- 1Perinatology Research Branch, Division of Obstetrics and Maternal-Fetal Medicine, Division of Intramural Research, Eunice Kennedy Shriver National Institute of Child Health and Human Development, National Institutes of Health, U. S. Department of Health and Human Services, Bethesda, MD and Detroit, MI, United States
- 2Department of Obstetrics and Gynecology, Wayne State University School of Medicine, Detroit, MI, United States
- 3Department of Obstetrics and Gynecology, University of Michigan, Ann Arbor, MI, United States
- 4Department of Epidemiology and Biostatistics, Michigan State University, East Lansing, MI, United States
- 5Center for Molecular Medicine and Genetics, Wayne State University, Detroit, MI, United States
- 6Department of Immunology, Microbiology and Biochemistry, Wayne State University School of Medicine, Detroit, MI, United States
Pregnancy success is orchestrated by the complex balance between the maternal and fetal immune systems. Herein, we summarize the potential role of innate lymphoid cells (ILCs) in the maternal and fetal compartments. We reviewed published literature describing different ILC subsets [ILC1s, ILC2s, ILC3s, and lymphoid tissue inducer (LTi) cells] in the uterus, decidua, fetal tissues [liver, secondary lymphoid organs (SLO), intestine, and lung] and amniotic cavity. ILC1s, ILC2s, and ILC3s are present in the murine uterus prior to and during pregnancy but have only been detected in the non-pregnant endometrium in humans. Specifically, ILC2s reside in the murine uterus from mid-pregnancy to term, ILC1s increase throughout gestation, and ILC3s remain constant. Yet, LTi cells have only been detected in the non-pregnant murine uterus. In the human decidua, ILC1s, ILC3s, and LTi-like cells are more abundant during early gestation, whereas ILC2s increase at the end of pregnancy. Decidual ILC1s were also detected during mid-gestation in mice. Interestingly, functional decidual ILC2s and ILC3s increased in women who underwent spontaneous preterm labor, indicating the involvement of such cells in this pregnancy complication. Fetal ILCs exist in the liver, SLO, intestine, lung, and amniotic cavity. The fetal liver is thought to be the source of ILC progenitors since the differentiation of these cells from hematopoietic stem cells occurs at this site, and mature ILC subsets can be found in this compartment as well. The interaction between LTi cells and specialized stromal cells is important during the formation of SLO. Mature ILCs are found at the mucosal surfaces of the lung and intestine, from where they can extravasate into the amniotic cavity. Amniotic fluid ILCs express high levels of RORγt, CD161, and CD103, hallmarks of ILC3s. Such cells are more abundant in the second trimester than later in gestation. Although amniotic fluid ILC3s produce IL-17A and TNFα, indicating their functionality, their numbers in patients with intra-amniotic infection/inflammation remain unchanged compared to those without this pregnancy complication. Collectively, these findings suggest that maternal (uterine and decidual) ILCs play central roles in both the initiation and maintenance of pregnancy, and fetal ILCs participate in the development of immunity.
Introduction
Successful pregnancy requires the participation of numerous immune cell subsets that must be maintained at perfect equilibrium in the maternal and fetal compartments (1, 2). Both innate and adaptive immune cells have been shown to play important roles in the maintenance and completion of pregnancy (3, 4). The discovery of innate lymphoid cells (ILCs), which bridge the innate and adaptive immune systems, has opened up a new field of investigation with the potential to further uncover the complex immune state of pregnancy.
Innate lymphoid cells (ILCs) are defined by the following characteristics: (1) a lack of antigen-specific receptors, (2) the absence of the expression of known immune cell lineage markers, and (3) lymphoid cellular morphology (5–7). ILCs were divided into three primary groups based on their phenotype and functions (6). Type 1 ILCs (ILC1s) include the prototypical natural killer (NK) cells as well as non-cytotoxic IFNγ-producing ILC1s, identified by expression of the transcription factor T-bet (6, 8, 9). Type 2 ILCs (ILC2s) function through the release of type 2 cytokines such as IL-5 and IL-13 (10–15) and are thought to rely primarily on GATA-binding protein 3 (GATA3) and retinoic acid receptor-related orphan receptor-α (RORα) during their differentiation (6, 16, 17). These ILC2s participate in immune responses such as parasitic infection (12) and allergy (18) but also serve as systemic regulators of homeostasis (19–21). Type 3 ILCs (ILC3s) were divided into two main groups: lymphoid tissue inducer (LTi) cells and non-LTi ILC3s, referred to hereafter as ILC3s (6). LTi cells are critical for the formation of secondary lymphoid organs (SLO) and isolated lymphoid tissues (i.e., Peyer's patches) during fetal development (22–26). Such cells are also found in adults, where they are referred to as LTi-like cells since they do not generate new lymphoid tissue (27). LTi cells and ILC3s rely on expression of RORγt for their development (26) and can express IL-17A and/or IL-22; however, multiple ILC3 subsets with slightly different phenotypes and functional profiles have been described (6). Moreover, a degree of plasticity exists between ILCs, creating an additional layer of complexity within the ILC family (28–31). Recently, it was proposed that the classification of ILCs be expanded to five subsets in order to reflect their distinct developmental pathways: NK cells, ILC1s, ILC2s, ILC3s, and LTi cells (7).
In this review, we aimed to highlight the potential roles of ILCs in the uterus, the decidua, which is the site of direct contact between the maternal and fetal (chorion or trophoblast) tissues, the fetal organs, and the amniotic cavity. Within the field of perinatal immunology, it has been established that uterine (decidual) NK cells play important roles in the maintenance of pregnancy, and their functions are well reviewed elsewhere (32–35). Recent studies have shown that the other ILC subsets exist in the maternal and fetal tissues, suggesting that they also contribute to pregnancy maintenance and outcome. Therefore, in this review, we have focused on ILC1s, ILC2s, ILC3s, and LTi cells. Despite recent advances in the study of ILCs during pregnancy, several gaps still exist in the current knowledge. This review may provide insight into the known roles of ILCs during pregnancy and reveal new potential areas for future studies.
Uterine Innate Lymphoid Cells
Over the last decade, valuable information has been provided about the presence of ILCs in both the non-pregnant and pregnant uterus. A subset of ILC-like cells was first described in the human uterine mucosa (36). Such cells were originally considered precursors to uterine NK cells, yet showed a divergent phenotype and functionality through the expression of ILC3- and LTi-specific genes such as RORC, LTA, and IL22 (36), indicating a different role for these cells. These results were confirmed later by the detection of ILC1s (37), ILC2s (38), and ILC3s (37, 38) in the human non-pregnant endometrium and reinforced by the demonstration that such cells are present in the murine uterus during pregnancy as well (37–41). Such studies have formed a foundation for the understanding of uterine ILCs; yet, future research is needed to further elucidate the role of these cells during pregnancy.
Uterine ILC1s
Uterine ILC1s were first described in non-pregnant mice as a distinct subset of NK-like cells (42). This ILC1-like population was maintained in the murine uterus of Nfil3−/− and Tbet−/− mice, whereas NK cell subsets were affected (42). The transcription factors Nfil3/E4BP4 (43–45) and Tbet (6, 8, 9, 46) are both thought to be important for general NK and ILC development; thus, this study indicates that uterine ILC1s may have alternative developmental pathways. This study was confirmed by the detection of a similar ILC1 subset in the uterine mucosa of non-pregnant mice which was negative for CD127 expression (38), highlighting the variability of uterine ILCs since CD127 (IL-7Rα) is also considered to be important for ILC development (6).
In mice, uterine ILC1s are increased throughout gestation compared to the non-pregnant state (37, 39, 40). The production of IFNγ by stimulated total uterine ILCs is increased during gestation (40), which would suggest that these cells have an enhanced capacity for activation in this reproductive tissue. Indeed, uterine ILC1s were shown to contribute to IFNγ production during pregnancy, although not to the same extent as uterine NK cells (38). Consistent with previous findings (42), the uterine ILC1 population was not affected by the knockout of Nfil3 (38); indeed, ILC1s were increased in these mice (38, 39), indicating that alternative developmental pathways exist for such cells. Since Nfil3 is crucial for expression of Eomes (47), a transcription factor associated with NK cells (48), it was proposed that the uterine ILC1 population observed in Nfil3−/− mice includes developmentally arrested NK cells (38). However, these residual ILC1s may not be sufficient for mediating uterine adaptation during pregnancy since placental and fetal abnormalities are observed in Nfil3−/− mice (39).
In humans, ILC1s are found in the non-pregnant endometrium as a subset of Lin-CD56+CD127-CD117-RORγt-cells, which are further distinguished based on expression of NKp44 and CD103 (37). ILC1-like cells, which are CD103+NKp44–, are the most significant source of IFNγ (37). Expression of CD103, which facilitates the communication between lymphocytes and epithelial cells (49), was previously described on tonsillar ILC1s (50), suggesting an epithelial association of such cells in the uterus. However, additional research is needed to determine the role of uterine ILC1s prior to pregnancy and whether such cells are present during gestation.
Uterine ILC2s
A low frequency of ILC2s has been detected in the human non-pregnant uterine wall (38, 51). In addition, ILC2s and ILC2-like cells have been identified in the non-pregnant murine myometrium (38, 40, 41). The ILC2-like population is the most abundant ILC subset in the pregnant murine myometrium (40). The proportions of murine uterine ILC2s and ILC2-like cells are higher during pregnancy compared to the non-pregnant state, reaching their peak during mid-gestation (38, 40, 51). However, the uterine ILC2-like population (CD45+Lin-Thy1.2+RORγt-NKp46-KLRG1+ cells) identified by Li et al. may have also included other cell types since conventional ILC2 markers such as GATA3 (16) or CRTH2 (52) were not included (40). A recent study utilizing conventional ILC2 markers identified a population of CD127-ILC2s in the non-pregnant human endometrium and in both the non-pregnant and pregnant murine myometrium, confirming the presence of such cells (51). Total uterine ILCs expressing IL-5 and IL-13 were increased during gestation (40), supporting functional roles for uterine ILC2s such as promotion of homeostatic immune cell phenotypes and resolution of inflammatory responses (51).
Uterine ILC2s are almost completely ablated in Nfil3−/− mice as opposed to the other subsets (38, 39), confirming that these cells are developmentally reliant on this transcription factor. It is possible that the placental and fetal changes observed in Nfil3−/− mice are due to the loss of ILC2-dependent regulatory mechanisms in the myometrium (39); however, since conventional NK cells were also greatly impacted in such mice (38, 39), this finding will require further studies to confirm.
It was recently shown that murine uterine ILC2s express the IL-33 receptor, ST2 (IL-1RL1) (41). A previous report highlighted the importance of IL-33/ST2 signaling for homeostatic immune responses such as those mediated by T helper 2 cells, regulatory T cells, M2-polarized macrophages, and ILC2s, among others (53). In line with these findings, uterine ILC2s were increased in proportion after in vitro stimulation with IL-33 (41). ILC2 activity was also increased by in vitro IL-33 stimulation as indicated by enhanced release of IL-5 and IL-13 (41). Moreover, an IL-5 reporter mouse (54) was used to verify that in vivo administration of IL-33 increased uterine ILC2 proportions and expression of IL-5 (41). Interestingly, the original research describing the IL-5 reporter mouse model demonstrated that the majority of IL-5+ cells in different murine tissues had an ILC2 phenotype, including expression of CD127 and ST2 (54), providing further evidence that IL-33-receptive ILC2s are important for the production of IL-5. Pups born to ST2−/− dams had significantly reduced viability (41), suggesting that this pathway may be beneficial for fetal development; however, IL33−/− mice do not experience any fertility or pregnancy complications (55). Additionally, IL-33 is important for type 2 mucosal immune responses (55). Together, these observations support pregnancy-specific functions for IL-33-receptive ILC2s in the murine uterus.
