- 1School of Biotechnology, KIIT University, Bhubaneswar, India
- 2Institute of Cytology of the Russian Academy of Sciences (RAS), St. Petersburg, Russia
- 3Klinikum Rechts der Isar, Technical University of Munich, Munich, Germany
- 4First Pavlov State Medical University of St.Petersburg, St. Petersburg, Russia
- 5Discipline of Biosciences and Biomedical Engineering, Indian Institute of Technology Indore, Indore, India
Due to emergence of new variants of pathogenic micro-organisms the treatment and immunization of infectious diseases have become a great challenge in the past few years. In the context of vaccine development remarkable efforts have been made to develop new vaccines and also to improve the efficacy of existing vaccines against specific diseases. To date, some vaccines are developed from protein subunits or killed pathogens, whilst several vaccines are based on live-attenuated organisms, which carry the risk of regaining their pathogenicity under certain immunocompromised conditions. To avoid this, the development of risk-free effective vaccines in conjunction with adequate delivery systems are considered as an imperative need to obtain desired humoral and cell-mediated immunity against infectious diseases. In the last several years, the use of nanoparticle-based vaccines has received a great attention to improve vaccine efficacy, immunization strategies, and targeted delivery to achieve desired immune responses at the cellular level. To improve vaccine efficacy, these nanocarriers should protect the antigens from premature proteolytic degradation, facilitate antigen uptake and processing by antigen presenting cells, control release, and should be safe for human use. Nanocarriers composed of lipids, proteins, metals or polymers have already been used to attain some of these attributes. In this context, several physico-chemical properties of nanoparticles play an important role in the determination of vaccine efficacy. This review article focuses on the applications of nanocarrier-based vaccine formulations and the strategies used for the functionalization of nanoparticles to accomplish efficient delivery of vaccines in order to induce desired host immunity against infectious diseases.
Introduction
In twenty-first Century, infectious diseases have emerged as a serious threat to the health of millions of people across the globe (1). According to the World Health Organization (WHO) report for 2016, ~3.2 million deaths have occurred due to lower respiratory infections and 1.4 million from tuberculosis alone worldwide (2). Over the past few decades, many new infectious diseases have emerged and few old diseases re-emerged, which were once considered to be no longer a threat to the human being (3–5). Collectively, these diseases account for millions of deaths that cause enormous impact on the global socio-economical and health-care sectors. The major challenges to combat such diseases are that for many of them, there are no effective drugs available. One of the plausible approaches could be based on the application of nanocarrier based vaccination (6). However, there are still no effective vaccines available against some of the most prevalent diseases including immune deficiency syndrome (AIDS) and tuberculosis. This underlines an urgent need for the development of desired vaccines against these diseases. Some of the important aspects of any optimal vaccine includes (i) safety, (ii) stability, and (iii) the ability to elicit durable and adequate immune response with a minimum number of doses (7–9). Presently, different generation vaccines such as attenuated or killed whole organisms (first generation), subunit (second generation) and RNA or DNA vaccines (third generation) are used to elicit protective immunity against diseases (10–12). Despite several advantages of RNA or DNA vaccines such as minimal risk of infection, ability to elicit immune response against specific pathogen and cost effective (13); there are a number of challenges associated with the efficient delivery of these vaccine molecules to the target sites and the requirement of the prime-boost vaccination regimens with other immunogenic agents. These includes premature degradation of molecules and the inability to translate into a functional immunogen (14). Similarly, protein based vaccines are used successfully against several infectious diseases such as Haemophilus influenza type b, diphtheria, tetanus, acellular pertusis, meningococcus and pneumococcus (15), however they require an adjuvant to potentiate their immunogenicity, and also encounter early degradation after exposure to hostile milieu. Introduced recombinant protein-based vaccines (e.g., recombinant hemagglutinin vaccine for influenza) further enhance the immunity toward infection indicating the applicability of the recombinant technology for the vaccine production (16). To overcome these hurdles, an efficient vaccine delivery system is required which not only delivers the vaccine molecules to the target site to evoke enduring immune responses but also has minimal side effects and requires less doses. Moreover, there is an increasing need to develop new generation composite vaccine molecules that will act as immunogen as well as an adjuvant. Nanotechnology based formulations offer numerous advantages for the development of new generation vaccines. Nanocarrier based delivery system can protect the vaccines from premature degradation, improve stability, has good adjuvant properties, and also assists in targeted delivery of an immunogen to the antigen presenting cells (APCs). There are several mechanisms by which vaccines can be delivered to the specific sites using nanocarriers. Vaccine antigens can be encapsulated within the nanocarriers or decorated on their surface (Figure 1). Encapsulation within the nanoparticles (NPs) can protect the antigen from premature protease degradation and elicit sustainable release, whereas the surface adsorption facilitates their interaction with cognate surface receptors such as toll like receptors (TLRs) of APCs (17). Nanocarrier based delivery systems provide a suitable route of administration of vaccine molecules and enhance cellular uptake thereby resulting in robust innate, humoral, cellular as well as mucosal immune responses when compared with unconjugated antigens. This review mainly focuses on the potential use of nano delivery systems as novel vaccine strategies for the induction of innate as well as adaptive immune responses against infectious diseases.
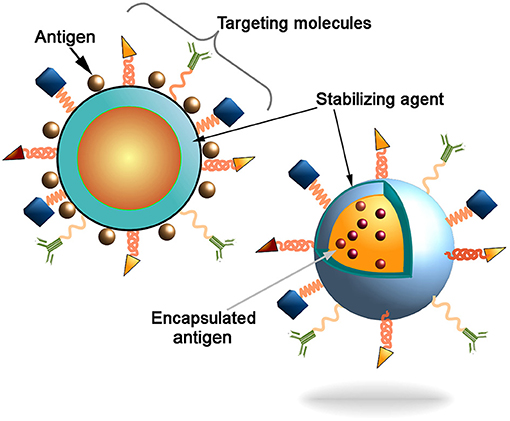
Figure 1. Schematic representation of the nanocarriers. Antigen can be conjugated to the nanoparticles surface or incapsulated into core of the particles. Decoration of the nanoparticles surface with targeting molecules (e.g., antibodies, Fab-fragments, peptides, etc) could further increase the delivery of particles into the antigen presenting cells (APCs) to induce innate and adaptive immune responses.
Key Cellular Components of the Immune System
The immune system is composed of a collection of mobile cells that traffic throughout the body as well as reside at the site of entry (i.e., skin, respiratory, gastrointestinal, and genital tracts) in search of invading pathogens. These cells belong to two major types of innate and adaptive immune system. The innate immune cells like macrophages and neutrophils rapidly respond to the pathogens by recognizing pathogen surface moieties, phagocytosis, and the elimination of pathogens through activation of different antibacterial effector functions. Similarly, two major components of the adaptive immunity i.e., T and B-cells are important for the generation of cell mediated and humoral immune responses, respectively. T cells including CD4+ helper T cells secrete different cytokines to modulate the functions of B cells, whereas CD8+ T cells recognize and destroy virally infected cells. Antibodies produced by the B cells can further neutralize the invading microbes or clear infected cell or opsonized pathogens through cell-mediated systems. APCs, in particular dendritic cells (DCs) and macrophages, migrate through the body to sample, process and present the antigens to T-cells to activate cellular immune responses. These cells express various surface receptors to recognize cognate ligands and danger signals to trigger activation of different signaling pathways that eventually lead to the activation of T-cells (18). After sampling the antigens, DCs migrate from the peripheral tissues into the draining lymph nodes to activate naive T-cells (19), whereas macrophages after ingestion of antigens increase their lysosomal degradative machinery to enhance the antigen presentation to activate helper T cells.
Types of Nano-Immuno Activators
Some NPs are themselves able to stimulate different immune cells to boost the host immunity. The size, shape and surface chemistry of NPs (described below in more detail) are important factors that determine their potential to activate immune responses. In general, NPs are able to stimulate immune reactions by increasing the synthesis of defense genes and inflammatory reactions (20). Various types of NPs like gold, carbon, dendrimers, polymers and liposomes have the capability to induce cytokine and antibody responses (21–26). This was observed in the case of administration of empty PEGylated liposomes, which were able to elicit IgM response in an in-vivo model. (27, 28). Besides their potential to deliver various immune stimulators to the specific sites as well as into the deep tissues where vaccine molecules alone may not able to reach, these NPs have also been exploited as adjuvants to augment immunogenicity of vaccine candidates. Nano-immuno stimulators are the nano scale (20–100 nm) vaccine particles that can improve the vaccine efficacy in vivo better than bulk molecules (20, 29). Some of the known nano-immuno stimulators that have been used for this specific purpose are inorganic NPs (iron and silica) (30, 31), polymeric NPs (chitosan, PLGA, PVPONAlk, γ-PGA) (32–37), liposomes (cholesterol and lipids) (33, 38) and virus like particles (VLPs) (39, 40). Different types of NPs used to deliver antigens to give protection against different diseases have been listed in Table 1.
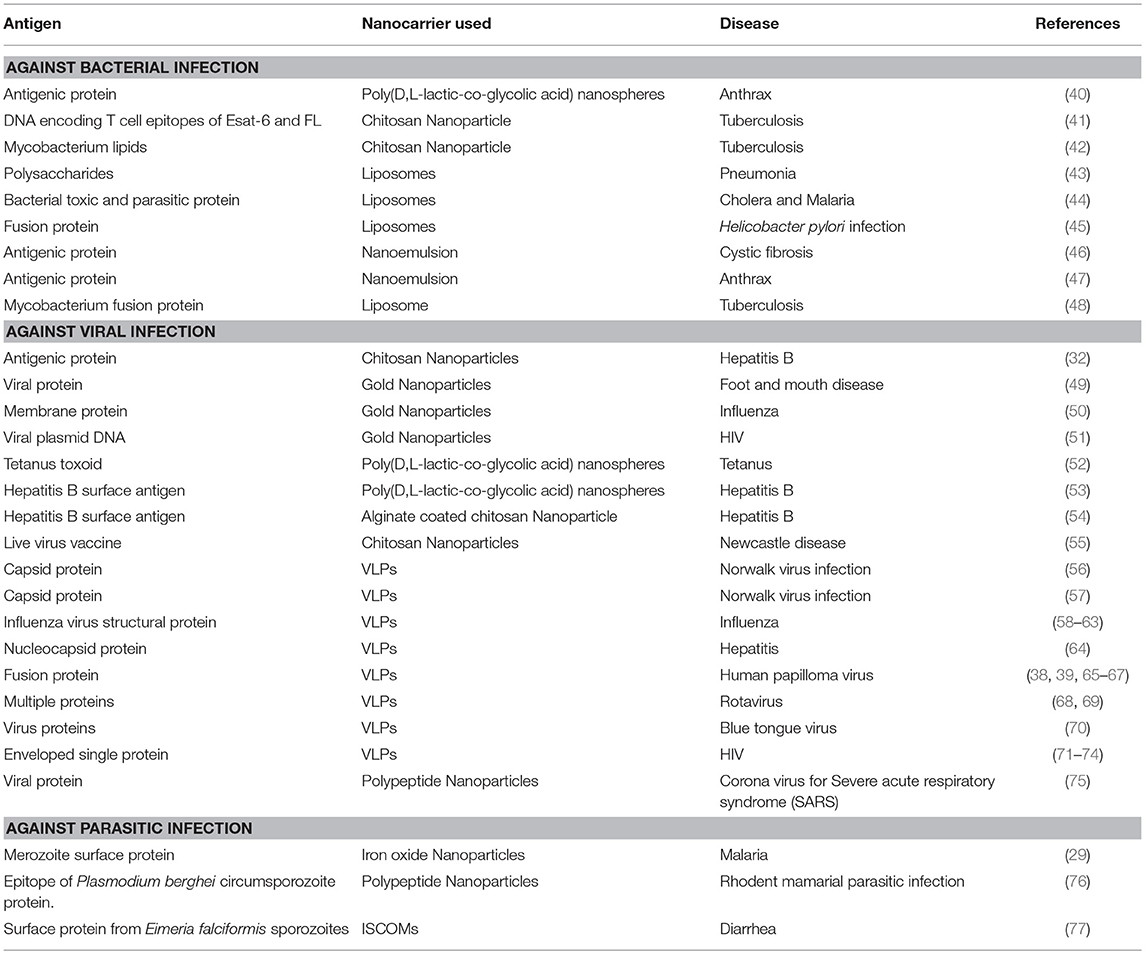
Table 1. List of antigens delivered by using different nanocarriers for the treatment of different diseases.
Inorganic NPs
Some biocompatible inorganic NPs such as gold, carbon and silica have been exploited in the vaccine delivery studies (50, 79–81). These NPs can be synthesized in various shapes, size and surface modified forms. Some of the viral antigens were successfully delivered using inorganic NPs as carriers. This caused increase in antigen stability by protecting them from premature degradation by proteolytic enzymes. Delivery of viral and bacterial antigens using gold NPs was also found to induce quite robust host immune responses against influenza, immunodeficiency virus, foot and mouth, and tuberculosis diseases in mice (51, 52, 82, 83). Encapsulation of plasmid DNA that encode mycobacterial hsp65 antigen in gold NPs exhibited significant reduction in the Mycobacterium tuberculosis, causative agent of human tuberculosis, burden in infected mice (52, 82). Few studies have used hollow mesosporous silica, nanotube and spherical forms of carbon NPs as adjuvants to improve the immunogenicity and delivery of protein and peptide antigens against viral infections (79, 83, 84). Silica based NPs contain abundant silanol groups that can be utilized to introduce specific functional groups on their surface to gain access for vaccine molecules into target cells (84–86). The major advantages of inorganic NPs include low production cost, reproducibility and safety in application.
