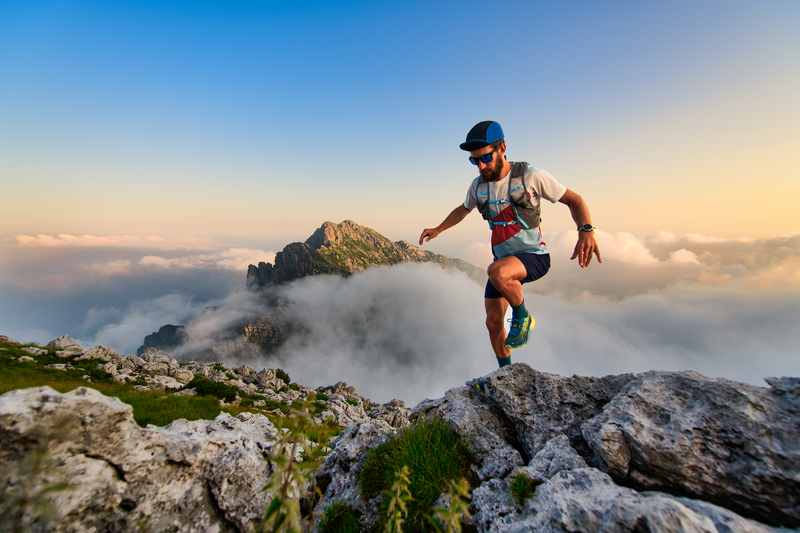
94% of researchers rate our articles as excellent or good
Learn more about the work of our research integrity team to safeguard the quality of each article we publish.
Find out more
REVIEW article
Front. Immunol. , 19 November 2018
Sec. Inflammation
Volume 9 - 2018 | https://doi.org/10.3389/fimmu.2018.02364
This article is part of the Research Topic Nano- and Microparticle-Induced Cell Death, Inflammation and Immune Responses View all 21 articles
Fibrosis, cancer, and autoimmunity developing upon particle exposure have been exclusively linked with uncontrolled inflammatory processes. The critical role of inflammation is now challenged by several contradictory observations indicating that the emergence of these chronic disorders may result from non-inflammatory events. A growing number of studies reveals that micro- and nano-particles can cause exaggerated and persistent immunosuppression characterized by the release of potent anti-inflammatory cytokines (IL-10 and TGF-β), and the recruitment of major regulatory immune cells (M2 macrophages, T and B regs, and MDSC). This persistent immunosuppressive environment is initially established to limit early inflammation but contributes later to fibrosis, cancer, and infection. Immunosuppression promotes fibroblast proliferation and matrix element synthesis and subverts innate and adaptive immune surveillance against tumor cells and microorganisms. This review details the contribution of immunosuppressive cells and their derived immunoregulatory mediators and delineates the mutual role of inflammatory vs. immunosuppressive mechanisms in the pathogenesis of chronic diseases induced by particles. The consideration of these new results explains how particle-related diseases can develop independently of chronic inflammation, enriches current bioassays predicting particle toxicity and suggests new clinical strategies for treating patients affected by particle-associated diseases.
The toxicity of inhaled particles and the subsequent development of health diseases including fibrosis, cancer and autoimmunity are recognized since several centuries (1). Unfortunately, the available scientific knowledge is not yet adequate for monitoring particle exposure-related health problems, delivering treatment to affected patients, and devising appropriate early recognition of reactive particles and individuals at risk (2–4). For example, although silicosis has been known for decades, exposure to dusts containing free crystalline silica remains uncontrolled in countless workplaces throughout the world and clinical cases of chronic diseases caused by inhaled particles are still frequent (5–7). Despite active research, the highly lethal disorders caused by silica inhalation continue to pose major clinical challenges because there are refractory to current therapeutic strategies (8). In addition, clinical detection of silica-associated lung diseases is currently dependent on radiological and lung function tests, which are both late manifestations of diseases (8). Therefore, effective therapeutic regimen and early disease detection have yet to be identified and developed. This problematic is not limited to naturally occurring mineral dusts. The recent ability to manipulate the structure and the morphology of particles, allowing the production of tailor-made dusts with specific size, form and function opens very attractive avenues in nanobiotechnologies and industries. However, for the successful application of these new materials now largely designed and produced worldwide, it is becoming evident that the biological fate and potential toxicity of these particles have to be actively determined (9). Hence, it is becoming vital to understand how complex pathologies (fibrosis, cancer, and autoimmune disorders) are induced by inhaled particles in order to reduce their impacts on human health.
The fundamental concept, usually put forward to explain the pathogenesis of particle-induced fibrosis, cancer, and autoimmune diseases, is relatively simple. An unresolved and chronic inflammatory process, characterized by marked inflammatory cell accumulation and sustained release of inflammatory molecules, damages tissues, orchestrates accumulation of mesenchymal cells and their matrix protein products, transforms normal cells to tumor cells or activates adaptive immune responses to self-antigens (10).
A precise cascade of cellular and molecular inflammatory events has been proposed from an extensive and growing number of data in human and animal exposed to particles (Figure 1). First, sentinel (alveolar and interstitial macrophages) and resident cells (epithelial cells and fibroblasts) actively recognize particles through sophisticated innate immune platforms (11, 12). These activated cells release various inflammatory mediators such as cytokines, chemokines, eicosanoids, and free radicals (13–16). These mediators induce a marked and persistent recruitment of inflammatory myeloid or lymphoid cells (inflammatory M1 macrophages; neutrophils, monocytes, and effector T lymphocytes) that results in tissue damages. Persistent inflammation-induced injury is subsequently followed by an exaggerated reparative phase in which polypeptide growth factors produced by inflammatory cells stimulate non-controlled fibroblast recruitment, proliferation, and matrix protein production (1). Released reactive oxygen or nitrogen species (ROS and RNS) play a major role in the genotoxic activity of particles. Under inflammatory conditions, free radicals generated from the particle itself or inflammatory cells induce DNA damage in target cell populations and participate in the carcinogenic process (17). Again, tissue damage and inflammation associated to inhaled particles are the suspected pathological pathway that leads to immune abnormalities, adaptive immunity activation, tolerance breaking, antinuclear autoantibody production and finally systemic autoimmune diseases including lupus and arthritis (10).
Figure 1. Predominant unresolved inflammation contributes in the development of chronic diseases related to particle exposure. The pathological pathway classically described suggests that a predominant and persistent inflammatory process (in green) orchestrates fibrosis, cancer, and autoimmune diseases caused by particles (in yellow). Reactive particles induce an inflammatory cascade, which implies TNF-α, IL-1 α, and β, IFNs (IFN-γ and β), IL-17, and free radicals (ROS and RNS) and precedes the influx of inflammatory macrophages (M1 Mϕ), inflammatory myeloid cells (iMono, inflammatory monocytes; iNeut, inflammatory neutrophils), and effector T lymphocytes (T eff, comprising Th1, γδ T cells, and Th17). When immunosuppressive activities are insufficient, these pro-inflammatory mediators and cells persist and result in to uncontrolled cycle of injury. Pro-inflammatory cytokines are also considered as potent polypeptide growth factors for mesenchymal cells and ultimately induce fibrosis. Sustained production of free radicals induces irreversible DNA damage and results in carcinogenesis. Constant tissue damage and inflammation activate adaptive immunity, autoantibody production and autoimmunity.
A myriad of inflammatory mediators accompanying inflammation are linked to scar formation, cell transformation, and autoimmunity but only few of them are definitively identified as important in the pathological processes. Among those, the master pro-inflammatory cytokines TNF-α, IL-1 (mainly produced by M1 macrophages), type I and II IFN and IL-17 (from effector T lymphocytes as Th1, γδ T cells, and Th17) are essential in the pathogenesis of particle-induced diseases (10, 18–21). These inflammatory mediators are responsible of leukocyte influx in damaged tissues and orchestrate aggregation of mature phagocytic macrophages and granuloma formation (22, 23). In addition, they promote fibroblast activation and differentiation (24), epithelial or mesothelial cell transformation (13, 25) and immune responses to self-antigens (26–28). Inflammatory cell accumulation, collagen deposition, or malignant mesothelioma in silica- or asbestos-treated mice was markedly decreased by suppressing genetically or pharmacologically these key inflammatory mediators (13, 29). Increased levels of TNF-α, IL-1, IFN-γ, and IL-17 have been observed in human under conditions of developing silicosis, asbestosis or mesothelioma (30–33). These findings convincingly indicate a causal relationship between excessive or maintained accumulation of pro-inflammatory cells and cytokines and the establishment of particle-induced chronic diseases.
Current treatment modalities for treating patients developing particle-related diseases have been based on the assumption that these disorders are exclusively related to chronic inflammation diseases. However, it is becoming evident that most available anti-inflammatory drugs (34, 35) or cytokine inhibitor therapy (36) are not effective for particle-exposed patients. Human validation studies have also failed to confirm the use of inflammatory mediators as biomarkers for univocally detecting the health effects of particles (37).