Murine uterine ILC2s can also express the estrogen receptor α (41). The proportion of these cells is increased in response to in vitro stimulation with 17β-estradiol; however, such a response is not seen in ILC2s from the murine lung (41), providing evidence for specific female sex hormone-driven regulation of uterine ILC2s during pregnancy. Yet, whether female sex hormones specifically target ILC2s, or the observed ILC2 proliferation was a secondary response due to signaling within the uterine tissues, has not been shown (41).
Collectively, these findings provide firm evidence of ILC2s in the non-pregnant uterine tissues from humans and mice, and that such cells are enhanced in number and function during murine gestation. Further studies are required to uncover the specific mechanisms and cellular interactions of uterine ILC2s.
Uterine ILC3s
ILC3s were first described in the human non-pregnant endometrium as a distinct subset of NK precursor-like cells expressing ILC-associated markers such as CD127 and CD161 (36). Further analysis of these cells revealed expression of the RORC and IL22 genes, indicative of an ILC3 phenotype (36). Later studies confirmed the presence of ILC3s in the human endometrium (37, 38) and indicated that these cells could be divided into two main subsets: NCR– (human NKp44-; mouse NKp46-) and NCR+ (human NKp44+; mouse NKp46+) ILC3s (7), with the NCR– ILC3s being the dominant population in mice and the NCR+ ILC3s in humans (38). During murine pregnancy, uterine ILC3s are elevated compared to non-pregnant mice (38) with the highest proportions occurring in early- and mid-gestation (40). Uterine ILC3s from both pregnant and non-pregnant mice constitutively produce IL-17A and IL-22, which is further upregulated in response to in vitro stimulation with IL-1β and IL-23 (38). Yet, production of IL-17A and IL-22 by uterine ILC3s from pregnant mice is not significantly elevated in mid-gestation compared to that of non-pregnant mice (38, 40), suggesting that either an increase in ILC3-specific functionality is not required for successful pregnancy, or that such an increase may only occur in late gestation/prior to parturition. Further studies are required to pursue this concept.
In contrast with ILC2s, the uterine ILC3 population is not affected in non-pregnant Nfil3−/− mice (38, 39); however, such cells fail to undergo the pregnancy-specific expansion observed in wildtype mice (39). This lack of ILC3 expansion is associated with fetal growth compromise and defective placentation (39), indicating that uterine ILC3s may be important for the physiological progression of pregnancy, e.g., decidualization (see decidual ILC section for more information).
Uterine LTi-like Cells
Information regarding LTi-like cells in the human and murine uterus is scarce. One potential explanation is that LTi-like cells have been identified as ILC3s due to the shared expression of markers such as RORγt (6). LTi-like cells were reported in the non-pregnant murine uterus in similar proportions to the closely-related ILC3s (38). Moreover, similar to ILC3s, LTi-like cells were not affected in the uterus of non-pregnant Nfil3−/− mice (38), suggesting a distinct developmental pathway for these cells. It will be interesting for future studies to uncover the functions of LTi-like cells in the human uterus.
In conclusion (Figure 1A), ILC1s, ILC2s, and ILC3s are present in the murine uterus prior to and during pregnancy, but have only been detected in the non-pregnant endometrium in humans. Specifically, ILC2s reside in the murine uterus from mid-pregnancy to term, ILC1s increase throughout gestation, and ILC3s remain constant. Yet, LTi cells have only been detected in the non-pregnant murine uterus. Further studies are needed to confirm the presence and functions of uterine ILCs during human pregnancy.
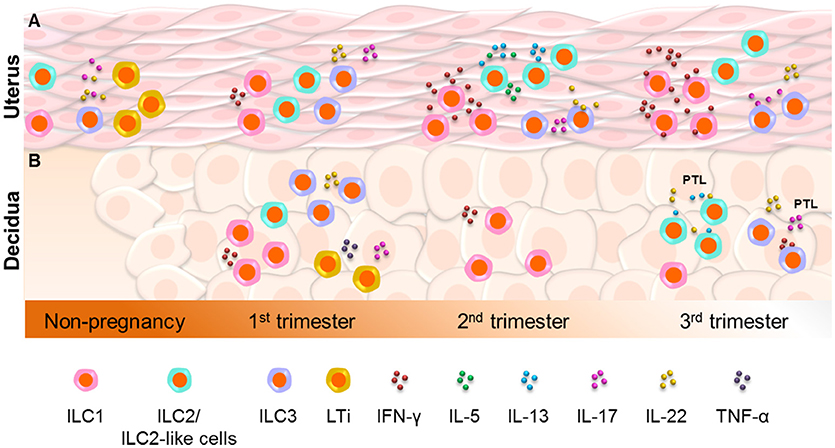
Figure 1. Innate lymphoid cells in the uterus and decidua. (A) ILC1s, ILC2s, and ILC3s are present in the murine uterus prior to and during pregnancy, but have only been detected in the non-pregnant endometrium in humans. ILC2s reside in the murine uterus from mid-pregnancy to term, whereas ILC1s increase throughout gestation and ILC3s remain constant. However, lymphoid tissue inducer (LTi) cells have only been detected in the non-pregnant murine uterus. Further studies are needed to confirm the presence and functions of uterine ILCs during human pregnancy. (B) In the human decidua, ILC1s, ILC3s, and LTi-like cells are more abundant during early gestation, whereas ILC2s are increased at the end of pregnancy. Decidual ILC1s were also detected during mid-gestation in mice. Interestingly, functional decidual ILC2s and ILC3s are increased in women who underwent spontaneous preterm labor (PTL), indicating the involvement of such cells in this pregnancy complication.
Decidual Innate Lymphoid Cells
Upon implantation, endometrial stromal cells undergo a specialized transformation that includes significant morphological and functional changes to the endometrium, a phenomenon termed as “decidualization” (56, 57). This process facilitates invasion of the fetal trophoblast (56) and leads to formation of the area of contact between the endometrium and the placenta (decidua basalis) or fetal membranes (decidua parietalis). The decidua is therefore an interface in which maternal and fetal cells converge and unique immune interactions take place.
ILCs have been identified in the decidua as early as 9–12 weeks of gestation (58). The origin of decidual ILCs is unclear. Several sources have been proposed for the prototypical ILC1s, NK cells, in the reproductive tissues including derivation from hematopoietic precursors (59), maturation from already-present endometrial NK cells (36), or migration from the periphery (60). Since hematopoietic precursor cells can express the ID2 transcription factor [required for ILC differentiation (61)], this is one plausible explanation for the source of decidual ILCs (59). The origin and developmental timeline of decidual ILCs requires further investigation. Importantly, phenotypic (58) and functional (62) evidence suggests that decidual ILC subsets have unique profiles that are not found in other non-reproductive tissues.
Decidual ILC1s
ILCs expressing an ILC1-like phenotype distinct from NK cells have been detected in the human decidua during the first trimester (37, 58). Two ILC1 subsets were detected. The first was identified within the CD56+ population (Lin-CD56+CD94-CD127-CD117-) and expressed the ILC1-associated Tbet as well as Eomes (37, 58). Interestingly, this ILC1 subset also expressed CD103 (36, 37), indicating a possible epithelial association (50). The other subset fell within the CD56- population (Lin-CD56-CD127-CD117-Tbet+Eomes-) and was therefore more distinguishable from decidual NK cells (36). In line with defined ILC1 phenotypes, both described subsets expressed IFNγ (37, 58). In mice, ILC1s were also described in the decidua during mid-gestation, where they produced IFNγ (38).
At the end of pregnancy, ILC1s are the rarest ILC subset in the human decidua and were not altered with the presence of spontaneous labor, suggesting that such cells may have only a minor role in late gestation that may be shared by other decidual ILC subsets due to the unique cytokine profile observed in such cells (62).
Decidual ILC2s
The first-trimester human decidua has been reported to contain a small proportion of ILC2s (38, 51, 58). The expression of the ILC2 marker CRTH2 (52) was only minimally detected on decidual ILCs (38, 58); however, CD161 (52) was expressed by two of the potential ILC subsets described, indicating possible plasticity or shared expression of ILC2 markers by other subsets (58). ILC2s were found in the murine uterus, but not the decidua, in mid-gestation (38, 51) and at term (51). In contrast, ILC2s were the most abundant decidual ILC subset in the third trimester (62) where they may play a role in maintaining the homeostatic environment at the maternal-fetal interface. ILC2s are considered to have a homeostatic phenotype (19, 20), rendering them unnecessary in early pregnancy when the inflammatory mechanisms of implantation and tissue remodeling occur within the endometrium. Interestingly, ILC2s were increased in the decidua basalis of women with spontaneous preterm labor compared to those who delivered preterm without labor (62), suggesting that this ILC subset may participate in the chronic inflammatory process that occurs during pathological pregnancy. Moreover, ILC2s from the third-trimester human decidua seemed to share the expression of cytokines such as IL-13 and IL-22 with ILC3s, suggesting that decidual ILC subsets may have shared functionality toward the end of pregnancy (62).
Decidual ILC3s
Among the described ILC subsets in the human decidua, ILC3s have been the most extensively studied (37, 38, 58, 62, 63). During the first trimester, a subset of ILCs expressing the traditional ILC3-associated transcription factor RORγt (26) is found in the human decidua (37, 38, 58). Decidual ILC3s expressed GM-CSF (63), IL-22 (58), and IL-8 (58). Notably, GM-CSF and IL-8 released by decidual ILC3s were shown to promote neutrophil migration and survival in the first-trimester decidua (63). This finding is consistent with a previous study that showed the participation of neutrophils in spiral artery remodeling during pregnancy (64), and adds a new layer of complexity to the role of decidual ILC3s in the successful establishment of pregnancy (63). Interestingly, the murine decidua did not contain ILC3s during mid-gestation (38).
During the third trimester, human decidual ILC3s express a unique cytokine profile that includes IFNγ, IL-13, IL-17A, and IL-22 (62). Previous findings have suggested that some degree of plasticity exists between ILC subsets (28, 29, 65), which would explain the expression of the ILC1-associated cytokine IFNγ by decidual ILC3s. This may also explain the low proportions of decidual ILC1s in late gestation (62), since their presence may be redundant.
Interestingly, increased proportions of ILC3s in the decidua parietalis are found in women who undergo spontaneous preterm labor (62), suggesting that a local dysregulation of such cells may occur in these patients. Whether decidual ILC3s directly participate in the inflammation associated with spontaneous preterm labor or are increased as a consequence of such a process remains to be determined.