Polymeric NPs
In recent years, polymeric NPs have received great attention for their applications in the delivery of a number of vaccines. This is primarily due to their ease in preparation, biodegradability, biocompatibility, reduced cytotoxicity, and the possibility to fine-tune surface properties as needed (87). Moreover, it is relatively easy to control the rate of vaccine release by altering the composition or ratio of co-polymers during the NP synthesis process (87). The most commonly used polymeric NPs for vaccine delivery are poly (lactic-co-glycolic acid; PLGA) or poly (lactic acid; PLA). PLGA NPs have already been tried in the delivery of a broad range of antigens, including hydrophobic antigens (34, 35), hepatitis-B virus antigens (54), Bacillus anthracis (41), tetanus toxoid (35), and ovalbumin (88). The use of PLGA conjugated antigens exhibited strong immuno-stimulatory property by inducing cytokine and nitric oxide production against mycobacteria infection (89). In addition to synthetic polymers, some natural biopolymers such as alginate, pullans, inulins, and chitosan have been used as adjuvants (90–93). Inulin, a known activator of the complement cascade (94), conferred better protection against hepatitis B and influenza viruses (92, 93). Similarly, chitosan NPs were demonstrated as nanocarrier molecules for HBV antigens (55), DNA vaccine (56), and Newcastle disease vaccine (42). The delivery of PLGA and chitosan NP conjugated vaccine molecules enhanced the immune responses at the mucosal site (95, 96). Our recent study also showed that delivery of M. tuberculosis lipids using biocompatible chitosan NPs was able to induce significant humoral as well as cellular immune responses when compared to lipids alone in mice (43). We also found that intraperitoneal administration of these conjugates showed better activation of splenic T-cells. Another study by de Titta et al. has shown that intradermal administration of CpG conjugated polymeric NPs increased dendritic cell activation by several fold, exhibited comparable vaccine efficacy at ~400 times lower dose, and also caused enduring cellular immunity in comparison to free CpG (97). These desired properties along with already known reduced toxicity and biocompatibility under both in vitro and in vivo conditions make polymeric NPs plausible candidates for further preclinical pharmacokinetics and therapeutic applications (98).
Liposomes
In addition to polymeric NPs, liposomes are the second most widely explored vaccine and drug delivery vehicle in the nanomedicine field. The synthesis of liposomes is a spontaneous process, where hydration of lipids enables the lipid bilayer formation around an aqueous core (99). So far, different types of liposomes, including unilamellar or multilamellar vesicles composed of biodegradable phospholipids (e.g., phosphatidylserine, phosphatidylcholin and cholesterol) were included in the vaccine studies (100). Liposomes deliver vaccines by fusion with the target cell membrane (101).The structurally flexible and versatile liposomes are able to encapsulate both hydrophilic and hydrophobic substances. The hydrophilic molecules can be incorporated into the aqueous core, while hydrophobic molecules are encased within the phospholipid bilayer. Earlier reports have shown that delivery of antigenic proteins entrapped in multilamellar lipid vesicles elicit strong T and B-cell responses (102). Similarly antigenic peptides conjugated to phosphatidylserine (PS)-liposomes were readily internalized by APCs to potentiate T-helper cell mediated immune responses (103) and delivery of heat shock protein encoding vaccine DNA using liposomes elicited strong protective immunity against fungal infection (104). Because of their foreseen applications, several liposome based vaccine nano-formulations have been approved for clinical trials against intracellular pathogens, including viruses and M. tuberculosis (105). One such study already demonstrated the potency of liposomal aerosol carriers in the generation of protective immunity against M. tuberculosis infection (106, 107). Other studies have tried a combination of dimethyl dioctadecyl ammonium (DDA) lipid based liposomes and various immunomodulators to enhance immunity against influenza, chlamydia, erythrocytic-stage malaria, and tuberculosis infections (108–112). In the context of DNA vaccines, lipid-DNA complexes have been successfully delivered to the lungs of monkeys (101).
VLPs (Virus Like Particles)
There are several reports that adequately proved applications of VLPs as a vaccine carrier, and also their ability to stimulate the host immune responses (113–115). VLPs are composed of self-assembled viral membrane that forms a monomeric complex displaying a high density of epitopes (115, 116). Interestingly, VLPs can also be engineered to express additional proteins either by fusion of proteins with the particles or by endogenous expression of multiple antigens (113, 117). It is also possible to chemically couple non-protein antigens and small organic molecules onto the viral surface to produce bioconjugates with VLPs (118, 119). Due to these distinct features, VLPs can provide protection not only against virus, but also against heterologous antigens (116). A specific immune response was successfully generated after the delivery of an antigen using virus capsid protein SV40 in mammalian cells (120). VLPs were also found to increase the immunogenicity of weak antigens. For example Salmonella typhi membrane antigen, influenza A M2 protein and H1V1 Nef gonadotropin releasing hormone (GnRH) assembled VLPs produced strong antigen specific humoral as well as cellular immune responses (121, 122). It is presumed that the use of VLP based nanoformulations could enable the antigens to achieve conformations resembling to native antigen structure, thus it may result in better stimulation of the host immune response (122).
Dendrimers
Dendrimers are three dimensional, mono-dispersed and hyperbranched nano structures that are made up of a mixture of amines and amides. Few studies have explored the application of dendrimers in the delivery of different antigenic molecules. The most commonly used dendrimers for vaccine delivery are polypropyleneimine (PPI) and polyamido amine (PAMAM) dendrimers. A single dose of dendrimer encapsulated multiple antigens was found to produce strong antibody and T-cell responses against Ebola virus, H1N1 influenza, and Toxoplasma gondii (123). This generation of robust immune response was found to be due to efficient uptake of dendrimers by the host cells. Similarly a significant increase in the vaccine efficacy of HIV transactivator of transcription (TAT) based DNA vaccine was observed due to enhanced cellular uptake of PMAM dendrimer (124). Hence, the possibility to tailor the dendrimers to attain certain biological and physico-chemical properties, and also the feasibility to conjugate several ligands to the single molecule have made dendrimers promising candidates for the development of new generation vaccines with enhanced immunogenic properties.
Delivery of Immune Stimulators Using Nanocarriers
Cytokines
Cytokines are known as important signaling molecules secreted by different cells in response to external stimuli. Some of the cytokines are able to activate immune cells to generate protective immunity against several diseases. However, cytokines are mostly susceptible to early degradation that subdue their participation in the generation of host immunity. Moreover, uncontrolled release of cytokines as immune responders may sometimes lead to harmful side effects (125). To overcome these limitations, several studies have attempted to synthesize engineered nanocarriers to achieve effective and controlled delivery of cytokines to the target sites. This approach was found to reduce their toxicity, improve circulation time and antigen specific T-cell responses in comparison to free cytokines (126, 127). Incorporation of granulocyte macrophage colony stimulating factor (GM-CSF) and interferon alpha (IFN-α) into nano-carriers exhibited great application in cancer therapy (128, 129). Nano-carrier conjugated cytokines also showed great potential in the treatment of infectious diseases. For example, IL-12 encapsulated microspheres induced strong protective immunity against tuberculosis (130). This effect was due to production of high antibody titers as a result of sustained and controlled release of IL-12 from the microspheres in immunized mice (130).
Toll Like Receptor Agonists
Like cytokines, several toll-like receptor (TLR) agonists were also explored as immune activators to augment immune surveillance mechanisms. Different immune effector cells such as macrophages, B-cells and DCs express different types of TLRs, which are known to interact with specific pathogen associated molecular patterns (PAMPs). These specific interactions eventually initiate downstream signaling cascades to ensure the elimination or generation of immunity against pathogens (131, 132). Conjugation of TLR specific agonists on nanocarriers helps to target the molecules to specific immune cells and therefore reduce the possibility of systemic biodistribution. One such study has shown that conjugation of TLR-7/8 agonist on nano polymers caused efficient internalization by APCs and also prolonged the T cell responses (133). Administration of NPs loaded with vaccine peptide antigen and TLR-7 and 9 ligands were also found to induce strong memory and effector CD8+ T-cell response (134). Another study has shown that conjugation of TLR-8 agonist to a polymer nanocarrier increased activation and maturation of naive DCs due to selective endocytosis and prolonged release of an immunogen by the nanocarrier inside DCs (135). Moreover, intradermal injection of CpG and antigen encapsulated polymeric NPs were rapidly drained into the lymph nodes to activate DCs (97). These studies indicate that NPs can be used as a tool to appropriately target presentation of antigens to T and B-cell rich lymphoid organs.
Nucleic Acids
The genetic molecules such as DNA, plasmids and RNA can also act as immuno-stimulants. Due to these characteristics, in addition to less risk to cause disease particularly in immunocompromised individuals, these genetic materials are considered as promising candidates for the development of next generation vaccines. After administration, the plasmid vector translocates to the nucleus to initiate transcription of recombinant genes using the host cellular machinery. A recombinant DNA segment encoding HspX-PPE44-esxV fusion antigen of M. tuberculosis showed great potential as a new tuberculosis DNA vaccine candidate (136). A similar type of study has been conducted in the past where the vaccination of DNA or RNA constructs expressing mycobacterium antigens were capable of inducing humoral as well as cellular immune responses (137). Likewise, plasmids harboring genes encoding for viral antigen have been encapsulated into alginate nanocarriers and targeted against viral infections (138).
Importance of Physicochemical Properties in Designing Nano-Immuno Formulations
In order to improve their delivery and vaccine characteristics, different approaches have been practiced to conjugate vaccine molecules to different nanocarriers. Vaccine molecules can be surface conjugated, encapsulated or surface adsorbed with the nanocarriers. Antigen adsorption on the nanocarrier is simply based on the presence of a charge or hydrophobic interactions between NPs and the candidate molecule (139, 140). This type of interaction is usually non-covalent, which may lead to rapid dissociation of antigens from nanocarriers depending upon the external milieu such as pH, ionic strength, temperature, and the antigen hydrophobicity. On the other hand, encapsulation and chemical conjugation of antigen to nanocarriers is more stable due to strong interactions and chemical bond formation between the target molecule and the nanocarrier. Further, antigens can also be encapsulated into nanocarriers by simple mixing reaction during the synthesis. In this case, the antigens are released only after partial or complete dissociation of the nanocarrier (141). These processes have already been used with silica and gold NPs (142). Similarly, chitosan and dextran sulfate NPs were used for the preparation of cationic and anionic antigenic formulations. Some viral antigens are known to bind to both positive as well as negative charged NPs through immobilization process and hydrogen bonds (143). The immobilization process depends on the charge, pH, ratio of NPs and antigens, and the protein partition coefficient between the solution and the colloid (143). Several antigens were successfully delivered to the target sites by chemical conjugation, adsorption and encapsulation to soft nanocarriers like VLPs, liposomes and immune stimulating complexes (ISCOM) (144–147). ISCOMs are a class of adjuvant formulations that consist of saponins, cholesterol and phospholipids in specific ratios. Antigens can be formulated into ISCOMs directly (148) or after the surface modification (149, 150). Since ISCOM particles are negatively charged, direct conjugation of most of the soluble proteins is a limiting factor. Nanocarriers can augment immunogenicity of a molecule. For example, influenza antigen H1N1 conjugated chitosan NPs and Yersinia pestis F1-antigen coated gold NPs (AuNPs) produced higher levels of antibody and cytokine responses in comparison to mice administered with unconjugated antigens (151).This was found to be due to stabilization and increased immunogenicity of vaccine antigens due to conjugation with NPs.
Another important aspect in the development of nano-immuno formulations is that they improve antigen delivery and presentation (152). In this context, NP shape, size and surface charge are key factors that affect NP circulation, biodistribution, bioavailability and specificity by crossing biological barriers. Besides these factors, particle geometry such as surface to volume ratio plays an important role in the determination of immunogen release and degradation kinetics (153, 154). Here, the importance of different physicochemical parameters such as size, shape, surface area, porosity, hydrophobicity, hydrophilicity and crystallinity in the interaction between NPs and the target cell is discussed.
Size
The size of NPs determines the mode of cellular uptake and specificity (155, 156). PLGA NPs of large size (1, 7 and 17 μm) showed reduced internalization rate in comparison to smaller NPs (300 nm) (157). The size of NPs also determines the cellular specificity and migration. Smaller NPs (20–200 nm) were readily endocytosed by the resident DCs, whereas larger size (500–2,000 nm) NPs were effectively taken up by the migratory DCs (158). NPs of less than 200 nm size were drained into the lymph nodes (159), while particles up to 20 nm range were suitably transported to the APCs (152, 160). Notably, NP curvature also affects the cellular interaction and phagocytosis rate (161). NPs of 150 nm diameter and 450 nm height showed more cellular uptake as compared to the particles having 1,200 × 200 nm size. Of note the size of NPs was also found to influence the activation of signaling pathways. A study has demonstrated that smaller NPs are able to alter the cell signaling processes more efficaciously than the large NPs (31).
Surface Charge
Vaccine loaded NPs can also be targeted to specific sites by modifying the NP surface charge. Delivery of such NPs at appropriate sites elicit strong immune responses against antigens. NP surface charge is responsible for the interaction with congnate surface molecules present on the target cells. This was exemplified from the observation that cationic polysterene NPs were efficiently internalized by the APCs in comparison to neutral surface charged NPs. This may be due to electrostatic interactions between the cationic NPs with anionic cell membranes (162, 163). Interestingly, pulmonary instillation of cationic and anionic NPs showed similar endocytosis rate in macrophages and draining lymph nodes, however cationic formulations showed more expression of Ccl2 and Cxc10 chemokines that caused more recruitment and maturation of CD11b DCs in comparison to anionic NPs in the lung (125, 156). Similarly, neutral silica-silane shell polymer NPs were less effective in the activation of innate immune cells (128). These studies clearly indicate appropriate surface modifications of NPs may help to generate stronger immunological responses against specific infection.