Besides the strong evidence of a major role of inflammation in particle-treated animals, several experimental studies did not find an evident association between inflammatory responses and experimental diseases and, thus, have also challenged this inflammatory dogma. Several studies, conducted in mice, reported that exacerbated lung inflammation is not always accompanied by a subsequent increase of fibrosis after silica or carbon nanotubes (CNT) treatment (38, 39). Inversely, limited inflammation can be associated to robust silica-induced long-term lung responses (40). More precisely, it has been found that a progressive neutrophilic inflammatory process and a sustained release of pro-inflammatory cytokines did not accompany instillation of silica or CNT in mice. In this case, a mild and non-progressive pulmonary inflammation and a transient production of pro-inflammatory cytokines such TNF-α and IL-1β was instead observed (22, 41–43). Additionally, these inflammatory components were dispensable for the development of particles-induced fibrogenesis since genetic deficiencies of these master pro-inflammatory cytokines did not completely limit lung fibrosis while they were efficient to abrogate inflammation and granuloma formation after silica or CNT (22, 44–46). Other key pro-inflammatory cytokines such as type I interferons, IL-12, IL-17, and IL-22 were also dispensable in the development of silica-induced fibrosis (21, 22, 47). The non-requirement of inflammation during experimental lung fibrosis was further supported by the fact that different treatments with anti-inflammatory molecules such as steroids, cox- or phosphodiesterase 5-inhibitors strongly reduced inflammation without modifying, however, collagen deposition (42, 43). The disconnection between inflammation and particle toxicity is not limited to fibrosis. In mice, co-exposure to silica and carcinogens from tobacco smoke resulted in rapid tumor growth in the lungs that is attenuated in the absence of leukotriene B4 axis. This mechanism is, however, independent of IL-1 activation, TNF-α production and chemokine release (48). In addition, early inflammatory reactions triggered by asbestos required NLRP3 inflammasome activation and IL-1β release, but this pathway was not critical in the chronic development of asbestos-induced mesothelioma (49). Recent investigations additionally showed that non-steroidal anti-inflammatory molecules do not reduce progression of asbestos-induced mesothelioma in animals (35, 50).
Altogether, these results strongly suggest that other pathophysiological mechanisms unrelated to inflammation are operational after particle exposure. There are now emerging studies from the literature that explain why diseases achieved by particles are not systematically related to inflammation. This review covers existing evidence supporting that immunosuppression represents an alternative pathological pathway in the development of particle-induced disorders. The most relevant studies delineating the immunosuppressive properties of (nano)particles are depicted in the following sections and commented in Table 1.
Table 1. Historical progression of immunosuppression in particle toxicology literature: Summary of the most relevant studies supporting immunosuppressive properties of (nano) particles.
Indubitably, TGF-β is associated with the development of responses to particles in human and animal. Elevated TGF-β expression has been observed in patients with asbestosis or silicosis (67–71). In a variety of animal models, TGF-β activation has been demonstrated following exposure to silica, asbestos, or CNT (57, 63, 72, 73). After particles exposure, TGF-β contributes to fibroproliferative reorganization of organ tissue by orchestrating myofibroblast differentiation, collagen overproduction, and scar formation (74, 75). Previous studies have demonstrated that treatment with TGF-β inhibitors decreased lung fibrosis in animals exposed to silica (76–78). Additionally, TGF-β contributes to generate a favorable microenvironment for tumor growth and metastasis in mesothelioma, assigning this cytokine as dominant during the development of cancer induced by particles (79). It is noteworthy that the main function of TGF-β is immunosuppression since TGF-β1-deficient mice die from uncontrolled systemic inflammation and autoreactive T lymphocyte accumulation (80). The pivotal role of TGF-β argues for the development of other mechanisms than inflammation after particle exposure and supports that master immunosuppressive cytokines are also linked to particle-related diseases.
Because the anti-inflammatory cytokine IL-10 was effective in the regulation of inflammation, several authors have suggested that this cytokine could also be active in controlling inflammation in the early and late responses to mineral particles and thus plays a beneficial role based on the inflammatory paradigm (81–83). However, several lines of evidence have demonstrated that IL-10 (as TGF-β) possesses deleterious and pro-fibrotic functions after particle exposure. First, lung expression of IL-10 was intimately associated to the development of particle-induced fibrosis and cancer and could explain the absence of chronic and progressive inflammation in human and mouse models (37, 53, 57, 84–88). Additional data supports that IL-10 participates in the extension of fibrosis since targeted overexpression of IL-10 in the murine airways caused, by itself, collagen deposition, accumulation of mesenchymal cells and airway remodeling with fibrosis (89, 90). Subsequently, it has been demonstrated that IL-10 limits inflammatory response but promotes fibrotic reaction. Indeed, mice deficient for IL-10 developed exaggerated acute inflammation but limited lung fibrosis in response to silica (51, 91). Conversely, overexpression of IL-10 in the lung, by using an adenovirus coding for this cytokine, limited the inflammatory process induced by silica, but increased the fibrotic response (92). It is now admitted that this potent immunosuppressive cytokine has direct effects on fibroblasts and matrix protein release (93). In addition, IL-10 induces the expression of pro-fibrotic mediators (i.e., TGF-β) by macrophages and limits the synthesis of anti-fibrotic mediators (i.e., prostaglandin E2) by epithelial cells (91). The deleterious effect of IL-10 is not limited to fibrosis development but is also implicated in the carcinogenic process resulting from particles. Recent results showed that asbestos exposure restricts effector T lymphocyte activation and impairs anti-tumor immunity through IL-10 release (69, 94). IL-10-expressing macrophages increased mesothelioma cell proliferation and resistance to treatment (85, 95). Collectively, these data additionally challenge the concept that only pro-inflammatory mediators and inflammation lead to fibrosis and cancer and conclusively demonstrate a key role of persistent immunosuppressive mediators in the development of particle-related disorders in the absence of inflammation (Figure 2).
Figure 2. Pathological functions of persistent immunosuppressive cells and mediators during long-term responses to particles. Unresolved Immunosuppression (in blue) represents an alternative event during the responses to particles. According to this new pathological pathway, fibrogenesis, and carcinogenesis are governed by a persistent accumulation of immunosuppressive myeloid (M2 and MDSC) and lymphoid (T and B regs) cells and a sustained production of their related cytokines (IL-10 and TGF-β). These immunoregulatory components limit both the recruitment of inflammatory cells and the activity of pro-inflammatory mediators (in green). The high amount of immunosuppressive cytokines produced can, in addition to their anti-inflammatory action, also act as profibrotic mediators, conceivably by stimulating mesenchymal cells to overproduce collagenase inhibitors and ultimately matrix elements under non-inflammatory conditions. The persistence of immunosuppressive cells and mediators is also incriminated in carcinogenesis and infection by preventing host immune responses directed against transformed cells and microorganisms.
Based on the immunosuppressive profile of silica-treated mice, the role of regulatory T lymphocytes (T regs) in the development of lung responses to particles was investigated. This specialized thymus-derived sub-population of Th lymphocytes acts to suppress immune responses, thereby maintaining homeostasis and self-tolerance. They mediate immunosuppression by producing the immunoregulatory cytokines IL-10 and TGF-β (96). Recent results indicated that T regs are progressively and specifically accumulated in lung tissue of mice treated with silica where they promote pulmonary immunosuppression and fibrosis (58, 60) (Figure 2). This hallmark of immunological tolerance and immunosuppressive environment was observed with other particles, detected locally and systemically, and induced by the Wnt/B-catenin pathway (57, 59, 97, 98). Lung T regs directly stimulated fibroblast proliferation and collagen deposition after transfer in non-treated mice (58). The direct profibrotic effects of T regs were reduced by inhibiting the PDGF-B/TGF-β signaling pathway, demonstrating that T regs increase tissue fibroblast numbers, and consequently, amplify collagen deposition. In vitro and in vivo studies additionally showed that mesotheliomagenic fibers such as asbestos directly enhance the immunosuppressive function of T regs and their capacity to release TGF-β and IL-10 (61, 94). In addition, the marked presence of T regs have been detected during lung carcinogenesis after silica or asbestos exposure (99, 100). From these last studies, it has been concluded that the effect of particles on T regs accumulation and function induces a maintained tolerant microenvironment counteracting anti-tumor host innate and adaptive immunity and favoring tumoral cell evasion and the occurrence of particle-induced tumors.
More recently, Chen and colleagues have identified an additional immunosuppressive subpopulation of B lymphocytes recruited in response to silica in human and animal (37, 101) (Figure 2). Regulatory B-lymphocytes (B regs) are immunosuppressive cells releasing IL-10 and TGF-β that support immunological tolerance and suppress immunopathology by limiting the expansion of inflammatory T cells. B regs are defined as the immunosuppressive counterpart of antibody-producing B2 lymphocytes (or conventional B cells) (102). The regulatory B-cell subpopulation has been reported to be recruited to the tumor aggregates and thereby promotes carcinogenesis by attenuating anti-tumor immune responses (103). Their pro-fibrotic functions have been attributed to their capacity to release IL-10 in silica-treated mice (101). As observed in IL-10-deficient mice, reduction of fibrosis in B reg-depleted mice was associated with an increased neutrophilic inflammation (101). Interestingly, IL-10-producing B regs convert T eff into T regs thus increasing immunological tolerance after particle exposure (104). The observation that immunosuppressive T and B-lymphocytes persistently populate damaged tissue strongly suggests that these cells are crucial immune components explaining the development of fibrotic and carcinogenic responses to particles in absence of substantial inflammation.
