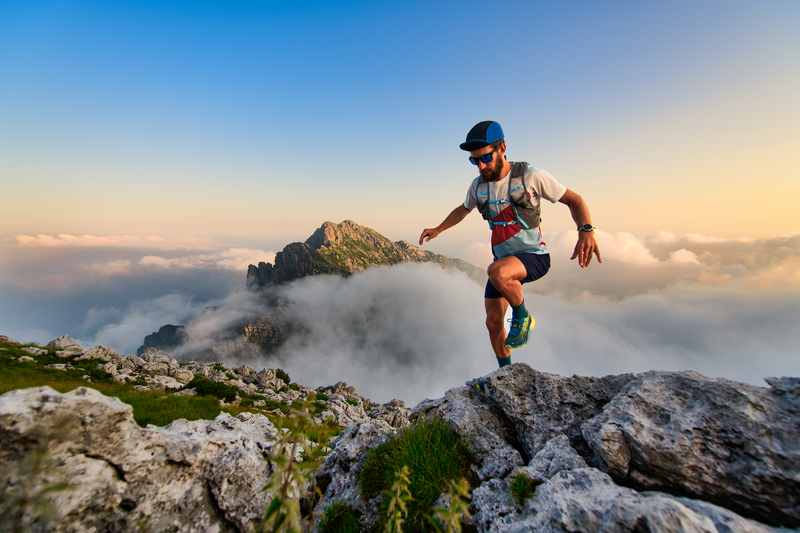
94% of researchers rate our articles as excellent or good
Learn more about the work of our research integrity team to safeguard the quality of each article we publish.
Find out more
MINI REVIEW article
Front. Immunol. , 10 August 2018
Sec. Immunological Memory
Volume 9 - 2018 | https://doi.org/10.3389/fimmu.2018.01860
This article is part of the Research Topic Tissue Resident Memory T Cells View all 16 articles
The induction of immunological memory, which is mediated by memory T and B cells, is central to adaptive protective immunity to pathogens induced by previous infection and is the cornerstone of effective vaccine design. Recent studies in mice have suggested that memory T cells that accumulate in tissues, termed tissue-resident memory T (TRM) cells, play a crucial role in maintaining long-term protective immunity to mucosal pathogens. CD4 and CD8 TRM cells can be induced following infection at mucosal sites or the skin, where they are maintained and poised to respond rapidly to reinfection with the same pathogen. TRM cells can also be generated by vaccination, but their induction is influenced by a number of factors, including the type of vaccine, the adjuvant, and the route of immunization. Live attenuated vaccines appear to be more effective than killed or subunit vaccines at inducing TRM cells and mucosal immunization, especially by intranasal route, is more effective than parenteral delivery. However, evidence is emerging that formulation of killed or subunit vaccines with novel adjuvants, especially those that generate Th1 and Th17 responses, can promote the induction of TRM cells. While TRM cells are also present at high number in mucosal tissues in humans, one of the challenge will be to develop methodologies for routine quantification of these cells in humans. Nevertheless, the identification of approaches for optimum induction of TRM cells in mice should assist in the design of more effective vaccines that sustain protective immunity against a range of human pathogens.