Shape
Beside size and surface charge, NP shape is also a critical determinant in the cellular interaction, intracellular trafficking and the rate of antigen release inside the host cells (79, 141). Spherical gold NPs were actively internalized by bone marrow derived dendritic cells in comparison to rod shaped particles of similar dimensions (33, 34), and that spherical NPs were able to induce strong immune response than cube or rod shaped NPs (164). Another study reported that worm-like particles were impaired in phagocytosis as compared to spherical NPs (151). These distinctions were ascribed to the differences in contact area between NPs and the target cell membrane. The shape of NPs also determines the localization of NPs inside the host cells. This was demonstrated by the fact that although nano rods and nano sheets were internalized via clathrin mediated endocytosis, nano rods were particularly delivered to the nucleus while nano-sheet were retained in the cytoplasm (146, 147, 155). This is an important aspect in the context of improving antigen processing and presentation to T-cells. It is well established that enhanced antigen processing and presentation can be achieved if the candidate molecules are delivered to the lysosomal compartment of the cells.
Hydrophobicity
Hydrophobicity of NPs plays a significant role in the interaction with soluble proteins and immune cells through recognition of hydrophobic moieties (165). Previous studies have shown that hydrophobic polymeric NPs are strong inducers of cytokines and co-stimulatory molecules than hydrophilic polymeric NPs (53, 105, 166). Exposure to hydrophobic NPs showed enhanced activation of DCs by inducing the expression of CD86 co-stimulatory molecules when compared with hydrophilic ones. Similar observations were reported in other innate immune cells, in which hydrophobic NPs were able to activate these cells by up-regulating the expression of proinflammatory cytokine encoding genes (102), and also facilitated opsonization process by increasing the adsorption of immunoglobulins on the cell surfaces (103). However, other studies have reported that polyethylene glycol coating (PEGylation) reduced the interaction of NPs with immune receptors (50, 80). This property is considered useful in the prevention of non-specific adsorption of proteins on NPs and thereby prevent their up-take by APCs (50). Such non-specific adsorption of proteins and their uptake by phagocytic cells can also be preventing by the incorporation of an alkyl linker between the PEG and thiol moieties on NPs (80).
Surface Modification
Surface modification of NPs alters ligand specificity and interaction with APCs (160). Conjugation of CD47 molecules on the surface of NPs modulated the down-stream signaling cascades and also reduced NP internalization by phagocytic cells (131). Functionalization of NPs with TLR-7, TLR-8, and TLR-9 agonists increased cytokine production and the expression of immunoregulatory genes (132–134). Similarly, conjugation of poly (methyl vinyl ether-co-maleic anhydride; PVMA), TLR2, and TLR4 agonists, and galactose polymer to NPs were shown to activate the complement pathway as a result of stable binding to C3b complement factor (139, 142). Further, lipoprotein-like NPs showed LPS scavenging activity, thereby resulting in the inhibition of TLR-4 dependent inflammatory responses (140). Overall, these studies strongly demonstrated that tuning of physico-chemical properties of NPs could be used as a fundamental tool to target vaccine molecules to specific sites to induce desired immune responses.
Implications of the Nanocarriers in the Vaccine Development
Emerging studies have proved that nanocarriers can be useful mediators in the development of vaccines against various diseases. In this context, it is important to develop NP formulations that can deliver immunogens to APCs especially DCs to induce effective antigen-specific T-cell responses (Figure 2). Several nanocarriers have been shown to specifically activate DCs to effectuate anti-tumor or anti-viral immune responses (167–170). Zhu et al. proposed that nano-TiO2 and Fe3O4-TiO2 particles could function as a useful vector to promote vaccine delivery in immune cells (168). Co-incubation of nano-TiO2 and Fe3O4-TiO2 with DCs resulted in an increased production of TNF-α, and also upregulated the expression of CD80, CD86 and MHC class II molecules through the NF-κB signaling pathway (163). In this way, immunization efficacy of various NP formulations such as erythrocyte membrane-enveloped poly(D,L-lactide-co-glycolide) (PLGA) NPs for antigenic peptide (hgp10025-33) and TLR-4 agonist, VLPs expressing RSV glycoproteins, chitosan-coated EphrinA1-PE38/GM-CSF, and several others have been improved (171–177). NPs can also control cell polarization and differentiation. Branched polyethylenimine-superparamagnetic iron oxide NPs (bPEI-SPIONs) promoted Th1 polarization of DCs (178). Another study by Sehgal et al. showed that NPs can also be used to target subsets of particular immune cells. They have shown that simultaneous targeting of DC subsets (i.e., DC-SIGN+ and BDCA3+DC) by NPs synergistically stimulated the activation of T cell-mediated immunity when compared with targeting of each DC subset separately (170).
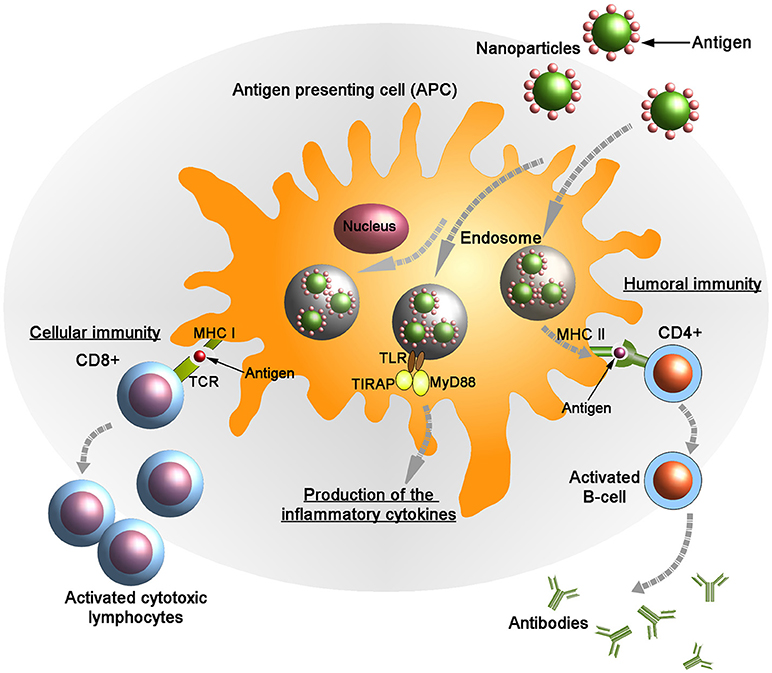
Figure 2. Targeted delivery of antigenic molecules using surface engineered nanoparticles into the antigen presenting cells (APCs). Endogenously generated antigens are presented in complex with class I major histocompatibility complex (MHC I) on the membrane of APCs to CD8+ T lymphocytes. Following the interaction between MHC I and T-cell receptor (TCR) in presence of co-stimulatory molecules and cytokines the activated CD8+ cells kill the infected cells by inducing cytotoxicity. Also the antigens are presented on the APC surface by class II MHC molecules to the helper (CD4+) T cells. Subsequently, CD4+ cells activate B-cells that produce anti-microbial antibodies. Upon stimulation the adaptor proteins MyD88 (myeloid differentiation marker 88) and TIRAP (TIR domain containing adaptor protein) colocalize with TLR (toll-like receptor) allowing for activation of the NF-κB pathway and leading to the production of pro-inflammatory cytokines.
Preclinical studies by different research groups have successfully demonstrated the efficacy of NP based vaccines in the induction of specific immune responses against tuberculosis (42, 179–182). Feng et al. developed a NP-based recombinant DNA vaccine that consists of Esat-6 and fms-like tyrosine kinase 3 ligand enveloped with chitosan NPs (42). Intramuscular prime vaccination followed by nasal boost of this recombinant DNA vaccine remarkably enhanced T cell responses in Mycobacterium tuberculosis challenged mice (42). Another study has shown that pulmonary administration of M. tuberculosis Ag85B antigen and CpG adjuvant conjugated polypropylene sulfide NPs (NP-Ag85B) induced M. tuberculosis specific polyfunctional Th1 responses and also reduced the lung bacterial burden (183).
Targeted Delivery of Nanoparticles Can Activate Innate and Adaptive Immune Responses
Innate Immunity
Macrophages and monocytes are highly heterologous cells that are distributed throughout the body. Macrophages process and present the antigens to elicit adaptive immune response. Due to their intrinsic phagocytic nature, macrophages can be easily targeted by surface engineered NPs, in which cognate ligands agonist to macrophage receptors can be conjugated on the NP surface (Figure 1). As discussed above several physico-chemical parameters of NPs such as size, surface charge, hydrophobicity, surface topography, and material composition can be optimized to facilitate the interactions between NPs and macrophage receptors (184–186). The rate of NP endocytosis also depends upon the type of cell surface receptors and the ligand conjugated to the NP surface. For example, NPs targeted via mannose and Fc receptors were rapidly internalized as compared to scavenger receptors (187). Endocytosis of IgG and anti-F4/80 antibody coated NPs showed more uptake rate and retention time inside the macrophages without affecting the cell viability (188, 189). Also, positively charged NPs interact more strongly with negatively charged phospholipid components of the cell membrane (190). Hyperactivation of some inflammatory cells can also be restricted through controlled release of stimulants using NPs. Upon activation, neutrophils can secrete variety of cytokines and hydrolytic enzymes in response to infection (191). Prolonged neutrophil activation often leads to acute inflammation and tissue damage at the localized site. Therefore, controlled release of molecules is necessary to prevent the hyperactivation and massive recruitment of neutrophils. It has been reported that bovine serum albumin (BSA) NPs were able to modulate the functions of neutrophils following their internalization. Intravenous injection of anti-inflammatory peptide encapsulated polymeric NPs reduced neutrophil recruitment and subsequently hyperinflammation to prevent further tissue damage (192). The use of NPs to deliver vaccine/drugs in a controlled fashion is now considered as an attractive approach to develop therapeutic strategies against a range of acute and chronic inflammatory diseases (193).
Adpative Immunity
T and B-cells of the adaptive immune system express a repertoire of receptors to recognize a range of antigens. Activation or suppression of T-cell immunity can determine the fate of a disease. A number of NP based therapeutic strategies have been developed to regulate T-cell activity against viral, bacterial, or fungal infections. For example, antiviral siRNA or retroviral drug encapsulated lipid NPs or dendrimers were effectively delivered to CD4+ T-cells to block HIV replication. This caused a significant reduction in HIV titer when compared with the use of non-encapsulated retroviral drugs (191, 194). T-cell activation also depends up on the type and size of NP used for the delivery of antigen. Liposome encapsulated antigens were better presented to CD4+ T cells by APCs (195, 196) and delivery of 200 nm ova conjugated NPs increased MHC class I and II expression and also produced a higher percentage of antigen specific CD4+T cells as compared to 30 nm ova conjugated particles (197).
B cells are able to recognize and respond to the microbial surface antigens through B-cell receptors (198). Activation and clonal expansion of antigen specific B-cells using engineered NPs have been exploited for the development of vaccines against different diseases (Figure 2). Encapsulation of antigen in virus like particles (VLPs) was able to induce strong and durable humoral responses when compared with the administration of exposed vaccine molecules (199). The potency of immune responses also depends upon the mode of antigen presentation to the target cells. Surface conjugated immunogenic proteins and peptides were able to activate B cells much stronger than encapsulated antigens (200). A single dose of PLGA NPs with surface displayed ovalbumin (OVA) elicited strong antibody responses in vivo as compared to free OVA (201, 202). NPs can also be used to activate specific immune responses. A study has shown that peptide conjugated carbon nanotubes showed significant antigen specific IgG response in comparison to peptide or adjuvant alone (83).
Nanoparticles Can Be Used to Increase Cross Antigen Presentation
In general, antigens captured by APCs from the extracellular environment are targeted to the endo-lysosomal compartments, where they are first processed into peptides and then loaded onto class II MHC molecules before presentation to CD4+ helper T cells. However, cytosolic antigens are loaded on MHC class I molecules and presented to CD8+ T-cells, which are crucial for the clearance of viral and intracellular infections (203). It is reported that some fraction of antigens delivered through NPs are trafficked to cytosolic vacuoles of APCs and presented by MHC class I molecules (203–205). The NP mediated cross antigen presentation was first demonstrated in antigens conjugated to iron oxide polymer NPs (206–209). In addition, inorganic and polymeric NPs have also been used for antigen delivery to cytosol (210,–212). In this context, lipid NPs were shown to induce CD8+ T cell expansion by efficient antigen cross presentation against viral infection in in-vivo models (102, 213). Similarly, invariant natural killer T cells (iNKT), which are a special subset of T-cells, recognize lipid antigens presented by CD1d cells. PLGA NPs conjugated with α-galactosylceramide glycolipid, an iNKT cell stimulant, increased cytokine release as well as expansion of antigen specific CD8+ T cells (214). The cross antigen presentation also depends upon the particle-antigen linkages. It has been shown that disulfide bonding between NP and antigens caused release of antigens into the endosomal compartment and also enhanced CD8+ T cell formation as compared to non-degradable linkers (215, 216). Similarly, pulmonary administration of NPs efficiently enhanced cross antigen presentation, which resulted in at least 10-fold more effector CD8+T cells in lungs (217).