Infiltrated macrophages in cancer or asthma acquire a particular phenotype called M2, which provides an immunosuppressive microenvironment for tumor growth and allergic tolerance (105, 106). Recent studies also revealed a preferential engagement of immunosuppressive M2 macrophages after inhalation of particles (Figure 2). There is an increased predominance of M2-polarized macrophages in patients developing asbestosis (53). In animal, M2-related mediators such as Arginase 1, crystallizable protein YM-1 or IL-10 were all increased in response to reactive particles such as silica, ultrafine amorphous silica and CNT, appointing M2-polarized macrophages as pivotal (53, 65, 66, 107–109). Authors have recently incriminated oxidized phospholipids, IL-4 or IL-13 in the polarization of M2 macrophages that display enhanced production of TGF-β. Evidence also showed that this last cytokine explains the deleterious activities of M2 macrophages after particle treatment (43, 53, 110–112). These results therefore argue that the continuous accumulation of M2 macrophages and their products, initially for counteracting the effects of inflammatory M1 macrophages, participates in the establishment of the fibrogenic and mesotheliomagenic lesions generated by particles.
Myeloid-derived suppressor cells (MDSC) represent a heterogeneous population of immature neutrophilic and monocytic myeloid cells that displays potent immunosuppressive activities and inhibits immune effector cell functions in diverse chronic pathologies such as cancer (113). The accumulation of immature and immunosuppressive myeloid cells is also a central event during dust-induced fibrosis and cancer with predominant immunosuppression (Figure 2). Indeed, recent investigations have elegantly demonstrated that CNT or asbestos exposure induces a robust accumulation of MDSC that actively release TGF-β and promote cancer progression in mice (62, 64, 114). Fibrotic responses to silica also comprise an accumulation of monocytic MDSC that possess the intrinsic capacity to promote release of the collagenase inhibitor TIMP-1 by fibroblasts. The subsequent elaboration of a non-degrading matrix environment was attributed to monocytic MDSC-derived TGF-β (63). These observations newly indicate that immunoregulatory M2 macrophages and MDSC, along with T and B regs, are implicated in fibrogenesis and carcinogenesis, especially when these cells are continuously accumulated and when inflammatory activity is limited (Figure 2).
The emerging concept that particles orchestrate the establishment of immunosuppressive responses is consolidated by several studies exploring the impact of nanoparticles on the immune system. As mentioned above for micrometric particles, the major concern in the field of nanotoxicology is historically related toward the capacity of nanoparticles to induce inflammation and immunostimulation (115). Nevertheless, this exclusive view has been revisited and recent attention has been given to anti-inflammatory and immunosuppressive properties of these particles (55). There is, indeed, a growing body of evidence showing that nanoparticles also display strong immunosuppressive effects (56). In 2007, Ryan and colleagues were the first to report that fullerene nanoparticles prevented mast-cell related diseases such as asthma, arthritis, and sclerosis (54). Other pioneer studies unexpectedly discovered that dendrimer nanoparticles improved disease scores in animal models of granulomatous, autoimmune, and edema diseases (116). Another example illustrating the immunosuppressive properties of nanoparticles is the study by Rajan (117). Liposomal nanoparticles possess the ability to elicit an immunosuppressive cell environment that subsequently suppress anti-tumor immunity and favor tumor progression.
The particles inducing immunosuppression mainly include metal (e.g., gold, silver, iron oxide, cerium oxide, and zinc oxide) and carbon (CNT, fullerenes) nanoparticles (56). The immunosuppressive effects of nanoparticles also depend on their physicochemical properties. For instance, small nanoparticles are more immunosuppressive than large particles (116, 118). The exact physicochemical determinants accountable for nanoparticle-induced immunosuppressive activities remain, however, unclear and require additional investigations. There is also a crucial need to determine whether molecules enrobing particles (e.g., LPS) are important for the immunosuppressive activities of (nano)particles (115).
Interestingly, research in nanotoxicology elucidated different molecular mechanisms explaining how particles promote immunosuppression. These mechanisms involve direct interference of nanoparticles with TLR-, NFκB-, or STAT1-signaling pathways (55, 119). Iron nanoparticles specifically alter IL-1β processing by preventing inflammasome assembly (120). A direct inhibitory interaction between gold nanoparticles and inflammatory cytokines have been also observed from the study of Sumbayev (118). Carbon and cerium nanomaterials exhibit potent free radical-scavenger properties and attenuate oxidant molecule production by particle-activated immune cells. These findings confer to these nanoparticles important antioxidant properties that suppress oxidative stress and inflammatory responses in vitro and in vivo (119, 121, 122).
Nanoparticles strongly and directly polarize the immune system and establish a preferentially immunosuppressive environment. CNT induce the expression of TGF-β and IL-10 (57) as observed for micrometric silica (see above). Nanoparticles also facilitate polarization of immunosuppressive T regs and anti-inflammatory Th2 lymphocytes (98, 123, 124). Mechanistic studies investigating the effects of nanoparticles on T-lymphocyte immunosuppressive lineage commitment revealed that nanoparticles (carbon, grapheme, and iron) directly interfere with autophagy, ROS production, NFκB-nuclear translocation or antigen processing by dendritic cells (124–126). While nanoparticles mainly operated through DC and T cells, they additionally target macrophages and myeloid cells. Liposomes reduced inflammatory functions of macrophages and increased anti-inflammatory mediator expression in myeloid cells (117).
The exact adverse effects of nanoparticle-induced unresolved immunosuppression are not yet delineated but fibrosis development caused by CNT or cerium has been linked to the immunosuppressive cytokines TGF-β and IL-10 (52, 72, 127, 128). Altered host resistance to infection and cancer represents another possible pathophysiological consequence of maintained immunosuppression. Administration of titanium oxide nanoparticles or liposomes inhibited lymphocyte- and macrophage-related immune responses and increased susceptibility to tumor growth (117, 129). Augmented viral and bacterial infections have also been observed after exposure to carbon nanoparticles (130, 131).
Taken together, the studies described above and documented in Table 1 support an additional and alternative pathological pathway, which is operative during tissue responses to particles. This new paradigm proposes that fibrogenesis, carcinogenesis, and infections can develop from persistent immunosuppressive developments induced by micro- or nano-particles under non-inflammatory conditions (Figure 3, below). Unresolved immunosuppression participates in the development of fibrosis by activating profibrotic factor release and inhibiting matrix degradation. The immunosuppressive milieu can also favor cancer and infection progression by facilitating tumor and microbial escape from immune surveillance.
Figure 3. Mutual roles of inflammatory and immunosuppressive responses in particle-induced chronic diseases. The classical scenario explaining the emergence of autoimmune diseases, fibrosis, and cancer after dust exposure mainly relies on predominant and persistent inflammation (blue). Inflammatory responses directly promote fibroblast growth and uncontrolled matrix deposition, trigger genomic instability, and cell transformation, and support autoantibody and immune complex production. An additional paradigm is now offered and comprises predominant and unresolved immunosuppression in response to particles (red). This pathway relies upon a sustained accumulation of immunosuppressive components that include mediators implicated in fibroblast activation, tumor cell and microbe evasion. The exclusive or simultaneous presence of immunoregulatory and immunostimulatory mechanisms can explain the diversity of immune responses and pathologies existing in exposed human or animal.
This new pathological avenue does not overshadow the relevance of the inflammatory scenario. Indeed, the compelling findings accumulated over the years indubitably support that uncontrolled fibroblast growth and genomic instability rely upon predominant inflammation, especially when immunosuppressive activity is lacking (Figure 3, above). The emergence of immune complex deposition and autoimmune disorders is additionally associated to persistent inflammation in animal or human exposed to particles. The fact that particle-related autoimmune diseases are not mechanistically covered by sustained immunosuppression indicates that inflammation remains a crucial event in response to particles. Importantly, the inflammatory process governs the early accumulation of immunosuppressive cells and mediators by activating several anti-inflammatory signaling pathways (e.g., IL-10) mediated predominantly by NFκB (132). This notion indicates that inflammation can predispose to a developing immunosuppressive environment and that inflammation and immunosuppression are functionally linked.
Altogether, these observations clearly argue for a more complicated mechanism in particle toxicology, wherein both types of immune responses (persistent inflammation and immunosuppression) could promote chronic diseases after particle inhalation. The mutual roles of inflammation and immunosuppression well explain the diversity of particle-induced immune polarization and pathologies (Figure 3). Interestingly, these apparently opposite processes are not systematically exclusive and may be, in contrast, concomitant. The view that uncontrolled immunoregulatory and immunostimulatory responses can co-exist is supported by recent human and animal studies showing mixed inflammatory and immunosuppressive elements (e.g., elevated levels of TNF-α, IL-1β, IL-10, and TGF-β) during fibrogenesis and carcinogenesis induced by particles (6, 133, 134).