The induction of immunological memory is central to antipathogen adaptive immunity induced by previous infection or vaccination. While circulating antibodies can confer protection against infection with certain pathogens, antibodies in the circulation and at mucosal sites usually wane over time and long-term protection is dependent on the induction of memory T and B cells. There is growing recognition that memory T cells that reside in tissues, called tissue-resident memory T (TRM) cells, play a crucial role in maintain long-term immunity, especially against pathogens that infect mucosal surfaces (1). TRM cells were identified as cells that retained in the non-lymphoid organs with limited ability to recirculate. Tissue-resident lymphocytes constitutively express adhesion molecules and integrins that help them to remain in the tissue. These include CD44, a receptor for hyaluronic acid that can also bind to collagens or matrix metalloproteinases, and CD69, a transmembrane C-type lectin that is critical for regulating the T cell egress from lymphoid organs and retention in peripheral tissues (2, 3). CD103, αE integrin, is often expressed on intraepithelial and airway CD8 TRM cells. As a receptor for E-cadherin, CD103 helps TRM cells to adhere to the epithelium and be positioned on the first line of defense (4). However, the expression of CD103 by CD4 TRM cells is more controversial. A study by Collins et al. demonstrated that CD103 can be expressed on CD4 memory T cells egressing from the skin and suggested that this marker may be modulated as CD4 T cells enter and leave the skin (5). In contrast, CD4 effector T cells that infiltrated and resided in the skin after primary infection with Candida albicans acquired expression of CD69 and CD103 (6). We have recently reported that infection with Bordetella pertussis induces CD69+ CD4 TRM cells and a significant proportion of these cells stably express CD103 through the course of infection and after clearance of the bacteria (7). Following reinfection with B. pertussis, CD103 was rapidly upregulated on these cells, and this was not affected by treatment with FTY720, which inhibits lymphocyte egress from the draining lymph node and tissues (7). Retention of TRM cells in tissues is facilitated by downregulation of CD62L and CCR7, “homing receptors” that allow T cells to enter secondary lymphoid organs, and sphingosine-1-phosphate receptor 1, which enables cells to egress from lymphoid tissues (8–10). The expression of other molecules like chemokine receptors on TRM cells are often shaped by the specific tissue environmental cues.
Newborns and infants are in a greater risk from infections than adult humans. For example, high levels of morbidity and mortality have been reported in infants following respiratory infections with pathogens like influenza virus or B. pertussis, suggesting impaired protective immunity in infants compared with adults (11, 12). A possible explanation is that in pediatric tissues, the dominant population of T cells are naïve T cell emigrants from the thymus, whereas adult tissues contain predominantly memory T cells (13). Moreover, results from a mouse model of influenza infection have indicated that impaired protective immunity induced by previous infection or vaccination during infancy may reflect reduced generation of TRM cells (14). Collectively, the emerging data on TRM cells suggest that they play a critical role in long-term protective immunity induced by previous infection or vaccination.
The key function of TRM cells is to rapidly respond to infection or reinfection with a pathogen and to orchestrate local immune responses in the tissue that mediate clearance of the pathogen. TRM cells that are generated by infection are sustained in the local tissue after clearance of the pathogen (15). During a life time, TRM cells accumulate in many tissues and provide long-term local protection against subsequent infection by reactivation with specific antigen (15, 16). The persistence of TRM cells in tissue after pathogen clearance and the mechanism of maintenance in the tissues is unclear. Although memory T cells classically require antigen-specific stimulation through the T cell receptor (TCR) and costimulation for proliferation, there is evidence that TRM cells may respond in an innate-like manner to cytokines, including IL-18, IL-12, IL-15, and tumor necrosis factor (TNF)-like cytokine 1A (TL1a), without TCR activation (17). Signaling from IL-15 and TGF-β has been shown to be critical for persistence of mature CD8 TRM cells (Figure 1) (18). Furthermore, while much of the focus has been on pathogen-induced TRM cells, these cells can also be generated by non-infectious agents, including allergens or autoantigens, and can mediate pathology in asthma or autoimmune disorders (19, 20). Nonetheless, there is growing evidence from mouse studies of a beneficial role for CD4 and CD8 TRM cells in protection against a variety of infectious pathogens.
Figure 1. Induction, expansion and function of CD4 TRM cells. (A) Following infection or immunization effector and memory T cell responses are induced in lymph nodes. Effector-memory T cells can migrate to the tissue where they are retained as CD4 TRM cells through expression of integrins and adhesion molecules, such as CD44, CD69 and/or CD103. Their induction is influenced by IL-2, IL-15 and TGF-β. (B) CD4 TRM cells are retained in the tissues and can be reactivated locally in MLCs during secondary responses following reinfection or after infection in an immunized host. CD4 TRM cells are expanded and can become effector Th1, Th17 or Th2-type cells that mediate rapid clearance of the infection. (C) There is emerging evidence that CD4 TRM cells can be non-specifically activated in the tissues by cytokines, such as IL-18, IL-12, IL-15 and TL1a, and develop into polyfunctional T cells that have potent anti-pathogen activity. (D) It has also been suggested that IL-7 and IL-33 may non-specifically activate CD4 TRM cells to become effector Th2 cells. DR3: death receptor 3; TL1a: tumor necrosis factor (TNF)-like cytokine 1a; MLCs: memory lymphocyte clusters; APC: antigen presenting cell; Mϕ: macrophage; NK: natural killer cell; Bc: B cells; Th: T helper (cell); Tn: naïve T cell; TEM: effector memory T cells; TRM: tissue-resident memory T cells.
