D, Ritchey JK, Holt MS, Abou-Ezzi G, Monlish D, Batoon L, et al. Continuous blockade of CXCR4 results in dramatic mobilization and expansion of hematopoietic stem and progenitor cells. Blood (2017) 129:2939–49. doi: 10.1182/blood-2016-10-746909
190. Kiel MJ, Yilmaz OH, Iwashita T, Yilmaz OH, Terhorst C, Morrison SJ. SLAM family receptors distinguish hematopoietic stem and progenitor cells and reveal endothelial niches for stem cells. Cell (2005) 121:1109–21. doi: 10.1016/j.cell.2005.05.026
191. Oguro H, Ding L, Morrison SJ. SLAM family markers resolve functionally distinct subpopulations of hematopoietic stem cells and multipotent progenitors. Cell Stem Cell. (2013) 13:102–16. doi: 10.1016/j.stem.2013.05.014
192. Beider K, Begin M, Abraham M, Wald H, Weiss ID, Wald O, et al. CXCR4 antagonist 4F-benzoyl-TN14003 inhibits leukemia and multiple myeloma tumor growth. Exp Hematol. (2011) 39:282–92. doi: 10.1016/j.exphem.2010.11.010
193. Passaro D, Irigoyen M, Catherinet C, Gachet S, Da Costa De Jesus C, Lasgi C, et al. CXCR4 is required for leukemia-initiating cell activity in T cell acute lymphoblastic leukemia. Cancer Cell (2015) 27:769–79. doi: 10.1016/j.ccell.2015.05.003
194. Pitt LA, Tikhonova AN, Hu H, Trimarchi T, King B, Gong Y, et al. CXCL12-producing vascular endothelial niches control acute T cell leukemia maintenance. Cancer Cell (2015) 27:755–68. doi: 10.1016/j.ccell.2015.05.002
195. Terhune J, Berk E, Czerniecki BJ. Dendritic cell-induced Th1 and Th17 cell differentiation for cancer therapy. Vaccines (Basel) (2013) 1:527–49. doi: 10.3390/vaccines1040527
196. Kabashima K, Sugita K, Shiraishi N, Tamamura H, Fujii N, Tokura Y. CXCR4 engagement promotes dendritic cell survival and maturation. Biochem Biophys Res Commun. (2007) 361:1012–6. doi: 10.1016/j.bbrc.2007.07.128
197. Kohara H, Omatsu Y, Sugiyama T, Noda M, Fujii N, Nagasawa T. Development of plasmacytoid dendritic cells in bone marrow stromal cell niches requires CXCL12-CXCR4 chemokine signaling. Blood (2007) 110:4153–60. doi: 10.1182/blood-2007-04-084210
198. Kabashima K, Shiraishi N, Sugita K, Mori T, Onoue A, Kobayashi M, et al. CXCL12-CXCR4 engagement is required for migration of cutaneous dendritic cells. Am J Pathol. (2007) 171:1249–57. doi: 10.2353/ajpath.2007.070225
199. Bryant J, Ahern DJ, Brennan FM. CXCR4 and vascular cell adhesion molecule 1 are key chemokine/adhesion receptors in the migration of cytokine-activated T cells. Arthritis Rheum. (2012) 64:2137–46. doi: 10.1002/art.34394
200. Moll NM, Cossoy MB, Fisher E, Staugaitis SM, Tucky BH, Rietsch AM, et al. Imaging correlates of leukocyte accumulation and CXCR4/CXCL12 in multiple sclerosis. Arch Neurol. (2009) 66:44–53. doi: 10.1001/archneurol.2008.512
201. Wang A, Guilpain P, Chong BF, Chouzenoux S, Guillevin L, Du Y, et al. Dysregulated expression of CXCR4/CXCL12 in subsets of patients with systemic lupus erythematosus. Arthritis Rheum. (2010) 62:3436–46. doi: 10.1002/art.27685
202. Gabrilovich DI. Myeloid-derived suppressor cells. Cancer Immunol Res. (2017) 5:3–8. doi: 10.1158/2326-6066.cir-16-0297
203. Deng Y, Cheng J, Fu B, Liu W, Chen G, Zhang Q, et al. Hepatic carcinoma-associated fibroblasts enhance immune suppression by facilitating the generation of myeloid-derived suppressor cells. Oncogene (2017) 36:1090–1101. doi: 10.1038/onc.2016.273
204. Benedicto A, Romayor I, Arteta B. CXCR4 receptor blockage reduces the contribution of tumor and stromal cells to the metastatic growth in the liver. Oncol Rep. (2018) 39:2022–30. doi: 10.3892/or.2018.6254
205. Wolf AM, Wolf D, Steurer M, Gastl G, Gunsilius E, Grubeck-Loebenstein B. Increase of regulatory T cells in the peripheral blood of cancer patients. Clin Cancer Res. (2003) 9:606–12.