It remains, however, to learn how to extrapolate and how to benefit from these observations in predictive toxicology and translational medicine. Current bioassays testing particle reactivity are exclusively based on the appreciation of inflammatory components and suffer from a lack of specificity (135, 136). These tests could be enriched by considering the expression of immunoregulatory mediators or signatures. For instance, release of TGF-β and IL-10 and subsequent abrogation of IL-1 and TNF-α production by treated macrophages could be used to identify reactive particles as proposed by the team of Boraschi and colleagues (137). Furthermore, blockade of inflammasome machinery or antigen-presenting capacity of macrophages and DC could be considered as clear sign of immunosuppressive responses. Inhibition of inflammatory pathologies in animal models could also reflect ability of particles to trigger immunosuppression (56).
In recent years, the increase of anti-tumor responses obtained in clinic trials by blocking the immunosuppressive environment of tumors have revolutionized cancer treatment. Monoclonal antibody-based immunotherapies targeting TGF-β and IL-10 or T cell immune checkpoint receptors (PD-1 or CTLA4) are now available and confer long-term benefit for patients (138–140). Additional experimental and clinical studies are necessary to determine whether this therapeutic option inhibiting immunosuppressive responses is also promising for the treatment of patients affected by particle-associated disorders.
The existence of at least two different pathogenic routes may explain why existing therapies are controversial and disappointing with a strong differential effect in specific subgroups of patients (141, 142). The opportunity to distinguish the prevailing immune response or profile (inflammation and immunosuppression) existing in patients should also help the clinician to categorize patients, assess disease progression and select the most appropriate therapy to inhibit key effector cells and mediators of inflammation (steroids) and/or immunosuppression (immunotherapy). This possibility has been already observed in available animal models. Silica-induced lung fibrosis in rats (associated with chronic inflammation) can be reverted by anti-inflammatory treatment while in mice (developing preferentially unresolved immunosuppressive responses), silicosis is resistant to anti-inflammatory therapy but prevented by T regs neutralization (43, 60). The enthusiasm for the discovery of novel drug targets to treat particle-related diseases should be tempered as inflammation and immunosuppression are extremely imbricated with each other, leading to the fact that inhibition of one response may be compensated by the extension of the other (22, 58).
The author confirms being the sole contributor of this work and has approved it for publication.
This work was funded by the Actions de Recherche Concertées, Fédération Wallonie-Bruxelles (ARC 09/14-021 and 14/19-056), the Fondation Contre le Cancer (2012-219, 2014-148, and 150), the Fonds de la Recherche Scientifique (FNRS; Projects PDR T.0119.13 and CDR J0049.16). FH is a Senior Research Associate with the FNRS, Belgium.
The author declares that the research was conducted in the absence of any commercial or financial relationships that could be construed as a potential conflict of interest.
Regulatory T or B lymphocytes, T or B regs; Myeloid Derived Suppressive Cells, MDSC; Alternatively differentiated macrophages, M2 macrophages; CD4+ T helper lymphocytes, Th; gamma and delta TCR chain positive T lymphocytes, γδ T cells; Interferon, IFN; Effector T lymphocytes, T eff; Carbon nanotubes, CNT.
1. Sayan M, Mossman BT. The NLRP3 inflammasome in pathogenic particle and fibre-associated lung inflammation and diseases. Part Fibre Toxicol. (2016) 13:51. doi: 10.1186/s12989-016-0162-4
2. Hoy RF, Brims F. Occupational lung diseases in Australia. Med J Aust. (2017) 207:443–8. doi: 10.5694/mja17.00601
3. Hoet P, Desvallees L, Lison D. Do current OELs for silica protect from obstructive lung impairment? A critical review of epidemiological data. Crit Rev Toxicol. (2017) 47:650–77. doi: 10.1080/10408444.2017.1315363
4. Lee S, Hayashi H, Mastuzaki H, Kumagai-Takei N, Otsuki T. Silicosis and autoimmunity. Curr Opin Allergy Clin Immunol. (2017) 17:78–84. doi: 10.1097/ACI.0000000000000350
5. Hoy RF, Baird T, Hammerschlag G, Hart D, Johnson AR, King P, et al. Artificial stone-associated silicosis: a rapidly emerging occupational lung disease. Occup Environ Med. (2018) 75:3–5. doi: 10.1136/oemed-2017-104428
6. Anlar HG, Bacanli M, Iritas S, Bal C, Kurt T, Tutkun E, et al. Effects of occupational silica exposure on OXIDATIVE stress and immune system parameters in ceramic workers in TURKEY. J Toxicol Environ Health A (2017) 80:688–96. doi: 10.1080/15287394.2017.1286923
7. Akgun M. Denim production and silicosis. Curr Opin Pulm Med. (2016) 22:165–9. doi: 10.1097/MCP.0000000000000249
8. Leung CC, Yu IT, Chen W. Silicosis. Lancet (2012) 379:2008–18. doi: 10.1016/S0140-6736(12)60235-9
9. Ou L, Song B, Liang H, Liu J, Feng X, Deng B, et al. Toxicity of graphene-family nanoparticles: a general review of the origins and mechanisms. Part Fibre Toxicol. (2016) 13:57. doi: 10.1186/s12989-016-0168-y
10. Pollard KM. Silica, silicosis, and autoimmunity. Front Immunol. (2016) 7:97. doi: 10.3389/fimmu.2016.00097
11. Rabolli V, Lison D, Huaux F. The complex cascade of cellular events governing inflammasome activation and IL-1β processing in response to inhaled particles. Part Fibre Toxicol. (2016) 13:40. doi: 10.1186/s12989-016-0150-8
12. Nakayama M. Macrophage recognition of crystals and nanoparticles. Front Immunol. (2018) 9:103. doi: 10.3389/fimmu.2018.00103
13. Kadariya Y, Menges CW, Talarchek J, Cai KQ, Klein-Szanto AJ, Pietrofesa RA, et al. Inflammation-related IL1β/IL1R signaling promotes the development of asbestos-induced malignant mesothelioma. Cancer Prev Res (Phila) (2016) 9:406–14. doi: 10.1158/1940-6207.CAPR-15-0347
14. Hegde B, Bodduluri SR, Satpathy SR, Alghsham RS, Jala VR, Uriarte SM, et al. Inflammasome-independent leukotriene B4 production drives crystalline silica-induced sterile inflammation. J Immunol. (2018) 200:3556–67. doi: 10.4049/jimmunol.1701504
15. Chen J, Yao Y, Su X, Shi Y, Song X, Xie L, et al. Comparative RNA-Seq transcriptome analysis on silica induced pulmonary inflammation and fibrosis in mice silicosis model. J Appl Toxicol. (2018) 38:773–82. doi: 10.1002/jat.3587
16. Alsaleh NB, Brown JM. Immune responses to engineered nanomaterials: current understanding and challenges. Curr Opin Toxicol. (2018) 10:8–14. doi: 10.1016/j.cotox.2017.11.011
17. Hiraku Y, Kawanishi S, Ichinose T, Murata M. The role of iNOS-mediated DNA damage in infection- and asbestos-induced carcinogenesis. Ann N Y Acad Sci. (2010) 1203:15–22. doi: 10.1111/j.1749-6632.2010.05602.x
18. Mossman BT, Shukla A, Heintz NH, Verschraegen CF, Thomas A, Hassan R. New insights into understanding the mechanisms, pathogenesis, and management of malignant mesotheliomas. Am J Pathol. (2013) 182:1065–77. doi: 10.1016/j.ajpath.2012.12.028
19. Winkler HC, Kornprobst J, Wick P, von Moos LM, Trantakis I, Schraner EM, et al. MyD88-dependent pro-interleukin-1β induction in dendritic cells exposed to food-grade synthetic amorphous silica. Part Fibre Toxicol. (2017) 14:21. doi: 10.1186/s12989-017-0202-8