CD8 cytotoxic T lymphocytes play a critical role in the control of viral infections by killing virally infected cells. However, CD4 T cells also play a vital role in protective immunity to viruses by helping antibody production and facilitate the induction and expansion of virus-specific memory CD8 T cells, including CD8 TRM cells (21–23). Recent studies demonstrated that respiratory infection with a number of different viruses can also induce CD4 TRM cells. It has been reported that influenza virus infection induces polyclonal, virus-specific memory CD4 T cells in the lungs and the spleens of infected mice (24). Adoptive transfer experiments with CD4 T cells specific for influenza hemagglutinin (HA) showed that lung-derived memory CD4 T cells were almost exclusively found in the lungs 7 days after transfer to naïve mice. In contrast, transferred splenic memory CD4 T cells were distributed in multiple tissues and were not retained in these tissues. Furthermore, only HA-specific CD4 TRM cells from the lungs conferred protection against lethal influenza infection (24). These findings suggested that lung-resident CD4 TRM cells, but not spleen memory CD4 T cells, play a crucial role in local protective immunity against influenza virus infection in the lungs.
Antigen-specific TRM cells induced during influenza virus infection are localized near the airways and bronchovascular bundles and are maintained long after viral clearance independently of replenishment from lymphoid stores (25). IL-2-dependent and -independent mechanisms have been described for generation of influenza-specific CD4 TRM cells, contributing to heterogeneity of protective TRM cells. The formation of IL-2-independent subset of TRM cells required a direct IL-15 signal to CD4 T-cell effectors (26, 27). Similarly, intranasal infection of mice with lymphocytic choriomeningitis virus (LCMV) required IL-2 signaling for the generation of virus-specific CD4 TRM cells. CD4 T cells that lacked CD25, the IL-2 receptor α chain, failed to develop into lung TRM cells in LCMV-infected mice (28). These studies suggest a broad mechanism involving IL-2 signaling pathway for the formation of CD4 TRM cells.
Similar to respiratory tissue, the female reproductive track is vulnerable for repeated infections. In a model of herpes simplex virus-2 (HSV-2) infection, where thymidine-kinase defective (TK−) HSV-2 was used to avoid neurovirulence (29), CD4 T cells infiltrated the female genital mucosa during infection and provided help for mobilizing cytotoxic effector CD8 T cells that cleared the infection (30). In addition, infection with TK− HSV-2 provided local, long-term protection against a secondary infection with wild-type HSV-2 based on the formation of CD4 TRM cells, which were retained mainly among memory lymphocyte clusters (MLCs) (31). Therefore, an effective vaccine against HSV-2 infection may be possible by targeting the induction of TRM cells.
A number of recent studies have indicated that CD4 TRM cells established in non-lymphoid tissues after primary infection provide protection against reinfection with the same pathogen. In a mouse model of B. pertussis infection, it was demonstrated that transfer of Th1-like cells resulted in pathogen clearance in the absence of specific antibodies (32). We have recently reported that infection of mice with B. pertussis induce the development of CD69+CD103+/− CD4 TRM cells in the lungs (7). Treatment of convalescent mice with FTY720 did not affect clearance of a secondary infection with B. pertussis, suggesting that an established population of TRM cells mediates local protective immunity against reinfection. Moreover, adoptive transfer of CD4 TRM cells from the lungs of convalescent mice conferred protection against B. pertussis infection in naïve mice (7). It has also been demonstrated that pulmonary infection with Mycobacterium tuberculosis is controlled by a subset of lung parenchymal-homing CD4 T cells. Adoptive transfer of parenchymal TRM cells into susceptible T cell-deficient hosts showed preferential migration back to the lung and superior control of infection compared with the intravascular CD4 T cells (33).