Decidual LTi-like Cells
Human LTi cells have important functions in the formation of fetal SLO (66), a process described in more detail below; however, their role at the maternal-fetal interface is less understood. A population of LTi-like cells has been described in the human first-trimester decidua where they express IL-17A and TNFα (58). These decidual LTi-like cells are closely related to ILC3s as evidenced by the shared expression of RORγt and production of IL-17A (58). Moreover, both decidual LTi-like cells and ILC3s display lymphoid tissue inducer-like functions (22–25, 67–69) through the upregulation of ICAM-1 and VCAM-1 on decidual stromal cells (58), further indicating a degree of redundancy between these two cell types (27, 70). However, the developmental pathways of LTi cells and ILC3s are different and, unlike other ILC subsets, no LTi plasticity has been reported (27). It is possible that the initiation of a lymphoid tissue induction-like process in the decidua is necessary for recruitment of other immune cells and pregnancy maintenance. Therefore, it is important for future studies to investigate the purpose of LTi-like activity at the maternal-fetal interface.
In conclusion (Figure 1B), the human decidua contains ILC1s, ILC3s, and LTi-like cells, which are more abundant during early gestation. In contrast, decidual ILC2s are increased at the end of pregnancy. Decidual ILC1s were also detected during mid-gestation in mice. Functional decidual ILC2s and ILC3s are increased in women who underwent spontaneous preterm labor, indicating the involvement of such cells in this pregnancy complication.
Fetal Innate Lymphoid Cells
Fetal ILCs are reported to exist in the human liver, SLO, intestine, and lung, which are described in detail below. The fetal liver is a center of hematopoiesis (71, 72), and it has been shown that ILC progenitors (ILCP) originate from this compartment (65, 73–76). Indeed, ILCPs can be detected in the cord blood as well, indicating that such cells may migrate to other sites of organogenesis (76). It has been proposed that the differentiation of ILCPs to mature ILC subsets primarily takes place after such cells have migrated to their sites of residence (76). In the fetus, the presence of specialized ILCs (i.e., LTi cells) is important for the successful formation of SLO such as the spleen, mesenteric lymph nodes (mLN), and Peyer's patches (23, 26, 77, 78). At non-lymphoid sites such as the intestine and lung, mature ILC subsets may participate in mucosal immunity after birth by regulating inflammation during colonization with commensal bacteria (79, 80).
In mice, development of the fetal lymphatic system is described as early as gestational day 10.5 (81). The murine fetal lymph nodes follow a staggered developmental timeline beginning with the mLN at gestational day 10.5, closely followed by the sacral and cervical lymph nodes and ending with the complete formation of Peyer's patches in the intestine [for more information about fetal lymphogenesis, please see (82, 83)]. The mesenteric and peripheral lymph nodes are present in the fetus by gestational day 16.5 (68). ILCPs are detected in the murine fetal liver at day 12.5 (84–88), and in both the fetal liver and intestine at day 13.5–14.5 (74, 89). Information regarding the presence of ILCPs in the fetal tissues in early pregnancy is lacking; therefore, further studies are required to determine the complete timeline for the generation of ILCPs and mature ILC subsets during fetal development.
ILCs in the Fetal Liver
A subset of ILCPs was described in the human fetal liver during the second trimester (76). It was shown that these ILCPs were generated from the CD34+ hematopoietic stem cells (76) also found in this compartment (71, 72, 76). These ILCPs primarily express RORγt and, after in vitro expansion, mainly produce IL-17A, indicating an ILC3 phenotype (76). However, subsets of fetal liver ILCPs also produce IFNγ or IL-13, suggesting that such cells have differentiation potential for ILC1s and ILC2s as well (76). The murine fetal liver also contains an ILCP subset with potential for differentiation into ILC1s, ILC2s, or ILC3s (75). Ablation of Zbtb16, which encodes the ILCP-associated transcription factor PLZF [reviewed in (88)], affected fetal ILC1s and ILC2s but not ILC3s or NK cells (75), supporting the existence of alternative progenitors or developmental pathways for these ILC subsets.
The human fetal liver also contains mature ILC populations during the first and second trimester (90). ILC1s, ILC2s, and both NCR+ and NCR– ILC3s are detected (90). Prior to 15 weeks of gestation, only NCR– ILC3s can be distinguished, whereas the remaining subsets appear later (90). A population of fetal liver ILC3s express neuropilin-1 (NRP-1) (90, 91), suggesting an LTi phenotype (92). Together, these findings indicate that the fetal liver is the primary site of ILC progenitors. Mature ILC subsets also exist within the fetal liver, yet their role is currently unknown.
ILCs in the Fetal Lymphoid Tissues
Murine experiments have shown that the interaction between LTi cells and mesenchymal stromal cells is fundamental for the formation of SLO (93). It has been observed that during murine embryogenesis a subset of stromal cells interacts with LTi cells at the site of LN formation (25, 26, 68). LTi cells express ligands such as lymphotoxins α and β (LTA and LTB) that activate specific stromal cells (25, 94–96). Such activated stromal cells will upregulate expression of the adhesion molecules ICAM-1 and VCAM-1 (25, 69, 78, 97) and begin the process of forming SLO (26).
A subset of Lin-CD127+ ILCs was originally described in human fetal mesenteric tissue (97). It was shown that these ILCs were localized at the same locations at which the mLN developed (91, 97), indicating that lymph node-specific ILCs are present in the fetal mesentery even prior to the complete formation of the mLN. Moreover, stromal organizer cells form a niche for LTi cells in the human fetal spleen and LN between 8 and 15 weeks of gestation (78), providing further evidence of an important role for fetal LTi cells in tissue neogenesis. The mLN from first- and second-trimester human fetuses have been shown to contain an ILC subset that expressed RORγt and had increased gene expression of IL17A and IL22 (98), and a similar subset was described in the fetal spleen that also expressed NRP-1 (91, 92). NRP1−/− knockout mice have severely affected yolk sac and embryonic development (99), suggesting that the expression of this receptor is required for organogenesis. Additionally, RORγt+ ILCs are found in specific physiological locations in the human fetal LN and spleen in the second trimester, where they are co-localized with specialized stromal cells (78). This interaction leads to induced expression of ICAM-1 and VCAM-1 on the stromal cells (78, 97), indicating that these ILCs have LTi functions. LTi cells in the human fetal LN express IL17A and IL22 and participate in LN formation (97).
ILCs in the Fetal Intestine
LTi cells cluster at the site where Peyer's patches are formed in the developing murine fetal intestine (22, 89). The development of intestinal lymphoid tissues such as the Peyer's patches is imperative for regulation of mucosal immunity in the intestine (100, 101), and fetal LTi cells have been shown to be crucial to this process (23, 26). Importantly, a subset of transitional ILCPs exists in the fetal intestine that can further differentiate into other ILC subsets, indicating that some of these ILCs are not terminally differentiated and can provide other functions even after SLO formation is complete (89).
Recently, the presence of mature ILC subsets in the fetal intestine was confirmed using mass cytometry (102). All known ILC subsets were detected together with several novel intermediates that included a subset with potential to differentiate into ILC3s or NK cells (102). These findings confirmed previous studies that indicated the presence of ILC2s (52) and ILC3s (52, 98, 103) in the second-trimester human fetal intestine. ILC2s in the fetal intestine produce IL-13 (52), whereas ILC3s and LTi-like cells produce IL-17A and IL-22 (98). ILC3s are increased in the fetal intestine during the second trimester compared to the first (98, 103). Importantly, fetal CD103+ ILC3s can be found in the amniotic fluid during the first and second trimesters (see amniotic cavity section for more information) (103), suggesting that these cells can migrate from the fetal tissues into the amniotic cavity.
ILCs are increased in the intestinal tissues from neonates with gastroschisis compared to those from healthy controls (104). This finding was corroborated using a murine model with gastroschisis-like symptoms showing that ILC2s and ILC3s are increased in the intestines of affected mice compared to littermate controls (104). Neutralization of IL-5 [a primary ILC2 cytokine (13)] during late gestation results in a dramatic decrease in eosinophil and ILC2 infiltration in the fetal intestine (104), implicating ILC2s in the chronic inflammatory process that accompanies this condition.
Collectively, these data confirm the requirement for LTi cells during the formation of fetal SLO, and indicate that mature ILC1s, ILC2s, and ILC3s are found in the intestinal mucosa where they may participate in inflammatory processes; however, their specific role during fetal life is unclear.
ILCs in the Fetal Lung
A single report established the presence of ILC2s (identified by CRTH2 and CD161 expression) in the human fetal lung mucosa during the second trimester (52). In the neonatal and adult lungs, the primary function of ILC2s is to protect against threats such as helminth infection (12, 13, 105). ILC2s are also implicated in asthma and its complications as well as allergy (18, 106, 107). A subset of CD103+ ILC3s is also detected in the human fetal lung, which is increased in the second trimester compared to the first (103). Recently, a population of ILC2s was described in the murine fetal lung just prior to birth (gestational day 19), which rapidly expanded during the first 2 weeks of life (108). It was suggested that these homeostatic cells may help prevent hyper-inflammation resulting from exposure of the newborn lungs to airborne particles (108). Future studies may further reveal the specific role of ILC2s and ILC3s in this fetal compartment.
In conclusion (Figure 2), fetal ILCs exist in the liver, SLO, intestine, lung, and amniotic cavity. The fetal liver is thought to be the source of ILC progenitors since the differentiation of these cells from hematopoietic stem cells occurs at this site, and mature ILC subsets can be found in this compartment as well. The interaction between LTi cells and specialized stromal cells is important during the formation of SLO. Mature ILCs are found at the mucosal surfaces of the lung and intestine, from where they can extravasate into the amniotic cavity. These findings support a role for ILCs as central regulators in fetal development and immunity.
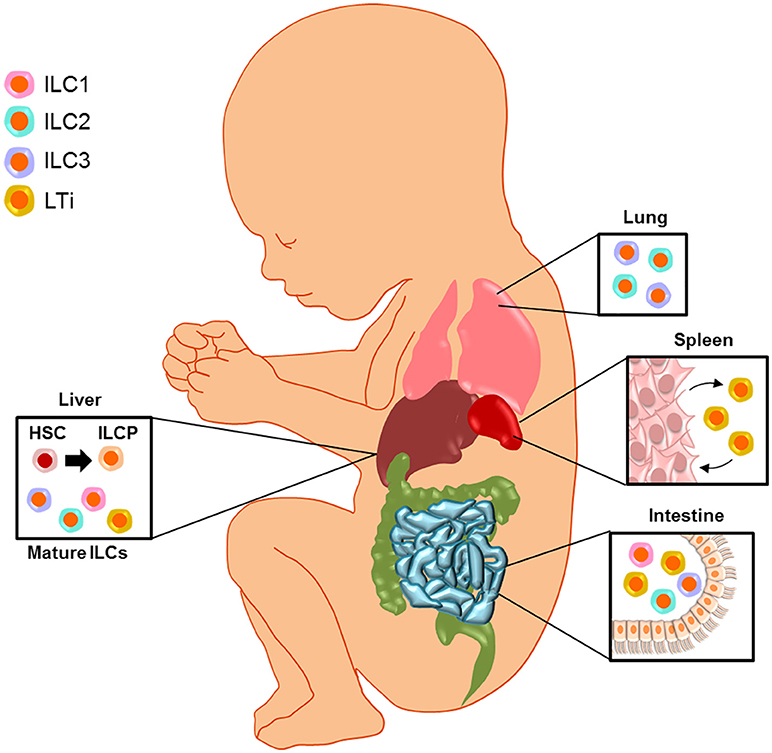
Figure 2. Fetal innate lymphoid cells. Fetal ILCs exist in the liver, secondary lymphoid organs (SLO), intestine, lung, and amniotic cavity. The fetal liver is thought to be the source of ILC progenitors (ILCP) since the differentiation of these cells from hematopoietic stem cells (HSC) occurs at this site, and mature ILC subsets can be found in this compartment as well. The interaction between lymphoid tissue inducer (LTi) cells and specialized stromal cells is important during the formation of SLO. Mature ILCs are found at the mucosal surfaces of the lung and intestine, from where they can extravasate into the amniotic cavity. These findings support a role for ILCs as central regulators in fetal development and immunity.