20. Thakur C, Wolfarth M, Sun J, Zhang Y, Lu Y, Battelli L, et al. Oncoprotein mdig contributes to silica-induced pulmonary fibrosis by altering balance between Th17 and Treg T cells. Oncotarget (2015) 6:3722–36. doi: 10.18632/oncotarget.2914
21. Lo Re S, Dumoutier L, Couillin I, Van Vyve C, Yakoub Y, Uwambayinema F, et al. IL-17A-producing gammadelta T and Th17 lymphocytes mediate lung inflammation but not fibrosis in experimental silicosis. J Immunol. (2010) 184:6367–77. doi: 10.4049/jimmunol.0900459
22. Re SL, Giordano G, Yakoub Y, Devosse R, Uwambayinema F, Couillin I, et al. Uncoupling between inflammatory and fibrotic responses to silica: evidence from MyD88 knockout mice. PLoS ONE (2014) 9:e99383. doi: 10.1371/journal.pone.0099383
23. Huaux F, Lo Re S, Giordano G, Uwambayinema F, Devosse R, Yakoub Y, et al. IL-1alpha induces CD11b(low) alveolar macrophage proliferation and maturation during granuloma formation. J Pathol. (2015) 235:698–709. doi: 10.1002/path.4487
24. Vietti G, Lison D, van den Brule S. Mechanisms of lung fibrosis induced by carbon nanotubes: towards an Adverse Outcome Pathway (AOP). Part Fibre Toxicol. (2016) 13:11. doi: 10.1186/s12989-016-0123-y
25. Zheng H, Hogberg J, Stenius U. ATM-activated autotaxin (ATX) propagates inflammation and DNA damage in lung epithelial cells: a new mode of action for silica-induced DNA damage? Carcinogenesis (2017) 38:1196–206. doi: 10.1093/carcin/bgx100
26. Ferro A, Zebedeo CN, Davis C, Ng KW, Pfau JC. Amphibole, but not chrysotile, asbestos induces anti-nuclear autoantibodies and IL-17 in C57BL/6 mice. J Immunotoxicol. (2014) 11:283–90. doi: 10.3109/1547691X.2013.847510
27. Zebedeo CN, Davis C, Pena C, Ng KW, Pfau JC. Erionite induces production of autoantibodies and IL-17 in C57BL/6 mice. Toxicol Appl Pharmacol. (2014) 275:257–64. doi: 10.1016/j.taap.2014.01.018
28. Bates MA, Brandenberger C, Langohr I, Kumagai K, Harkema JR, Holian A, et al. Silica triggers inflammation and ectopic lymphoid neogenesis in the lungs in parallel with accelerated onset of systemic autoimmunity and glomerulonephritis in the lupus-prone NZBWF1 mouse. PLoS ONE (2015) 10:e0125481. doi: 10.1371/journal.pone.0125481
29. Biswas R, Trout KL, Jessop F, Harkema JR, Holian A. Imipramine blocks acute silicosis in a mouse model. Part Fibre Toxicol. (2017) 14:36. doi: 10.1186/s12989-017-0217-1
30. Miao R, Ding B, Zhang Y, Xia Q, Li Y, Zhu B. Proteomic profiling change during the early development of silicosis disease. J Thorac Dis. (2016) 8:329–41. doi: 10.21037/jtd.2016.02.46
31. Comar M, Zanotta N, Bonotti A, Tognon M, Negro C, Cristaudo A, et al. Increased levels of C-C chemokine RANTES in asbestos exposed workers and in malignant mesothelioma patients from an hyperendemic area. PLoS ONE (2014) 9:e104848. doi: 10.1371/journal.pone.0104848
32. Polatli M, Tuna HT, Yenisey C, Serter M, Cildag O. Lung function and IFN-gamma levels in the sera of silica-exposed workers. J Interferon Cytokine Res. (2008) 28:311–6. doi: 10.1089/jir.2007.0093
33. Robinson BW, Rose AH, Hayes A, Musk AW. Increased pulmonary gamma interferon production in asbestosis. Am Rev Respir Dis. (1988) 138:278–83. doi: 10.1164/ajrccm/138.2.278
34. Greenberg MI, Waksman J, Curtis J. Silicosis: a review. Dis Mon. (2007) 53:394–416. doi: 10.1016/j.disamonth.2007.09.020
35. Robinson C, Alfonso H, Woo S, Olsen N, Bill Musk AW, Robinson BW, et al. Effect of NSAIDS and COX-2 inhibitors on the incidence and severity of asbestos-induced malignant mesothelioma: evidence from an animal model and a human cohort. Lung Cancer (2014) 86:29–34. doi: 10.1016/j.lungcan.2014.08.005
36. Baird T, Putt M, Dettrick A. A stonemason with accelerated silicosis in the setting of tumour necrosis factor alpha inhibitor therapy. Respirol Case Rep. (2016) 4:e00171. doi: 10.1002/rcr2.171
37. Chen Y, Li C, Lu Y, Zhuang H, Gu W, Liu B, et al. IL-10-Producing CD1dhiCD5+ regulatory B cells may play a critical role in modulating immune homeostasis in silicosis patients. Front Immunol. (2017) 8:110. doi: 10.3389/fimmu.2017.00110
38. Beamer CA, Seaver BP, Shepherd DM. Aryl hydrocarbon receptor (AhR) regulates silica-induced inflammation but not fibrosis. Toxicol Sci. (2012) 126:554–68. doi: 10.1093/toxsci/kfs024
39. Shvedova AA, Kisin ER, Murray AR, Kommineni C, Castranova V, Fadeel B, et al. Increased accumulation of neutrophils and decreased fibrosis in the lung of NADPH oxidase-deficient C57BL/6 mice exposed to carbon nanotubes. Toxicol Appl Pharmacol. (2008) 231:235–40. doi: 10.1016/j.taap.2008.04.018
40. Brass DM, McGee SP, Dunkel MK, Reilly SM, Tobolewski JM, Sabo-Attwood T, et al. Gender influences the response to experimental silica-induced lung fibrosis in mice. Am J Physiol Lung Cell Mol Physiol. (2010) 299:L664–71. doi: 10.1152/ajplung.00389.2009
41. Dong J, Porter DW, Batteli LA, Wolfarth MG, Richardson DL, Ma Q. Pathologic and molecular profiling of rapid-onset fibrosis and inflammation induced by multi-walled carbon nanotubes. Arch Toxicol. (2015) 89:621–33. doi: 10.1007/s00204-014-1428-y
42. Rabolli V, Lo Re S, Uwambayinema F, Yakoub Y, Lison D, Huaux F. Lung fibrosis induced by crystalline silica particles is uncoupled from lung inflammation in NMRI mice. Toxicol Lett. (2011) 203:127–34. doi: 10.1016/j.toxlet.2011.03.009
43. Barbarin V, Nihoul A, Misson P, Arras M, Delos M, Leclercq I, et al. The role of pro- and anti-inflammatory responses in silica-induced lung fibrosis. Respir Res. (2005) 6:112. doi: 10.1186/1465-9921-6-112
44. Rydman EM, Ilves M, Vanhala E, Vippola M, Lehto M, Kinaret PA, et al. A single aspiration of rod-like carbon nanotubes induces asbestos-like pulmonary inflammation mediated in part by the IL-1 receptor. Toxicol Sci. (2015) 147:140–55. doi: 10.1093/toxsci/kfv112
45. Song L, Weng D, Dai W, Tang W, Chen S, Li C, et al. Th17 can regulate silica-induced lung inflammation through an IL-1β-dependent mechanism. J Cell Mol Med. (2014) 18:1773–84. doi: 10.1111/jcmm.12341
46. Guo J, Gu N, Chen J, Shi T, Zhou Y, Rong Y, et al. Neutralization of interleukin-1 beta attenuates silica-induced lung inflammation and fibrosis in C57BL/6 mice. Arch Toxicol. (2013) 87:1963–73. doi: 10.1007/s00204-013-1063-z
47. Davis GS, Pfeiffer LM, Hemenway DR, Rincon M. Interleukin-12 is not essential for silicosis in mice. Part Fibre Toxicol. (2006) 3:2. doi: 10.1186/1743-8977-3-2
48. Satpathy SR, Jala VR, Bodduluri SR, Krishnan E, Hegde B, Hoyle GW, et al. Crystalline silica-induced leukotriene B4-dependent inflammation promotes lung tumour growth. Nat Commun. (2015) 6:7064. doi: 10.1038/ncomms8064
49. Chow MT, Tschopp J, Moller A, Smyth MJ. NLRP3 promotes inflammation-induced skin cancer but is dispensable for asbestos-induced mesothelioma. Immunol Cell Biol. (2012) 90:983–6. doi: 10.1038/icb.2012.46
50. Robinson C, Walsh A, Larma I, O'Halloran S, Nowak AK, Lake RA. MexTAg mice exposed to asbestos develop cancer that faithfully replicates key features of the pathogenesis of human mesothelioma. Eur J Cancer (2011) 47:151–61. doi: 10.1016/j.ejca.2010.08.015
51. Huaux F, Louahed J, Hudspith B, Meredith C, Delos M, Renauld JC, et al. Role of interleukin-10 in the lung response to silica in mice. Am J Respir Cell Mol Biol. (1998) 18:51–9. doi: 10.1165/ajrcmb.18.1.2911
52. Park EJ, Roh J, Kim SN, Kang MS, Han YA, Kim Y, et al. A single intratracheal instillation of single-walled carbon nanotubes induced early lung fibrosis and subchronic tissue damage in mice. Arch Toxicol. (2011) 85:1121–31. doi: 10.1007/s00204-011-0655-8
