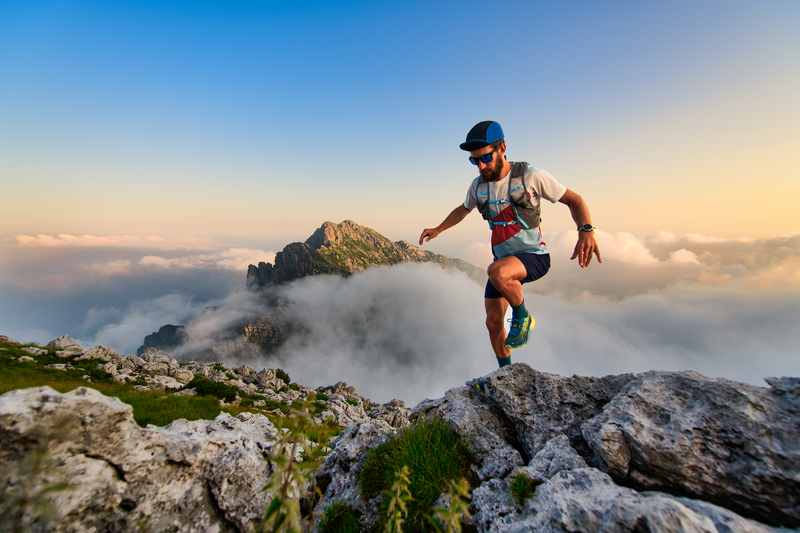
94% of researchers rate our articles as excellent or good
Learn more about the work of our research integrity team to safeguard the quality of each article we publish.
Find out more
REVIEW article
Front. Immunol. , 06 November 2018
Sec. Microbial Immunology
Volume 9 - 2018 | https://doi.org/10.3389/fimmu.2018.02549
This article is part of the Research Topic Update on the Immune Mechanisms Against Respiratory Pathogens View all 29 articles
Pulmonary aspergillosis is a severe infectious disease caused by some members of the Aspergillus genus, that affects immunocompetent as well as immunocompromised patients. Among the different disease forms, Invasive Aspergillosis is the one causing the highest mortality, mainly, although not exclusively, affecting neutropenic patients. This genus is very well known by humans, since different sectors like pharmaceutical or food industry have taken advantage of the biological activity of some molecules synthetized by the fungus, known as secondary metabolites, including statins, antibiotics, fermentative compounds or colorants among others. However, during infection, in response to a hostile host environment, the fungal secondary metabolism is activated, producing different virulence factors to increase its survival chances. Some of these factors also contribute to fungal dissemination and invasion of adjacent and distant organs. Among the different secondary metabolites produced by Aspergillus spp. Gliotoxin (GT) is the best known and better characterized virulence factor. It is able to generate reactive oxygen species (ROS) due to the disulfide bridge present in its structure. It also presents immunosuppressive activity related with its ability to kill mammalian cells and/or inactivate critical immune signaling pathways like NFkB. In this comprehensive review, we will briefly give an overview of the lung immune response against Aspergillus as a preface to analyse the effect of different secondary metabolites on the host immune response, with a special attention to GT. We will discuss the results reported in the literature on the context of the animal models employed to analyse the role of GT as virulence factor, which is expected to greatly depend on the immune status of the host: why should you hide when nobody is seeking for you? Finally, GT immunosuppressive activity will be related with different human diseases predisposing to invasive aspergillosis in order to have a global view on the potential of GT to be used as a target to treat IA.
The genus Aspergillus comprise different saprophytic fungal species with a high environmental prevalence that, under specific circumstances, might infect humans and other animals causing different infectious diseases. Among them Aspergillus fumigatus is a well-known human pathogen, responsible for an important morbimortality in immunocompromised and immunocompetent patients like cancer, transplanted, COPD and critically ill patients (1–3). It causes several diseases including invasive aspergillosis (IA), chronic pulmonary aspergillosis (CPA) and allergic bronchopulmonary aspergillosis (ABPA) (4).
Among them IA is a common cause of mortality in patients with hematological malignancies and it is an emerging problem for solid organ transplant recipients, critical care patients and those receiving immunomodulatory therapies, with mortality rates ranging between 30 to 90% (1–3).
In order to colonize the host, A. fumigatus must use different evasion strategies to avoid the host protective response. These include physicochemical and anatomical barriers of the respiratory track like enzymes, mucus or epithelial cells as well as others that prevent spore and hyphae clearance by innate and adaptive immune system. Among these strategies the production of mycotoxins and other substances with immunosuppressive activity has been the focus of extensive research during the last years, although in most cases, the biological relevance of the findings has not been completely clarified. In this short review we will first summarize the main strategies used by the host to fight Aspergillus within the respiratory track, focusing on cellular innate and adaptive immune responses. Subsequently, we will present the main mycotoxins and products of the secondary metabolism with potential immunosuppressive activity. We will pay special attention to Gliotoxin (GT) that has been shown to affect a great variety of innate and adaptive immune responses and act as a virulence factor in vivo in mouse models (5). Finally, we will discuss unsolved questions and future directions to be addressed on the field, with special attention in the potential of immunosuppressive mycotoxins to exacerbate infection (act as virulence factors) depending on the immunosuppressive host status.
The respiratory system is formed by the upper respiratory tract, nasal cavity, pharynx, larynx, the lower respiratory tract, trachea, bronchi, bronchioles and the respiratory zone represented by alveoli. To carry out gaseous exchange, the respiratory system is exposed daily to thousands liters of air, introducing numerous particles and potentially harmful microorganisms to the alveolar surface (6). To avoid injuries and infections, the respiratory tree has various defense mechanisms such as cough and the mucociliary transport system, formed by four major cell types that produce a physico-chemical barrier against microorganisms, including ciliated cells, mucus-secreting cells and basal cells (7). Nevertheless, if the potentially harmful microorganisms manage to overcome these elements, the bronchial tree still presents different defense mechanisms consisting of soluble molecules and humoral and cellular factors belonging to the innate and adaptive immune system.
Inhalation of Aspergillus spp. conidia is very frequent, because Aspergillus species are found in decomposing vegetation, soil, water, food and air. However, immunocompetent individuals are capable to eliminate Aspergillus conidia by different immune mechanisms, preventing germination and fungal growth (8, 9) (Figure).
Resident alveolar macrophages (AM) and epithelial cells interact with germinating Aspergillus spores in the lung. These cells recognize pathogen-associated molecular patterns (PAMPs) present in fungal surface like galactomannan and β-1,3-glucan among others, through pathogen-recognition receptors (PRR) such as Toll-like receptors (specially TLR-1,−3,−4, and-6), the C-type lectin receptor-Dectin-1 (9) or Nod-like receptors (10). Aspergillus recognition leads to the generation of proinflammatory cytokines like IL-1α, IL-1β, TNF-α, IL-8, and MIP-1α by activation of the NFkB and inflammasome pathways (10–12).
AM are also capable of eliminating directly conidia and initiate an inflammatory response to fungal infection. AM phagocytose conidia and kill them using different mechanisms including acidification of the phagolysosome and activation of antimicrobial enzymes (cathepsin D and chitinase), and the production of reactive oxygen species (ROS) (13, 14). Chemokines and proinflammatory cytokines act as chemoattractants and activators for other immune cells including neutrophils, Natural Killer and T cells that will arrive to the infected site to fight infection and prevent host colonization. The role of these receptors and cytokines in humans mainly proceed from studies showing a higher risk of IA in patients presenting Singe Nucleotide Polymorphisms (SNPs) for these genes (15, 16). Paradoxically, others like NOD2 SNP, decrease the risk of IA in Stem-Cell transplanted (SCT) patients (17).
After epithelial cells and resident AM initiate the inflammatory response, neutrophils are among the first cells arriving at the infected site. These cells have also been found to be critical during the immune defense against Aspergillus spp. both mouse models and humans. This role was mainly characterized in patients treated with neutropenia-inducing drugs as well as in those presenting mutations in molecules involved in neutrophil activity like NADP oxidases (18). Neutrophils are attracted to the site of infection by chemokines and cytokines, especially IL-8 and IL-17, albeit as indicated below, the role of IL-17 during IA is not clear (19–23). Apart from enhancing the inflammatory response by producing cytokines and chemokines, these cells can directly phagocyte and kill the fungus by the production of ROS and antimicrobial compounds (24). Neutrophils have another antifungal mechanism, the neutrophil extracellular traps (NET). NETs are formed when neutrophils release DNA, histones, and granular proteins, including calprotectin and PTX3 into the surrounding environment after autolysis, avoiding the progression of infection (24). Indeed, a recent study has shown that Stem Cell transplanted patients with a SNPs in PTX3 present a higher risk of IA (25). The role of NET formation in IA has also been demonstrated in patients with chronic granulomatous disease who received a gene therapy to restore NET and may resolve a preexisting pulmonary aspergillosis (26). Activated neutrophils also amplified immune response producing cytokines like IL-12 and IL-18 (24).