206. Curotto de Lafaille MA, Lafaille JJ. Natural and adaptive foxp3+ regulatory T cells: more of the same or a division of labor? Immunity (2009) 30:626–35. doi: 10.1016/j.immuni.2009.05.002
207. Curiel TJ, Coukos G, Zou L, Alvarez X, Cheng P, Mottram P, et al. Specific recruitment of regulatory T cells in ovarian carcinoma fosters immune privilege and predicts reduced survival. Nat Med. (2004) 10:942–9. doi: 10.1038/nm1093
208. Schmidt A, Zhang XM, Joshi RN, Iqbal S, Wahlund C, Gabrielsson S, et al. Human macrophages induce CD4(+)Foxp3(+) regulatory T cells via binding and re-release of TGF-beta. Immunol Cell Biol. (2016) 94:747–62. doi: 10.1038/icb.2016.34
209. Collison LW, Workman CJ, Kuo TT, Boyd K, Wang Y, Vignali KM, et al. The inhibitory cytokine IL-35 contributes to regulatory T-cell function. Nature. (2007) 450:566–9. doi: 10.1038/nature06306
210. Olson BM, Jankowska-Gan E, Becker JT, Vignali DA, Burlingham WJ, McNeel DG. Human prostate tumor antigen-specific CD8+ regulatory T cells are inhibited by CTLA-4 or IL-35 blockade. J Immunol. (2012) 189:5590–601. doi: 10.4049/jimmunol.1201744
211. Turnis ME, Sawant DV, Szymczak-Workman AL, Andrews LP, Delgoffe GM, Yano H, et al. Interleukin-35 limits anti-tumor immunity. Immunity (2016) 44:316–29. doi: 10.1016/j.immuni.2016.01.013
212. Wang Z, Liu JQ, Liu Z, Shen R, Zhang G, Xu J, et al. Tumor-derived IL-35 promotes tumor growth by enhancing myeloid cell accumulation and angiogenesis. J Immunol. (2013) 190:2415–23. doi: 10.4049/jimmunol.1202535
213. Cao X, Cai SF, Fehniger TA, Song J, Collins LI, Piwnica-Worms DR, et al. Granzyme B and perforin are important for regulatory T cell-mediated suppression of tumor clearance. Immunity (2007) 27:635–46. doi: 10.1016/j.immuni.2007.08.014
214. Borsellino G, Kleinewietfeld M, Di Mitri D, Sternjak A, Diamantini A, Giometto R, et al. Expression of ectonucleotidase CD39 by Foxp3+ Treg cells: hydrolysis of extracellular ATP and immune suppression. Blood (2007) 110:1225–32. doi: 10.1182/blood-2006-12-064527
215. Liang B, Workman C, Lee J, Chew C, Dale BM, Colonna L, et al. Regulatory T cells inhibit dendritic cells by lymphocyte activation gene-3 engagement of MHC class II. J Immunol. (2008) 180:5916–26. doi: 10.4049/jimmunol.180.9.5916
216. Qureshi OS, Zheng Y, Nakamura K, Attridge K, Manzotti C, Schmidt EM, et al. Trans-endocytosis of CD80 and CD86: a molecular basis for the cell-extrinsic function of CTLA-4. Science (2011) 332:600–3. doi: 10.1126/science.1202947
217. Wing K, Onishi Y, Prieto-Martin P, Yamaguchi T, Miyara M, Fehervari Z, et al. CTLA-4 control over Foxp3+ regulatory T cell function. Science (2008) 322:271–5. doi: 10.1126/science.1160062
218. Deaglio S, Dwyer KM, Gao W, Friedman D, Usheva A, Erat A, et al. Adenosine generation catalyzed by CD39 and CD73 expressed on regulatory T cells mediates immune suppression. J Exp Med. (2007) 204:1257–65. doi: 10.1084/jem.20062512
219. Pandiyan P, Zheng L, Ishihara S, Reed J, Lenardo MJ. CD4+CD25+Foxp3+ regulatory T cells induce cytokine deprivation-mediated apoptosis of effector CD4+ T cells. Nat Immunol. (2007) 8:1353–62. doi: 10.1038/ni1536
220. Fallarino F, Grohmann U, Hwang KW, Orabona C, Vacca C, Bianchi R, et al. Modulation of tryptophan catabolism by regulatory T cells. Nat Immunol. (2003) 4:1206–12. doi: 10.1038/ni1003