53. Murthy S, Larson-Casey JL, Ryan AJ, He C, Kobzik L, Carter AB. Alternative activation of macrophages and pulmonary fibrosis are modulated by scavenger receptor, macrophage receptor with collagenous structure. FASEB J. (2015) 29:3527–36. doi: 10.1096/fj.15-271304
54. Ryan JJ, Bateman HR, Stover A, Gomez G, Norton SK, Zhao W, et al. Fullerene nanomaterials inhibit the allergic response. J Immunol. (2007) 179:665–72. doi: 10.4049/jimmunol.179.1.665
55. Ilinskaya AN, Dobrovolskaia MA. Immunosuppressive and anti-inflammatory properties of engineered nanomaterials. Br J Pharmacol. (2014) 171:3988–4000. doi: 10.1111/bph.12722
56. Ngobili TA, Daniele MA. Nanoparticles and direct immunosuppression. Exp Biol Med (Maywood) (2016) 241:1064–73. doi: 10.1177/1535370216650053
57. Mitchell LA, Lauer FT, Burchiel SW, McDonald JD. Mechanisms for how inhaled multiwalled carbon nanotubes suppress systemic immune function in mice. Nat Nanotechnol. (2009) 4:451–6. doi: 10.1038/nnano.2009.151
58. Lo Re S, Lecocq M, Uwambayinema F, Yakoub Y, Delos M, Demoulin JB, et al. Platelet-derived growth factor-producing CD4+ Foxp3+ regulatory T lymphocytes promote lung fibrosis. Am J Respir Crit Care Med. (2011) 184:1270–81. doi: 10.1164/rccm.201103-0516OC
59. Hess KL, Oh E, Tostanoski LH, Andorko JI, Susumu K, Deschamps JR, et al. Engineering immunological tolerance using quantum dots to tune the density of self-antigen display. Adv Funct Mater. (2017) 27:1700290. doi: 10.1002/adfm.201700290
60. Liu F, Liu J, Weng D, Chen Y, Song L, He Q, et al. CD4+CD25+Foxp3+ regulatory T cells depletion may attenuate the development of silica-induced lung fibrosis in mice. PLoS ONE (2010) 5:e15404. doi: 10.1371/journal.pone.0015404
61. Ying C, Maeda M, Nishimura Y, Kumagai-Takei N, Hayashi H, Matsuzaki H, et al. Enhancement of regulatory T cell-like suppressive function in MT-2 by long-term and low-dose exposure to asbestos. Toxicology (2015) 338:86–94. doi: 10.1016/j.tox.2015.10.005
62. Shvedova AA, Tkach AV, Kisin ER, Khaliullin T, Stanley S, Gutkin DW, et al. Carbon nanotubes enhance metastatic growth of lung carcinoma via up-regulation of myeloid-derived suppressor cells. Small (2013) 9:1691–5. doi: 10.1002/smll.201201470
63. Lebrun A, Lo Re S, Chantry M, Izquierdo Carerra X, Uwambayinema F, Ricci D, et al. CCR2+ monocytic myeloid-derived suppressor cells (M-MDSCs) inhibit collagen degradation and promote lung fibrosis by producing transforming growth factor-beta1. J Pathol. (2017) 243:320–30. doi: 10.1002/path.4956
64. Shvedova AA, Kisin ER, Yanamala N, Tkach AV, Gutkin DW, Star A, et al. MDSC and TGFbeta are required for facilitation of tumor growth in the lungs of mice exposed to carbon nanotubes. Cancer Res. (2015) 75:1615–23. doi: 10.1158/0008-5472.CAN-14-2376
65. Xiang GA, Zhang YD, Su CC, Ma YQ, Li YM, Zhou X, et al. Dynamic changes of mononuclear phagocytes in circulating, pulmonary alveolar and interstitial compartments in a mouse model of experimental silicosis. Inhal Toxicol. (2016) 28:393–402. doi: 10.1080/08958378.2016.1188186
66. Dong J, Ma Q. Macrophage polarization and activation at the interface of multi-walled carbon nanotube-induced pulmonary inflammation and fibrosis. Nanotoxicology (2018) 12:153–68. doi: 10.1080/17435390.2018.1425501
67. Helmig S, Belwe A, Schneider J. Association of transforming growth factor beta1 gene polymorphisms and asbestos-induced fibrosis and tumors. J Investig Med. (2009) 57:655–61. doi: 10.2310/JIM.0b013e3181a4f32a
68. Jagirdar J, Begin R, Dufresne A, Goswami S, Lee TC, Rom WN. Transforming growth factor-beta (TGF-beta) in silicosis. Am J Respir Crit Care Med. (1996) 154(4 Pt 1):1076–81. doi: 10.1164/ajrccm.154.4.8887610
69. Matsuzaki H, Kumagai-Takei N, Lee S, Maeda M, Sada N, Hatayama T, et al. Search for biomarkers of asbestos exposure and asbestos-induced cancers in investigations of the immunological effects of asbestos. Environ Health Prev Med. (2017) 22:53. doi: 10.1186/s12199-017-0661-4
70. Li Y, Karjalainen A, Koskinen H, Vainio H, Pukkala E, Hemminki K, et al. Serum growth factors in asbestosis patients. Biomarkers (2009) 14:61–6. doi: 10.1080/13547500802676868
71. Khalil N, O'Connor RN, Flanders KC, Unruh H. TGF-beta 1, but not TGF-beta 2 or TGF-beta 3, is differentially present in epithelial cells of advanced pulmonary fibrosis: an immunohistochemical study. Am J Respir Cell Mol Biol. (1996) 14:131–8. doi: 10.1165/ajrcmb.14.2.8630262
72. Dong J, Ma Q. Osteopontin enhances multi-walled carbon nanotube-triggered lung fibrosis by promoting TGF-beta1 activation and myofibroblast differentiation. Part Fibre Toxicol. (2017) 14:18. doi: 10.1186/s12989-017-0198-0
73. Polimeni M, Gulino GR, Gazzano E, Kopecka J, Marucco A, Fenoglio I, et al. Multi-walled carbon nanotubes directly induce epithelial-mesenchymal transition in human bronchial epithelial cells via the TGF-beta-mediated Akt/GSK-3beta/SNAIL-1 signalling pathway. Part Fibre Toxicol. (2016) 13:27. doi: 10.1186/s12989-016-0138-4
74. Fang Y, Zhang S, Li X, Jiang F, Ye Q, Ning W. Follistatin like-1 aggravates silica-induced mouse lung injury. Sci Rep. (2017) 7:399. doi: 10.1038/s41598-017-00478-0
75. Wang Z, Wang C, Liu S, He W, Wang L, Gan J, et al. Specifically formed corona on silica nanoparticles enhances transforming growth factor beta1 activity in triggering lung fibrosis. ACS Nano (2017) 11:1659–72. doi: 10.1021/acsnano.6b07461
76. Mi S, Li Z, Yang HZ, Liu H, Wang JP, Ma YG, et al. Blocking IL-17A promotes the resolution of pulmonary inflammation and fibrosis via TGF-beta1-dependent and -independent mechanisms. J Immunol. (2011) 187:3003–14. doi: 10.4049/jimmunol.1004081
77. Wang X, Dai W, Wang Y, Gu Q, Yang D, Zhang M. Blocking the Wnt/beta-catenin pathway by lentivirus-mediated short hairpin RNA targeting beta-catenin gene suppresses silica-induced lung fibrosis in mice. Int J Environ Res Public Health (2015) 12:10739–54. doi: 10.3390/ijerph120910739
78. Rong Y, Shen Y, Zhang Z, Cui X, Xiao L, Liu Y, et al. Blocking TGF-beta expression inhibits silica particle-induced epithelial-mesenchymal transition in human lung epithelial cells. Environ Toxicol Pharmacol. (2015) 40:861–9. doi: 10.1016/j.etap.2015.09.014
79. Neuzillet C, Tijeras-Raballand A, Cohen R, Cros J, Faivre S, Raymond E, et al. Targeting the TGFbeta pathway for cancer therapy. Pharmacol Ther. (2015) 147:22–31. doi: 10.1016/j.pharmthera.2014.11.001
80. Shull MM, Ormsby I, Kier AB, Pawlowski S, Diebold RJ, Yin M, et al. Targeted disruption of the mouse transforming growth factor-beta 1 gene results in multifocal inflammatory disease. Nature (1992) 359:693–9. doi: 10.1038/359693a0
81. Driscoll KE, Carter JM, Howard BW, Hassenbein D, Burdick M, Kunkel SL, et al. Interleukin-10 regulates quartz-induced pulmonary inflammation in rats. Am J Physiol. (1998) 275(5 Pt 1):L887–94. doi: 10.1152/ajplung.1998.275.5.L887
82. Hara K, Shirasuna K, Usui F, Karasawa T, Mizushina Y, Kimura H, et al. Interferon-tau attenuates uptake of nanoparticles and secretion of interleukin-1beta in macrophages. PLoS ONE (2014) 9:e113974. doi: 10.1371/journal.pone.0113974
83. Wang X, Coradin T, Helary C. Modulating inflammation in a cutaneous chronic wound model by IL-10 released from collagen-silica nanocomposites via gene delivery. Biomater Sci. (2018) 6:398–406. doi: 10.1039/c7bm01024a
84. Cohen C, Fireman E, Ganor E, Man A, Ribak J, Lerman Y. Accelerated silicosis with mixed-dust pneumoconiosis in a hard-metal grinder. J Occup Environ Med. (1999) 41:480–5. doi: 10.1097/00043764-199906000-00014