Nanoparticles As Adjuvants to Generate Immune Responses in Lymphoid Organs
Adjuvants are known to enhance and prolong the immune responses against antigens. Delivery of adjuvants and antigens using NPs have been found useful to prolong their exposure in the lymphoid organs such as lymph nodes to generate robust immune responses. This is especially important for small adjuvant molecules, which are rapidly cleared from the bloodstream. NPs with a size ranging from 10–100 nm can penetrate the extracellular matrix and travel to the lymph nodes where they can be internalized by the resident macrophages to activate T-cell responses (218–220). The bio-distribution of NPs also depends upon the route of administration and size. It was observed that larger particles accumulated near the site of NPs and were subsequently endocytosed by the local APCs (160), whereas the smaller NPs drained to the blood capillaries (158, 218). PEG coated liposomes of 80–90 nm diameter showed higher accumulation in lymph nodes after subcutaneous administration as compared to intravenous and intraperitoneal administration (221).
Conclusions
The nano-immuno formulations can improve the antigen stability, targeted delivery and also enhance their immunogenicity properties. Most soluble antigens cannot be efficiently endocytosed by the APCs and hence are poorly effective in inducing protective immunity. The immunogenicity of such soluble vaccine antigens can be improved by conjugating them with nanocarriers that can facilitate the recognition and uptake by APCs. This strategy has already been proved effective for inducing/increasing the immunogenicity of poorly immunogenic antigens, such as polysaccharides of pneumococcal vaccines (222). In the last few years, the application of nanotechnology in the field of immune engineering is growing rapidly with a number of new carrier synthesis strategies. Furthermore, novel nano formulations also contain immunostimulatory molecules to enhance the adjuvant properties of the nanoparticles. Co-encapsulation of the TLR agonists [e.g., CpG, poly(I:C)] (77) or imiquimoid (78) into dextran or chitosan NPs, respectively enhanced receptor-based recognition of the nanovaccines with subsequent cell activation. The recent study by Margaroni et al. showed that vaccination with poly(D,L-lactide-co-glycolide; PLGA) nanoparticles with Leishmania infantum antigens (sLiAg) and surface-modified with a TNFα-mimicking eight-amino-acid peptide (p8) induced significant protection against parasite infection in BALB/c mice accompanied by activation of CD8+ T cells and increase in IFNγ production (223).
Additionally, NPs can be tailored for non-invasive administration and prolonged delivery of the vaccine antigens to a specific location, thus providing the possibility for formulation of the single dose vaccine. Several studies clearly demonstrated the efficacy of the non-invasively administered vaccines such as intranasal application of influenza nano vaccine (224), chitosan NPs with hemagglutinin protein of H1N1 influenza virus (225), Streptococcus equi proteins (226), hepatitis B surface antigen (pRc/CMV-HBs) (227) and plasmid encoding a multi-epitope protein against M. tuberculosis (pHSP65pep) (228) or antigen 85B (229) were used to provide protective immunity against infections. These considerations can improve the progress of ongoing strategies in the development of nanoparticle-based vaccines. In future, development of nanovaccines will address not only the possibility to induce the immune response but also the anti-infective therapeutic activity of NPs thus representing the feasibility to apply multifunctional particles for the treatment of diseases.
Author Contributions
AS and RP wrote the manuscript. AS supervised the process. MS wrote the part on Use of nanocarriers in vaccine delivery to dendritic cells.
Funding
For financial support, we thank Department of Science and Technology (SR/NM/NS-1159/2016), Govt of India and Alexander von Humboldt Fellowship to AS. MS was supported by the Alexander von Humboldt Fellowship and by a grant of the Russian Science Foundation 14-50-00068 and by the Federal Agency of Scientific Organizations, Russia.
Conflict of Interest Statement
The authors declare that the research was conducted in the absence of any commercial or financial relationships that could be construed as a potential conflict of interest.
Acknowledgments
The authors thank Nan-Jong Lee for figures preparation.
References
1. Dye C. After 2015: infectious diseases in a new era of health and development. Philos Trans R Soc Lond B Biol Sci. (2014) 369:20130426. doi: 10.1098/rstb.2013.0426
3. Kahn RE, Ma W, Richt JA. Swine and influenza: a challenge to one health research, in Curr Top Microbiol Immunol. (2014) 385:205–18. doi: 10.1007/82_2014_392
4. Braden CR, Dowell SF, Jernigan DB, Hughes JM. Progress in global surveillance and response capacity 10 years after severe acute respiratory syndrome. Emerg Infect Dis. (2013) 19:864–9. doi: 10.3201/eid1906.130192
5. Wejse C, Patsche CB, Kühle A, Bamba FJV, Mendes MS, Lemvik G, et al. Impact of HIV-1, HIV-2, and HIV-1+2 dual infection on the outcome of tuberculosis. Int J Infect Dis. (2015) 32:128–34. doi: 10.1016/j.ijid.2014.12.015
6. Greenwood B. The contribution of vaccination to global health: past, present and future. Philos Trans R Soc B Biol Sci. (2014) 369:20130433. doi: 10.1098/rstb.2013.0433
8. Atkins HS, Morton M, Griffin KF, Stokes MGM, Nataro JP, Titball RW. Recombinant Salmonella vaccines for biodefence. Vaccine (2006) 24:2710–7. doi: 10.1016/j.vaccine.2005.12.046
10. Ulmer JB, Donnelly JJ, Parker SE, Rhodes GH, Felgner PL, Dwarki VJ, et al. Heterologous protection against influenza by injection of DNA encoding a viral protein. Science (1993) 259:1745–9. doi: 10.1126/science.8456302
11. Scallan CD, Tingley DW, Lindbloom JD, Toomey JS, Tucker SN. An adenovirus-based vaccine with a double-stranded RNA adjuvant protects mice and ferrets against H5N1 avian influenza in oral delivery models. Clin Vaccine Immunol. (2013) 20:85–94. doi: 10.1128/CVI.00552-12
12. Altenburg AF, Kreijtz JH, de Vries RD, Song F, Fux R, Rimmelzwaan GF, et al. Modified vaccinia virus ankara (MVA) as production platform forvaccines against influenza and other viral respiratory diseases. Viruses (2014) 6:2735–61. doi: 10.3390/v6072735
13. Nascimento IP, Leite LCC. Recombinant vaccines and the development of new vaccine strategies. Braz J Med Biol Res. (2012) 45:1102–11. doi: 10.1590/S0100-879X2012007500142
14. Donnelly JJ, Wahren B, Liu MA. DNA vaccines: progress and challenges. J Immunol. (2005) 175:633–9. doi: 10.4049/jimmunol.175.2.633
15. Skibinski DA, Baudner BC, Singh M, O'Hagan DT. Combination vaccines. J Glob Infect Dis. (2011) 3:63–72. doi: 10.4103/0974-777X.77298
16. Huber VC. Influenza vaccines: from whole virus preparations to recombinant protein technology. Expert Rev Vaccines. (2014) 13:31–42. doi: 10.1586/14760584.2014.852476
17. Means TK, Hayashi F, Smith KD, Aderem A, Luster AD. The Toll-like receptor 5 stimulus bacterial flagellin induces maturation and chemokine production in human dendritic cells. J Immunol. (2003) 170:5165–75. doi: 10.4049/jimmunol.170.10.5165
18. Mogensen TH. Pathogen recognition and inflammatory signaling in innate immune defenses. Clin Microbiol Rev. (2009) 22:240–73. doi: 10.1128/CMR.00046-08
19. Randolph GJ, Ochando J, Partida-Sánchez S. Migration of dendritic cell subsets and their precursors. Annu Rev Immunol. (2008) 26:293–316. doi: 10.1146/annurev.immunol.26.021607.090254
20. Smith DM, Simon JK, Baker JR. Applications of nanotechnology for immunology. Nat Rev Immunol. (2013) 13:592–605. doi: 10.1038/nri3488
21. Fifis T, Gamvrellis A, Crimeen-Irwin B, Pietersz GA, Li J, Mottram PL, et al. Size-dependent immunogenicity: therapeutic and protective properties of nano-vaccines against tumors. J Immunol. (2004) 173:3148–54. doi: 10.4049/jimmunol.173.5.3148
22. Mottram PL, Leong D, Crimeen-Irwin B, Gloster S, Xiang SD, Meanger J, et al. Type 1 and 2 immunity following vaccination is influenced by nanoparticle size: formulation of a model vaccine for respiratory syncytial virus. Mol Pharm. (2007) 4:73–84. doi: 10.1021/mp060096p
23. Schöler N, Hahn H, Müller RH, Liesenfeld O. Effect of lipid matrix and size of solid lipid nanoparticles (SLN) on the viability and cytokine production of macrophages. Int J Pharm. (2002) 231:167–76. doi: 10.1016/S0378-5173(01)00882-1
24. Schöler N, Olbrich C, Tabatt K, Müller RH, Hahn H, Liesenfeld O. Surfactant, but not the size of solid lipid nanoparticles (SLN) influences viability and cytokine production of macrophages. Int J Pharm. (2001) 221:57–67. doi: 10.1016/S0378-5173(01)00660-3
25. Vallhov H, Qin J, Johansson SM, Ahlborg N, Muhammed MA, Scheynius A, et al. The importance of an endotoxin-free environment during the production of nanoparticles used in medical applications. Nano Lett. (2006) 6:1682–6. doi: 10.1021/nl060860z
26. Shvedova AA, Kisin ER, Mercer R, Murray AR, Johnson VJ, Potapovich AI, et al. Unusual inflammatory and fibrogenic pulmonary responses to single-walled carbon nanotubes in mice. Am J Physiol Cell Mol Physiol. (2005) 289:L698–708. doi: 10.1152/ajplung.00084.2005
27. Wang X, Ishida T, Kiwada H. Anti-PEG IgM elicited by injection of liposomes is involved in the enhanced blood clearance of a subsequent dose of PEGylated liposomes. J Control Release (2007) 119:236–44. doi: 10.1016/j.jconrel.2007.02.010
28. Ishida T, Wang X, Shimizu T, Nawata K, Kiwada H. PEGylated liposomes elicit an anti-PEG IgM response in a T cell-independent manner. J Control Release (2007) 122:349–55. doi: 10.1016/j.jconrel.2007.05.015
29. Irvine DJ, Swartz MA, Szeto GL. Engineering synthetic vaccines using cues from natural immunity. Nat Mater. (2013) 12:978–90. doi: 10.1038/nmat3775
30. Pusic K, Aguilar Z, McLoughlin J, Kobuch S, Xu H, Tsang M, et al. Iron oxide nanoparticles as a clinically acceptable delivery platform for a recombinant blood-stage human malaria vaccine. FASEB J. (2013) 27:1153–66. doi: 10.1096/fj.12-218362
31. Lim JS, Lee K, Choi JN, Hwang YK, Yun MY, Kim HJ, et al. Intracellular protein delivery by hollow mesoporous silica capsules with a large surface hole. Nanotechnology (2012) 23:85101. doi: 10.1088/0957-4484/23/8/085101
32. Akagi T, Wang X, Uto T, Baba M, Akashi M. Protein direct delivery to dendritic cells using nanoparticles based on amphiphilic poly(amino acid) derivatives. Biomaterials (2007) 28:3427–36. doi: 10.1016/j.biomaterials.2007.04.023
33. Prego C, Paolicelli P, Díaz B, Vicente S, Sánchez A, González-Fernández Á, et al. Chitosan-based nanoparticles for improving immunization against hepatitis B infection. Vaccine (2010) 28:2607–14. doi: 10.1016/j.vaccine.2010.01.011
34. Shen H, Ackerman AL, Cody V, Giodini A, Hinson ER, Cresswell P, et al. Enhanced and prolonged cross-presentation following endosomal escape of exogenous antigens encapsulated in biodegradable nanoparticles. Immunology (2006) 117:78–88. doi: 10.1111/j.1365-2567.2005.02268.x
35. Diwan M, Tafaghodi M, Samuel J. Enhancement of immune responses by co-delivery of a CpG oligodeoxynucleotide and tetanus toxoid in biodegradable nanospheres. J Control Release (2002) 85:247–62. doi: 10.1016/S0168-3659(02)00275-4
36. Mintern JD, Percival C, Kamphuis MMJ, Chin WJ, Caruso F, Johnston APR. Targeting dendritic cells: the role of specific receptors in the internalization of polymer capsules. Adv Healthc Mater. (2013) 2:940–4. doi: 10.1002/adhm.201200441
37. Wang X, Uto T, Akagi T, Akashi M, Baba M. Induction of potent CD8+ T-cell responses by novel biodegradable nanoparticles carrying human immunodeficiency virus type 1 gp120. J Virol. (2007) 81:10009–16. doi: 10.1128/JVI.00489-07
38. Richards RL, Rao M, Wassef NM, Glenn GM, Rothwell SW, Alving CR. Liposomes containing lipid A serve as an adjuvant for induction of antibody and cytotoxic T-cell responses against RTS,S malaria antigen. Infect Immun. (1998) 66:2859–65.