In a mouse model of pneumonia, repeated respiratory infections with Streptococcus pneumoniae (pneumococcus) seeded the lungs with antibacterial CD4 TRM cells that mediated heterotypic protection (34). Furthermore, oral infection of mice with Listeria monocytogenes induced robust pathogen-specific CD4 T cell response, the majority of which migrated to the intestine and were transitioned to long-lived TRM cells with a polyfunctional Th1 profile, secreting predominantly IFN-γ, TNF, and IL-2, and detectable level of IL-17 (35).
There is also emerging data to suggest that CD4 TRM cells play a central role in protection against Chlamydia trachomatis infection (36). It has been shown that lymphoid aggregates, which contained CD4 T cells, are formed in the genital tract of mice during infection with C. trachomatis. These aggregates, which resembled MLCs described by Iijima and Iwasaki (31), persisted long after the infection had resolved (37). The formation of lymphoid aggregates with TRM cells during primary infection provided a robust response to secondary infectious challenge and was dependent on B cell antigen presentation in established MLCs (38). These findings demonstrate that bacterial infection at various mucosal site (lungs, gut, and gential tract) induce CD4 TRM cells that mediate protective immunity against reinfection of the mucosa with the relevant pathogen.
The development of Th2-type immune responses are required for protective immunity against infection with helminths, such as Nippostrongylus brasiliensis (39). Recent studies on lung infection with N. brasiliensis revealed that a Th2-type polarized pulmonary CD4 T cell population established during infection and can drive effective local adaptive immunity to reinfection with the same parasite (40). In a mouse model of intestinal infection with Heligmosomoides polygyrus, functional memory Th2 cells persisted in the lamina propria and the peritoneal cavity after resolution of infection. Interestingly, cells at both locations produced Th2 cytokines after restimulation; however, only peritoneal CD4 TRM cells mediated protective immunity against the helminth infection. The Th2-type CD4 TRM cells expressed high levels of the IL-33 receptor and produced effector cytokines in response to IL-33 and IL-7 independently to TCR activation (41). CD4 TRM cells have also been identified in the skin after infection with Leishmania major where they persisted long after the pathogen was cleared (42, 43). Interestingly, CD4 TRM cells were also found in the flank skin far from the primary infection site in the ear. Pathogen-specific CD4 TRM cells produced IFN-γ in response to secondary infection and rapidly recruited other memory cells from the circulation; however, recruitment and activation of inflammatory monocytes was required for optimal protection (42, 43). These findings suggest that Th1- and Th2-type TRM cells are induced by infection with different parasites and these cells mediate host protective immunity against the relevant parasite.
A key research question that is beginning to be addressed is whether there are distinct Th1, Th2, and Th17 subtypes of TRM cells and whether effector Th1, Th2, and Th17 arise from TRM cells in the tissues after reinfection with a pathogen. It has been reported that skin infection with C. albicans in humans or mice leads to formation of IL-17-producing CD4 TRM cells that reside in papillary dermis and rapidly clear the infection after re-exposure to the pathogen (6). It was also shown that protection against oropharyngeal candidiasis is mediated by oral-resident natural Th17 cells (44). Th1 cells have an established protective role in immunity to viruses and intracelluar bacteria and evidence is emerging that IFN-γ-seceting TRM cells are critical for long-term protection against these pathogens. The findings from the parasite field also suggest that Th2 or Th1-type TRM cells may play key roles in protective immunity against extracellular and intracellular parasites, respectively. However, the factors that control the development or specific activation of effector Th1, Th2, and Th17 from TRM cells in the tissues after reinfection with a pathogen are still unclear (Figure 1).