AM and neutrophils express cytokines and chemokines that attract antigen-presenting cells (APC) like dendritic cells (DC) and monocytes from the blood and surrounding tissues to the infection site. DCs link innate and adaptive immune response to fungal infection (27). They are a heterogeneous population characterized by the expression of different specific surface markers. Three main groups have been established: conventional DCs (cDCs), plasmacytoid DCs (pDCs), and monocyte-derived dendritic cells (moDCs). DCs are responsible for capturing, processing and presenting antigens associated to HLA-I/-II (MHC-I/-II) to CD8+ and CD4+ T cells, respectively, in the lymph nodes. This interaction leads to the generation of CD4+ Th-1, Th-2, Treg or Th17 subsets that regulate different immune responses. DCs also provide co-stimulatory signals (CD86, CD80) and secrete IL-12, a cytokine necessary for acquisition of cytotoxic activity in CD8 T+ cells (28). DCs have also been involved in NK cell activation during IA by the expression of SYK and IL2RA (29). It has been reported that pDCs play an important role in vivo during the control of infection, since its depletion in mice increased susceptibility to IA (30). Intriguingly, these authors also demonstrated that pDCs were able to directly inhibit the growth of A. fumigatus hyphae.
During Aspergillus infection monocytes migrate to the lungs where they differentiate into moDC. It has been demonstrated that moDCs are important for the maintenance and development of protective Th1 cell response against A. fumigatus (31). Monocytes are capable to recognize PAMPs in conidia and hyphae during A. fumigatus infection increasing the expression of several cytokines and chemokines. It has been recently described a new member of the C-type lectin receptor family, MelLec, expressed by endothelial, epithelial and myeloid cells, that recognizes DHN-melanin in Aspergillus conidia and is critical for host protection in a mouse model of IA (32). The relevance of these findings in humans was provided by showing that a SNP in MelLec increased the risk of IA in SCT patients.
In addition, it was found that monocytes may contribute to thrombosis and local lung tissue injury during A. fumigatus infection, increasing the expression of urokinase type plasminogen activator (uPA), urokinase type plasminogen activator receptor (uPAR), plasminogen activator inhibitor (PAI), pentraxin-3 (PTX3) and intercellular adhesion molecule-1 (ICAM-1) (33). Some evidence indicates that Natural Killer cells (NK cells) are involved in the control of Aspergillus infection. In vitro studies have demonstrated that NK cells exhibit antifungal activity against hyphal form of A. fumigatus but are not able to exhibit fungicidal activity against conidia (34). Another study reported that antifungal activity of NK cells against Aspergillus was IFN-γ-mediated and was independent of their cytotoxic mechanisms (35). In vivo studies have shown the important role of NK cells during Aspergillus infection. In a mouse model of Aspergillosis in neutropenic mice it has been demonstrated the beneficial effect of transference of NK cells (36). NK-cell-derived interferon (IFN)-γ also contributes to control infection activating macrophage-dependent fungal clearance mechanisms (37). NK cells have been shown to interact with neutrophils. NK cells activated by Aspergillus express TNF-α, IFN-γ and GM-CSF which directly stimulate neutrophil activation (38). However, so far it has not been identified any genetic deficiency, like Natural Cell Receptors SNPs, linking NK cells with IA susceptibility in humans, which would confirm such a role.
Other innate immune cells including mast cells, basophils, and eosinophils may contribute to fungal protection. The role of mast cells in Aspergillus infection is poorly understood. An in vitro study found that A. fumigatus hyphae induced degranulation of mast cells via an IgE-independent mechanism (39). However the biological relevance of this finding remains to be established (40). The role of eosinophils in Aspergillus infection has been established using mice that exhibit a selective deficiency in eosinophils. These mice showed impairment in A. fumigatus clearance and evidence of germinating organisms in the lung (41).
The innate immunity response during Aspergillus infection triggers the development of an acquired immune response inducing the differentiation of CD4 T helper cells into Th1, Th2, Th17, or Treg cell phenotypes which contribute to IA protection. However, the relative role of each subset is still a matter of controversy.
Th1 cells may improve the antifungal activity of macrophages and neutrophils in the site of infection throw the expression of proinflammatory cytokines TNF-α and IFN-γ (42). Furthermore, in healthy individuals it has been demonstrated the predominance of Th1 response against A. fumigatus employing peripheral blood (43). On the other hand, Th2 cells do not seem to play a protective role during A. fumigatus infection. In contrast, these cells may activate M2 macrophages and decreased Th1 cell response, which could be detrimental in patients with severe fungal infections (44). In contrast, in patients with allergic bronchopulmonary aspergillosis (ABPA), A. fumigatus-specific Th2 CD4+ T cells are predominant (45) and a recent work has identified SNPs in genes related with Th2 responses like IL13 and IL4R that increase ABPA susceptibility (46).
The role of Th17 cell response during A. fumigatus infection is controversial. In a mouse model of A. fumigatus infection, it has been reported that IL-17 and IL-23 do not play a protective role due to its ability to negatively regulate the development of Th1 cells and to affect the neutrophil antifungal activity in vitro (19). Supporting this conclusion they showed that in vivo blocking of IL-23 and IL-17 increased infection clearance (19). In contrast, another mouse model of A. fumigatus infection showed a protective role of IL-17. In this model, in vivo neutralization of IL-17 early during infection increased fungal pulmonary burden (21). Concerning humans, it was found low frequency of IL17 producing cells and high frequency of IFN-γ producing cells after Ag-stimulation of PBMCs from healthy donors (22) or from patients with IA (23). Intriguingly, the last work also found a marked induction of IL-10 producing cells, which could modulate the generation of specific CD8+ T cell activity among other responses. More recently, it was found that meanwhile T cells from peripheral blood from IA patients showed a Th1 IFN-γ producing profile, the majority of lung-derived Aspergillus-specific T-cells displayed a Th17 phenotype, and only low percentages of cells produced IFN-γ. However, it has been shown that SNPs in the IFN-γ gene increases the susceptibility to IA in SCT patients (16). These results indicate that during A. fumigatus infection both Th1 and Th17 cell responses may play an important role in host immunity.
Treg cells may play a protective role during A. fumigatus infections modulating the exacerbated inflammation due to a strong Th1 response in early stage of A. fumigatus infection as well as hypersensitivity reactions associated with Th2 responses in later stages (47, 48).
CD8+ T cell response may play a protective role during A. fumigatus infection. In a mouse model of A. fumigatus infection, it has been observed an increment of IFN-γ-producing CD8+ T cells in bronchoalveolar fluids of mice repeatedly challenged with A. fumigatus conidia with the maintenance of airway memory phenotype CD8+ T cells (49). However, functional evidences of such role were not investigated.
Aspergillus species produce a large number of secondary metabolites that are not critical for its life cycle, but confer competitive survival advantages. These metabolites include aflatoxins, naptho-γ-pyrones, ochratoxins, cyclopiazonic acid, fumonisins, patulin, gliotoxin, kojic acid, malformins, emodin, bicoumarins, csypyrone B1, DHBA, nitropropionic acid, aflatrem, ophiobolins, etc. Many of these compounds exhibit interesting biological properties like antibiotic, anti-carcinogenic or anti-inflammatory activity (50). Thus, Aspergillus spp. are used as biological factories with a great range of applications in food, textile or pharmaceutical industry.
Within these metabolites, mycotoxins have focused special attention due to its toxicity, carcinogenic and/or immunosuppressive activity for both humans and livestock. Among them, fumifungin, fumiquinazoline A/B and D, fumitremorgin B, gliotoxin, sphingofungins, pseurotins, and verruculogen are found in A. fumigatus, being gliotoxin (GT) the most abundant and best characterized mycotoxin produced by A. fumigatus (51).
In Table 1 the main secondary metabolites and mycotoxins with immunosuppressive activity are summarized, including the main Aspegillus spp. producing them and the effect on host immunity. Notably, aflatoxins, ochratoxin, and gliotoxin are the most extensive studied and, thus, for which more immunosuppressive activities have been described. It is worth to note that in all cases these compounds mainly affect innate macrophage and neutrophil responses, especially the pro-inflammatory response, highlighting the importance of these cells in the elimination and prevention of Aspergillus infection as described above.
In several cases the immunosuppressive activity of these compounds has been related to its toxicity against immune cells like some aflatoxins, ochratoxin, gliotoxin or sterigmatocystin. Ochratoxin A is toxic for several immune cells in vitro as well as in vivo in different animal models, causing the reduction in the size of different immune organs including spleen, tonsil or lymph nodes (52). Sterigmatocystin has also been described to be toxic for dendritic cells causing a reduction in its number in vivo (66, 67).
More interestingly, other mycotoxins can affect different immune responses at a concentration that do not cause cell toxicity. For example, citrinin and aflatoxin B1 inhibit NO production in macrophages without cell death (53). As indicated, a common feature of most of these compounds is its ability to inhibit inflammatory cytokine production by macrophages by blocking different mechanisms like TLR expression, RIG or NFkB activation, all of which are involved in the synthesis of cytokines following a pro-inflammatory stimulus (Table 1). Thus, some of them like gliotoxin or emodin have been proposed as anti-inflammatory agents to treat different pathologies like septic shock or colitis in mouse models (64, 65, 74). However, its application for humans is still pending and might be very difficult due to likely secondary toxic effects, unless they are formulated in compositions that allow local selective delivery in affected tissues.