Innate Lymphoid Cells in the Amniotic Cavity
The amniotic cavity serves as the fetal habitat, which is surrounded by the protective liquid termed amniotic fluid (109). Besides providing mechanical cushioning, the amniotic fluid contains nutrients as well as other factors required for fetal growth and represents an immunological barrier against invading pathogens (109, 110). In clinical medicine, the amniotic fluid is used to assess fetal well-being (111–114), lung maturity (115–117), karyotype (118, 119), and intra-amniotic inflammation associated with bacteria [intra-amniotic infection (120–132)] or danger signals [sterile intra-amniotic inflammation (133–138)]. In the context of intra-amniotic inflammation, the most abundant leukocytes in the amniotic fluid are neutrophils (139, 140), which can be of fetal and/or maternal origin (141, 142). These innate immune cells actively participate in the mechanisms of host defense against microbial invasion of the amniotic cavity by releasing cytokines (140) and anti-microbial molecules (143–145), performing phagocytosis (146), and forming neutrophil extracellular traps or NETs (147, 148). Therefore, it was thought that, in the absence of intra-amniotic inflammation, the cellular component of the amniotic fluid was of limited research value. Recent studies have shown that, indeed, the opposite is true (103, 149). The amniotic fluid contains both innate (monocyte/macrophages, neutrophils, NK cells, and ILCs), and adaptive (T cells and B cells) immune cell populations, each of which fluctuates independently throughout gestation (149).
Amniotic fluid ILCs are abundant during the second trimester (103) and their numbers decay as gestation progresses (149) (Figure 3). In this compartment, ILCs express high levels of RORγt (103, 149), a hallmark of ILC3s (6, 26). Amniotic fluid ILC3s also express CD127, CD117, CD161, and CD56 but not NK cell-markers such as Eomes, T-bet, CD94/NKG2A, and CD16 (103). Such ILCs are functional since they produce high levels of IL-17A and TNFα upon PMA/ionomycin stimulation (103). The fetal origin of amniotic fluid ILC3s was demonstrated by the expression of HLA class I molecules, which were not expressed on maternal peripheral blood mononuclear cells (103). Interestingly, amniotic fluid ILC3s seem to originate in the fetal lungs and intestine since a similar ILC subpopulation was identified in these organs (103). Amniotic fluid ILC3s expressed CD103, indicating an epithelial association (49, 50) that was confirmed by detection of these cells in the fetal intestinal epithelium (103). Moreover, CD103+ ILC3s were not detected in the amnion or chorion (chorioamniotic membranes), eliminating those tissues as a source of such cells in the amniotic fluid (103). Together with the observation that immune cells in the amniotic fluid during preterm gestation can be predominantly of fetal origin (142), evidence points to the fetus as a likely source of CD103+ ILC3s (Figure 3). It was proposed that these cells participate in regulating intra-amniotic infection (103); yet, their numbers remain constant between patients with and without this clinical condition (149). This finding does not discard the possibility that amniotic fluid ILC3s can acquire a regulatory phenotype, which can then participate in controlling the inflammatory response induced by microbes or danger signals in the amniotic cavity.
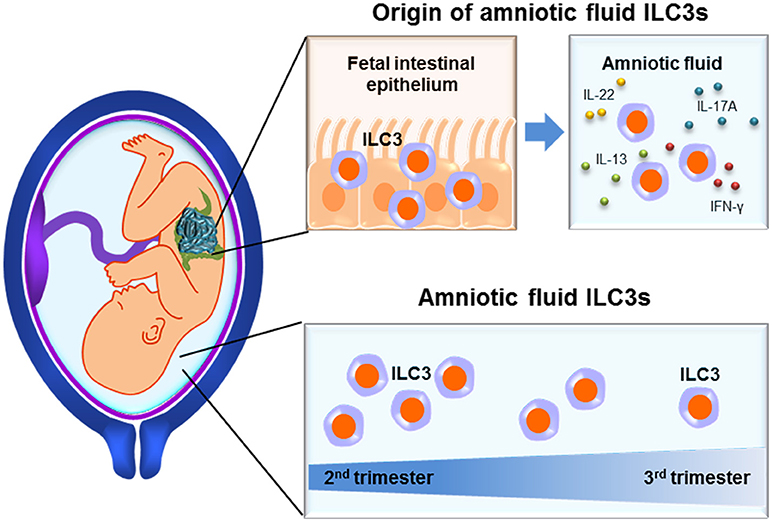
Figure 3. Innate lymphoid cells in the amniotic cavity. Functional ILC3s are found in the mucosal epithelium of fetal tissues such as the intestines, from where they may extravasate into the amniotic cavity. Amniotic fluid ILCs reach their highest proportions during the second trimester, yet are still present at the end of gestation.
Together, these studies demonstrate the presence of functional ILC3s in the amniotic cavity, which are likely derived from the fetal tissues. Such cells reach their highest proportions in the second trimester yet are still present at the end of gestation. Moreover, the detection of ILC3s in the amniotic cavity of patients with intra-amniotic inflammation suggests the participation of these cells in such a clinical condition.
Molecular studies have suggested that there is a placental microbiome (150–161). Nonetheless, recent publications have not confirmed that the placenta harbors a unique microbiome [(162); Theis et al., Am J Obstet Gynecol; in press], which supports the ongoing controversy [(162–166); Theis et al., Am J Obstet Gynecol; in press]. The absence of a placental microbiota, however, does not exclude the possibility that the fetus is exposed to microbial products from the mother. This concept is supported by another recent study showing that transient microbial colonization of the maternal gut during pregnancy induces short- and long-term innate immune changes in the offspring (167). Of interest to the ILC field, neonates born to mothers transiently microbial-colonized displayed an increased number of ILC3s in their mucosal tissues (167). The proposed mechanism for fetal exposure to maternal gut microbiota was mediated by transmission of microbial-derived metabolites via antibodies (167). Such education of the neonatal immune system was enhanced by breastfeeding (167). Therefore, microbial-derived metabolites, rather than viable bacteria, may be required for fetal and neonatal development of the ILC system.
Conclusion
The discovery of ILCs in the reproductive and fetal tissues has led to new knowledge of the immune cellular processes required for successful pregnancy and fetal development. At the same time, new questions have arisen as to the functions and interactions of ILCs in the maternal and fetal compartments. The studies reviewed herein have provided evidence that ILCs fill an important role during pregnancy, especially in mucosal defenses and fetal development, yet also share certain functions with other innate and adaptive immune cell subsets. In the mother, uterine ILCs may participate in mucosal immunity and help facilitate tissue remodeling and homeostasis during and after implantation, while decidual ILCs take part in the immune interactions required for pregnancy maintenance and maternal-fetal tolerance. Meanwhile, fetal ILCs mediate the formation of lymphoid tissues during organogenesis and reside at key mucosal surfaces, such as the intestine and lung, in preparation for fetal exposure to both commensal and pathogenic microbes. Importantly, such fetal ILCs may migrate to the amniotic fluid during intra-amniotic infection/inflammation to further participate in host defense. Collectively, the presented findings paint a complex picture of the ILC network during pregnancy, and future studies will be required in order to reveal the complete story of these unique immune cells.
Author Contributions
DM, KM, VG-F, RR, and NG-L participated in the conception, interpretation, and writing of the manuscript.
Funding
The authors are supported by the Perinatology Research Branch (PRB), Division of Intramural Research, Eunice Kennedy Shriver National Institute of Child Health and Human Development, National Institutes of Health, U.S. Department of Health and Human Services (NICHD/NIH/DHHS), and with federal funds from the NICHD/NIH/DHHS under Contract No. HHSN275201300006C. NG-L is also supported by the Wayne State University Perinatal Initiative in Maternal, Perinatal and Child Health.
Conflict of Interest Statement
The authors declare that the research was conducted in the absence of any commercial or financial relationships that could be construed as a potential conflict of interest.
Acknowledgments
The authors gratefully acknowledge Marcia Arenas-Hernandez, MSc., for her participation in critical discussions.