85. Chene AL, d'Almeida S, Blondy T, Tabiasco J, Deshayes S, Fonteneau JF, et al. Pleural effusions from patients with mesothelioma induce recruitment of monocytes and their differentiation into M2 macrophages. J Thorac Oncol. (2016) 11:1765–73. doi: 10.1016/j.jtho.2016.06.022
86. Jackaman C, Yeoh TL, Acuil ML, Gardner JK, Nelson DJ. Murine mesothelioma induces locally-proliferating IL-10+TNF-alpha+CD206−CX3CR1+ M3 macrophages that can be selectively depleted by chemotherapy or immunotherapy. Oncoimmunology (2016) 5:e1173299. doi: 10.1080/2162402X.2016.1173299
87. Bode C, Kinjo T, Alvord WG, Klinman DM. Suppressive oligodeoxynucleotides reduce lung cancer susceptibility in mice with silicosis. Carcinogenesis (2014) 35:1078–83. doi: 10.1093/carcin/bgu005
88. Huaux F, Arras M, Vink A, Renauld JC, Lison D. Soluble tumor necrosis factor (TNF) receptors p55 and p75 and interleukin-10 downregulate TNF-alpha activity during the lung response to silica particles in NMRI mice. Am J Respir Cell Mol Biol. (1999) 21:137–45. doi: 10.1165/ajrcmb.21.1.3570
89. Lee CG, Homer RJ, Cohn L, Link H, Jung S, Craft JE, et al. Transgenic overexpression of interleukin (IL)-10 in the lung causes mucus metaplasia, tissue inflammation, and airway remodeling via IL-13-dependent and -independent pathways. J Biol Chem. (2002) 277:35466–74. doi: 10.1074/jbc.M206395200
90. Sun L, Louie MC, Vannella KM, Wilke CA, LeVine AM, Moore BB, et al. New concepts of IL-10-induced lung fibrosis: fibrocyte recruitment and M2 activation in a CCL2/CCR2 axis. Am J Physiol Lung Cell Mol Physiol. (2011) 300:L341–53. doi: 10.1152/ajplung.00122.2010
91. Barbarin V, Arras M, Misson P, Delos M, McGarry B, Phan SH, et al. Characterization of the effect of interleukin-10 on silica-induced lung fibrosis in mice. Am J Respir Cell Mol Biol. (2004) 31:78–85. doi: 10.1165/rcmb.2003-0299OC
92. Barbarin V, Xing Z, Delos M, Lison D, Huaux F. Pulmonary overexpression of IL-10 augments lung fibrosis and Th2 responses induced by silica particles. Am J Physiol Lung Cell Mol Physiol. (2005) 288:L841–8. doi: 10.1152/ajplung.00329.2004
93. Balaji S, Wang X, King A, Le LD, Bhattacharya SS, Moles CM, et al. Interleukin-10-mediated regenerative postnatal tissue repair is dependent on regulation of hyaluronan metabolism via fibroblast-specific STAT3 signaling. FASEB J. (2017) 31:868–81. doi: 10.1096/fj.201600856R
94. Miura Y, Nishimura Y, Katsuyama H, Maeda M, Hayashi H, Dong M, et al. Involvement of IL-10 and Bcl-2 in resistance against an asbestos-induced apoptosis of T cells. Apoptosis (2006) 11:1825–35. doi: 10.1007/s10495-006-9235-4
95. Maeda M, Miura Y, Nishimura Y, Murakami S, Hayashi H, Kumagai N, et al. Immunological changes in mesothelioma patients and their experimental detection. Clin Med Circ Respirat Pulm Med. (2008) 2:11–7. doi: 10.4137/ccrpm.s577
96. Shitara K, Nishikawa H. Regulatory T cells: a potential target in cancer immunotherapy. Ann N Y Acad Sci. (2018) 1417:104–15. doi: 10.1111/nyas.13625
97. Dai W, Liu F, Li C, Lu Y, Lu X, Du S, et al. Blockade of Wnt/beta-catenin pathway aggravated silica-induced lung inflammation through tregs regulation on Th immune responses. Mediators Inflamm. (2016) 2016:6235614. doi: 10.1155/2016/6235614
98. Mohamud R, LeMasurier JS, Boer JC, Sieow JL, Rolland JM, O'Hehir RE, et al. Synthetic nanoparticles that promote tumor necrosis factor receptor 2 expressing regulatory T cells in the lung and resistance to allergic airways inflammation. Front Immunol. (2017) 8:1812. doi: 10.3389/fimmu.2017.01812
99. Freire J, Ajona D, de Biurrun G, Agorreta J, Segura V, Guruceaga E, et al. Silica-induced chronic inflammation promotes lung carcinogenesis in the context of an immunosuppressive microenvironment. Neoplasia (2013) 15:913–24. doi: 10.1593/neo.13310
100. Cornelissen R, Hegmans JP, Maat AP, Kaijen-Lambers ME, Bezemer K, Hendriks RW, et al. Extended tumor control after dendritic cell vaccination with low-dose cyclophosphamide as adjuvant treatment in patients with malignant pleural mesothelioma. Am J Respir Crit Care Med. (2016) 193:1023–31. doi: 10.1164/rccm.201508-1573OC
101. Liu F, Dai W, Li C, Lu X, Chen Y, Weng D, et al. Role of IL-10-producing regulatory B cells in modulating T-helper cell immune responses during silica-induced lung inflammation and fibrosis. Sci Rep. (2016) 6:28911. doi: 10.1038/srep28911
102. Rosser EC, Mauri C. Regulatory B cells: origin, phenotype, and function. Immunity (2015) 42:607–12. doi: 10.1016/j.immuni.2015.04.005
103. Sarvaria A, Madrigal JA, Saudemont A. B cell regulation in cancer and anti-tumor immunity. Cell Mol Immunol. (2017) 14:662–74. doi: 10.1038/cmi.2017.35
104. Lu Y, Liu F, Li C, Chen Y, Weng D, Chen J. IL-10-producing B cells suppress effector T cells activation and promote regulatory T cells in crystalline silica-induced inflammatory response in vitro. Mediators Inflamm. (2017) 2017:8415094. doi: 10.1155/2017/8415094
105. Saradna A, Do DC, Kumar S, Fu QL, Gao P. Macrophage polarization and allergic asthma. Transl Res. (2018) 191:1–14. doi: 10.1016/j.trsl.2017.09.002
106. Fujimura T, Kambayashi Y, Fujisawa Y, Hidaka T, Aiba S. Tumor-associated macrophages: therapeutic targets for skin cancer. Front Oncol. (2018) 8:3. doi: 10.3389/fonc.2018.00003
107. Choi M, Cho WS, Han BS, Cho M, Kim SY, Yi JY, et al. Transient pulmonary fibrogenic effect induced by intratracheal instillation of ultrafine amorphous silica in A/J mice. Toxicol Lett. (2008) 182:97–101. doi: 10.1016/j.toxlet.2008.08.019
108. Misson P, van den Brule S, Barbarin V, Lison D, Huaux F. Markers of macrophage differentiation in experimental silicosis. J Leukoc Biol. (2004) 76:926–32. doi: 10.1189/jlb.0104019
109. Kumar S, Meena R, Paulraj R. Role of macrophage (M1 and M2) in titanium-dioxide nanoparticle-induced oxidative stress and inflammatory response in rat. Appl Biochem Biotechnol. (2016) 180:1257–75. doi: 10.1007/s12010-016-2165-x
110. Romero F, Shah D, Duong M, Penn RB, Fessler MB, Madenspacher J, et al. A pneumocyte-macrophage paracrine lipid axis drives the lung toward fibrosis. Am J Respir Cell Mol Biol. (2015) 53:74–86. doi: 10.1165/rcmb.2014-0343OC
111. Migliaccio CT, Buford MC, Jessop F, Holian A. The IL-4Ralpha pathway in macrophages and its potential role in silica-induced pulmonary fibrosis. J Leukoc Biol. (2008) 83:630–9. doi: 10.1189/jlb.0807533
112. Ferreira TP, de Arantes AC, do Nascimento CV, Olsen PC, Trentin PG, Rocco PR, et al. IL-13 immunotoxin accelerates resolution of lung pathological changes triggered by silica particles in mice. J Immunol. (2013) 191:5220–9. doi: 10.4049/jimmunol.1203551
113. Tcyganov E, Mastio J, Chen E, Gabrilovich DI. Plasticity of myeloid-derived suppressor cells in cancer. Curr Opin Immunol. (2018) 51:76–82. doi: 10.1016/j.coi.2018.03.009
114. Veltman JD, Lambers ME, van Nimwegen M, Hendriks RW, Hoogsteden HC, Aerts JG, et al. COX-2 inhibition improves immunotherapy and is associated with decreased numbers of myeloid-derived suppressor cells in mesothelioma. Celecoxib influences MDSC function. BMC Cancer (2010) 10:464. doi: 10.1186/1471-2407-10-464
115. Boraschi D, Italiani P, Palomba R, Decuzzi P, Duschl A, Fadeel B, et al. Nanoparticles and innate immunity: new perspectives on host defence. Semin Immunol. (2017) 34:33–51. doi: 10.1016/j.smim.2017.08.013
116. Chauhan AS, Diwan PV, Jain NK, Tomalia DA. Unexpected in vivo anti-inflammatory activity observed for simple, surface functionalized poly(amidoamine) dendrimers. Biomacromolecules (2009) 10:1195–202. doi: 10.1021/bm9000298