In addition to macrophages, patulin has been shown to directly affect T cell responses, affecting the polarization between Th1 and Th2 by a mechanism dependent on intracellular glutathione (59). Fumonisin prevents dendritic cell maturation and antigen presentation, blocking antigen-specific T cell responses (52). Thus, fumonisin exposure might cause specific T cell immunosuppression and enhance susceptibility to intracellular pathogen infections like viruses, although this hypothesis has not been tested yet.
Finally, some of them might regulate very specific processes like malformin, that has been shown to inhibit IL-1β activity by preventing IL-1β binding to its receptor (75). Indeed, Malformin is commercialized as a specific IL-1β inhibitor. Since inflammasome activation and IL-1β production are critical for initiation of innate and adaptive immune responses, this compound could regulate a broad range of immune responses, including intracellular and extracellular pathogens. Concerning, inflammasome activity and IL1 production Emodin has been shown to inhibit inflammasome activation mediated by ATP and to prevent LPS-mediated septic shock in animal models (64).
Despite the immunosuppressive properties described for several mycotoxins, as shown in Table 1, GT is the one that affects a wider variety of immune responses. This is likely because it has been the most extensively studied and characterized, since it is the most abundant mycotoxin produced by A. fumigatus, the main Aspergillus spp. causing IA.
One the main structural features of GT that regulates its biological activity, including toxicity (cell death) and immunosuppression, is the presence of a disulphide bond, conserved in most members of the epipolythiodioxopiperazine (ETP) family (76). GT can bind and inactivate proteins through cysteine residues and generate ROS thanks to the disulfide bridge present in its structure. It is believed that the generation of ROS and activation of the mitochondrial pathway by Bak is responsible for the toxicity of the GT (77) and is produced by redox reactions between the oxidized (GT) and reduced form (SH2-GT) (78). Morever, the entry of GT in tumor (71) and immune cells (79) has also been shown to be dependent on the presence of the intact disulphide bond.
Most of the studies presented below concerning GT activity on specific cellular functions have been shown in vitro, although it is well known that GT also exerts immunosuppressive effects in vivo in mouse (80) and rat models (81). Indeed, it was suggested as a potential immunosuppressive drug during organ and bone marrow transplantation (82, 83). The mechanism involved seems to be related, at least in the mouse model, with the ability of GT to kill immune cells in spleen, thymus and lymph nodes. However, depicting the effects of GT in vivo against specific cell responses is challenging, and in most cases, it seems that they will be a consequence of its pleitropism to affect most of the immune responses involved in Aspergillus immunity (Figure 1).
Figure 1. Overview of the lung immune response against Aspergillus, indicating the main targets for GT-induced immunosuppression. Resident alveolar macrophages (AM) and epithelial cells (EpC) interact with germinating Aspergillus spores in the lung by different PRRs (mainly TLRs, C-type lectin receptors/CLRs and NLRs), activating the NFkB transcription factor mainly responsible of the synthesis of inflammatory cytokines and chemokines. In addition, AM are able to directly kill phagocytosed spores in phagolysosomes. In response to inflammation, several cells are attracted to the infected site and activated like neutrophils (NF), monocytes (Mo) and NK cells. NF are the first cells extravasating from circulation to the infection site, where they phagocytose and kill Aspergillus conidia and enhance the inflammatory response. In addition, they are able to kill hyphae generated from conidia that have avoided AM, by releasing ROS produced by NADPH oxidase (Nox) as well as to trap them in structures released when neutrophils die known as NETs. Other cells like circulating Mo and NK cells also contribute to Aspergillus clearance, directly or by releasing cytokines that enhance anti-fungal activity of AM and NF. Meanwhile the innate immune response tries to eliminate Aspergillus conidia and hyphae, interstitial immature Dendritic Cells (iDC) phagocytose conidia and hyphae and migrate to lymph nodes, where fully mature DCs present these antigens to CD4+ Th and CD8+ T cells. Here depending on the nature of the Ag presented and the cytokines produced by DC, Th cells differentiate into the different subsets, CD8+ T cells are activated generating cytotoxic T cells (Tc/CTL) and B cells transformed in antibody producing plasma cells. All these cells migrate to the site of infection and contribute to the elimination of the fungus (Th1 and Th17 cells) and to avoid an exacerbated inflammatory response (Treg cells), by expressing cytokines and ligands with different activities. As described in the text GT can interfere with host immune response at different levels. The most pronounced effect seems to be related with its ability to block the inflammatory immune response of macrophages by direct killing or by inhibiting NFkB as well as phagocytosis. In addition, GT is able to kill epithelial cells, and, thus, the fungus could potentially use GT to completely inhibit the generation of the immune response. However, it should be noted that GT is only produced at the hyphae level, and, thus, AM and EpC will be able to activate the immune response, before hyphae are produced. At this stage NFs are critical to eliminate hyphae and, GT has been described as a potent inhibitor of NOX as well as phagocytosis. Finally, GT would inhibit the adaptive immune response at different levels, contributing to host colonization. From this scheme, it seems clear that the contribution of GT to Aspergillus infection will depend on the balance between host immune activity and hyphae development.
In late '80s the group of Arno Mullbacher in Canberra observed in a culture of macrophages accidentally contaminated with a mold that cells spontaneously detached from plates and apparently, remained alive. Macrophages are known to be very difficult to detach from plastic surfaces without killing them, and, thus, the group decided to characterize the compound responsible for this activity (84). They identified it as gliotoxin. Subsequently they found out that it presented a variety of immunosuppressive activity in vitro in macrophages by preventing H2O2 production and bactericidal activity (85). And in antigen presenting cells (APCs) including anti-phagocytic and immunomodulating activity, preventing phagocytosis and activation of T cell responses, including cytotoxic CD8+ T cells (84). Notably, GT did not prevent T cell mediated cytotoxicity once cells were activated, confirming that GT modulated APC activation, preventing APC-mediated T cell activation.
Later on it was shown that apart from its immunomodulatory activity, GT was able to induce apoptosis in macrophages (86) by a mechanism involving ROS production, caspase activation and the intrinsic mitochondrial pathway in mouse macrophages and human monocytes (87). GT induces apoptosis in cultured macrophages via production of ROS and cytochrome c release without mitochondrial depolarization (88). Notably, this effect was not observed in neutrophils, although it does affect its phagocytic capacity (89). The ability of GT to kill macrophages has been related to the inhibition of macrophage function including phagocytosis and pro-inflammatory cytokine production in response to Listeria monocytogens infection (90) or LPS stimulation (62). Concerning the physiological relevance of these in vitro findings, it was shown that the ability of GT to inhibit macrophage function in vitro correlated with an increase replication of Listeria in vivo (90).
It should be noted here that the biological effects of GT on macrophage function, as well as against other immune cells, could be dependent on the concentration. At high concentrations most effects are related to the ability of GT to kill immune cells, meanwhile at lower concentrations specific immunosuppressive effects non-related to cell death could be observed (85).
Concerning the effects non-related to cell death, GT is a well-known inhibitor of NFkB activation in different cells including macrophages, which blocks the production of pro-inflammatory cytokines in response to different stimuli (81). However, it should be indicated that it is not a trivial question to find out a concentration that inhibits NFkB activation and pro-inflammatory cytokine production in macrophages, independently of GT-mediated killing, at least in mouse macrophages (Unpublished data), which might affect the proper interpretation of these findings. However, the relevance of these findings in humans is not clear, since a recent study has shown that SNP in different molecules of NFkB pathway do not increase the risk of IA in SCT patients (91). Thus, in order to clarify the role of GT-mediated NFkB inhibition during IA, studies comparing NFkB activity during infection with GT producing and non-producing A. fumigatus strains should be carried out.
If confirmed in relevant in vivo models, this finding could be a key in order to confirm GT as a prominent immunosuppressive virulence factor: blocking NFkB would affect host immunity early during infection, since this transcription factor is critical for the generation of the inflammatory response after activation of most PRRs involved in Aspergillus immunity including TLR and CLRs.
Another mechanism by which it has been recently described that GT affects macrophage phagocytosis is the interference with IP3 metabolism, which affects integrin activation as well as actin cytoskeleton remodeling, both of which are required for efficient phagocytosis (92).
Another key feature of GT, regarding its immunosuppressive activity, is the ability to affect several neutrophil functions in the absence of cell death. Here it should be noted that it was described that GT was not cytotoxic for human neutrophils at concentrations where monocyte/macrophages were readily killed (89). Thus, it seems that in this cell type the effects observed for GT can be clearly analyzed in the absence of cell death contribution and, as discussed below neutrophil inactivation might be the most relevant immunosuppressive function of GT during IA.