References
1. Gomez-Lopez N, Stlouis D, Lehr MA, Sanchez-Rodriguez EN, Arenas-Hernandez M. Immune cells in term and preterm labor. Cell Mol Immunol. (2014) 11:571–81. doi: 10.1038/cmi.2014.46
2. Bonney EA. Immune regulation in pregnancy: a matter of perspective? Obstet Gynecol Clin. (2016) 43:679–98. doi: 10.1016/j.ogc.2016.07.004
3. Erlebacher A. Immunology of the maternal-fetal interface. Annu Rev Immunol. (2013) 31:387–411. doi: 10.1146/annurev-immunol-032712-100003
4. Bonney EA. Alternative theories: pregnancy and immune tolerance. J Reprod Immunol. (2017) 123:65–71. doi: 10.1016/j.jri.2017.09.005
5. Spits H, Cupedo T. Innate lymphoid cells: emerging insights in development, lineage relationships, and function. Annu Rev Immunol. (2012) 30:647–75. doi: 10.1146/annurev-immunol-020711-075053
6. Spits H, Artis D, Colonna M, Diefenbach A, Di Santo JP, Eberl G, et al. Innate lymphoid cells–a proposal for uniform nomenclature. Nat Rev Immunol. (2013) 13:145–9. doi: 10.1038/nri3365
7. Vivier E, Artis D, Colonna M, Diefenbach A, Di Santo JP, Eberl G, et al. Innate lymphoid cells: 10 Years On. Cell (2018) 174:1054–66. doi: 10.1016/j.cell.2018.07.017
8. Powell N, Walker AW, Stolarczyk E, Canavan JB, Gokmen MR, Marks E, et al. The transcription factor T-bet regulates intestinal inflammation mediated by interleukin-7 receptor+ innate lymphoid cells. Immunity (2012) 37:674–84. doi: 10.1016/j.immuni.2012.09.008
9. Bernink JH, Peters CP, Munneke M, Te Velde AA, Meijer SL, Weijer K, et al. Human type 1 innate lymphoid cells accumulate in inflamed mucosal tissues. Nat Immunol. (2013) 14:221–9. doi: 10.1038/ni.2534
10. Fort MM, Cheung J, Yen D, Li J, Zurawski SM, Lo S, et al. IL-25 induces IL-4, IL-5, and IL-13 and Th2-associated pathologies in vivo. Immunity (2001) 15:985–95. doi: 10.1016/S1074-7613(01)00243-6
11. Hurst SD, Muchamuel T, Gorman DM, Gilbert JM, Clifford T, Kwan S, et al. New IL-17 family members promote Th1 or Th2 responses in the lung: in vivo function of the novel cytokine IL-25. J Immunol. (2002) 169:443–53. doi: 10.4049/jimmunol.169.1.443
12. Fallon PG, Ballantyne SJ, Mangan NE, Barlow JL, Dasvarma A, Hewett DR, et al. Identification of an interleukin (IL)-25-dependent cell population that provides IL-4, IL-5, and IL-13 at the onset of helminth expulsion. J Exp Med. (2006) 203:1105–16. doi: 10.1084/jem.20051615
13. Moro K, Yamada T, Tanabe M, Takeuchi T, Ikawa T, Kawamoto H, et al. Innate production of T(H)2 cytokines by adipose tissue-associated c-Kit(+)Sca-1(+) lymphoid cells. Nature (2010) 463:540–4. doi: 10.1038/nature08636
14. Neill DR, Wong SH, Bellosi A, Flynn RJ, Daly M, Langford TK, et al. Nuocytes represent a new innate effector leukocyte that mediates type-2 immunity. Nature (2010) 464:1367–70. doi: 10.1038/nature08900
15. Price AE, Liang HE, Sullivan BM, Reinhardt RL, Eisley CJ, Erle DJ, et al. Systemically dispersed innate IL-13-expressing cells in type 2 immunity. Proc Natl Acad Sci USA. (2010) 107:11489–94. doi: 10.1073/pnas.1003988107
16. Mjosberg J, Bernink J, Golebski K, Karrich JJ, Peters CP, Blom B, et al. The transcription factor GATA3 is essential for the function of human type 2 innate lymphoid cells. Immunity (2012) 37:649–59. doi: 10.1016/j.immuni.2012.08.015
17. Wong SH, Walker JA, Jolin HE, Drynan LF, Hams E, Camelo A, et al. Transcription factor RORalpha is critical for nuocyte development. Nat Immunol. (2012) 13:229–36. doi: 10.1038/ni.2208
18. Halim TY, Krauss RH, Sun AC, Takei F. Lung natural helper cells are a critical source of Th2 cell-type cytokines in protease allergen-induced airway inflammation. Immunity (2012) 36:451–63. doi: 10.1016/j.immuni.2011.12.020
19. Monticelli LA, Sonnenberg GF, Abt MC, Alenghat T, Ziegler CG, Doering TA, et al. Innate lymphoid cells promote lung-tissue homeostasis after infection with influenza virus. Nat Immunol. (2011) 12:1045–54. doi: 10.1038/ni.2131
20. Molofsky AB, Nussbaum JC, Liang HE, Van Dyken SJ, Cheng LE, Mohapatra A, et al. Innate lymphoid type 2 cells sustain visceral adipose tissue eosinophils and alternatively activated macrophages. J Exp Med. (2013) 210:535–49. doi: 10.1084/jem.20121964
21. Nussbaum JC, Van Dyken SJ, Von Moltke J, Cheng LE, Mohapatra A, Molofsky AB, et al. Type 2 innate lymphoid cells control eosinophil homeostasis. Nature (2013) 502:245–8. doi: 10.1038/nature12526
22. Adachi S, Yoshida H, Kataoka H, Nishikawa S. Three distinctive steps in Peyer's patch formation of murine embryo. Int Immunol. (1997) 9:507–14. doi: 10.1093/intimm/9.4.507
23. Yoshida H, Honda K, Shinkura R, Adachi S, Nishikawa S, Maki K, et al. IL-7 receptor alpha+ CD3(-) cells in the embryonic intestine induces the organizing center of Peyer's patches. Int Immunol. (1999) 11:643–55. doi: 10.1093/intimm/11.5.643
24. Hashi H, Yoshida H, Honda K, Fraser S, Kubo H, Awane M, et al. Compartmentalization of Peyer's patch anlagen before lymphocyte entry. J Immunol. (2001) 166:3702–9. doi: 10.4049/jimmunol.166.6.3702
25. Honda K, Nakano H, Yoshida H, Nishikawa S, Rennert P, Ikuta K, et al. Molecular basis for hematopoietic/mesenchymal interaction during initiation of Peyer's patch organogenesis. J Exp Med. (2001) 193:621–30. doi: 10.1084/jem.193.5.621
26. Eberl G, Marmon S, Sunshine MJ, Rennert PD, Choi Y, Littman DR. An essential function for the nuclear receptor RORgamma(t) in the generation of fetal lymphoid tissue inducer cells. Nat Immunol. (2004) 5:64–73. doi: 10.1038/ni1022
27. Zhong C, Zheng M, Zhu J. Lymphoid tissue inducer-a divergent member of the ILC family. Cytokine Growth Factor Rev. (2018) 42:5–12. doi: 10.1016/j.cytogfr.2018.02.004
28. Cella M, Otero K, Colonna M. Expansion of human NK-22 cells with IL-7, IL-2, and IL-1beta reveals intrinsic functional plasticity. Proc Natl Acad Sci USA. (2010) 107:10961–6. doi: 10.1073/pnas.1005641107
29. Vonarbourg C, Mortha A, Bui VL, Hernandez PP, Kiss EA, Hoyler T, et al. Regulated expression of nuclear receptor RORgammat confers distinct functional fates to NK cell receptor-expressing RORgammat(+) innate lymphocytes. Immunity (2010) 33:736–51. doi: 10.1016/j.immuni.2010.10.017
30. Klose CS, Kiss EA, Schwierzeck V, Ebert K, Hoyler T, D'hargues Y, et al. A T-bet gradient controls the fate and function of CCR6-RORgammat+ innate lymphoid cells. Nature (2013) 494:261–5. doi: 10.1038/nature11813
31. Bernink JH, Krabbendam L, Germar K, De Jong E, Gronke K, Kofoed-Nielsen M, et al. Interleukin-12 and-23 control plasticity of CD127(+) group 1 and group 3 innate lymphoid cells in the intestinal lamina propria. Immunity (2015) 43:146–60. doi: 10.1016/j.immuni.2015.06.019
32. Moffett-King A. Natural killer cells and pregnancy. Nat Rev Immunol. (2002) 2:656–63. doi: 10.1038/nri886
33. Vacca P, Mingari MC, Moretta L. Natural killer cells in human pregnancy. J Reprod Immunol. (2013) 97:14–9. doi: 10.1016/j.jri.2012.10.008
34. Moffett A, Colucci F. Uterine NK cells: active regulators at the maternal-fetal interface. J Clin Invest. (2014) 124:1872–9. doi: 10.1172/JCI68107
35. Gaynor LM, Colucci F. Uterine natural killer cells: functional distinctions and influence on pregnancy in humans and mice. Front Immunol. (2017) 8:467. doi: 10.3389/fimmu.2017.00467
36. Male V, Hughes T, Mcclory S, Colucci F, Caligiuri MA, Moffett A. Immature NK cells, capable of producing IL-22, are present in human uterine mucosa. J Immunol. (2010) 185:3913–8. doi: 10.4049/jimmunol.1001637
37. Montaldo E, Vacca P, Chiossone L, Croxatto D, Loiacono F, Martini S, et al. Unique eomes(+) NK cell subsets are present in uterus and decidua during early pregnancy. Front Immunol. (2015) 6:646. doi: 10.3389/fimmu.2015.00646
38. Doisne JM, Balmas E, Boulenouar S, Gaynor LM, Kieckbusch J, Gardner L, et al. Composition, development, and function of uterine innate lymphoid cells. J Immunol. (2015) 195:3937–45. doi: 10.4049/jimmunol.1500689
39. Boulenouar S, Doisne JM, Sferruzzi-Perri A, Gaynor LM, Kieckbusch J, Balmas E, et al. The residual innate lymphoid cells in NFIL3-deficient mice support suboptimal maternal adaptations to pregnancy. Front Immunol. (2016) 7:43. doi: 10.3389/fimmu.2016.00043
40. Li M, Gao Y, Yong L, Huang D, Shen J, Liu M, et al. Molecular signature and functional analysis of uterine ILCs in mouse pregnancy. J Reprod Immunol. (2017) 123:48–57. doi: 10.1016/j.jri.2017.09.003
41. Bartemes K, Chen CC, Iijima K, Drake L, Kita H. IL-33-Responsive group 2 innate lymphoid cells are regulated by female sex hormones in the uterus. J Immunol. (2018) 200:229–36. doi: 10.4049/jimmunol.1602085
42. Sojka DK, Plougastel-Douglas B, Yang L, Pak-Wittel MA, Artyomov MN, Ivanova Y, et al. Tissue-resident natural killer (NK) cells are cell lineages distinct from thymic and conventional splenic NK cells. eLife (2014) 3:e01659. doi: 10.7554/eLife.01659
43. Geiger TL, Abt MC, Gasteiger G, Firth MA, O'connor MH, Geary CD, et al. Nfil3 is crucial for development of innate lymphoid cells and host protection against intestinal pathogens. J Exp Med. (2014) 211:1723–31. doi: 10.1084/jem.20140212
44. Seillet C, Rankin LC, Groom JR, Mielke LA, Tellier J, Chopin M, et al. Nfil3 is required for the development of all innate lymphoid cell subsets. J Exp Med. (2014) 211:1733–40. doi: 10.1084/jem.20140145
45. Yu X, Wang Y, Deng M, Li Y, Ruhn KA, Zhang CC, et al. The basic leucine zipper transcription factor NFIL3 directs the development of a common innate lymphoid cell precursor. eLife (2014) 3:e04406. doi: 10.7554/eLife.04406
46. Daussy C, Faure F, Mayol K, Viel S, Gasteiger G, Charrier E, et al. T-bet and Eomes instruct the development of two distinct natural killer cell lineages in the liver and in the bone marrow. J Exp Med. (2014) 211:563–77. doi: 10.1084/jem.20131560