221. Cederbom L, Hall H, Ivars F. CD4+CD25+ regulatory T cells down-regulate co-stimulatory molecules on antigen-presenting cells. Eur J Immunol. (2000) 30:1538–43. doi: 10.1002/1521-4141(200006)30:6<1538::aid-immu1538>3.0.co;2-x
222. Delgoffe GM, Woo SR, Turnis ME, Gravano DM, Guy C, Overacre AE, et al. Stability and function of regulatory T cells is maintained by a neuropilin-1-semaphorin-4a axis. Nature (2013) 501:252–6. doi: 10.1038/nature12428
223. Teng MW, Ngiow SF, von Scheidt B, McLaughlin N, Sparwasser T, Smyth MJ. Conditional regulatory T-cell depletion releases adaptive immunity preventing carcinogenesis and suppressing established tumor growth. Cancer Res. (2010) 70:7800–9. doi: 10.1158/0008-5472.can-10-1681
224. Teng MW, Swann JB, von Scheidt B, Sharkey J, Zerafa N, McLaughlin N, et al. Multiple antitumor mechanisms downstream of prophylactic regulatory T-cell depletion. Cancer Res. (2010) 70:2665–74. doi: 10.1158/0008-5472.can-09-1574
225. Colombo MP, Piconese S. Regulatory-T-cell inhibition versus depletion: the right choice in cancer immunotherapy. Nat Rev Cancer (2007) 7:880–7. doi: 10.1038/nrc2250
226. Righi E, Kashiwagi S, Yuan J, Santosuosso M, Leblanc P, Ingraham R, et al. CXCL12/CXCR4 blockade induces multimodal antitumor effects that prolong survival in an immunocompetent mouse model of ovarian cancer. Cancer Res. (2011) 71:5522–34. doi: 10.1158/0008-5472.can-10-3143
227. Gaur P, Verma V, Gupta S, Sorani E, Haras AV, Oberkovitz G, et al. CXCR4 antagonist (BL-8040) to enhance antitumor effects by increasing tumor infiltration of antigen-specific effector T-cells. J Clin Oncol. (2018) 36(5_Suppl.):73. doi: 10.1200/JCO.2018.36.5_suppl.73
228. Portella L, Vitale R, De Luca S, D'Alterio C, Ierano C, Napolitano M, et al. Preclinical development of a novel class of CXCR4 antagonist impairing solid tumors growth and metastases. PLoS ONE (2013) 8:e74548. doi: 10.1371/journal.pone.0074548
229. Santagata S, Napolitano M, D'Alterio C, Desicato S, Maro SD, Marinelli L, et al. Targeting CXCR4 reverts the suppressive activity of T-regulatory cells in renal cancer. Oncotarget (2017) 8:77110–20. doi: 10.18632/oncotarget.20363
230. Molon B, Gri G, Bettella M, Gomez-Mouton C, Lanzavecchia A, Martinez AC, et al. T cell costimulation by chemokine receptors. Nat Immunol. (2005) 6:465–71. doi: 10.1038/ni1191
231. Kallikourdis M, Trovato AE, Anselmi F, Sarukhan A, Roselli G, Tassone L, et al. The CXCR4 mutations in WHIM syndrome impair the stability of the T-cell immunologic synapse. Blood (2013) 122:666–73. doi: 10.1182/blood-2012-10-461830
232. Chaix J, Nish SA, Lin WH, Rothman NJ, Ding L, Wherry EJ, et al. Cutting edge: CXCR4 is critical for CD8+ memory T cell homeostatic self-renewal but not rechallenge self-renewal. J Immunol. (2014) 193:1013–6. doi: 10.4049/jimmunol.1400488
233. Abraham M, Mishalian I, Harel Y, Klein S, Pereg Y, Oberkovitz G, et al. Effect of BL-8040, high-affinity CXCR4 antagonist, on T-cell infiltration, tumor growth, and synergy with immunomodulatory agents. J Clin Oncol. (2017) 35(15_Suppl.):e14544. doi: 10.1200/JCO.2017.35.15_suppl.e14544
234. Tuve S, Chen BM, Liu Y, Cheng TL, Toure P, Sow PS, et al. Combination of tumor site-located CTL-associated antigen-4 blockade and systemic regulatory T-cell depletion induces tumor-destructive immune responses. Cancer Res. (2007) 67:5929–39. doi: 10.1158/0008-5472.can-06-4296