The first report on the immunosuppressive effect of GT on human neutrophil function was published when it was found that H2O2 production was reduced after GT exposure (85). Subsequently, a more detailed analysis of GT on neutrophil function revealed that it affected ROS production, but, in addition, inhibited phagocytosis. Notably, other functions like degranulation or myeloperoxidase activity were not affected (89). Inhibition of phagocytosis was confirmed by another independent study (93). The molecular mechanism behind the anti-oxidant activity of GT was solved in 2004, showing that GT disrupted the formation of a functional NADPH oxidase complex (94), a key finding concerning the ability of GT to interfere neutrophil function, since NADPH oxidase is critical for host protection against Aspergillus.
Intriguingly, it was shown that the effect of GT on neutrophils could be completely different in the presence of corticosteroids (89). In this case, GT increased ROS production in neutrophils treated with methyl-prednisolone, commonly used in patients at risk of IA, which could enhance inflammatory and tissue damage in non-neutropenic patients, a process that has been related with a high infiltration of neutrophils in lungs from Aspergillus infected patients. Thus, the contribution of GT during IA could be related not only to its ability to favor immune evasion, but, in addition, to an exacerbation of tissue damage induced by neutrophils in corticosteroid-treated patients.
Concerning other PMN cells, it was reported that inhibition of NFkB by GT increases eosinophil apoptosis mediated by TNF-α (95). However, the role of this inhibition during the interaction between host eosinophils and Aspergillus is unclear. On the one hand elimination of eosinophils could favor Aspergillus infection since these cells contribute to host defense against Aspergillus. In contrast, it has been recently reported that death eosinophils release NETs after interacting with A. fumigatus (96), which might contribute to Aspergillus clearance, although this hypothesis was not tested and remains to be solved.
NET formation is used by death neutrophils to trap microorganisms, facilitating its clearance and favoring the presentation of associated antigens by dendritic cells and the generation of adaptive immune responses. Recently, it was shown in a mouse model of pulmonary aspergillosis, that neutrophil NADPH oxidase activity is critical for NETosis and apoptosis during aspergillosis (97). Here it is tempting to speculate that GT could interfere with NETosis and Aspergillus clearance by inhibiting neutrophil NADPH oxidase, and thus, affect the transition from innate to adaptive immune system by reducing the amount of Ags available for DC uptake and processing. However, before all these hypotheses are experimentally addressed, the role of NETosis in Aspergillus killing should be clarified since a recent study indicates that NETosis is not a mechanism employed by human neutrophils to kill Aspergillus hyphae (98).
As indicated above, most immunosuppressive effects of GT have been related to innate immune responses, specially macrophages and neutrophils, in concordance with the key role of these cells during Aspergillus infection. However, adaptive immune responses, like T cells, have also found to be important for Aspergillus host defense by enhancing the activity of PMNs and macrophages (CD4 Th1 responses). The relevance of T cells in Aspergillus immunity has been shown in mice (99, 100) and human (101–103). Indeed, some patients undergoing specific therapies affecting T cell function also show increased susceptibility to Aspergillus infection, as in the case of solid organ transplantation (104).
Ag presentation and DC function have been shown to be modulated by GT by several independent groups, affecting subsequent T cell responses. Again, as in the case of macrophages, most effects seem to be related to the ability of GT to induce cell death on DCs. GT was found to kill monocyte-derived dendritic cells blocking Ag presentation and T cell activation, suppressing CMV specific T cell responses (87). In agreement with these findings it was also found that GT killed bone marrow derived DC inhibiting IL12 production and the generation of Listeria-specific CD8+ T cells (78). It was also found in vivo that GT eliminated Langerhans cells (LC), a type of skin associated DCs (105).
Apart from the ability to block T cell generation by affecting DC function, GT is able to directly kill and/or inhibit different T cell functions. Indeed, GT was shown to block NFkB activation in B and T cells by preventing IkBα degradation (106) and later on to kill CD8+ T cells, preventing cytotoxic T cell-mediated cytotoxicity (90). In contrast, at non-toxic doses, GT did not prevent CD8+ T cell function (107). Regarding cytotoxic T cell function, it was reported that GT inhibited CTL-mediated cell death by blocking granule exocytosis- and FasL-mediated cell death (108). The mechanism proposed for this action was the interference with CTL:target cell conjugation. Although authors argued that this defect was not due to GT toxicity on CTLs, from the results presented in that work it is not clear whether GT induced cell death on CTL or not. Notably this work contrast with previous findings indicating that GT did not prevent T cell mediated cytotoxicity once cells were activated (84).
In addition, GT has been shown to affect IFN-γ production by CD4+ T cells (60) which might reduce the ability of CD4+ T cells to enhance macrophage and neutrophil activity against Aspergillus.
Other cells from the innate immune system in which GT might have immunosuppressive activity are Natural Killer cells and Mast cells, both of which have been suggested to be involved in the control of Aspergillus infection (109).
However, meanwhile the evidences for a role of mast cells in Aspergillus immunity are mostly based on in vitro findings, NK cells have been shown to contribute in vitro (110) as well as in vivo (111, 112). However, up to date GT has not been shown to affect NK cell activity.
Concerning Mast cells, GT was shown to block both FcE receptor-dependent and independent activation including degranulation and lipid and cytokine production (113). The mechanism involved was related to the ability of GT to produce intracellular ROS in the absence of cell death.
Although some secondary metabolites, especially GT, can contribute to infection and fungal colonization, as previously indicated (Table 1) most studies have been performed employing human and mouse in vitro cell models, and few in vivo evidences indicate a role for these metabolites in immune evasion and host colonization. An exception is GT, which was shown to act as a virulence factor in vivo in mouse models by employing A. fumigatus mutant strains genetically modified to delete specific genes involved in GT synthesis, like GliP or GliZ (5, 114–117).
However, a question that remains to be solved in humans, albeit it has been addressed in mouse models, is the fact that the role of GT as a virulence factor might be related to the immune status of the host; specifically, the absence of host immune cells that are targeted by GT in immunocompromised patients. In the studies mentioned above the results indicated that in mice treated with cyclophosphamide and corticosteroids, a combination that induces neutropenia, GT was unimportant for fungal virulence (114, 116). In contrast, in mice treated with corticosteroids, which just inhibit neutrophil activity without inducing neutropenia, GT synthesis significantly contributed to virulence (5, 117). Although this explanation has been accepted to reconcile the apparent contradictory results obtained in different studies, it is not completely clear whether this is the only difference to explain the contribution of GT to A. fumigatus virulence. Indeed, in mice treated with vinblastine, a chemotherapy drug that induces neutropenia, the mutant A. fumigatus GliP strain that did not produce GT, was less virulent than a wild type strain. However it should be indicated that neutrophil levels were not determined in these mice, albeit treatment was enough to promote infection (Pardo and Galvez, unpublished data).
Thus, it will be required further studies to solve whether GT only contributes to virulence in corticosteroid treated non-neutropenic host or whether it can also worsen IA evolution by affecting other immune cells involved in host defense such as macrophages, NK or T cells. Here it will be very interesting to test whether GT enhances virulence by promoting immune evasion and/or by enhancing neutrophil-mediated inflammatory tissue damage in corticosteroid-treated host as suggested (89).
In addition, GT could promote fungal invasion by affecting epithelial and/or endothelial cell barriers. Indeed, GT has been shown to kill lung epithelial cells in vitro (118). However, this hypothesis will require further experimental evaluation in mouse in vivo models.
Concerning humans, it will be very difficult to confirm whether GT actually contributes to virulence. Several groups have reported that most A. fumigatus strains isolated from humans are able to synthetize GT as well as the inactive derivative bmGT (79, 119) suggesting that at least ex vivo all fungal isolates synthetize GT, irrespectively of the host immune status from whom they were isolated (neutropenic or not). Confirming these in vitro findings, bmGT, which is synthetized from GT, has been identified in neutropenic (79, 120) and non-neutropenic (121) patients in vivo, suggesting that the fungus produces GT in vivo, even in situations where a priori should not be required (i.e., neutropenia). Here it should be noted that GT cannot be detected in vivo due to its high reactivity, and thus bmGT might be considered as a marker of GT synthesis. In order to confirm whether GT might help Aspergillus to colonize and invade human host, it will be required to analyze whether bmGT presence correlates with prognosis and survival in neutropenic and non-neutropenic patients.
Alternatively, even in situations where GT would not enhance Aspergillus virulence, it could promote, enhance and/or re-activate other infections by blocking macrophage, dendritic cell NK cell and/or T cell function like CMV, EBV or tuberculosis. Studies correlating GT (or bmGT) presence in vivo and risk of viral and/or bacterial co-infections will be required to solve this question.
LS, SR, PML, LC, MPD, MV-G, and AR contributed to drafting the article. MA and EMG contributed to revising it critically and wrote the final version.
This work was supported by Fondo Social Europeo (FSE; Gobierno de Aragón), ASPANOA and by grants SAF2017-83120-C2-1-R, SAF2014- 54763-C2-1-R, SAF2014-54763-C2-2-R from Spanish Ministry of Economy and Competitivenes. MV-G was granted by a Río Hortega contract of National Institute of Health Carlos III (CM16/00236) and MA by a Juan de la Cierva contract of Spanish Ministry of Economy and Competitiveness (FJCI-2017-31629).