47. Male V, Nisoli I, Kostrzewski T, Allan DS, Carlyle JR, Lord GM, et al. The transcription factor E4bp4/Nfil3 controls commitment to the NK lineage and directly regulates Eomes and Id2 expression. J Exp Med. (2014) 211:635–42. doi: 10.1084/jem.20132398
48. Gordon SM, Chaix J, Rupp LJ, Wu J, Madera S, Sun JC, et al. The transcription factors T-bet and Eomes control key checkpoints of natural killer cell maturation. Immunity (2012) 36:55–67. doi: 10.1016/j.immuni.2011.11.016
49. Cepek KL, Shaw SK, Parker CM, Russell GJ, Morrow JS, Rimm DL, et al. Adhesion between epithelial cells and T lymphocytes mediated by E-cadherin and the alpha E beta 7 integrin. Nature (1994) 372:190–3. doi: 10.1038/372190a0
50. Fuchs A, Vermi W, Lee JS, Lonardi S, Gilfillan S, Newberry RD, et al. Intraepithelial type 1 innate lymphoid cells are a unique subset of IL-12- and IL-15-responsive IFN-gamma-producing cells. Immunity (2013) 38:769–81. doi: 10.1016/j.immuni.2013.02.010
51. Balmas E, Rana BM, Hamilton RS, Shreeve N, Kieckbusch J, Aye I, et al. Maternal group 2 innate lymphoid cells control fetal growth and protect from endotoxin-induced abortion in mice. bioRxiv [Preprint]. bioRxiv:348755 (2018). doi: 10.1101/348755
52. Mjosberg JM, Trifari S, Crellin NK, Peters CP, Van Drunen CM, Piet B, et al. Human IL-25- and IL-33-responsive type 2 innate lymphoid cells are defined by expression of CRTH2 and CD161. Nat Immunol. (2011) 12:1055–62. doi: 10.1038/ni.2104
53. Griesenauer B, Paczesny S. The ST2/IL-33 axis in immune cells during inflammatory diseases. Front Immunol. (2017) 8:475. doi: 10.3389/fimmu.2017.00475
54. Ikutani M, Yanagibashi T, Ogasawara M, Tsuneyama K, Yamamoto S, Hattori Y, et al. Identification of innate IL-5-producing cells and their role in lung eosinophil regulation and antitumor immunity. J Immunol. (2012) 188:703–13. doi: 10.4049/jimmunol.1101270
55. Oboki K, Ohno T, Kajiwara N, Arae K, Morita H, Ishii A, et al. IL-33 is a crucial amplifier of innate rather than acquired immunity. Proc Natl Acad Sci USA. (2010) 107:18581–6. doi: 10.1073/pnas.1003059107
56. Gellersen B, Brosens IA, Brosens JJ. Decidualization of the human endometrium: mechanisms, functions, and clinical perspectives. Semin Reprod Med. (2007) 25:445–53. doi: 10.1055/s-2007-991042
57. Mori M, Bogdan A, Balassa T, Csabai T, Szekeres-Bartho J. The decidua-the maternal bed embracing the embryo-maintains the pregnancy. Semin Immunopathol. (2016) 38:635–49. doi: 10.1007/s00281-016-0574-0
58. Vacca P, Montaldo E, Croxatto D, Loiacono F, Canegallo F, Venturini PL, et al. Identification of diverse innate lymphoid cells in human decidua. Mucosal Immunol. (2015) 8:254–64. doi: 10.1038/mi.2014.63
59. Vacca P, Vitale C, Montaldo E, Conte R, Cantoni C, Fulcheri E, et al. CD34+ hematopoietic precursors are present in human decidua and differentiate into natural killer cells upon interaction with stromal cells. Proc Natl Acad Sci USA. (2011) 108:2402–7. doi: 10.1073/pnas.1016257108
60. Carlino C, Stabile H, Morrone S, Bulla R, Soriani A, Agostinis C, et al. Recruitment of circulating NK cells through decidual tissues: a possible mechanism controlling NK cell accumulation in the uterus during early pregnancy. Blood (2008) 111:3108–15. doi: 10.1182/blood-2007-08-105965
61. Yokota Y, Mansouri A, Mori S, Sugawara S, Adachi S, Nishikawa S, et al. Development of peripheral lymphoid organs and natural killer cells depends on the helix-loop-helix inhibitor Id2. Nature (1999) 397:702–6. doi: 10.1038/17812
62. Xu Y, Romero R, Miller D, Silva P, Panaitescu B, Theis KR, et al. Innate lymphoid cells at the human maternal-fetal interface in spontaneous preterm labor. Am J Reprod Immunol. (2018) 79:e12820. doi: 10.1111/aji.12820
63. Croxatto D, Micheletti A, Montaldo E, Orecchia P, Loiacono F, Canegallo F, et al. Group 3 innate lymphoid cells regulate neutrophil migration and function in human decidua. Mucosal Immunol. (2016) 9:1372–83. doi: 10.1038/mi.2016.10
64. Amsalem H, Kwan M, Hazan A, Zhang J, Jones RL, Whittle W, et al. Identification of a novel neutrophil population: proangiogenic granulocytes in second-trimester human decidua. J Immunol. (2014) 193:3070–9. doi: 10.4049/jimmunol.1303117
65. Klose CSN, Flach M, Mohle L, Rogell L, Hoyler T, Ebert K, et al. Differentiation of type 1 ILCs from a common progenitor to all helper-like innate lymphoid cell lineages. Cell (2014) 157:340–56. doi: 10.1016/j.cell.2014.03.030
66. Cherrier M, Eberl G. The development of LTi cells. Curr Opin Immunol. (2012) 24:178–83. doi: 10.1016/j.coi.2012.02.003
67. Adachi S, Yoshida H, Honda K, Maki K, Saijo K, Ikuta K, et al. Essential role of IL-7 receptor alpha in the formation of Peyer's patch anlage. Int Immunol. (1998) 10:1–6. doi: 10.1093/intimm/10.1.1
68. Cupedo T, Vondenhoff MF, Heeregrave EJ, De Weerd AE, Jansen W, Jackson DG, et al. Presumptive lymph node organizers are differentially represented in developing mesenteric and peripheral nodes. J Immunol. (2004) 173:2968–75. doi: 10.4049/jimmunol.173.5.2968
69. Okuda M, Togawa A, Wada H, Nishikawa S. Distinct activities of stromal cells involved in the organogenesis of lymph nodes and Peyer's patches. J Immunol. (2007) 179:804–11. doi: 10.4049/jimmunol.179.2.804
70. Song C, Lee JS, Gilfillan S, Robinette ML, Newberry RD, Stappenbeck TS, et al. Unique and redundant functions of NKp46+ ILC3s in models of intestinal inflammation. J Exp Med. (2015) 212:1869–82. doi: 10.1084/jem.20151403
71. Pahal GS, Jauniaux E, Kinnon C, Thrasher AJ, Rodeck CH. Normal development of human fetal hematopoiesis between eight and seventeen weeks' gestation. Am J Obstet Gynecol. (2000) 183:1029–34. doi: 10.1067/mob.2000.106976
72. Rollini P, Faes-Van't Hull E, Kaiser S, Kapp U, Leyvraz S. Phenotypic and functional analysis of human fetal liver hematopoietic stem cells in culture. Stem Cells Dev. (2007) 16:281–96. doi: 10.1089/scd.2006.0096
73. Mebius RE, Miyamoto T, Christensen J, Domen J, Cupedo T, Weissman IL, et al. The fetal liver counterpart of adult common lymphoid progenitors gives rise to all lymphoid lineages, CD45+CD4+CD3- cells, as well as macrophages. J Immunol. (2001) 166:6593–601. doi: 10.4049/jimmunol.166.11.6593
74. Cherrier M, Sawa S, Eberl G. Notch, Id2, and RORgammat sequentially orchestrate the fetal development of lymphoid tissue inducer cells. J Exp Med. (2012) 209:729–40. doi: 10.1084/jem.20111594
75. Constantinides MG, Mcdonald BD, Verhoef PA, Bendelac A. A committed precursor to innate lymphoid cells. Nature (2014) 508:397–401. doi: 10.1038/nature13047
76. Lim AI, Li Y, Lopez-Lastra S, Stadhouders R, Paul F, Casrouge A, et al. Systemic human ILC precursors provide a substrate for tissue ILC differentiation. Cell (2017) 168:1086–1100.E10. doi: 10.1016/j.cell.2017.02.021
77. Tan JK, Watanabe T. Murine spleen tissue regeneration from neonatal spleen capsule requires lymphotoxin priming of stromal cells. J Immunol. (2014) 193:1194–203. doi: 10.4049/jimmunol.1302115
78. Hoorweg K, Narang P, Li Z, Thuery A, Papazian N, Withers DR, et al. A stromal cell niche for human and mouse type 3 innate lymphoid cells. J Immunol. (2015) 195:4257–63. doi: 10.4049/jimmunol.1402584
79. Artis D, Spits H. The biology of innate lymphoid cells. Nature (2015) 517:293–301. doi: 10.1038/nature14189
80. Yu JC, Khodadadi H, Malik A, Davidson B, Salles E, Bhatia J, et al. Innate immunity of neonates and infants. Front Immunol. (2018) 9:1759. doi: 10.3389/fimmu.2018.01759
81. Wigle JT, Oliver G. Prox1 function is required for the development of the murine lymphatic system. Cell (1999) 98:769–78. doi: 10.1016/S0092-8674(00)81511-1
82. Mebius RE. Organogenesis of lymphoid tissues. Nat Rev Immunol. (2003) 3:292–303. doi: 10.1038/nri1054
83. Van De Pavert SA, Mebius RE. New insights into the development of lymphoid tissues. Nat Rev Immunol. (2010) 10:664–74. doi: 10.1038/nri2832
84. Yoshida H, Kawamoto H, Santee SM, Hashi H, Honda K, Nishikawa S, et al. Expression of alpha(4)beta(7) integrin defines a distinct pathway of lymphoid progenitors committed to T cells, fetal intestinal lymphotoxin producer, NK, and dendritic cells. J Immunol. (2001) 167:2511–21. doi: 10.4049/jimmunol.167.5.2511
85. Possot C, Schmutz S, Chea S, Boucontet L, Louise A, Cumano A, et al. Notch signaling is necessary for adult, but not fetal, development of RORgammat(+) innate lymphoid cells. Nat Immunol. (2011) 12:949–58. doi: 10.1038/ni.2105
86. Tachibana M, Tenno M, Tezuka C, Sugiyama M, Yoshida H, Taniuchi I. Runx1/Cbfbeta2 complexes are required for lymphoid tissue inducer cell differentiation at two developmental stages. J Immunol. (2011) 186:1450–7. doi: 10.4049/jimmunol.1000162
87. Serafini N, Klein Wolterink RG, Satoh-Takayama N, Xu W, Vosshenrich CA, Hendriks RW, et al. Gata3 drives development of RORgammat+ group 3 innate lymphoid cells. J Exp Med. (2014) 211:199–208. doi: 10.1084/jem.20131038
88. Ishizuka IE, Constantinides MG, Gudjonson H, Bendelac A. The innate lymphoid cell precursor. Annu Rev Immunol. (2016) 34:299–316. doi: 10.1146/annurev-immunol-041015-055549
89. Bando JK, Liang HE, Locksley RM. Identification and distribution of developing innate lymphoid cells in the fetal mouse intestine. Nat Immunol. (2015) 16:153–60. doi: 10.1038/ni.3057
90. Forkel M, Berglin L, Kekalainen E, Carlsson A, Svedin E, Michaelsson J, et al. Composition and functionality of the intrahepatic innate lymphoid cell-compartment in human nonfibrotic and fibrotic livers. Eur J Immunol. (2017) 47:1280–94. doi: 10.1002/eji.201646890