235. Yoshimura K, Laird LS, Chia CY, Meckel KF, Slansky JE, Thompson JM, et al. Live attenuated Listeria monocytogenes effectively treats hepatic colorectal cancer metastases and is strongly enhanced by depletion of regulatory T cells. Cancer Res. (2007) 67:10058–66. doi: 10.1158/0008-5472.can-07-0573
236. Vianello F, Papeta N, Chen T, Kraft P, White N, Hart WK, et al. Murine B16 melanomas expressing high levels of the chemokine stromal-derived factor-1/CXCL12 induce tumor-specific T cell chemorepulsion and escape from immune control. J Immunol. (2006) 176:2902–14. doi: 10.4049/jimmunol.176.9.5683-a
237. Bernardini G, Sciume G, Santoni A. Differential chemotactic receptor requirements for NK cell subset trafficking into bone marrow. Front Immunol. (2013) 4:12. doi: 10.3389/fimmu.2013.00012
238. Bernardini G, Sciume G, Bosisio D, Morrone S, Sozzani S, Santoni A. CCL3 and CXCL12 regulate trafficking of mouse bone marrow NK cell subsets. Blood (2008) 111:3626–34. doi: 10.1182/blood-2007-08-106203
239. Yang J, Kumar A, Vilgelm AE, Chen SC, Ayers GD, Novitskiy SV, et al. Loss of CXCR4 in myeloid cells enhances antitumor immunity and reduces melanoma growth through NK cell and FASL mechanisms. Cancer Immunol Res. (2018) 6:1186–98. doi: 10.1158/2326-6066.CIR-18-0045
240. Obermajer N, Muthuswamy R, Odunsi K, Edwards RP, Kalinski P. PGE(2)-induced CXCL12 production and CXCR4 expression controls the accumulation of human MDSCs in ovarian cancer environment. Cancer Res. (2011) 71:7463–70. doi: 10.1158/0008-5472.can-11-2449
241. Chen Y, Ramjiawan RR, Reiberger T, Ng MR, Hato T, Huang Y, et al. CXCR4 inhibition in tumor microenvironment facilitates anti-programmed death receptor-1 immunotherapy in sorafenib-treated hepatocellular carcinoma in mice. Hepatology (2015) 61:1591–602. doi: 10.1002/hep.27665
Keywords: immunotherapy, cancer radiotherapy, CXCR4, SDF-1 (CXCL12), T cells, dendritic cells, NK cells, regulatory T cells
Citation: Eckert F, Schilbach K, Klumpp L, Bardoscia L, Sezgin EC, Schwab M, Zips D and Huber SM (2018) Potential Role of CXCR4 Targeting in the Context of Radiotherapy and Immunotherapy of Cancer. Front. Immunol. 9:3018. doi: 10.3389/fimmu.2018.03018
Received: 29 August 2018; Accepted: 06 December 2018;
Published: 21 December 2018.
Edited by:
Christian Ostheimer, Martin Luther University of Halle-Wittenberg, GermanyReviewed by:
Luis De La Cruz-Merino, Hospital Universitario Virgen Macarena, SpainMagdalena Plebanski, RMIT University, Australia
Viktor Umansky, German Cancer Research Center (DKFZ), Germany
Copyright © 2018 Eckert, Schilbach, Klumpp, Bardoscia, Sezgin, Schwab, Zips and Huber. This is an open-access article distributed under the terms of the Creative Commons Attribution License (CC BY). The use, distribution or reproduction in other forums is permitted, provided the original author(s) and the copyright owner(s) are credited and that the original publication in this journal is cited, in accordance with accepted academic practice. No use, distribution or reproduction is permitted which does not comply with these terms.
*Correspondence: Franziska Eckert, ZnJhbnppc2thLmVja2VydEBtZWQudW5pLXR1ZWJpbmdlbi5kZQ==