The authors declare that the research was conducted in the absence of any commercial or financial relationships that could be construed as a potential conflict of interest.
The handling Editor declared a shared affiliation, though no other collaboration, with several of the authors LS, PL, and AR.
1. Thornton CR. Detection of invasive Aspergillosis. Adv Appl Microbiol. (2009) 70:187–216. doi: 10.1016/S0065-2164(10)70006-X
3. Latge JP. Aspergillus fumigatus and aspergillosis. Clin Microbiol Rev. (1999) 12:310–50. doi: 10.1128/CMR.12.2.310
4. Lamoth F. Aspergillus fumigatus-related species in clinical practice. Front Microbiol. (2016) 7:683. doi: 10.3389/fmicb.2016.00683
5. Sugui JA, Pardo J, Chang YC, Zarember KA, Nardone G, Galvez EM, et al. Gliotoxin is a virulence factor of Aspergillus fumigatus: gliP deletion attenuates virulence in mice immunosuppressed with hydrocortisone. Eukaryot Cell (2007) 6:1562–9. doi: 10.1128/EC.00141-07
6. Hasenberg M, Stegemann-Koniszewski S, Gunzer M. Cellular immune reactions in the lung. Immunol Rev. (2013) 251:189–214. doi: 10.1111/imr.12020
7. Iwasaki A, Foxman EF, Molony R.D. Early local immune defenses in the respiratory tract. Nat Rev Immunol. (2017) 17:7–20. doi: 10.1038/nri.2016.117
8. Hasenberg M, Behnsen J, Krappmann S, Brakhage A, Gunzer M. Phagocyte responses towards Aspergillus fumigatus. Int J Med Microbiol. (2011) 301:436–444. doi: 10.1016/j.ijmm.2011.04.012
9. Morton CO, Bouzani M, Loeffler J, Rogers TR. Direct interaction studies between Aspergillus fumigatus and human immune cells; what have we learned about pathogenicity and host immunity? Front Microbiol. (2012) 3:413. doi: 10.3389/fmicb.2012.00413
10. Karki R, Man SM, Malireddi RKS, Gurung P, Vogel P, Lamkanfi M, et al. Concerted activation of the AIM2 and NLRP3 inflammasomes orchestrates host protection against Aspergillus infection. Cell Host Microbe (2015) 17:357–68. doi: 10.1016/j.chom.2015.01.006
11. Brummer E, Kamberi M, Stevens DA. Regulation by granulocyte-macrophage colony-stimulating factor and/or steroids given in vivo of proinflammatory cytokine and chemokine production by bronchoalveolar macrophages in response to Aspergillus conidia. J Infect Dis. (2003) 187:705–9. doi: 10.1086/368383
12. Man SM, Karki R, Briard B, Burton A, Gingras S, Pelletier S, et al. Differential roles of caspase-1 and caspase-11 in infection and inflammation. Sci Rep. (2017) 7:45126. doi: 10.1038/srep45126
13. Ibrahim-Granet O, Philippe B, Boleti H, Boisvieux-Ulrich E, Grenet D, Stern M, et al. Phagocytosis and intracellular fate of Aspergillus fumigatus conidia in alveolar macrophages. Infect Immun. (2003) 71:891–903. doi: 10.1128/IAI.71.2.891-903.2003
14. Philippe B, Ibrahim-Granet O, Prevost MC, Gougerot-Pocidalo MA, Sanchez Perez, M Van der Meeren A, et al. Killing of Aspergillus fumigatus by alveolar macrophages is mediated by reactive oxidant intermediates. Infect Immun. (2003) 71:3034–42. doi: 10.1128/IAI.71.6.3034-3042.2003
15. Cunha C, Aversa F, Romani L, Carvalho A. Human genetic susceptibility to invasive aspergillosis. PLoS Pathog. (2013) 9:e1003434. doi: 10.1371/journal.ppat.1003434
16. Fisher CE, Hohl TM, Fan W, Storer BE, Levine DM, Zhao LP, et al. Validation of single nucleotide polymorphisms in invasive aspergillosis following hematopoietic cell transplantation. Blood (2017) 129:2693–701. doi: 10.1182/blood-2016-10-743294
17. Gresnigt MS, Cunha C, Jaeger M, Goncalves SM, Malireddi RKS, Ammerdorffer A, et al. Genetic deficiency of NOD2 confers resistance to invasive aspergillosis. Nat Commun. (2018) 9:2636. doi: 10.1038/s41467-018-04912-3
18. King J, Henriet SSV, Warris A. Aspergillosis in chronic granulomatous disease. J Fungi (2016) 2:15. doi: 10.3390/jof2020015
19. Zelante T, De Luca A, Bonifazi P, Montagnoli C, Bozza S, Moretti S, et al. IL-23 and the Th17 pathway promote inflammation and impair antifungal immune resistance. Eur J Immunol. (2007) 37:2695–706. doi: 10.1002/eji.200737409
20. Werner JL, Metz AE, Horn D, Schoeb TR, Hewitt MM, Schwiebert LM, et al. Requisite role for the dectin-1 beta-glucan receptor in pulmonary defense against Aspergillus fumigatus. J Immunol. (2009) 182:4938–46. doi: 10.4049/jimmunol.0804250
21. Jolink H, de Boer R, Hombrink P, Jonkers RE, van Dissel JT, Falkenburg JH, et al. Pulmonary immune responses against Aspergillus fumigatus are characterized by high frequencies of IL-17 producing T-cells. J Infect. (2017) 74:81–8. doi: 10.1016/j.jinf.2016.10.010
22. Chai LY, van de Veerdonk F, Marijnissen RJ, Cheng SC, Khoo AL, Hectors M, et al. Anti-Aspergillus human host defence relies on type 1 T helper (Th1), rather than type 17 T helper (Th17), cellular immunity. Immunology (2010) 130:46–54. doi: 10.1111/j.1365-2567.2009.03211.x
23. Potenza L, Vallerini D, Barozzi P, Riva G, Forghieri F, Beauvais A, et al. Characterization of specific immune responses to different Aspergillus antigens during the course of invasive aspergillosis in hematologic patients. PLoS ONE (2013) 8:e74326. doi: 10.1371/journal.pone.0074326
24. Ermert D, Zychlinsky A, Urban C. Fungal and bacterial killing by neutrophils. Methods Mol Biol. (2009) 470:293–312. doi: 10.1007/978-1-59745-204-5_21
25. Cunha C, Aversa F, Lacerda JF, Busca A, Kurzai O, Grube M, et al. Genetic PTX3 deficiency and aspergillosis in stem-cell transplantation. N Engl J Med. (2014) 370:421–32. doi: 10.1056/NEJMoa1211161
26. Bianchi M, Hakkim A, Brinkmann V, Siler U, Seger RA, Zychlinsky A, et al. Restoration of NET formation by gene therapy in CGD controls aspergillosis. Blood (2009) 114:2619–22. doi: 10.1182/blood-2009-05-221606
27. Ramirez-Ortiz ZG, Means TK. The role of dendritic cells in the innate recognition of pathogenic fungi (A. fumigatus, C. neoformans and C. albicans). Virulence (2012) 3:635–46. doi: 10.4161/viru.22295
28. Curtsinger JM, Lins DC, Johnson CM, Mescher MF. Signal 3 tolerant CD8 T cells degranulate in response to antigen but lack granzyme B to mediate cytolysis. J Immunol. (2005) 175:4392–9. doi: 10.4049/jimmunol.175.7.4392
29. Mezger M, Kneitz S, Wozniok I, Kurzai O, Einsele H, Loeffler J. Proinflammatory response of immature human dendritic cells is mediated by dectin-1 after exposure to Aspergillus fumigatus germ tubes. J Infect Dis. (2008) 197:924–31. doi: 10.1086/528694
30. Ramirez-Ortiz ZG, Lee CK, Wang JP, Boon L, Specht CA, Levitz SM. A nonredundant role for plasmacytoid dendritic cells in host defense against the human fungal pathogen Aspergillus fumigatus. Cell Host Microbe (2011) 9:415–24. doi: 10.1016/j.chom.2011.04.007
31. Cenci E, Perito S, Enssle KH, Mosci P, Latge JP, Romani L, et al. Th1 and Th2 cytokines in mice with invasive aspergillosis. Infect Immun. (1997) 65:564–70.