39. Tyler M, Tumban E, Peabody DS, Chackerian B. The use of hybrid virus-like particles to enhance the immunogenicity of a broadly protective HPV vaccine. Biotechnol Bioeng. (2014) 111:2398–406. doi: 10.1002/bit.25311
40. Slupetzky K, Gambhira R, Culp TD, Shafti-Keramat S, Schellenbacher C, Christensen ND, et al. A papillomavirus-like particle (VLP) vaccine displaying HPV16 L2 epitopes induces cross-neutralizing antibodies to HPV11. Vaccine (2007) 25:2001–10. doi: 10.1016/j.vaccine.2006.11.049
41. Manish M, Rahi A, Kaur M, Bhatnagar R, Singh S. A single-dose PLGA encapsulated protective antigen domain 4 nanoformulation protects mice against Bacillus anthracis spore challenge. PLoS ONE (2013) 8:e61885. doi: 10.1371/journal.pone.0061885
42. Feng G, Jiang Q, Xia M, Lu Y, Qiu W, Zhao D, et al. Enhanced immune response and protective effects of nano-chitosan-based DNA vaccine encoding T cell epitopes of Esat-6 and FL against Mycobacterium Tuberculosis infection. PLoS ONE (2013) 8:e61135. doi: 10.1371/journal.pone.0061135
43. Das I, Padhi A, Mukherjee S, Dash DP, Kar S, Sonawane A. Biocompatible chitosan nanoparticles as an efficient delivery vehicle for Mycobacterium tuberculosis lipids to induce potent cytokines and antibody response through activation of γδ T cells in mice. Nanotechnology (2017) 28:165101. doi: 10.1088/1361-6528/aa60fd
44. Abraham E. Intranasal immunization with bacterial polysaccharide containing liposomes enhances antigen-specific pulmonary secretory antibody response. Vaccine (1992) 10:461–8. doi: 10.1016/0264-410X(92)90395-Z
45. Alving CR, Richards RL, Moss J, Alving LI, Clements JD, Shiba T, et al. Effectiveness of liposomes as potential carriers of vaccines: applications to cholera toxin and human malaria sporozoite antigen. Vaccine (1986) 4:166–72. doi: 10.1016/0264-410X(86)90005-8
46. Zhao W, Wu W, Xu X. Oral vaccination with liposome-encapsulated recombinant fusion peptide of urease B epitope and cholera toxin B subunit affords prophylactic and therapeutic effects against H. pylori infection in BALB/c mice. Vaccine (2007) 25:7664–73. doi: 10.1016/j.vaccine.2007.08.034
47. Makidon PE, Knowlton J, Groom J V, Blanco LP, LiPuma JJ, Bielinska AU, et al. Induction of immune response to the 17 kDa OMPA Burkholderia cenocepacia polypeptide and protection against pulmonary infection in mice after nasal vaccination with an OMP nanoemulsion-based vaccine. Med Microbiol Immunol. (2010) 199:81–92. doi: 10.1007/s00430-009-0137-2
48. Bielinska AU, Janczak KW, Landers JJ, Makidon P, Sower LE, Peterson JW, et al. Mucosal immunization with a novel nanoemulsion-based recombinant anthrax protective antigen vaccine protects against Bacillus anthracis spore challenge. Infect Immun. (2007) 75:4020–9. doi: 10.1128/IAI.00070-07
49. Kamath AT, Rochat AF, Christensen D, Agger EM, Andersen P, Lambert PH, et al. A liposome-based mycobacterial vaccine induces potent adult and neonatal multifunctional T cells through the exquisite targeting of dendritic cells. PLoS ONE (2009) 4:e5771. doi: 10.1371/journal.pone.0005771
50. Chen YS, Hung YC, Lin WH, Huang GS. Assessment of gold nanoparticles as a size-dependent vaccine carrier for enhancing the antibody response against synthetic foot-and-mouth disease virus peptide. Nanotechnology (2010) 21:195101. doi: 10.1088/0957-4484/21/19/195101
51. Tao W, Gill HS. M2e-immobilized gold nanoparticles as influenza A vaccine: role of soluble M2e and longevity of protection. Vaccine (2015) 33:2307–2315. doi: 10.1016/j.vaccine.2015.03.063
52. Xu L, Liu Y, Chen Z, Li W, Liu Y, Wang L, et al. Surface-engineered gold nanorods: promising DNA vaccine adjuvant for HIV-1 treatment. Nano Lett. (2012) 12:2003–12. doi: 10.1021/nl300027p
53. Raghuvanshi RS, Katare YK, Lalwani K, Ali MM, Singh O, Panda AK. Improved immune response from biodegradable polymer particles entrapping tetanus toxoid by use of different immunization protocol and adjuvants. Int J Pharm. (2002) 245:109–21. doi: 10.1016/S0378-5173(02)00342-3
54. Thomas C, Rawat A, Hope-Weeks L, Ahsan F. Aerosolized PLA and PLGA nanoparticles enhance humoral mucosal and cytokine responses to hepatitis B vaccine. Mol Pharm. (2011) 8:405–15. doi: 10.1021/mp100255c
55. Borges O, Cordeiro-da-Silva A, Tavares J, Santarém N, de Sousa A, Borchard G, et al. Immune response by nasal delivery of hepatitis B surface antigen and codelivery of a CpG ODN in alginate coated chitosan nanoparticles. Eur J Pharm Biopharm. (2008) 69:405–16. doi: 10.1016/j.ejpb.2008.01.019
56. Zhao K, Chen G, Shi X, Gao T, Li W, Zhao Y, et al. Preparation and efficacy of a live newcastle disease virus vaccine encapsulated in chitosan nanoparticles. PLoS ONE (2012) 7:e53314. doi: 10.1371/journal.pone.0053314
57. Ball JM, Hardy ME, Atmar RL, Conner ME, Estes MK. Oral immunization with recombinant Norwalk virus-like particles induces a systemic and mucosal immune response in mice. J Virol. (1998) 72:1345–53.
58. Ball JM, Graham DY, Opekun AR, Gilger MA, Guerrero RA, Estes MK. Recombinant Norwalk virus-like particles given orally to volunteers: phase I study. Gastroenterology (1999) 117:40–8. doi: 10.1016/S0016-5085(99)70548-2
59. Bright RA, Carter DM, Daniluk S, Toapanta FR, Ahmad A, Gavrilov V, et al. Influenza virus-like particles elicit broader immune responses than whole virion inactivated influenza virus or recombinant hemagglutinin. Vaccine (2007) 25:3871–8. doi: 10.1016/j.vaccine.2007.01.106
60. Quan FS, Huang C, Compans RW, Kang SM. Virus-like particle vaccine induces protective immunity against homologous and heterologous strains of influenza virus. J Virol. (2007) 81:3514–24. doi: 10.1128/JVI.02052-06
61. Matassov D, Cupo A, Galarza JM. A novel intranasal virus-like particle (VLP) vaccine designed to protect against the pandemic 1918 influenza A virus (H1N1). Viral Immunol. (2007) 20:441–52. doi: 10.1089/vim.2007.0027
62. Bright RA, Carter DM, Crevar CJ, Toapanta FR, Steckbeck JD, Cole KS, et al. Cross-clade protective immune responses to influenza viruses with H5N1 HA and NA elicited by an influenza virus-like particle. PLoS ONE (2008) 3:e1501. doi: 10.1371/journal.pone.0001501
63. Mahmood K, Bright RA, Mytle N, Carter DM, Crevar CJ, Achenbach JE, et al. H5N1 VLP vaccine induced protection in ferrets against lethal challenge with highly pathogenic H5N1 influenza viruses. Vaccine (2008) 26:5393–9. doi: 10.1016/j.vaccine.2008.07.084
64. Guo L, Lu X, Kang SM, Chen C, Compans RW, Yao Q. Enhancement of mucosal immune responses by chimeric influenza HA/SHIV virus-like particles. Virology (2003) 313:502–13. doi: 10.1016/S0042-6822(03)00372-6
65. Geldmacher A, Skrastina D, Borisova G, Petrovskis I, Krüger DH, Pumpens P et al. A hantavirus nucleocapsid protein segment exposed on hepatitis B virus core particles is highly immunogenic in mice when applied without adjuvants or in the presence of pre-existing anti-core antibodies. Vaccine (2005) 23:3973–83. doi: 10.1016/j.vaccine.2005.02.025
66. Sadeyen JR, Tourne S, Shkreli M, Sizaret PY, Coursaget P. Insertion of a foreign sequence on capsid surface loops of human papillomavirus type 16 virus-like particles reduces their capacity to induce neutralizing antibodies and delineates a conformational neutralizing epitope. Virology (2003) 309:32–40. doi: 10.1016/S0042-6822(02)00134-4
67. Paz De la Rosa G, Monroy-García A, Mora-García M, de L, Peña CGR, Hernández-Montes J, Weiss-Steider B, et al. An HPV 16 L1-based chimeric human papilloma virus-like particles containing a string of epitopes produced in plants is able to elicit humoral and cytotoxic T-cell activity in mice. Virol J. (2009) 6:2. doi: 10.1186/1743-422X-6-2
68. Oh YK, Sohn T, Park JS, Kang MJ, Choi HG, Kim JA, et al. Enhanced mucosal and systemic immunogenicity of human papillomavirus-like particles encapsidating interleukin-2 gene adjuvant. Virology (2004) 328:266–73. doi: 10.1016/j.virol.2004.06.047
69. O'Neal CM, Crawford SE, Estes MK, Conner ME. Rotavirus virus-like particles administered mucosally induce protective immunity. J Virol. (1997) 71:8707–17.
70. Parez N, Fourgeux C, Mohamed A, Dubuquoy C, Pillot M, Dehee A, et al. Rectal immunization with rotavirus virus-like particles induces systemic and mucosal humoral immune responses and protects mice against rotavirus infection. J Virol. (2006) 80:1752–61. doi: 10.1128/JVI.80.4.1752-1761.2006
71. Roy P, Bishop DH, LeBlois H, Erasmus BJ. Long-lasting protection of sheep against bluetongue challenge after vaccination with virus-like particles: evidence for homologous and partial heterologous protection. Vaccine (1994) 12:805–11. doi: 10.1016/0264-410X(94)90289-5
72. Deml L, Kratochwil G, Osterrieder N, Knüchel R, Wolf H, Wagner R. Increased incorporation of chimeric human immunodeficiency virus type 1 gp120 proteins into Pr55gag virus-like particles by an Epstein-Barr virus gp220/350-derived transmembrane domain. Virology (1997) 235:10–25. doi: 10.1006/viro.1997.8669
73. Crooks ET, Moore PL, Franti M, Cayanan CS, Zhu P, Jiang P, et al. A comparative immunogenicity study of HIV-1 virus-like particles bearing various forms of envelope proteins, particles bearing no envelope and soluble monomeric gp120. Virology (2007) 366:245–62. doi: 10.1016/j.virol.2007.04.033
74. Buonaguro L, Visciano ML, Tornesello ML, Tagliamonte M, Biryahwaho B, Buonaguro FM. Induction of systemic and mucosal cross-clade neutralizing antibodies in BALB/c mice immunized with human immunodeficiency virus type 1 clade A virus-like particles administered by different routes of inoculation. J Virol. (2005) 79:7059–67. doi: 10.1128/JVI.79.11.7059-7067.2005
75. Wang BZ, Liu W, Kang SM, Alam M, Huang C, Ye L, et al. Incorporation of high levels of chimeric human immunodeficiency virus envelope glycoproteins into virus-like particles. J Virol. (2007) 81:10869–78. doi: 10.1128/JVI.00542-07
76. Pimentel TAPF, Yan Z, Jeffers SA, Holmes K V, Hodges RS, Burkhard P. Peptide nanoparticles as novel immunogens: design and analysis of a prototypic severe acute respiratory syndrome vaccine. Chem Biol Drug Des. (2009) 73:53–61. doi: 10.1111/j.1747-0285.2008.00746.x
77. Kaba SA, Brando C, Guo Q, Mittelholzer C, Raman S, Tropel D, et al. A nonadjuvanted polypeptide nanoparticle vaccine confers long-lasting protection against rodent malaria. J Immunol. (2009) 183:7268–77. doi: 10.4049/jimmunol.0901957
78. Kazanji M, Laurent F, Péry P. Immune responses and protective effect in mice vaccinated orally with surface sporozoite protein of Eimeria falciformis in ISCOMs. Vaccine (1994) 12:798–804. doi: 10.1016/0264-410X(94)90288-7
79. Wang T, Zou M, Jiang H, Ji Z, Gao P, Cheng G. Synthesis of a novel kind of carbon nanoparticle with large mesopores and macropores and its application as an oral vaccine adjuvant. Eur J Pharm Sci. (2011) 44:653–9. doi: 10.1016/j.ejps.2011.10.012
80. Zhou X, Zhang X, Yu X, Zha X, Fu Q, Liu B, et al. The effect of conjugation to gold nanoparticles on the ability of low molecular weight chitosan to transfer DNA vaccine. Biomaterials (2008) 29:111–7. doi: 10.1016/j.biomaterials.2007.09.007
81. Turkevich J, Stevenson PC, Hillier J. A study of the nucleation and growth processes in the synthesis of colloidal gold. Discuss Faraday Soc. (1951) 11:55. doi: 10.1039/df9511100055
82. Silva CL, Bonato VLD, Coelho-Castelo AAM, De Souza AO, Santos, SA, Lima KM, et al. Immunotherapy with plasmid DNA encoding mycobacterial hsp65 in association with chemotherapy is a more rapid and efficient form of treatment for tuberculosis in mice. Gene Ther. (2005) 12:281–7. doi: 10.1038/sj.gt.3302418
83. Villa CH, Dao T, Ahearn I, Fehrenbacher N, Casey E, Rey DA, et al. Single-walled carbon nanotubes deliver peptide antigen into dendritic cells and enhance IgG responses to tumor-associated antigens. ACS Nano. (2011) 5:5300–11. doi: 10.1021/nn200182x
84. Yu M, Jambhrunkar S, Thorn P, Chen J, Gu W, et al. Hyaluronic acid modified mesoporous silica nanoparticles for targeted drug delivery to CD44-overexpressing cancer cells. Nanoscale (2013) 5:178–83. doi: 10.1039/C2NR32145A
85. Xia T, Kovochich M, Liong M, Meng H, Kabehie S, George S, et al. Polyethyleneimine coating enhances the cellular uptake of mesoporous silica nanoparticles and allows safe delivery of siRNA and DNA constructs. ACS Nano (2009) 3:3273–86. doi: 10.1021/nn900918w
86. He X, Wang K, Tan W, Liu B, Lin X, et al. Bioconjugated nanoparticles for DNA protection from cleavage. J Am Chem Soc. (2003) 125:7168–9. doi: 10.1021/ja034450d
87. Li X, Deng X, Huang Z. In vitro protein release and degradation of poly-dl-lactide-poly(ethylene glycol) microspheres with entrapped human serum albumin: quantitative evaluation of the factors involved in protein release phases. Pharm Res. (2001) 18:117–24. doi: 10.1023/A:1011043230573
88. Demento SL, Cui W, Criscione JM, Stern E, Tulipan J, Kaech SM, et al. Role of sustained antigen release from nanoparticle vaccines in shaping the T cell memory phenotype. Biomaterials (2012) 33:4957–64. doi: 10.1016/j.biomaterials.2012.03.041
89. Lima VM, Bonato VL, Lima KM, Dos Santos SA, Dos Santos RR, Gonçalves ED, et al. Role of trehalose dimycolate in recruitment of cells and modulation of production of cytokines and NO in tuberculosis. Infect Immun. (2001) 69:5305–12. doi: 10.1128/IAI.69.9.5305-5312.2001
90. Hasegawa K, Noguchi Y, Koizumi F, Uenaka A, Tanaka M, Shimono M, et al. In vitro stimulation of CD8 and CD4 T cells by dendritic cells loaded with a complex of cholesterol-bearing hydrophobized pullulan and NY-ESO-1 protein: identification of a new HLA-DR15-binding CD4 T-cell epitope. Clin Cancer Res. (2006) 12:1921–7. doi: 10.1158/1078-0432.CCR-05-1900
91. Li P, Luo Z, Liu P, Gao N, Zhang Y, Pan H, et al. Bioreducible alginate-poly(ethylenimine) nanogels as an antigen-delivery system robustly enhance vaccine-elicited humoral and cellular immune responses. J Control Release (2013) 168:271–9. doi: 10.1016/j.jconrel.2013.03.025
92. Honda-Okubo Y, Saade F, Petrovsky N. AdvaxTM, a polysaccharide adjuvant derived from delta inulin, provides improved influenza vaccine protection through broad-based enhancement of adaptive immune responses. Vaccine (2012) 30:5373–81. doi: 10.1016/j.vaccine.2012.06.021
93. Saade F, Honda-Okubo Y, Trec S, Petrovsky N. A novel hepatitis B vaccine containing AdvaxTM, a polysaccharide adjuvant derived from delta inulin, induces robust humoral and cellular immunity with minimal reactogenicity in preclinical testing. Vaccine (2013) 31:1999–2007. doi: 10.1016/j.vaccine.2012.12.077
94. Götze O, Müller-Eberhard HJ. The C3-activator system: an alternate pathway of complement activation. J Exp Med. (1971) 134:90s−108s.