91. Shikhagaie MM, Bjorklund AK, Mjosberg J, Erjefalt JS, Cornelissen AS, Ros XR, et al. Neuropilin-1 is expressed on lymphoid tissue residing LTi-like group 3 innate lymphoid cells and associated with ectopic lymphoid aggregates. Cell Rep. (2017) 18:1761–73. doi: 10.1016/j.celrep.2017.01.063
92. Robinette ML, Fuchs A, Cortez VS, Lee JS, Wang Y, Durum SK, et al. Transcriptional programs define molecular characteristics of innate lymphoid cell classes and subsets. Nat Immunol. (2015) 16:306–17. doi: 10.1038/ni.3094
93. Roozendaal R, Mebius RE. Stromal cell-immune cell interactions. Annu Rev Immunol. (2011) 29:23–43. doi: 10.1146/annurev-immunol-031210-101357
94. De Togni P, Goellner J, Ruddle NH, Streeter PR, Fick A, Mariathasan S, et al. Abnormal development of peripheral lymphoid organs in mice deficient in lymphotoxin. Science (1994) 264:703–7. doi: 10.1126/science.8171322
95. Koni PA, Sacca R, Lawton P, Browning JL, Ruddle NH, Flavell RA. Distinct roles in lymphoid organogenesis for lymphotoxins alpha and beta revealed in lymphotoxin beta-deficient mice. Immunity (1997) 6:491–500. doi: 10.1016/S1074-7613(00)80292-7
96. Futterer A, Mink K, Luz A, Kosco-Vilbois MH, Pfeffer K. The lymphotoxin beta receptor controls organogenesis and affinity maturation in peripheral lymphoid tissues. Immunity (1998) 9:59–70. doi: 10.1016/S1074-7613(00)80588-9
97. Cupedo T, Crellin NK, Papazian N, Rombouts EJ, Weijer K, Grogan JL, et al. Human fetal lymphoid tissue-inducer cells are interleukin 17-producing precursors to RORC+ CD127+ natural killer-like cells. Nat Immunol. (2009) 10:66–74. doi: 10.1038/ni.1668
98. Hoorweg K, Peters CP, Cornelissen F, Aparicio-Domingo P, Papazian N, Kazemier G, et al. Functional differences between human NKp44(-) and NKp44(+) RORC(+) innate lymphoid cells. Front Immunol. (2012) 3:72. doi: 10.3389/fimmu.2012.00072
99. Takashima S, Kitakaze M, Asakura M, Asanuma H, Sanada S, Tashiro F, et al. Targeting of both mouse neuropilin-1 and neuropilin-2 genes severely impairs developmental yolk sac and embryonic angiogenesis. Proc Natl Acad Sci USA. (2002) 99:3657–62. doi: 10.1073/pnas.022017899
100. Sonnenberg GF, Monticelli LA, Elloso MM, Fouser LA, Artis D. CD4(+) lymphoid tissue-inducer cells promote innate immunity in the gut. Immunity (2011) 34:122–34. doi: 10.1016/j.immuni.2010.12.009
101. Hashiguchi M, Kashiwakura Y, Kojima H, Kobayashi A, Kanno Y, Kobata T. Peyer's patch innate lymphoid cells regulate commensal bacteria expansion. Immunol Lett. (2015) 165:1–9. doi: 10.1016/j.imlet.2015.03.002
102. Li N, Van Unen V, Hollt T, Thompson A, Van Bergen J, Pezzotti N, et al. Mass cytometry reveals innate lymphoid cell differentiation pathways in the human fetal intestine. J Exp Med. (2018) 215:1383–96. doi: 10.1084/jem.20171934
103. Marquardt N, Ivarsson MA, Sundstrom E, Akesson E, Martini E, Eidsmo L, et al. Fetal CD103+ IL-17-producing group 3 innate lymphoid cells represent the dominant lymphocyte subset in human amniotic fluid. J Immunol. (2016) 197:3069–75. doi: 10.4049/jimmunol.1502204
104. Frascoli M, Jeanty C, Fleck S, Moradi PW, Keating S, Mattis AN, et al. Heightened immune activation in fetuses with gastroschisis may be blocked by targeting IL-5. J Immunol. (2016) 196:4957–66. doi: 10.4049/jimmunol.1502587
105. Koyasu S, Moro K. Type 2 innate immune responses and the natural helper cell. Immunology (2011) 132:475–81. doi: 10.1111/j.1365-2567.2011.03413.x
106. Chang YJ, Kim HY, Albacker LA, Baumgarth N, Mckenzie AN, Smith DE, et al. Innate lymphoid cells mediate influenza-induced airway hyper-reactivity independently of adaptive immunity. Nat Immunol. (2011) 12:631–8. doi: 10.1038/ni.2045
107. Lambrecht BN, Hammad H. The immunology of asthma. Nat Immunol. (2015) 16:45–56. doi: 10.1038/ni.3049
108. Saluzzo S, Gorki AD, Rana BMJ, Martins R, Scanlon S, Starkl P, et al. First-Breath-induced type 2 pathways shape the lung immune environment. Cell Rep. (2017) 18:1893–905. doi: 10.1016/j.celrep.2017.01.071
109. Schmidt W. The amniotic fluid compartment: the fetal habitat. Adv Anat Embryol Cell Biol. (1992) 127:1–100.
110. Davis LE, Mclaren LC, Stewart JA, James CG, Levine MD, Skipper BJ. Immunological and microbiological studies of midtrimester amniotic fluid. Gynecol Obstet Invest. (1983) 16:261–8. doi: 10.1159/000299275
111. Cherry SH. Amniotic fluid analysis as an index of fetal health in utero. Med Times (1967) 95:713–7.
112. Barham KA. Amnioscopy, meconium and fetal well-being. J Obstet Gynaecol Br Commonw. (1969) 76:412–8. doi: 10.1111/j.1471-0528.1969.tb05856.x
113. Clark SL, Romero R, Dildy GA, Callaghan WM, Smiley RM, Bracey AW, et al. Proposed diagnostic criteria for the case definition of amniotic fluid embolism in research studies. Am J Obstet Gynecol. (2016) 215:408–12. doi: 10.1016/j.ajog.2016.06.037
114. Tarui T, Kim A, Flake A, Mcclain L, Stratigis JD, Fried I, et al. Amniotic fluid transcriptomics reflects novel disease mechanisms in fetuses with myelomeningocele. Am J Obstet Gynecol. (2017) 217:587. e1–10. doi: 10.1016/j.ajog.2017.07.022
116. Hobbins JC, Brock W, Speroff L, Anderson GG, Caldwell B. L-S ratio in predicting pulmonary maturity in utero. Obstet Gynecol. (1972) 39:660–4.
117. Palacio M, Bonet-Carne E, Cobo T, Perez-Moreno A, Sabria J, Richter J, et al. Prediction of neonatal respiratory morbidity by quantitative ultrasound lung texture analysis: a multicenter study. Am J Obstet Gynecol. (2017) 217:196. e1–14. doi: 10.1016/j.ajog.2017.03.016
118. Jacobson CB, Barter RH. Intrauterine diagnosis and management of genetic defects. Am J Obstet Gynecol. (1967) 99:796–807. doi: 10.1016/0002-9378(67)90395-X
119. Lisgar F, Gertner M, Cherry S, Hsu LY, Hirschhorn K. Prenatal chromosome analysis. Nature (1970) 225:280–1. doi: 10.1038/225280a0
120. Romero R, Emamian M, Quintero R, Wan M, Hobbins JC, Mitchell MD. Amniotic fluid prostaglandin levels and intra-amniotic infections. Lancet (1986) 1:1380. doi: 10.1016/S0140-6736(86)91685-5
121. Romero R, Emamian M, Quintero R, Wan M, Hobbins JC, Mazor M, et al. The value and limitations of the Gram stain examination in the diagnosis of intraamniotic infection. Am J Obstet Gynecol. (1988) 159:114–9. doi: 10.1016/0002-9378(88)90503-0
122. Romero R, Sirtori M, Oyarzun E, Avila C, Mazor M, Callahan R, et al. Infection and labor. V Prevalence, microbiology, and clinical significance of intraamniotic infection in women with preterm labor and intact membranes Am J Obstet Gynecol. (1989) 161:817–24. doi: 10.1016/0002-9378(89)90409-2
123. Romero R, Avila C, Santhanam U, Sehgal PB. Amniotic fluid interleukin 6 in preterm labor. Association with infection. J Clin Invest. (1990) 85:1392–400. doi: 10.1172/JCI114583
124. Romero R, Jimenez C, Lohda AK, Nores J, Hanaoka S, Avila C, et al. Amniotic fluid glucose concentration: a rapid and simple method for the detection of intraamniotic infection in preterm labor. Am J Obstet Gynecol. (1990) 163:968–74. doi: 10.1016/0002-9378(90)91106-M
125. Hsu CD, Meaddough E, Aversa K, Copel JA. The role of amniotic fluid L-selectin, GRO-alpha, and interleukin-8 in the pathogenesis of intraamniotic infection. Am J Obstet Gynecol. (1998) 178:428–32. doi: 10.1016/S0002-9378(98)70414-4
126. Yoon BH, Romero R, Moon JB, Shim SS, Kim M, Kim G, et al. Clinical significance of intra-amniotic inflammation in patients with preterm labor and intact membranes. Am J Obstet Gynecol. (2001) 185:1130–6. doi: 10.1067/mob.2001.117680
127. Keelan JA, Yang J, Romero RJ, Chaiworapongsa T, Marvin KW, Sato TA, et al. Epithelial cell-derived neutrophil-activating peptide-78 is present in fetal membranes and amniotic fluid at increased concentrations with intra-amniotic infection and preterm delivery. Biol Reprod. (2004) 70:253–9. doi: 10.1095/biolreprod.103.016204
128. Kusanovic JP, Espinoza J, Romero R, Goncalves LF, Nien JK, Soto E, et al. Clinical significance of the presence of amniotic fluid 'sludge' in asymptomatic patients at high risk for spontaneous preterm delivery. Ultrasound Obstet Gynecol. (2007) 30:706–14. doi: 10.1002/uog.4081
129. Chaemsaithong P, Romero R, Korzeniewski SJ, Martinez-Varea A, Dong Z, Yoon BH, et al. A rapid interleukin-6 bedside test for the identification of intra-amniotic inflammation in preterm labor with intact membranes. J Matern Fetal Neonatal Med. (2016) 29:349–59. doi: 10.3109/14767058.2015.1006620
130. Yoneda N, Yoneda S, Niimi H, Ueno T, Hayashi S, Ito M, et al. Polymicrobial amniotic fluid infection with mycoplasma/ureaplasma and other bacteria induces severe intra-amniotic inflammation associated with poor perinatal prognosis in preterm labor. Am J Reprod Immunol. (2016) 75:112–25. doi: 10.1111/aji.12456
131. Yoneda S, Shiozaki A, Yoneda N, Ito M, Shima T, Fukuda K, et al. Antibiotic therapy increases the risk of preterm birth in preterm labor without intra-amniotic microbes, but may prolong the gestation period in preterm labor with microbes, evaluated by rapid and high-sensitive PCR system. Am J Reprod Immunol. (2016) 75:440–50. doi: 10.1111/aji.12484
132. Rowlands S, Danielewski JA, Tabrizi SN, Walker SP, Garland SM. Microbial invasion of the amniotic cavity in midtrimester pregnancies using molecular microbiology. Am J Obstet Gynecol. (2017) 217:71. e1–5. doi: 10.1016/j.ajog.2017.02.051
133. Combs CA, Gravett M, Garite TJ, Hickok DE, Lapidus J, Porreco R, et al. Amniotic fluid infection, inflammation, and colonization in preterm labor with intact membranes. Am J Obstet Gynecol. (2014) 210:125. e1–15. doi: 10.1016/j.ajog.2013.11.032