While most successful vaccines in use today mediate protective immunity through the induction of antibodies, optimum protection against many pathogens requires the generation of appropriate cellular immune responses, including CD4 T cells. Indeed, there are increasing number of studies showing that the formation of CD4 TRM cells after natural infection mediates protective immunity against secondary exposure to the same pathogen. Although there is less evidence of a role for CD4 TRM cells in protective immunity generated with vaccines in use today, the recent studies in mice have suggested that the induction of CD4 TRM cells may be central to persistent vaccine-induced protection against a range of mucosal pathogens. Immunization approaches that induce systemic and tissue-retained memory CD4 T cells may be critical to persistent protection, because they are long-lived in the tissues and are more polyclonal than CD8 T cells (45, 46). It has also been suggested that CD4 T cell are less prone than CD8 T cell to immune escape from antigenic variation in T cell epitopes (47). Therefore, the induction of CD4 TRM cells may be a promising approach for the design of new or improved vaccines. In the light of recent findings on the development of TRM cells in different mucosal tissues, several important factors have to be considered in the development optimal immunization approaches for the induction of these cells.
The efficacy of certain vaccines is influenced by the route of immunization. A comparison of two different licensed influenza vaccines given by intranasal or parenteral routes demonstrated that the route of administration, as well the type of vaccine (live versus killed), influenced the induction of CD4 TRM cells. Intranasal administration of attenuated influenza virus vaccine (FluMist) generated CD4 TRM cells in the lungs, which mediated long-term protection against non-vaccine strains of influenza virus. In contrast, an inactivated influenza virus vaccine (Fluzone) induced strain-specific neutralizing antibodies, but failed to induce TRM cells, even when delivered intranasally (48). Studies with coronaviruses (CoVs), which cause a severe respiratory disease in humans, showed that intranasal, but not subcutaneous, immunization with SARS-CoV nucleocapsid (N) protein induced airway and lung-parenchymal antigen-specific memory CD4 TRM cells (49). However, protection was lost following depletion of airway, but not parenchymal, memory TRM cells. These results provide evidence of compartmentalization of the immune response induced by vaccination and suggest that TRM cells may preferentially populate the site of induction/immunization.
Vaccine-induced TRM cells can be localized not only near the site of immunization but can be spread to other parts of the same tissue. Mucosal vaccination can induce broad mucosal-tropic memory lymphocytes. Intranasal immunization with attenuated TK− HSV-2 resulted in long-lasting protection mediated by HSV-2-specific CD4 TRM in distant tissues, the vaginal mucosa (50, 51). Similarly, transmucosal protection against Chlamydia muridarum infection was established after oral vaccination. Colonization of the gastrointestinal tract with non-pathogenic bacterium induced protective immunity in the genital tract (52). Furthermore, it was shown that intranasal, but not subcutaneous, vaccination with ultraviolet light (UV)-inactivated C. trachomatis complexed with charge-switching synthetic adjuvant particles induced protective CD4 T cells that rapidly populated uterine mucosa with TRM cells (53).
Conserved vaccine antigens have the potential to induce broadly cross-protective immunity against many strains of the same pathogen. This is particularly important for pathogens like influenza virus, where the HA molecule, the target antigen for neutralizing antibodies, undergoes significant antigen variation allowing escape from protective immunity against seasonal strains of influenza virus. It was reported that intranasal immunization with influenza virus matrix protein ectodomain (M2e) adjuvanted with CTA1-DD generated highly protective M2e-specific lung-resident Th17 TRM cells (54). Moreover, immunized mice were protected against a potentially lethal challenge with H3N2 or H1N1 influenza virus strains, demonstrating effective cross-protection. These results demonstrate that induction of TRM cells and their ability to protect against mucosal infections is influenced by the route of immunization. Therefore, the design of more effective vaccines against mucosal pathogens needs to move beyond the common approach of using injectable vaccines and should utilize appropriate routes of mucosal immunization to promote protective TRM cells at the sites of infection.
The choice of adjuvant can influence the induction of cellular immune response and formation of TRM cells following vaccination. Stary et al. showed that genital infection with C. trachomatis induced protective immunity in the uterus, whereas immunization with UV-inactivated C. trachomatis, which favored generation of regulatory T cells, exacerbated subsequent infection (53). However, an experimental vaccine comprising UV-inactivated C. trachomatis complexed with charge-switching synthetic adjuvant particles was effective at inducing antigen-specific CD4 TRM cells and long-term protection (53). It has been reported that IL-1β may act as an adjuvant for the induction of TRM that mediate protective immune responses against influenza virus infection. Intranasal administration of a novel vaccine, based on recombinant adenoviral vectors (rAd) encoding influenza HA and nucleoprotein in combination with rAd-IL-1β promoted the generation of CD103+CD69+ TRM cells that mediated protection against infection with homologous and heterologous influenza virus strains (55).