117. Rajan R, Sabnani MK, Mavinkurve V, Shmeeda H, Mansouri H, Bonkoungou S, et al. Liposome-induced immunosuppression and tumor growth is mediated by macrophages and mitigated by liposome-encapsulated alendronate. J Control Release (2018) 271:139–48. doi: 10.1016/j.jconrel.2017.12.023
118. Sumbayev VV, Yasinska IM, Garcia CP, Gilliland D, Lall GS, Gibbs BF, et al. Gold nanoparticles downregulate interleukin-1beta-induced pro-inflammatory responses. Small (2013) 9:472–7. doi: 10.1002/smll.201201528
119. Ma JS, Kim WJ, Kim JJ, Kim TJ, Ye SK, Song MD, et al. Gold nanoparticles attenuate LPS-induced NO production through the inhibition of NF-kappaB and IFN-beta/STAT1 pathways in RAW264.7 cells. Nitric Oxide (2010) 23:214–9. doi: 10.1016/j.niox.2010.06.005
120. Wu HY, Chung MC, Wang CC, Huang CH, Liang HJ, Jan TR. Iron oxide nanoparticles suppress the production of IL-1beta via the secretory lysosomal pathway in murine microglial cells. Part Fibre Toxicol. (2013) 10:46. doi: 10.1186/1743-8977-10-46
121. Lee SS, Song W, Cho M, Puppala HL, Nguyen P, Zhu H, et al. Antioxidant properties of cerium oxide nanocrystals as a function of nanocrystal diameter and surface coating. ACS Nano (2013) 7:9693–703. doi: 10.1021/nn4026806
122. Gharbi N, Pressac M, Hadchouel M, Szwarc H, Wilson SR, Moussa F. [60]fullerene is a powerful antioxidant in vivo with no acute or subacute toxicity. Nano Lett. (2005) 5:2578–85. doi: 10.1021/nl051866b
123. Yamashita K, Sakai M, Takemoto N, Tsukimoto M, Uchida K, Yajima H, et al. Attenuation of delayed-type hypersensitivity by fullerene treatment. Toxicology (2009) 261:19–24. doi: 10.1016/j.tox.2009.04.034
124. Tomic S, Janjetovic K, Mihajlovic D, Milenkovic M, Kravic-Stevovic T, Markovic Z, et al. Graphene quantum dots suppress proinflammatory T cell responses via autophagy-dependent induction of tolerogenic dendritic cells. Biomaterials (2017) 146:13–28. doi: 10.1016/j.biomaterials.2017.08.040
125. Blank F, Gerber P, Rothen-Rutishauser B, Sakulkhu U, Salaklang J, De Peyer K, et al. Biomedical nanoparticles modulate specific CD4+ T cell stimulation by inhibition of antigen processing in dendritic cells. Nanotoxicology (2011) 5:606–21. doi: 10.3109/17435390.2010.541293
126. Tkach AV, Shurin GV, Shurin MR, Kisin ER, Murray AR, Young SH, et al. Direct effects of carbon nanotubes on dendritic cells induce immune suppression upon pulmonary exposure. ACS Nano (2011) 5:5755–62. doi: 10.1021/nn2014479
127. Mishra A, Stueckle TA, Mercer RR, Derk R, Rojanasakul Y, Castranova V, et al. Identification of TGF-beta receptor-1 as a key regulator of carbon nanotube-induced fibrogenesis. Am J Physiol Lung Cell Mol Physiol. (2015) 309:L821–33. doi: 10.1152/ajplung.00002.2015
128. Ma J, Bishoff B, Mercer RR, Barger M, Schwegler-Berry D, Castranova V. Role of epithelial-mesenchymal transition (EMT) and fibroblast function in cerium oxide nanoparticles-induced lung fibrosis. Toxicol Appl Pharmacol. (2017) 323:16–25. doi: 10.1016/j.taap.2017.03.015
129. Moon EY, Yi GH, Kang JS, Lim JS, Kim HM, Pyo S. An increase in mouse tumor growth by an in vivo immunomodulating effect of titanium dioxide nanoparticles. J Immunotoxicol. (2011) 8:56–67. doi: 10.3109/1547691X.2010.543995
130. Sanpui P, Zheng X, Loeb JC, Bisesi JH Jr, Khan IA, Afrooz AR, et al. Single-walled carbon nanotubes increase pandemic influenza A H1N1 virus infectivity of lung epithelial cells. Part Fibre Toxicol. (2014) 11:66. doi: 10.1186/s12989-014-0066-0
131. Findlay F, Pohl J, Svoboda P, Shakamuri P, McLean K, Inglis NF, et al. Carbon nanoparticles inhibit the antimicrobial activities of the human cathelicidin LL-37 through structural alteration. J Immunol. (2017) 199:2483–90. doi: 10.4049/jimmunol.1700706
132. Kanterman J, Sade-Feldman M, Baniyash M. New insights into chronic inflammation-induced immunosuppression. Semin Cancer Biol. (2012) 22:307–18. doi: 10.1016/j.semcancer.2012.02.008
133. Rocha-Parise M, Santos LM, Damoiseaux JG, Bagatin E, Lido AV, Torello CO, et al. Lymphocyte activation in silica-exposed workers. Int J Hyg Environ Health (2014) 217:586–91. doi: 10.1016/j.ijheh.2013.11.002
134. Rimal B, Greenberg AK, Rom WN. Basic pathogenetic mechanisms in silicosis: current understanding. Curr Opin Pulm Med. (2005) 11:169–73. doi: 10.1097/01.mcp.0000152998.11335.24
135. Breznan D, Karthikeyan S, Phaneuf M, Kumarathasan P, Cakmak S, Denison MS, et al. Development of an integrated approach for comparison of in vitro and in vivo responses to particulate matter. Part Fibre Toxicol. (2016) 13:41. doi: 10.1186/s12989-016-0152-6
136. Kasper J, Hermanns MI, Bantz C, Maskos M, Stauber R, Pohl C, et al. Inflammatory and cytotoxic responses of an alveolar-capillary coculture model to silica nanoparticles: comparison with conventional monocultures. Part Fibre Toxicol. (2011) 8:6. doi: 10.1186/1743-8977-8-6
137. Italiani P, Mazza EM, Lucchesi D, Cifola I, Gemelli C, Grande A, et al. Transcriptomic profiling of the development of the inflammatory response in human monocytes in vitro. PLoS ONE (2014) 9:e87680. doi: 10.1371/journal.pone.0087680
138. Lamichhane P, Karyampudi L, Shreeder B, Krempski J, Bahr D, Daum J, et al. IL10 release upon PD-1 blockade sustains immunosuppression in ovarian cancer. Cancer Res. (2017) 77:6667–78. doi: 10.1158/0008-5472.CAN-17-0740
139. Stockis J, Lienart S, Colau D, Collignon A, Nishimura SL, Sheppard D, et al. Blocking immunosuppression by human tregs in vivo with antibodies targeting integrin αVβ8. Proc Natl Acad Sci USA. (2017) 114:E10161–8. doi: 10.1073/pnas.1710680114
140. Patel SA, Minn AJ. Combination cancer therapy with immune checkpoint blockade: mechanisms and strategies. Immunity (2018) 48:417–33. doi: 10.1016/j.immuni.2018.03.007
141. Maeda M, Nishimura Y, Kumagai N, Hayashi H, Hatayama T, Katoh M, et al. Dysregulation of the immune system caused by silica and asbestos. J Immunotoxicol. (2010) 7:268–78. doi: 10.3109/1547691X.2010.512579
Keywords: immunosuppression, inflammation, TGF-β, IL-10, immunosuppressive lymphocytes, immunosuppressive myeloid cells
Citation: Huaux F (2018) Emerging Role of Immunosuppression in Diseases Induced by Micro- and Nano-Particles: Time to Revisit the Exclusive Inflammatory Scenario. Front. Immunol. 9:2364. doi: 10.3389/fimmu.2018.02364
Received: 04 May 2018; Accepted: 24 September 2018;
Published: 19 November 2018.
Edited by:
Martin Herrmann, Universitätsklinikum Erlangen, GermanyReviewed by:
Ahmed El-Fiqi, Dankook University, South KoreaCopyright © 2018 Huaux. This is an open-access article distributed under the terms of the Creative Commons Attribution License (CC BY). The use, distribution or reproduction in other forums is permitted, provided the original author(s) and the copyright owner(s) are credited and that the original publication in this journal is cited, in accordance with accepted academic practice. No use, distribution or reproduction is permitted which does not comply with these terms.
*Correspondence: François Huaux, ZnJhbmNvaXMuaHVhdXhAdWNsb3V2YWluLmJl
Disclaimer: All claims expressed in this article are solely those of the authors and do not necessarily represent those of their affiliated organizations, or those of the publisher, the editors and the reviewers. Any product that may be evaluated in this article or claim that may be made by its manufacturer is not guaranteed or endorsed by the publisher.
Research integrity at Frontiers
Learn more about the work of our research integrity team to safeguard the quality of each article we publish.