134. Romero R, Miranda J, Chaiworapongsa T, Chaemsaithong P, Gotsch F, Dong Z, et al. A novel molecular microbiologic technique for the rapid diagnosis of microbial invasion of the amniotic cavity and intra-amniotic infection in preterm labor with intact membranes. Am J Reprod Immunol. (2014) 71:330–58. doi: 10.1111/aji.12189
135. Romero R, Miranda J, Chaiworapongsa T, Chaemsaithong P, Gotsch F, Dong Z, et al. Sterile intra-amniotic inflammation in asymptomatic patients with a sonographic short cervix: prevalence and clinical significance. J Matern Fetal Neonatal Med. (2015) 28:1343–59. doi: 10.3109/14767058.2014.954243
136. Romero R, Miranda J, Chaiworapongsa T, Korzeniewski SJ, Chaemsaithong P, Gotsch F, et al. Prevalence and clinical significance of sterile intra-amniotic inflammation in patients with preterm labor and intact membranes. Am J Reprod Immunol. (2014) 72:458–74. doi: 10.1111/aji.12296
137. Romero R, Miranda J, Chaemsaithong P, Chaiworapongsa T, Kusanovic JP, Dong Z, et al. Sterile and microbial-associated intra-amniotic inflammation in preterm prelabor rupture of membranes. J Matern Fetal Neonatal Med. (2015) 28:1394–409. doi: 10.3109/14767058.2014.958463
138. Gomez-Lopez N, Romero R, Panaitescu B, Leng Y, Xu Y, Tarca AL, et al. Inflammasome activation during spontaneous preterm labor with intra-amniotic infection or sterile intra-amniotic inflammation. Am J Reprod Immunol. (2018) 18:e13049. doi: 10.1111/aji.13049
139. Romero R, Quintero R, Nores J, Avila C, Mazor M, Hanaoka S, et al. Amniotic fluid white blood cell count: a rapid and simple test to diagnose microbial invasion of the amniotic cavity and predict preterm delivery. Am J Obstet Gynecol. (1991) 165:821–30. doi: 10.1016/0002-9378(91)90423-O
140. Martinez-Varea A, Romero R, Xu Y, Miller D, Ahmed AI, Chaemsaithong P, et al. Clinical chorioamnionitis at term VII: the amniotic fluid cellular immune response. J Perinat Med. (2017) 45:523–38. doi: 10.1515/jpm-2016-0225
141. Sampson JE, Theve RP, Blatman RN, Shipp TD, Bianchi DW, Ward BE, et al. Fetal origin of amniotic fluid polymorphonuclear leukocytes. Am J Obstet Gynecol. (1997) 176:77–81. doi: 10.1016/S0002-9378(97)80015-4
142. Gomez-Lopez N, Romero R, Xu Y, Leng Y, Garcia-Flores V, Miller D, et al. Are amniotic fluid neutrophils in women with intraamniotic infection and/or inflammation of fetal or maternal origin? Am J Obstet Gynecol. (2017) 217:693. e1–e16. doi: 10.1016/j.ajog.2017.09.013
143. Espinoza J, Chaiworapongsa T, Romero R, Edwin S, Rathnasabapathy C, Gomez R, et al. Antimicrobial peptides in amniotic fluid: defensins, calprotectin and bacterial/permeability-increasing protein in patients with microbial invasion of the amniotic cavity, intra-amniotic inflammation, preterm labor and premature rupture of membranes. J Matern Fetal Neonatal Med. (2003) 13:2–21. doi: 10.1080/jmf.13.1.2.21
144. Soto E, Espinoza J, Nien JK, Kusanovic JP, Erez O, Richani K, et al. Human beta-defensin-2: a natural antimicrobial peptide present in amniotic fluid participates in the host response to microbial invasion of the amniotic cavity. J Matern Fetal Neonatal Med. (2007) 20:15–22. doi: 10.1080/14767050601036212
145. Varrey A, Romero R, Panaitescu B, Miller D, Chaiworapongsa T, Patwardhan M, et al. Human beta-defensin-1: a natural antimicrobial peptide present in amniotic fluid that is increased in spontaneous preterm labor with intra-amniotic infection. Am J Reprod Immunol. (2018) 80:e13031. doi: 10.1111/aji.13031
146. Gomez-Lopez N, Romero R, Garcia-Flores V, Xu Y, Leng Y, Alhousseini A, et al. Amniotic fluid neutrophils can phagocytize bacteria: a mechanism for microbial killing in the amniotic cavity. Am J Reprod Immunol. (2017) 78:e12723. doi: 10.1111/aji.12723
147. Gomez-Lopez N, Romero R, Leng Y, Garcia-Flores V, Xu Y, Miller D, et al. Neutrophil extracellular traps in acute chorioamnionitis: a mechanism of host defense. Am J Reprod Immunol. (2017) 77:e12617. doi: 10.1111/aji.12617
148. Gomez-Lopez N, Romero R, Xu Y, Miller D, Unkel R, Shaman M, et al. Neutrophil extracellular traps in the amniotic cavity of women with intra-amniotic infection: a new mechanism of host defense. Reprod Sci. (2017) 24:1139–53. doi: 10.1177/1933719116678690
149. Gomez-Lopez N, Romero R, Xu Y, Miller D, Leng Y, Panaitescu B, et al. The immunophenotype of amniotic fluid leukocytes in normal and complicated pregnancies. Am J Reprod Immunol. (2018) 79:e12827. doi: 10.1111/aji.12827
150. Aagaard K, Ma J, Antony KM, Ganu R, Petrosino J, Versalovic J. The placenta harbors a unique microbiome. Sci Transl Med. (2014) 6:237ra265. doi: 10.1126/scitranslmed.3008599
151. Doyle RM, Alber DG, Jones HE, Harris K, Fitzgerald F, Peebles D, et al. Term and preterm labour are associated with distinct microbial community structures in placental membranes which are independent of mode of delivery. Placenta (2014) 35:1099–101. doi: 10.1016/j.placenta.2014.10.007
152. Antony KM, Ma J, Mitchell KB, Racusin DA, Versalovic J, Aagaard K. The preterm placental microbiome varies in association with excess maternal gestational weight gain. Am J Obstet Gynecol. (2015) 212:653.e1–16. doi: 10.1016/j.ajog.2014.12.041
153. Zheng J, Xiao X, Zhang Q, Mao L, Yu M, Xu J. The placental microbiome varies in association with low birth weight in full-term neonates. Nutrients (2015) 7:6924–37. doi: 10.3390/nu7085315
154. Bassols J, Serino M, Carreras-Badosa G, Burcelin R, Blasco-Baque V, Lopez-Bermejo A, et al. Gestational diabetes is associated with changes in placental microbiota and microbiome. Pediatr Res. (2016) 80:777–84. doi: 10.1038/pr.2016.155
155. Collado MC, Rautava S, Aakko J, Isolauri E, Salminen S. Human gut colonisation may be initiated in utero by distinct microbial communities in the placenta and amniotic fluid. Sci Rep. (2016) 6:23129. doi: 10.1038/srep23129
156. Prince AL, Ma J, Kannan PS, Alvarez M, Gisslen T, Harris RA, et al. The placental membrane microbiome is altered among subjects with spontaneous preterm birth with and without chorioamnionitis. Am J Obstet Gynecol. (2016) 214:627.e1–16. doi: 10.1016/j.ajog.2016.01.193
157. Doyle RM, Harris K, Kamiza S, Harjunmaa U, Ashorn U, Nkhoma M, et al. Bacterial communities found in placental tissues are associated with severe chorioamnionitis and adverse birth outcomes. PLoS ONE (2017) 12:e0180167. doi: 10.1371/journal.pone.0180167
158. Gomez-Arango LF, Barrett HL, Mcintyre HD, Callaway LK, Morrison M, Nitert MD. Contributions of the maternal oral and gut microbiome to placental microbial colonization in overweight and obese pregnant women. Sci Rep. (2017) 7:2860. doi: 10.1038/s41598-017-03066-4
159. Parnell LA, Briggs CM, Cao B, Delannoy-Bruno O, Schrieffer AE, Mysorekar IU. Microbial communities in placentas from term normal pregnancy exhibit spatially variable profiles. Sci Rep. (2017) 7:11200. doi: 10.1038/s41598-017-11514-4
160. Zheng J, Xiao XH, Zhang Q, Mao LL, Yu M, Xu JP, et al. Correlation of placental microbiota with fetal macrosomia and clinical characteristics in mothers and newborns. Oncotarget (2017) 8:82314–25. doi: 10.18632/oncotarget.19319
161. Leon LJ, Doyle R, Diez-Benavente E, Clark TG, Klein N, Stanier P, et al. Enrichment of clinically relevant organisms in spontaneous preterm delivered placenta and reagent contamination across all clinical groups in a large pregnancy cohort in the United Kingdom. Appl Environ Microbiol. (2018) 84:e00483–18. doi: 10.1128/AEM.00483-18
162. Lauder AP, Roche AM, Sherrill-Mix S, Bailey A, Laughlin AL, Bittinger K, et al. Comparison of placenta samples with contamination controls does not provide evidence for a distinct placenta microbiota. Microbiome (2016) 4:29. doi: 10.1186/s40168-016-0172-3
163. Hornef M, Penders J. Does a prenatal bacterial microbiota exist? Mucosal Immunol. (2017) 10:598–601. doi: 10.1038/mi.2016.141
164. Perez-Munoz ME, Arrieta MC, Ramer-Tait AE, Walter J. A critical assessment of the “sterile womb” and “in utero colonization” hypotheses: implications for research on the pioneer infant microbiome. Microbiome (2017) 5:48. doi: 10.1186/s40168-017-0268-4
165. de Goffau MC, Lager S, Salter SJ, Wagner J, Kronbichler A, Charnock-Jones DS, et al. Recognizing the reagent microbiome. Nat Microbiol. (2018) 3:851–3. doi: 10.1038/s41564-018-0202-y
166. Willyard C. Could baby's first bacteria take root before birth? Nature (2018) 553:264–6. doi: 10.1038/d41586-018-00664-8
Keywords: amniotic cavity, decidua, LTi, maternal-fetal interface, neonate, pregnancy, preterm labor, uterus
Citation: Miller D, Motomura K, Garcia-Flores V, Romero R and Gomez-Lopez N (2018) Innate Lymphoid Cells in the Maternal and Fetal Compartments. Front. Immunol. 9:2396. doi: 10.3389/fimmu.2018.02396
Received: 30 August 2018; Accepted: 27 September 2018;
Published: 26 October 2018.
Edited by:
Jessica Borger, Monash University, AustraliaReviewed by:
Francesco Colucci, University of Cambridge, United KingdomRachel Golub, Paris Diderot University, France
Copyright © 2018 Miller, Motomura, Garcia-Flores, Romero and Gomez-Lopez. This is an open-access article distributed under the terms of the Creative Commons Attribution License (CC BY). The use, distribution or reproduction in other forums is permitted, provided the original author(s) and the copyright owner(s) are credited and that the original publication in this journal is cited, in accordance with accepted academic practice. No use, distribution or reproduction is permitted which does not comply with these terms.
*Correspondence: Nardhy Gomez-Lopez, bmFyZGh5LmdvbWV6LWxvcGV6QHdheW5lLmVkdQ==; bmdvbWV6bG9AbWVkLndheW5lLmVkdQ==
†These authors have contributed equally to this work