95. Pawar D, Mangal S, Goswami R, Jaganathan KS. Development and characterization of surface modified PLGA nanoparticles for nasal vaccine delivery: effect of mucoadhesive coating on antigen uptake and immune adjuvant activity. Eur J Pharm Biopharm. (2013) 85:550–9. doi: 10.1016/j.ejpb.2013.06.017
96. Sonaje K, Chuang EY, Lin KJ, Yen TC, Su FY, et al. Opening of epithelial tight junctions and enhancement of paracellular permeation by chitosan: microscopic, ultrastructural, and computed-tomographic observations. Mol Pharm. (2012) 9:1271–9. doi: 10.1021/mp200572t
97. de Titta A, Ballester M, Julier Z, Nembrini C, Jeanbart L, van der Vlies AJ, et al. Nanoparticle conjugation of CpG enhances adjuvancy for cellular immunity and memory recall at low dose. Proc Natl Acad Sci USA. (2013) 110:19902–7. doi: 10.1073/pnas.1313152110
98. Mohammed MA, Syeda JTM, Wasan KM, Wasan EK. An overview of chitosan nanoparticles and its application in non-parenteral drug delivery. Pharmaceutics (2017) 9:53. doi: 10.3390/pharmaceutics9040053
99. Sharma A. Liposomes in drug delivery: Progress and limitations. Int J Pharm. (1997) 154:123–40. doi: 10.1016/S0378-5173(97)00135-X
100. Storm G, Crommelin DJ. Liposomes: quo vadis? Pharm Sci Technolo Today (1998) 1:19–31. doi: 10.1016/S1461-5347(98)00007-8
101. Tyagi RK, Garg NK, Sahu T. Vaccination strategies against malaria: novel carrier(s) more than a tour de force. J Control Release (2012) 162:242–54. doi: 10.1016/j.jconrel.2012.04.037
102. Moon JJ, Suh H, Bershteyn A, Stephan MT, Liu H, Huang B, et al. Interbilayer-crosslinked multilamellar vesicles as synthetic vaccines for potent humoral and cellular immune responses. Nat Mater. (2011) 10:243–51. doi: 10.1038/nmat2960
103. Ichihashi T, Satoh T, Sugimoto C, Kajino K. Emulsified phosphatidylserine, simple and effective peptide carrier for induction of potent epitope-specific T cell responses. PLoS ONE (2013) 8:e60068. doi: 10.1371/journal.pone.0060068
104. Ribeiro AM, Souza ACO, Amaral AC, Vasconcelos NM, Jeronimo MS, Carneiro FP, et al. Nanobiotechnological approaches to delivery of DNA vaccine against fungal infection. J Biomed Nanotechnol. (2013) 9:221–30. doi: 10.1166/jbn.2013.1491
105. Watson DS, Endsley AN, Huang L. Design considerations for liposomal vaccines: influence of formulation parameters on antibody and cell-mediated immune responses to liposome associated antigens. Vaccine (2012) 30:2256–72. doi: 10.1016/j.vaccine.2012.01.070
106. Vyas SP, Kannan ME, Jain S, Mishra V, Singh P. Design of liposomal aerosols for improved delivery of rifampicin to alveolar macrophages. Int J Pharm. (2004) 269:37–49. doi: 10.1016/j.ijpharm.2003.08.017
107. Vyas S, Quraishi S, Gupta S, Jaganathan K. Aerosolized liposome-based delivery of amphotericin B to alveolar macrophages. Int J Pharm. (2005) 296:12–25. doi: 10.1016/j.ijpharm.2005.02.003
108. Joseph A, Itskovitz-Cooper N, Samira S, Flasterstein O, Eliyahu H, Simberg D, et al. A new intranasal influenza vaccine based on a novel polycationic lipid–ceramide carbamoyl-spermine (CCS) I. Immunogenicity and efficacy studies in mice. Vaccine (2006) 24:3990–4006. doi: 10.1016/j.vaccine.2005.12.017
109. Postma NS, Hermsen CC, Zuidema J, Eling WM. Plasmodium vinckei: optimization of desferrioxamine B delivery in the treatment of murine malaria. Exp Parasitol. (1998) 89:323–30. doi: 10.1006/expr.1998.4282
110. Christensen D, Korsholm KS, Andersen P, Agger EM. Cationic liposomes as vaccine adjuvants. Expert Rev Vaccines (2011) 10:513–21. doi: 10.1586/erv.11.17
111. McNeil SE, Perrie Y. Gene delivery using cationic liposomes. Expert Opin Ther Pat. (2006) 16:1371–82. doi: 10.1517/13543776.16.10.1371
112. Alving CR, Beck Z, Matyas GR, Rao M. Liposomal adjuvants for human vaccines. Expert Opin Drug Deliv. (2016) 13:807–16. doi: 10.1517/17425247.2016.1151871
113. Kingsman SM, Kingsman AJ. Polyvalent recombinant antigens: a new vaccine strategy. Vaccine (1988) 6:304–6. doi: 10.1016/0264-410X(88)90174-0
114. Roldão A, Mellado MCM, Castilho LR, Carrondo MJ, Alves PM. Virus-like particles in vaccine development. Expert Rev Vaccines (2010) 9:1149–76. doi: 10.1586/erv.10.115
115. Zeltins A. Construction and characterization of virus-like particles: a review. Mol Biotechnol. (2013) 53:92–107. doi: 10.1007/s12033-012-9598-4
116. Grgacic EVL, Anderson DA. Virus-like particles: passport to immune recognition. Methods (2006) 40:60–5. doi: 10.1016/j.ymeth.2006.07.018
117. Strable E, Finn MG. Chemical modification of viruses and virus-like particles. Curr Top Microbiol Immunol. (2009) 327:1–21. doi: 10.1007/978-3-540-69379-6_1
118. Maurer P, Jennings GT, Willers J, Rohner F, Lindman Y, Roubicek K, et al. A therapeutic vaccine for nicotine dependence: preclinical efficacy, and Phase I safety and immunogenicity. Eur J Immunol. (2005) 35:2031–40. doi: 10.1002/eji.200526285
119. Patel KG, Swartz JR. Surface functionalization of virus-like particles by direct conjugation using azide–alkyne click chemistry. Bioconjug Chem. (2011) 22:376–87. doi: 10.1021/bc100367u
120. Kawano M, Matsui M, Handa H. SV40 virus-like particles as an effective delivery system and its application to a vaccine carrier. Expert Rev Vaccines (2013) 12:199–210. doi: 10.1586/erv.12.149
121. Tissot AC, Renhofa R, Schmitz N, Cielens I, Meijerink E, Ose V, et al. Versatile virus-like particle carrier for epitope based vaccines. PLoS ONE (2010) 5:e9809. doi: 10.1371/journal.pone.0009809
122. Gao Y, Wijewardhana C, Mann JFS. Virus-like particle, liposome, and polymeric particle-based vaccines against HIV-1. Front Immunol. (2018) 9:345. doi: 10.3389/fimmu.2018.00345
123. Chahal JS, Khan OF, Cooper CL, McPartlan JS, Tsosie JK, Tilley LD, et al. Dendrimer-RNA nanoparticles generate protective immunity against lethal Ebola, H1N1 influenza, and Toxoplasma gondii challenges with a single dose. Proc Natl Acad Sci USA. (2016) 113:E4133–42. doi: 10.1073/pnas.1600299113
124. Bahadoran A, Moeini H, Bejo MH, Hussein MZ, Omar AR. Development of Tat-conjugated dendrimer for transdermal DNA vaccine delivery. J Pharm Pharm Sci. (2016) 19:325–38. doi: 10.18433/J3G31Q
125. Jaffer U, Wade RG, Gourlay T. Cytokines in the systemic inflammatory response syndrome: a review. HSR Proc Intensive Care Cardiovasc Anesth. (2010) 2:161–75.
126. Hora MS, Rana RK, Nunberg JH, Tice TR, Gilley RM, Hudson ME. Controlled release of interleukin-2 from biodegradable microspheres. Biotechnology (1990) 8:755–8.
127. Melissen PM, van Vianen W, Bidjai O, van Marion M, Bakker-Woudenberg IA. Free versus liposome-encapsulated muramyl tripeptide phosphatidylethanolamide (MTPPE) and interferon-y (IFN-y) in experimental infection with Listeria monocytogenes. Biotherapy (1993) 6:113–24. doi: 10.1007/BF01877424
128. Ali OA, Huebsch N, Cao L, Dranoff G, Mooney DJ. Infection-mimicking materials to program dendritic cells in situ. Nat Mater. (2009) 8:151–58. doi: 10.1038/nmat2357
129. Killion JJ, Fishbeck R, Bar-Eli M, Chernajovsky Y. Delivery of interferon to intracellular pathways by encapsulation of interferon into multilamellar liposomes is independent of the status of interferon receptors. Cytokine (1994) 6:443–9. doi: 10.1016/1043-4666(94)90069-8
130. Ha SJ, Park SH, Kim HJ, Kim SC, Kang HJ, Lee EG, et al. Enhanced immunogenicity and protective efficacy with the use of interleukin-12-encapsulated microspheres plus AS01B in tuberculosis subunit vaccination. Infect Immun. (2006) 74:4954–9. doi: 10.1128/IAI.01781-05
131. Kawai T, Akira S. Toll-like receptors and their crosstalk with other innate receptors in infection and immunity. Immunity (2011) 34:637–50. doi: 10.1016/j.immuni.2011.05.006
132. Schenten D, Medzhitov R. The control of adaptive immune responses by the innate immune system. Adv Immunol. (2011) 109:87–124. doi: 10.1016/B978-0-12-387664-5.00003-0
133. Lynn GM, Laga R, Darrah PA, Ishizuka AS, Balaci AJ, Dulcey AE, et al. In vivo characterization of the physicochemical properties of polymer-linked TLR agonists that enhance vaccine immunogenicity. Nat Biotechnol. (2015) 33:1201–10. doi: 10.1038/nbt.3371
134. Goldinger SM, Dummer R, Baumgaertner P, Mihic-Probst D, Schwarz K, Hammann-Haenni A, et al. Nano-particle vaccination combined with TLR-7 and−9 ligands triggers memory and effector CD8+ T-cell responses in melanoma patients. Eur J Immunol. (2012) 42:3049–61. doi: 10.1002/eji.201142361
135. Dowling DJ, Scott EA, Scheid A, Bergelson I, Joshi S, Pietrasanta C, et al. Toll-like receptor 8 agonist nanoparticles mimic immunomodulating effects of the live BCG vaccine and enhance neonatal innate and adaptive immune responses. J Allergy Clin Immunol. (2017) 140:1339–50. doi: 10.1016/j.jaci.2016.12.985
136. Moradi B, Sankian M, Amini Y, Meshkat Z. Construction of a novel DNA vaccine candidate encoding an HspX-PPE44-EsxV fusion antigen of Mycobacterium tuberculosis. Reports Biochem Mol Biol. (2016) 4:89–97.