32. Stappers MHT, Clark AE, Aimanianda V, Bidula S, Reid DM, Asamaphan P, et al. Recognition of DHN-melanin by a C-type lectin receptor is required for immunity to aspergillus. Nature (2018) 555:382–6. doi: 10.1038/nature25974
33. Loeffler J, Haddad Z, Bonin M, Romeike N, Mezger M, Schumacher U, et al. Interaction analyses of human monocytes co-cultured with different forms of Aspergillus fumigatus. J Med Microbiol. (2009) 58(Pt 1):49–58. doi: 10.1099/jmm.0.003293-0
34. Schmidt S, Tramsen L, Hanisch M, Latgé JP, Huenecke S, Koehl U, et al. Human natural killer cells exhibit direct activity against Aspergillus fumigatus hyphae, but not against resting conidia. J Infect Dis. (2011) 203:430–5. doi: 10.1093/infdis/jiq062
35. Bouzani M, Ok M, McCormick A, Ebel F, Kurzai O, Morton CO, et al. Human NK cells display important antifungal activity against Aspergillus fumigatus, which is directly mediated by IFN-gamma release. J Immunol. (2011) 187:1369–76. doi: 10.4049/jimmunol.1003593
36. Morrison BE, Park SJ, Mooney JM, Mehrad B. Chemokine-mediated recruitment of NK cells is a critical host defense mechanism in invasive aspergillosis. J Clin Invest. (2003) 112:1862–70. doi: 10.1172/JCI18125
37. Park SJ, Hughes MA, Burdick M, Strieter RM, Mehrad B. Early NK cell-derived IFN-{gamma} is essential to host defense in neutropenic invasive aspergillosis. J Immunol. (2009) 182:4306–12. doi: 10.4049/jimmunol.0803462
38. Cunha C, Kurzai O, Loffler J, Aversa F, Romani L, Carvalho A. Neutrophil responses to aspergillosis: new roles for old players. Mycopathologia (2014) 178:387–93. doi: 10.1007/s11046-014-9796-7
39. Urb M, Pouliot P, Gravelat FN, Olivier M, Sheppard DC. Aspergillus fumigatus induces immunoglobulin E-independent mast cell degranulation. J Infect Dis. (2009) 200:464–72. doi: 10.1086/600070
40. Piliponsky AM, Romani L. The contribution of mast cells to bacterial and fungal infection immunity. Immunol Rev. (2018) 282:188–97. doi: 10.1111/imr.12623
41. Lilly LM, Scopel M, Nelson MP, Burg AR, Dunaway CW, Steele C. Eosinophil deficiency compromtises lung defense against Aspergillus fumigatus. Infect Immun. (2014) 82:1315–25. doi: 10.1128/IAI.01172-13
42. Sales-Campos H, Tonani L, Cardoso CR, Kress MR. The immune interplay between the host and the pathogen in Aspergillus fumigatus lung infection. Biomed Res Int. (2013) 2013:693023. doi: 10.1155/2013/693023
43. Chaudhary N, Staab JF, Marr KA. Healthy human T-cell responses to Aspergillus fumigatus antigens. PLoS ONE (2010) 5:e9036. doi: 10.1371/journal.pone.0009036
44. Allard JB, Rinaldi L, Wargo MJ, Allen G, Akira S, Uematsu S, et al. Th2 allergic immune response to inhaled fungal antigens is modulated by TLR-4-independent bacterial products. Eur J Immunol. (2009) 39:776–88. doi: 10.1002/eji.200838932
45. Kreindler JL, Steele C, Nguyen N, Chan YR, Pilewski JM, Alcorn JF, et al. Vitamin D3 attenuates Th2 responses to Aspergillus fumigatus mounted by CD4+ T cells from cystic fibrosis patients with allergic bronchopulmonary aspergillosis. J Clin Invest. (2010) 120:3242–54. doi: 10.1172/JCI42388
46. Overton NL, Denning DW, Bowyer P, Simpson A. Genetic susceptibility to allergic bronchopulmonary aspergillosis in asthma: a genetic association study. Allergy Asthma Clin Immunol. (2016) 12:47. doi: 10.1186/s13223-016-0152-y
47. Montagnoli C, Fallarino F, Gaziano R, Bozza S, Bellocchio S, Zelante T, et al. Immunity and tolerance to aspergillus involve functionally distinct regulatory T cells and tryptophan catabolism. J Immunol. (2006) 176:1712–23. doi: 10.4049/jimmunol.176.3.1712
48. Murdock BJ, Shreiner AB, McDonald RA, Osterholzer JJ, White ES, Toews GB, et al. Coevolution of TH1, TH2, and TH17 responses during repeated pulmonary exposure to Aspergillus fumigatus conidia. Infect Immun. (2011) 79:125–35. doi: 10.1128/IAI.00508-10
49. Templeton SP, Buskirk AD, Law B, Green BJ, Beezhold DH. Role of germination in murine airway CD8+ T-cell responses to Aspergillus conidia. PLoS ONE (2011) 6:e18777. doi: 10.1371/journal.pone.0018777
50. Shafiquzzaman S. Chapter 4 - Recent Advancements on the Role of Biologically Active Secondary Metabolites from Aspergillus. New Future Develop Microb Biotechnol Bioeng. (2018) 2018:69–94. doi: 10.1016/B978-0-444-63501-3.00004-1
51. Tamiya H, Ochiai E, Kikuchi K, Yahiro M, Toyotome T, Watanabe A, et al. Secondary metabolite profiles and antifungal drug susceptibility of Aspergillus fumigatus and closely related species, Aspergillus lentulus, Aspergillus udagawae, and Aspergillus viridinutans. J Infect Chemother. (2015) 21:385–91. doi: 10.1016/j.jiac.2015.01.005
52. Pierron A, Alassane-Kpembi I, Oswald IP. Impact of mycotoxin on immune response and consequences for pig health. Anim Nutri. (2016) 2:63–8. doi: 10.1016/j.aninu.2016.03.001
53. Herzog-Soares JD, Freire RB. Effect of citrinin and in association with aflatoxin B1 on the infectivity and proliferation of Toxoplasma gondii in vitro. Braz J Infect Dis. (2004) 8:101–8. doi: 10.1590/S1413-86702004000100007
54. Ubagai T, Tansho S, Ito T, Ono Y. Influences of aflatoxin B1 on reactive oxygen species generation and chemotaxis of human polymorphonuclear leukocytes. Toxicol In Vitro (2008) 22:1115–20. doi: 10.1016/j.tiv.2008.01.007
55. Moon EY, Pyo S. Aflatoxin B1-induced suppression of nitric oxide production in murine peritoneal macrophages. Int J Immunopharm. (2000) 22:237–43. doi: 10.1016/S0192-0561(99)00081-8
56. Jiang M, Peng X, Fang J, Cui H, Yu Z, Chen Z. Effects of aflatoxin b1 on T-cell subsets and mRNA expression of cytokines in the intestine of broilers. Int J Mol Sci. (2015) 16:6945–59. doi: 10.3390/ijms16046945
57. Tomková I, Sevcíková Z, Levkut M, Revajová V, Conková E, Laciaková A, et al. Effect of a?atoxin B1 on CD3 T cells and alkaline phosphatase in the intestine of mice. Mycopathologia (2002) 154:15–9. doi: 10.1023/A:1015296523901
58. Fallon JP, Reeves EP, Kavanagh K. Inhibition of neutrophil function following exposure to the Aspergillus fumigatus toxin fumagillin. J Med Microbiol. (2010) 59(Pt 6):625–33. doi: 10.1099/jmm.0.018192-0
59. Luft P, Oostingh GJ, Gruijthuijsen Y, Horejs-Hoeck J, Lehmann I, Duschl A. Patulin influences the expression of Th1/Th2 cytokines by activated peripheral blood mononuclear cells and T cells through depletion of intracellular glutathione. Environ Toxicol. (2008) 23:84–95. doi: 10.1002/tox.20309
60. Wichmann G, Herbarth O, Lehmann I. The mycotoxins citrinin, gliotoxin, and patulin affect interferon-gamma rather than interleukin-4 production in human blood cells. Environ Toxicol. (2002) 17:211–8. doi: 10.1002/tox.10050
61. Tsai WT, Lo YC, Wu MS, Li CY, Kuo YP, Lai YH, et al. Mycotoxin patulin suppresses innate immune responses by mitochondrial dysfunction and p62/Sequestosome-1-dependent mitophagy. J Biol Chem. (2016) 291:19299–311. doi: 10.1074/jbc.M115.686683
62. Johannessen LN, Nilsen AM, Løvik M. The mycotoxins citrinin and gliotoxin differentially affect production of the pro-inflammatory cytokines tumour necrosis factor-alpha and interleukin-6, and the anti-inflammatory cytokine interleukin-10. Clin Exp Allergy (2005) 35:782–9. doi: 10.1111/j.1365-2222.2005.02249.x
63. Liu BH, Chi JY, Hsiao YW, Tsai KD, Lee YJ, Lin CC, et al. The fungal metabolite, citrinin, inhibits lipopolysaccharide/interferon-γ-induced nitric oxide production in glomerular mesangial cells. Int Immunopharmacol. (2010) 10:1608–15. doi: 10.1016/j.intimp.2010.09.017
64. Han JW, Shim DW, Shin WY, Heo KH, Kwak SB, Sim E, et al. Anti-inflammatory effect of emodin via attenuation of NLRP3 inflammasome activation. Int J Mol Sci. (2015) 16:8102–9. doi: 10.3390/ijms16048102
65. Zhu S, Wang Y, Wang X, Li J, Hu F. Emodin inhibits ATP-induced IL-1β secretion, ROS production and phagocytosis attenuation in rat peritoneal macrophages via antagonizing P2X7 receptor. Pharm Biol. (2014) 52:51–7. doi: 10.3109/13880209.2013.810648
66. Liu Y, Xing X, Wang J, Xing L, Su Y, Yao Z, et al. Sterigmatocystin alters the number of FoxP3+ regulatory T cells and plasmacytoid dendritic cells in BALB/c mice. Food Chem Toxicol. (2012) 50:1920–6. doi: 10.1016/j.fct.2012.03.005
67. Wang X, Robertson AL, Li J, Chai RJ, Haishan W, Sadiku P, et al. Inhibitors of neutrophil recruitment identified using transgenic zebrafish to screen a natural product library. Dis Model Mech. (2014) 7:163–9. doi: 10.1242/dmm.012047
68. Atkinson JP, Michael JM, Chaplin H Jr, Parker CW. Modulation of macrophage C3b receptor function by cytochalasin-sensitive structures. J Immunol. (1977) 118:1292–9.