137. Xue T, Stavropoulos E, Yang M, Ragno S, Vordermeier M, Chambers M, et al. RNA encoding the MPT83 antigen induces protective immune responses against Mycobacterium tuberculosis infection. Infect Immun. (2004) 72:6324–9. doi: 10.1128/IAI.72.11.6324-6329.2004
138. Romalde JL, Luzardo-Alvárez A, Ravelo C, Toranzo AE, Blanco-Méndez J. Oral immunization using alginate microparticles as a useful strategy for booster vaccination against fish lactoccocosis. Aquaculture (2004) 236:119–29. doi: 10.1016/j.aquaculture.2004.02.028
139. Wendorf J, Singh M, Chesko J, Kazzaz J, Soewanan E, Ugozzoli M, et al. A practical approach to the use of nanoparticles for vaccine delivery. J Pharm Sci. (2006) 95:2738–50. doi: 10.1002/jps.20728
140. Stieneker F, Kreuter J, Löwer J. High antibody titres in mice with polymethylmethacrylate nanoparticles as adjuvant for HIV vaccines. AIDS (1991) 5:431–5.
141. He Q, Mitchell AR, Johnson SL, Wagner-Bartak C, Morcol T, Bell SJ. Calcium phosphate nanoparticle adjuvant. Clin Diagn Lab Immunol. (2000) 7:899–903. doi: 10.1128/CDLI.7.6.899-903.2000
142. Oyewumi MO, Kumar A, Cui Z. Nano-microparticles as immune adjuvants: correlating particle sizes and the resultant immune responses. Expert Rev Vaccines (2010) 9:1095–107. doi: 10.1586/erv.10.89
143. Biabanikhankahdani R, Alitheen NBM, Ho KL, Tan WS. pH-responsive virus-like nanoparticles with enhanced tumour-targeting ligands for cancer drug delivery. Sci Rep. (2016) 6:37891. doi: 10.1038/srep37891
144. Slütter B, Soema PC, Ding Z, Verheul R, Hennink W, Jiskoot W. Conjugation of ovalbumin to trimethyl chitosan improves immunogenicity of the antigen. J Control Release (2010) 143:207–14. doi: 10.1016/j.jconrel.2010.01.007
145. Giddam AK, Giddam AK, Zaman M, Skwarczynski M, Toth I. Liposome-based delivery system for vaccine candidates: constructing an effective formulation. Nanomedicine (2012) 7:1877–93. doi: 10.2217/nnm.12.157
146. Glück R, Moser C, Metcalfe IC. Influenza virosomes as an efficient system for adjuvanted vaccine delivery. Expert Opin Biol Ther. (2004) 4:1139–45. doi: 10.1517/14712598.4.7.1139
147. Morein B, Sundquist B, Höglund S, Dalsgaard K, Osterhaus A. Iscom, a novel structure for antigenic presentation of membrane proteins from enveloped viruses. Nature (1984) 308:457–60.
148. Homhuan A, Prakongpan S, Poomvises P, Maas RA, Crommelin DJA, Kersten GFA, et al. Virosome and ISCOM vaccines against Newcastle disease: preparation, characterization and immunogenicity. Eur J Pharm Sci. (2004) 22:459–68. doi: 10.1016/j.ejps.2004.05.005
149. Pedersen JS, Oliveira CLP, Hübschmann HB, Arleth L, Manniche S, Kirkby N, et al. Structure of immune stimulating complex matrices and immune stimulating complexes in suspension determined by small-angle X-ray scattering. Biophys J. (2012) 102:2372–80. doi: 10.1016/j.bpj.2012.03.071
150. Reid G. Soluble proteins incorporate into ISCOMs after covalent attachment of fatty acid. Vaccine (1992) 10:597–602. doi: 10.1016/0264-410X(92)90439-Q
151. Gregory AE, Williamson ED, Prior JL, Butcher WA, Thompson IJ, Shaw AM, et al. Conjugation of Y. pestis F1-antigen to gold nanoparticles improves immunogenicity. Vaccine (2012) 30:6777–82. doi: 10.1016/j.vaccine.2012.09.021
152. Reddy ST, Rehor A, Schmoekel HG, Hubbell JA, Swartz MA. In vivo targeting of dendritic cells in lymph nodes with poly (propylene sulfide) nanoparticles. J Control Release (2006) 112:26–34. doi: 10.1016/j.jconrel.2006.01.006
153. Sunshine JC, Perica K, Schneck JP, Green JJ. Particle shape dependence of CD8+ T cell activation by artificial antigen presenting cells. Biomaterials (2014) 35:269–77. doi: 10.1016/j.biomaterials.2013.09.050
154. Yameen B, Choi W Il Vilos, C, Swami, A, Shi, J, Farokhzad OC. Insight into nanoparticle cellular uptake and intracellular targeting. J Control Release (2014) 190:485–99. doi: 10.1016/j.jconrel.2014.06.038
155. Dobrovolskaia MA, Aggarwal P, Hall JB, McNeil SE. Preclinical studies to understand nanoparticle interaction with the immune system and its potential effects on nanoparticle biodistribution. Mol Pharm. (2008) 5:487–95. doi: 10.1021/mp800032f
156. Zolnik BS, González-Fernández Á, Sadrieh N, Dobrovolskaia MA. Minireview: nanoparticles and the immune system. Endocrinology (2010) 151:458–65. doi: 10.1210/en.2009-1082
157. Joshi VB, Geary SM, Salem AK. Biodegradable particles as vaccine delivery systems: size matters. AAPS J. (2013) 15:85–94. doi: 10.1208/s12248-012-9418-6
158. Manolova V, Flace A, Bauer M, Schwarz K, Saudan P, Bachmann MF. Nanoparticles target distinct dendritic cell populations according to their size. Eur J Immunol. (2008) 38:1404–13. doi: 10.1002/eji.200737984
159. Nishioka Y, Yoshino H. Lymphatic targeting with nanoparticulate system. Adv Drug Deliv Rev. (2001) 47:55–64. doi: 10.1016/S0169-409X(00)00121-6
160. Reddy ST, van der Vlies AJ, Simeoni E, Angeli V, Randolph GJ, O'Neil CP, et al. Exploiting lymphatic transport and complement activation in nanoparticle vaccines. Nat Biotechnol. (2007) 25:1159–64. doi: 10.1038/nbt1332
161. Kostarelos K, Lacerda L, Pastorin G, Wu W, Wieckowski S, Luangsivilay J, et al. Cellular uptake of functionalized carbon nanotubes is independent of functional group and cell type. Nat Nanotechnol. (2007) 2:108–13. doi: 10.1038/nnano.2006.209
162. Foged C, Brodin B, Frokjaer S, Sundblad A. Particle size and surface charge affect particle uptake by human dendritic cells in an in vitro model. Int J Pharm. (2005) 298:315–22. doi: 10.1016/j.ijpharm.2005.03.035
163. Thiele L, Merkle HP, Walter E. Phagocytosis and phagosomal fate of surface-modified microparticles in dendritic cells and macrophages. Pharm Res. (2003) 20:221–8. doi: 10.1023/A:1022271020390
164. Niikura K, Matsunaga T, Suzuki T, Kobayashi S, Yamaguchi H, Orba Y, et al. Gold nanoparticles as a vaccine platform: influence of size and shape on immunological responses in vitro and in vivo. ACS Nano (2013) 7:3926–38. doi: 10.1021/nn3057005
165. Kim ST, Saha K, Kim C, Rotello VM. The role of surface functionality in determining nanoparticle cytotoxicity. Acc Chem Res. (2013) 46:681–91. doi: 10.1021/ar3000647
166. Hillaireau H, Couvreur P. Nanocarriers' entry into the cell: relevance to drug delivery. Cell Mol Life Sci. (2009) 66:2873–96. doi: 10.1007/s00018-009-0053-z
167. Li A, Qin L, Zhu D, Zhu R, Sun J, Wang S. Signalling pathways involved in the activation of dendritic cells by layered double hydroxide nanoparticles. Biomaterials (2010) 31:748–56. doi: 10.1016/j.biomaterials.2009.09.095
168. Zhu R, Zhu Y, Zhang M, Xiao Y, Du X, Liu H, et al. The induction of maturation on dendritic cells by TiO2 and Fe3O4@TiO2 nanoparticles via NF-κB signaling pathway. Mater Sci Eng C (2014) 39:305–14. doi: 10.1016/j.msec.2014.03.005
169. Nawwab AL-Deen FM, Selomulya C, Kong YY, Xiang SD, Ma C, Coppel RL, et al. Design of magnetic polyplexes taken up efficiently by dendritic cell for enhanced DNA vaccine delivery. Gene Ther. (2014) 21:212–8. doi: 10.1038/gt.2013.77
170. Toki S, Omary RA, Wilson K, Gore JC, Peebles RS, Pham W A. comprehensive analysis of transfection-assisted delivery of iron oxide nanoparticles to dendritic cells. Nanomedicine (2013) 9:1235–44. doi: 10.1016/j.nano.2013.05.010
171. Lee YT, Ko EJ, Hwang HS, Lee JS, Kim KH, Kwon YM, et al. Respiratory syncytial virus-like nanoparticle vaccination induces long-term protection without pulmonary disease by modulating cytokines and T-cells partially through alveolar macrophages. Int J Nanomed. (2015) 10:4491–505. doi: 10.2147/IJN.S83493
172. Guo Y, Wang D, Song Q, Wu T, Zhuang X, Bao Y, et al. Erythrocyte membrane-enveloped polymeric nanoparticles as nanovaccine for induction of antitumor immunity against melanoma. ACS Nano (2015) 9:6918–33. doi: 10.1021/acsnano.5b01042
173. Li M, Wang B, Wu Z, Shi X, Zhang J, Han S. Treatment of Dutch rat models of glioma using EphrinA1-PE38/GM-CSF chitosan nanoparticles by in situ activation of dendritic cells. Tumor Biol. (2015) 36:7961–6. doi: 10.1007/s13277-015-3486-z
174. Campbell DF, Saenz R, Bharati IS, Seible D, Zhang L, Esener S, et al. Enhanced anti-tumor immune responses and delay of tumor development in human epidermal growth factor receptor 2 mice immunized with an immunostimulatory peptide in poly(D,L-lactic-co-glycolic) acid nanoparticles. Breast Cancer Res. (2015) 17:48. doi: 10.1186/s13058-015-0552-9
175. Hernández-Gil J, Cobaleda-Siles M, Zabaleta A, Salassa L, Calvo J, Mareque-Rivas JC. An iron oxide nanocarrier loaded with a Pt(IV) prodrug and immunostimulatory dsRNA for combining complementary cancer killing effects. Adv Healthc Mater. (2015) 4:1034–42. doi: 10.1002/adhm.201500080
176. Lu F, Mencia A, Bi L, Taylor A, Yao Y, HogenEsch H. Dendrimer-like alpha-d-glucan nanoparticles activate dendritic cells and are effective vaccine adjuvants. J Control Release (2015) 204:51–9. doi: 10.1016/j.jconrel.2015.03.002
177. Sehgal K, Ragheb R, Fahmy TM, Dhodapkar M V, Dhodapkar KM. Nanoparticle-mediated combinatorial targeting of multiple human Dendritic Cell (DC) subsets leads to enhanced T cell activation via IL-15–dependent DC crosstalk. J Immunol. (2014) 193:2297–305. doi: 10.4049/jimmunol.1400489
178. Hoang MD, Lee HJ, Lee HJ, Jung SH, Choi NR, Vo MC, et al. Branched Polyethylenimine-Superparamagnetic Iron Oxide Nanoparticles (bPEI-SPIONs) improve the immunogenicity of tumor antigens and enhance Th1 polarization of dendritic cells. J Immunol Res. (2015) 2015:706379. doi: 10.1155/2015/706379
179. Mundayoor S, Kumar RA, Dhanasooraj S. Vaccine delivery system for tuberculosis based on nano-sized hepatitis B virus core protein particles. Int J Nanomedicine (2013) 8:835. doi: 10.2147/IJN.S40238
180. Amini Y, Moradi B, Tafaghodi M, Meshkat Z, Ghazvini K, Fasihi-Ramandi M. TB trifusion antigen adsorbed on calcium phosphate nanoparticles stimulates strong cellular immunity in mice. Biotechnol Bioprocess Eng. (2016) 21:653–8. doi: 10.1007/s12257-016-0326-y
181. Speth MT, Repnik U, Müller E, Spanier J, Kalinke U, Corthay A, et al. Poly(I:C)-encapsulating nanoparticles enhance innate immune responses to the tuberculosis vaccine Bacille Calmette–Guérin (BCG) via synergistic activation of innate immune receptors. Mol Pharm. (2017) 14:4098–112. doi: 10.1021/acs.molpharmaceut.7b00795
182. Poecheim J, Barnier-Quer C, Collin N, Borchard G. Ag85A DNA vaccine delivery by nanoparticles: influence of the formulation characteristics on immune responses. Vaccines (2016) 4:32. doi: 10.3390/vaccines4030032
183. Ballester M, Nembrini C, Dhar N, de Titta A, de Piano C, et al. Nanoparticle conjugation and pulmonary delivery enhance the protective efficacy of Ag85B and CpG against tuberculosis. Vaccine (2011) 29:6959–66. doi: 10.1016/j.vaccine.2011.07.039
184. Nel AE, Mädler L, Velegol D, Xia T, Hoek EM V, Somasundaran P, et al. Understanding biophysicochemical interactions at the nano-bio interface. Nat Mater. (2009) 8:543–57. doi: 10.1038/nmat2442
185. Lynch I, Dawson KA. Protein-nanoparticle interactions. (2008) 3:40–7. doi: 10.1016/S1748-0132(08)70014-8
186. Vertegel AA, Siegel RW, Dordick JS. Silica nanoparticle size influences the structure and enzymatic activity of adsorbed lysozyme. Langmuir (2004) 20:6800–7. doi: 10.1021/la0497200
187. Taylor PR, Martinez-Pomares L, Stacey M, Lin HH, Brown GD, Gordon S. Macrophage receptors and immune recognition. Annu Rev Immunol. (2005) 23:901–44. doi: 10.1146/annurev.immunol.23.021704.115816
188. Beduneau A, Ma Z, Grotepas CB, Kabanov A, Rabinow BE, Gong N, et al. Facilitated monocyte-macrophage uptake and tissue distribution of superparmagnetic iron-oxide nanoparticles. PLoS ONE (2009) 4:e4343. doi: 10.1371/journal.pone.0004343
189. Laroui H, Viennois E, Xiao B, Canup BSB, Geem D, Denning TL, et al. Fab'-bearing siRNA TNFα-loaded nanoparticles targeted to colonic macrophages offer an effective therapy for experimental colitis. J Control Release (2014) 186:41–53. doi: 10.1016/j.jconrel.2014.04.046
190. Verma A, Stellacci F. Effect of surface properties on nanoparticle-cell interactions. Small (2010) 6:12–21. doi: 10.1002/smll.200901158
191. Kolaczkowska E, Kubes P. Neutrophil recruitment and function in health and inflammation. Nat Rev Immunol. (2013) 13:159–75. doi: 10.1038/nri3399
192. Kamaly N, Fredman G, Subramanian M, Gadde S, Pesic A, Cheung L, et al. Development and in vivo efficacy of targeted polymeric inflammation-resolving nanoparticles. Proc Natl Acad Sci USA., (2013) 110:6506–11. doi: 10.1073/pnas.1303377110
193. Chu D, Gao J, Wang Z. Neutrophil-mediated delivery of therapeutic nanoparticles across blood vessel barrier for treatment of inflammation and infection. ACS Nano (2015) 9:11800–11. doi: 10.1021/acsnano.5b05583
194. Endsley AN, Ho RJY. Enhanced anti-HIV efficacy of indinavir after inclusion in CD4-targeted lipid nanoparticles. JAIDS J Acquir Immune Defic Syndr. (2012) 61:417–24. doi: 10.1097/QAI.0b013e3182653c1f
195. Perisé-Barrios AJ, Jiménez JL, Domínguez-Soto A, de la Mata FJ, Corbí AL, Gomez R, et al. Carbosilane dendrimers as gene delivery agents for the treatment of HIV infection. J Control Release (2014) 184:51–7. doi: 10.1016/j.jconrel.2014.03.048
196. Harding C V, Collins DS, Slot JW, Geuze HJ, Unanue ER. Liposome-encapsulated antigens are processed in lysosomes, recycled, and presented to T cells. Cell (1991) 64:393–401. doi: 10.1016/0092-8674(91)90647-H
197. Stano A, Nembrini C, Swartz MA, Hubbell JA, Simeoni E. Nanoparticle size influences the magnitude and quality of mucosal immune responses after intranasal immunization. Vaccine (2012) 30:7541–6. doi: 10.1016/j.vaccine.2012.10.050
198. Kim YM, Pan JYJ, Korbel GA, Peperzak V, Boes M, Ploegh HL. Monovalent ligation of the B cell receptor induces receptor activation but fails to promote antigen presentation. Proc Natl Acad Sci USA. (2006) 103:3327–32. doi: 10.1073/pnas.0511315103
199. Chackerian B, Lowy DR, Schiller JT. Conjugation of a self-antigen to papillomavirus-like particles allows for efficient induction of protective autoantibodies. J Clin Invest. (2001) 108:415–23. doi: 10.1172/JCI11849
200. Friede M, Muller S, Briand JP, Van Regenmortel MH, Schuber F. Induction of immune response against a short synthetic peptide antigen coupled to small neutral liposomes containing monophosphoryl lipid A. Mol Immunol. (1993) 30:539–47. doi: 10.1016/0161-5890(93)90028-A
201. Bershteyn A, Hanson MC, Crespo MP, Moon JJ, Li A V Suh, H, et al. Robust IgG responses to nanograms of antigen using a biomimetic lipid-coated particle vaccine. J Control Release (2012) 157:354–65. doi: 10.1016/j.jconrel.2011.07.029
202. Temchura VV, Kozlova D, Sokolova V, Uberla K, Epple M. Targeting and activation of antigen-specific B-cells by calcium phosphate nanoparticles loaded with protein antigen. Biomaterials (2014) 35:6098–105. doi: 10.1016/j.biomaterials.2014.04.010
203. Joffre OP, Segura E, Savina A, Amigorena S. Cross-presentation by dendritic cells. Nat Rev Immunol. (2012) 12:557–69. doi: 10.1038/nri3254
204. Brode S, Macary PA. Cross-presentation: dendritic cells and macrophages bite off more than they can chew! Immunology (2004) 112:345–51. doi: 10.1111/j.1365-2567.2004.01920.x
205. Amigorena S, Savina A. Intracellular mechanisms of antigen cross presentation in dendritic cells. Curr Opin Immunol. (2010) 22:109–17. doi: 10.1016/j.coi.2010.01.022
206. Kovacsovics-Bankowski M, Clark K, Benacerraf B, Rock KL. Efficient major histocompatibility complex class I presentation of exogenous antigen upon phagocytosis by macrophages. Proc Natl Acad Sci USA. (1993) 90:4942–6. doi: 10.1073/pnas.90.11.4942
207. Kovacsovics-Bankowski M, Rock KL. A phagosome-to-cytosol pathway for exogenous antigens presented on MHC class I molecules. Science (1995) 267:243–6. doi: 10.1126/science.7809629
208. Reis e Sousa C, Germain RN. Major histocompatibility complex class I presentation of peptides derived from soluble exogenous antigen by a subset of cells engaged in phagocytosis. J Exp Med. (1995) 182:841–51.
209. Harding C V, Song R. Phagocytic processing of exogenous particulate antigens by macrophages for presentation by class I MHC molecules. J Immunol. (1994) 153:4925–33.
210. Jain S, Yap WT, Irvine DJ. Synthesis of protein-loaded hydrogel particles in an aqueous two-phase system for coincident antigen and CpG oligonucleotide delivery to antigen-presenting cells. Biomacromolecules (2005) 6:2590–600. doi: 10.1021/bm0503221
211. Hamdy S, Elamanchili P, Alshamsan A, Molavi O, Satou T, Samuel J. Enhanced antigen-specific primary CD4+ and CD8+ responses by codelivery of ovalbumin and toll-like receptor ligand monophosphoryl lipid A in poly(D,L-lactic-co-glycolic acid) nanoparticles. J Biomed Mater Res A (2007) 81:652–62. doi: 10.1002/jbm.a.31019
212. Tanaka Y, Taneichi M, Kasai M, Kakiuchi T, Uchida T. Liposome-coupled antigens are internalized by antigen-presenting cells via pinocytosis and cross-presented to CD8 T cells. PLoS ONE (2010) 5:e15225. doi: 10.1371/journal.pone.0015225
213. Brandtzaeg P. Induction of secretory immunity and memory at mucosal surfaces. Vaccine (2007) 25:5467–84. doi: 10.1016/j.vaccine.2006.12.001
214. Macho Fernandez E, Chang J, Fontaine J, Bialecki E, Rodriguez F, Werkmeister E, et al. Activation of invariant Natural Killer T lymphocytes in response to the α-galactosylceramide analogue KRN7000 encapsulated in PLGA-based nanoparticles and microparticles. Int J Pharm. (2012) 423:45–54. doi: 10.1016/j.ijpharm.2011.04.068
215. van der Vlies AJ, O'Neil CP, Hasegawa U, Hammond N, Hubbell JA. Synthesis of pyridyl disulfide-functionalized nanoparticles for conjugating thiol-containing small molecules, peptides, and proteins. Bioconjug Chem. (2010) 21:653–62. doi: 10.1021/bc9004443
216. Hirosue S, Kourtis IC, van der Vlies AJ, Hubbell JA, Swartz MA. Antigen delivery to dendritic cells by poly(propylene sulfide) nanoparticles with disulfide conjugated peptides: cross-presentation and T cell activation. Vaccine (2010) 28:7897–906. doi: 10.1016/j.vaccine.2010.09.077
217. Nembrini C, Stano A, Dane KY, Ballester M, van der Vlies AJ, Marsland BJ, et al. Nanoparticle conjugation of antigen enhances cytotoxic T-cell responses in pulmonary vaccination. Proc Natl Acad Sci USA. (2011) 108:E989–97. doi: 10.1073/pnas.1104264108
218. Swartz MA. The physiology of the lymphatic system. Adv Drug Deliv Rev. (2001) 50:3–20. doi: 10.1016/S0169-409X(01)00150-8
219. Cubas R, Zhang S, Kwon S, Sevick-Muraca EM, Li M, Chen C, et al. Virus-like particle (VLP) lymphatic trafficking and immune response generation after immunization by different routes. J Immunother. (2009) 32:118–28. doi: 10.1097/CJI.0b013e31818f13c4
220. Dane KY, Nembrini C, Tomei AA, Eby JK, O'Neil CP, Velluto D, et al. Nano-sized drug-loaded micelles deliver payload to lymph node immune cells and prolong allograft survival. J Control Release (2011) 156:154–60. doi: 10.1016/j.jconrel.2011.08.009
221. Allen TM, Hansen CB, Guo LS. Subcutaneous administration of liposomes: a comparison with the intravenous and intraperitoneal routes of injection. Biochim Biophys Acta (1993) 1150:9–16. doi: 10.1016/0005-2736(93)90115-G
222. Vetro M, Safari D, Fallarini S, Salsabila K, Lahmann M, Penadés S, et al. Preparation and immunogenicity of gold glyco-nanoparticles as antipneumococcal vaccine model. Nanomedicine (Lond) (2017) 12:13–23. doi: 10.2217/nnm-2016-0306
223. Margaroni M, Agallou M, Athanasiou E, Kammona O, Kiparissides C, Gaitanaki C, et al. Vaccination with poly(D,L-lactide-co-glycolide) nanoparticles loaded with soluble Leishmania antigens and modified with a TNFα-mimicking peptide or monophosphoryl lipid A confers protection against experimental visceral leishmaniasis. Int J Nanomedicine (2017) 12:6169–84. doi: 10.2147/IJN.S141069
224. Qi M, Zhang XE, Sun X, Zhang X, Yao Y, Liu S, et al. Intranasal nanovaccine confers homo- and hetero-subtypic influenza protection. Small (2018) 14:e1703207. doi: 10.1002/smll.201703207
225. Sawaengsak C, Mori Y, Yamanishi K, Mitrevej A, Sinchaipanid N. Chitosan nanoparticle encapsulated hemagglutinin-split influenza virus mucosal vaccine. AAPS PharmSciTech (2014) 15:317–25. doi: 10.1208/s12249-013-0058-7
226. Figueiredo L, Cadete A, Gonçalves L, Corvo M, Almeida AJ. Intranasal immunisation of mice against Streptococcus equi using positively charged nanoparticulate carrier systems. Vaccine (2012) 30:6551–8. doi: 10.1016/j.vaccine.2012.08.050
227. Khatri K, Goyal AK, Gupta PN, Mishra N, Vyas SP. Plasmid DNA loaded chitosan nanoparticles for nasal mucosal immunization against hepatitis B. Int J Pharm (2008) 354:235–41. doi: 10.1016/j.ijpharm.2007.11.027
228. Ai W, Yue Y, Xiong S, Xu W. Enhanced protection against pulmonary mycobacterial challenge by chitosan-formulated polyepitope gene vaccine is associated with increased pulmonary secretory IgA and gamma-interferon(+) T cell responses. Microbiol Immunol (2013) 57:224–35. doi: 10.1111/1348-0421.12027
Keywords: nanoparticles, vaccine development, human diseases, targeted vaccine delivery, antigens
Citation: Pati R, Shevtsov M and Sonawane A (2018) Nanoparticle Vaccines Against Infectious Diseases. Front. Immunol. 9:2224. doi: 10.3389/fimmu.2018.02224
Received: 13 April 2018; Accepted: 07 September 2018;
Published: 04 October 2018.
Edited by:
Nahid Ali, Indian Institute of Chemical Biology, IndiaReviewed by:
Randy A. Albrecht, Icahn School of Medicine at Mount Sinai, United StatesMichael Schotsaert, Icahn School of Medicine at Mount Sinai, United States
Katie Louise Flanagan, RMIT University, Australia
Copyright © 2018 Pati, Shevtsov and Sonawane. This is an open-access article distributed under the terms of the Creative Commons Attribution License (CC BY). The use, distribution or reproduction in other forums is permitted, provided the original author(s) and the copyright owner(s) are credited and that the original publication in this journal is cited, in accordance with accepted academic practice. No use, distribution or reproduction is permitted which does not comply with these terms.
*Correspondence: Avinash Sonawane, YXNvbmF3YW5lQGlpdGkuYWMuaW4=