69. Nolz JC, Fernandez-Zapico ME, Billadeau DD. TCR/CD28-stimulated actin dynamics are required for NFAT1-mediated transcription of c-rel leading to CD28 response element activation. J Immunol. (2007) 179:1104–12. doi: 10.4049/jimmunol.179.2.1104
70. Standeven LJ, Carlin LM, Borszcz P, Davis DM, Burshtyn DN. The actin cytoskeleton controls the efficiency of killer Ig-like receptor accumulation at inhibitory NK cell immune synapses. J Immunol. (2004) 173:5617–25. doi: 10.4049/jimmunol.173.9.5617
71. Bernardo PH, Brasch N, Chai CL, Waring P. A novel redox mechanism for the glutathione-dependent reversible uptake of a fungal toxin in cells. J Biol Chem. (2003) 278:46549–55. doi: 10.1074/jbc.M304825200
72. Scharf DH, Heinekamp T, Remme N, Hortschansky P, Brakhage AA, Hertweck C. Biosynthesis and function of gliotoxin in Aspergillus fumigatus. Appl Microbiol Biotechnol. (2012) 93:467–72. doi: 10.1007/s00253-011-3689-1
73. Kwon-Chung KJ, Janyce AS. What do we know about the role of gliotoxin in the pathobiology of Aspergillus fumigatus? Med Mycol. (2009) 47(Suppl. 1): S97–103. doi: 10.1080/13693780802056012
74. Jun CD, Kim Y, Choi EY, Kim M, Park B, Youn B, et al. Gliotoxin reduces the severity of trinitrobenzene sulfonic acid-induced colitis in mice: evidence of the connection between heme oxygenase-1 and the nuclear factor-kappaB pathway in vitro and in vivo. Inflamm Bowel Dis. (2006) 12:619–29. doi: 10.1097/01.ibd.0000225340.99108.8a
75. Herbert JM, Savi P, Lalé A, Laplace MC, Baudry N, Pereillo JM, et al. Malformin-a1 inhibits the binding of interleukin-1β (il1β) and suppresses the expression of tissue factor in human endothelial cells and monocytes. Biochem Pharm. (1994) 48:1211–7.
76. Gardiner DM, Waring P, Howlett B. The epipolythiodioxopiperazine (ETP) class of fungal toxins: distribution, mode of action, functions and biosynthesis. Microbiology (2005) 151:1021–32. doi: 10.1099/mic.0.27847-0
77. Pardo J, Urban C, Galvez EM, Ekert PG, Muller U, Kwon-Chung J, et al. The mitochondrial protein Bak is pivotal for gliotoxin-induced apoptosis and a critical host factor of Aspergillus fumigatus virulence in mice. J Cell Biol. (2006) 174:509–19. doi: 10.1083/jcb.200604044
78. Dolan SK, O'Keeffe G, Jones GW, Doyle S. Resistance is not futile: gliotoxin biosynthesis, functionality and utility. Trends Microbiol. (2015) 23:419–28. doi: 10.1016/j.tim.2015.02.005
79. Domingo MP, Colmenarejo C, Martínez-Lostao L, Müllbacher A, Jarne C, Revillo MJ, et al. Bis(methyl)gliotoxin proves to be a more stable and reliable marker for invasive aspergillosis than gliotoxin and suitable for use in diagnosis. Diagn Microbiol Infect Dis. (2012) 73:57–64. doi: 10.1016/j.diagmicrobio.2012.01.012
80. Sutton P, Newcombe NR, Waring P, Müllbacher A. In vivo immunosuppressive activity of gliotoxin, a metabolite produced by human pathogenic fungi. Infect Immun. (1994) 62:1192–8.
81. Fitzpatrick LR, Wang J, Le T. In vitro and in vivo effects of gliotoxin, a fungal metabolite: efficacy against dextran sodium sulfate-induced colitis in rats. Dig Dis Sci. (2000) 45:2327–36. doi: 10.1023/A:1005630723111
82. Mullbacher A, Moreland AF, Waring P, Sjaarda A, Eichner RD. Prevention of graft-versus-host disease by treatment of bone marrow with gliotoxin in fully allogeneic chimeras and their cytotoxic T cell repertoire. Transplantation (1988) 46:120–5. doi: 10.1097/00007890-198807000-00022
83. Sutton P, Moreland A, Hutchinson IV, Mullbacher A. Investigation of the potential use of immunosuppressive agent gliotoxin in organ transplantation. Transplantation (1995) 60:900–2. doi: 10.1097/00007890-199511000-00003
84. Müllbacher A, Waring P, Eichner RD. Identification of an agent in cultures of Aspergillus fumigatus displaying anti-phagocytic and immunomodulating activity in vitro. J Gen Microbiol. (1985) 131:1251–8.
85. Eichner RD, Al Salami M, Wood PR, Müllbacher A. The effect of gliotoxin upon macrophage function. Int J Immunopharmacol. (1986) 8:789–97. doi: 10.1016/0192-0561(86)90016-0
86. Waring P. Gliotoxin induces apoptosis in macrophages unrelated to its antiphagocytic properties. J Biol Chem. (1990) 265:14476–80.
87. Stanzani M, Orciuolo E, Lewis R, Kontoyiannis DP, Martins SL, St John LS, et al. Aspergillus fumigatus suppresses the human cellular immune response via gliotoxin-mediated apoptosis of monocytes. Blood (2005) 105:2258–65. doi: 10.1182/blood-2004-09-3421
88. Suen YK, Fung KP, Lee CY, Kong SK. Gliotoxin induces apoptosis in cultured macrophages via production of reactive oxygen species and cytochrome c release without mitochondrial depolarization. (2001) Free Radical Res. 35:1–10. doi: 10.1080/10715760100300541
89. Orciuolo E, Stanzani M, Canestraro M, Galimberti S, Carulli G, Lewis R, et al. Effects of Aspergillus fumigatus gliotoxin and methylprednisolone on human neutrophils: implications for the pathogenesis of invasive aspergillosis. J Leukoc Biol. (2007) 82:839–48. doi: 10.1189/jlb.0207090
90. Kupfahl C, Geginat G, Hof H. Gliotoxin mediated suppression of innate and adaptive immune functions directed against Listeria monocyto-genes. Med Mycol. (2006) 44:591–9. doi: 10.1080/13693780600815411
91. Lupianez CB, Villaescusa MT, Carvalho A, Springer J, Lackner M, Sanchez-Maldonado JM, et al. Common genetic polymorphisms within NFkappaB-related genes and the risk of developing invasive aspergillosis. Front Microbiol. (2016) 7:1243. doi: 10.3389/fmicb.2016.01243
92. Schlam D, Canton J, Carreño M, Kopinski H, Freeman SA, Grinstein S, et al. Gliotoxin suppresses macrophage immune function by subverting phosphatidylinositol 3,4,5-trisphosphate homeostasis. mBio (2016) 7:e02242. doi: 10.1128/mBio.02242-15
93. Coméra C, André K, Laffitte J, Collet X, Galtier P, Maridonneau-Parini I. Gliotoxin from Aspergillus fumigatus affects phagocytosis and the organization of the actin cytoskeleton by distinct signalling pathways in human neutrophils. Microbes Infect. (2007) 9:47–54. doi: 10.1016/j.micinf.2006.10.009
94. Tsunawaki S, Yoshida LS, Nishida S, Kobayashi T, Shimoyama T. Fungal metabolite gliotoxin inhibits assembly of the human respiratory burst NADPH oxidase. Infect Immun. (2004) 72:3373–82. doi: 10.1128/IAI.72.6.3373-3382.2004
95. Fujihara S, Ward C, Dransfield I, Hay RT, Uings IJ, Hayes B, et al. Inhibition of nuclear factor-kappaB activation un-masks the ability of TNF-alpha to induce human eosinophil apoptosis. Eur J Immunol. (2002) 32:457–66. doi: 10.1002/1521-4141(200202)32:2 < 457::AID-IMMU457>3.0.CO;2-1
96. Muniz VS, Silva JC, Braga YAV, Melo RCN, Ueki S, Takeda M, et al. Eosinophils release extracellular DNA traps in response to Aspergillus fumigatus. J Allergy Clin Immunol. (2018) 141:571–85. doi: 10.1016/j.jaci.2017.07.048
97. Röhm M, Grimm MJ, D'Auria AC, Almyroudis NG, Segal BH, Urban CF. NADPH oxidase promotes neutrophil extracellular trap formation in pulmonary aspergillosis. Infect Immun. (2014) 82:1766–77. doi: 10.1128/IAI.00096-14
98. Gazendam RP, van Hamme JL, Tool AT, Hoogenboezem M, van den Berg JM, Prins JM, et al. Human neutrophils use different mechanisms to kill Aspergillus fumigatus conidia and hyphae: evidence from phagocyte defects. J Immunol. (2016) 196:1272–83. doi: 10.4049/jimmunol.1501811
99. Cenci E, Mencacci A, Bacci A, Bistoni F, Kurup VP, Romani L. T cell vaccination in mice with invasive pulmonary aspergillosis. J Immunol. (2000) 165:381–8. doi: 10.4049/jimmunol.165.1.381
100. Bozza S, Gaziano R, Spreca A, Bacci A, Montagnoli C, di Francesco P, et al. Dendritic cells transport conidia and hyphae of Aspergillus fumigatus from the airways to the draining lymph nodes and initiate disparate Th responses to the fungus. J Immunol. (2002) 168:1362–71. doi: 10.4049/jimmunol.168.3.1362
101. Hebart H, Bollinger C, Fisch P, Sarfati J, Meisner C, Baur M, et al. Analysis of T-cell responses to Aspergillus fumigatus antigens in healthy individuals and patients with hematologic malignancies. Blood (2002) 100:4521–8. doi: 10.1182/blood-2002-01-0265
102. Cui N, Wang H, Long Y, Liu D. CD8? T-cell counts: an early predictor of risk and mortality in critically ill immunocompromised patients with invasive pulmonary aspergillosis. Crit Care (2013) 17:R157. doi: 10.1186/cc12836
103. Carvalho A, De Luca A, Bozza S, Cunha C, D'Angelo C, Moretti S, et al. TLR3 essentially promotes protective class I-restricted memory CD8? T-cell responses to Aspergillus fumigatus in hematopoietic transplanted patients. Blood (2012) 119:967–77. doi: 10.1182/blood-2011-06-362582
104. Geltner C, Lass-Flörl C. Invasive pulmonary aspergillosis in organ transplants–Focus on lung transplants. Respir Investig. (2016) 54:76–84. doi: 10.1016/j.resinv.2015.08.005
105. McMinn PC, Halliday GM, Waring P, Muller HK. Langerhans cell depletion in gliotoxin-treated murine epidermis. Pathology (1991) 23:39–44. doi: 10.3109/00313029109061439
106. Pahl HL, Krauss B, Schulze-Osthoff K, Decker T, Traenckner EB, Vogt M, et al. The immunosuppressive fungal metabolite gliotoxin specifically inhibits transcription factor NF-kappaB. J Exp Med. (1996) 183:1829–40. doi: 10.1084/jem.183.4.1829
107. Müllbacher A, Eichner RD. Immunosuppression in vitro by a metabolite of a human pathogenic fungus. Proc Natl Acad Sci USA. (1984) 81:3835–7. doi: 10.1073/pnas.81.12.3835
108. Yamada A, Kataoka T, Nagai K. The fungal metabolite gliotoxin: immunosuppressive activity on CTL-mediated cytotoxicity. Immunol Lett. (2000) 71:27–32. doi: 10.1016/S0165-2478(99)00155-8
109. Schmidt S, Tramsen L, Lehrnbecher T. Natural killer cells in antifungal immunity. Front Immunol. (2017) 8:1623. doi: 10.3389/fimmu.2017.01623
110. Bouzani M, Ok M, Kurzai O, Einsele H, Loeffler J. Human natural killer cells are able to kill Aspergillus Fumigatus but not via the perforin - granzyme pathway. Blood (2009) 114:1640. Available online at: http://www.bloodjournal.org/content/114/22/1640
111. Stuehler C, Kuenzli E, Jaeger VK, Baettig V, Ferracin F, Rajacic Z, et al. Immune reconstitution after allogeneic hematopoietic stem cell transplantation and association with occurrence and outcome of invasive aspergillosis. J Infect Dis. (2015) 212:959–67. doi: 10.1093/infdis/jiv143
112. Fernández-Ruiz M, López-Medrano F, San Juan R, Allende LM, Paz-Artal E, Aguado JM. Low natural killer cell counts and onset of invasive fungal disease after solid organ transplantation. J Infect Dis. (2016) 213:873–4. doi: 10.1093/infdis/jiv552
113. Niide O, Suzuki Y, Yoshimaru T, Inoue T, Takayama T, Ra C. Fungal metabolite gliotoxin blocks mast cell activation by a calcium- and superoxide-dependent mechanism: implications for immunosuppressive activities. Clin Immunol. (2006) 118:108–16. doi: 10.1016/j.clim.2005.08.012
114. Kupfahl C, Heinekamp T, Geginat G, Ruppert T, Härtl A, Hof H, et al. Deletion of the gliP gene of Aspergillus fumigatus results in loss of gliotoxin production but has no effect on virulence of the fungus in a low-dose mouse infection model. Mol Microbiol. (2006) 62:292–302. doi: 10.1111/j.1365-2958.2006.05373.x
115. Bok JW, Chung D, Balajee SA, Marr KA, Andes D, Nielsen KF, et al. GliZ, a transcriptional regulator of gliotoxin biosynthesis, contributes to Aspergillus fumigatus virulence. Infect Immun. (2006) 74:6761–8. doi: 10.1128/IAI.00780-06
116. Cramer RA Jr, Gamcsik MP, Brooking RM, Najvar LK, Kirkpatrick WR, Patterson TF, et al. Disruption of a nonribosomal peptide synthetase in Aspergillus fumigatus eliminates gliotoxin production. Eukaryotic Cell (2006) 5:972–80. doi: 10.1128/EC.00049-06
117. Spikes S, Xu R, Nguyen CK, Chamilos G, Kontoyiannis DP, Jacobson RH, et al. Gliotoxin production in Aspergillus fumigatus contributes to host-specific differences in virulence. J Infect Dis. (2008) 197:479–86. doi: 10.1086/525044
118. Geissler A, Haun F, Frank DO, Wieland K, Simon MM, Idzko M, et al. Apoptosis induced by the fungal pathogen gliotoxin requires a triple phosphorylation of Bim by JNK. Cell Death Differ. (2013) 20:1317–29. doi: 10.1038/cdd.2013.78
119. Vidal-García M, Redrado S, Domingo MP, Marquina P, Colmenarejo C, Meis JF, et al. Production of the invasive aspergillosis Biomarker Bis(methylthio)gliotoxin within the genus aspergillus: in vitro and in vivo metabolite quantification and genomic analysis. Front Microbiol. (2018) 9:1246. doi: 10.3389/fmicb.2018.01246
120. Vidal-García M, Sánchez-Chueca P, Domingo MP, Ballester C, Roc L, Ferrer I, et al. Disseminated aspergillosis in an immunocompetent patient with detectable bis(methylthio)gliotoxin and negative galactomannan. Rev Iberoam Micol. (2017) 34:49–52. doi: 10.1016/j.riam.2016.05.007
Keywords: aspergillus, pulmonary aspergillosis, secondary metabolism, Host Lung Immunity, Gliotoxin
Citation: Arias M, Santiago L, Vidal-García M, Redrado S, Lanuza P, Comas L, Domingo MP, Rezusta A and Gálvez EM (2018) Preparations for Invasion: Modulation of Host Lung Immunity During Pulmonary Aspergillosis by Gliotoxin and Other Fungal Secondary Metabolites. Front. Immunol. 9:2549. doi: 10.3389/fimmu.2018.02549
Received: 20 July 2018; Accepted: 17 October 2018;
Published: 06 November 2018.
Edited by:
Jesús Gonzalo-Asensio, Universidad de Zaragoza, SpainReviewed by:
Joshua J. Obar, Dartmouth College, United StatesCopyright © 2018 Arias, Santiago, Vidal-García, Redrado, Lanuza, Comas, Domingo, Rezusta and Gálvez. This is an open-access article distributed under the terms of the Creative Commons Attribution License (CC BY). The use, distribution or reproduction in other forums is permitted, provided the original author(s) and the copyright owner(s) are credited and that the original publication in this journal is cited, in accordance with accepted academic practice. No use, distribution or reproduction is permitted which does not comply with these terms.
*Correspondence: Maykel Arias, bWF5a2VsYXJpYXNjYWJyZXJvQGdtYWlsLmNvbQ==
Eva M. Gálvez, ZXZhQGljYi5jc2ljLmVz
Disclaimer: All claims expressed in this article are solely those of the authors and do not necessarily represent those of their affiliated organizations, or those of the publisher, the editors and the reviewers. Any product that may be evaluated in this article or claim that may be made by its manufacturer is not guaranteed or endorsed by the publisher.
Research integrity at Frontiers
Learn more about the work of our research integrity team to safeguard the quality of each article we publish.