Current vaccines against whooping cough (pertussis) are administered parenterally, usually by intramuscular route; however, immunity is relatively short lived, especially after immunization with acellular pertussis (aP) vaccines, which is administered with alum as the adjuvant (56). Studies in a baboon model have shown that the current aP vaccine fails to prevent nasal colonization and transmission of B. pertussis (57). In contrast, immunization with an attenuated B. pertussis vaccine, BPZE1, protected baboons against nasopharyngeal colonization and disease induced by a highly virulent strain of B. pertussis (58). Since BPZE1 is replicating bacterium delivered by the intranasal route, it is likely to induce respiratory TRM cells. Current parenterally delivered aP vaccines preferentially induce strong antibody and Th2-type responses, whereas experimental aP vaccines formulated with more potent adjuvants, such as TLR agonists, induce potent Th1 and Th17 responses in mice (59, 60). Therefore, it should also be possible to develop an intranasally delivered aP vaccine with an appropriate adjuvant that induces IL-17 and IFN-γ-secreting TRM cells in the lungs and nasal tissue. It has been reported that the formation of CD8 TRM cells in the nasal epithelium after immunization are key for limiting influenza viral spread to the lower respiratory track (61). Therefore, induction of respiratory TRM cells by intranasal immunization appears to be an ideal approach for inducing long-term protection in the upper and lower respiratory tract.
In the vaccine field, the big questions include (1) whether CD4 TRM cells are really important for long-term protective immunity in humans, (2) how antigen-specific TRM cells can be optimally induced by vaccination, and (3) how antigen-specific TRM cells can be detected and quantified after infection or vaccination in humans. Most of the vaccines in use today protect by induction of antibody responses that either neutralize viruses or bacterial toxins or opsonize bacteria for killing by phagocytic cells. However, there are other infectious diseases, such as HIV, malaria, and tuberculosis where we do not have an effective vaccine, and where T-cell responses may be more important in preventing or clearing the infection. Furthermore, there is a move away from killed and live attenuated vaccine to subunit vaccines, which are usually delivered by injectable routes. However, the first choice adjuvant alum, while capable of promoting the induction of antibody and Th2 responses is not very effective at inducing Th1 responses. In addition, injected alum-adjuvant vaccines do not appear to be capable of inducing TRM cells. The current pertussis aP vaccine is a good example; it fails to induce Th1 cells (59) and protective immunity wanes rapidly after immunization in children (62). This is likely to reflect a failure to induce CD4 TRM cells. Effort to develop more effective aP and other subunit vaccines need to focus on mucosal routes of immunization and adjuvants that induce TRM cells, as well Th1 and Th17 cells that can be detected in the periphery. It was reported that subcutaneous priming followed by intranasal boosting with group A streptococcal C5a peptidase formulated with a cationic adjuvant induced persistent local immune response including IgA, Th17 cells, and TRM cells (63). The “Prime and Pull” strategy may be a useful approach for eliciting both systemic and local immunity and immunological memory with subunit vaccines (64).
The vast majority of the published work on TRM cells have been based on studies in mouse models. CD69+ TRM cells have also been identified in human tissues (65). However, since TRM cells are in the tissue rather than the blood, one of the challenges in translating the mouse studies to humans is the difficulty in getting routine access to human mucosal tissue samples to study and quantify the induction of TRM cells following infection or vaccination. This could be overcome by the identification of precursors of TRM in the circulation as they migrate from lymph nodes to tissues. Peripheral memory CD8 T cells that express CX3CR1 have been identified in mice (66). However, it has also been reported that intravascular CX3CR1+KLRG1+ Th1 cells did not migrate into the lungs and were unable to control M. tuberculosis infection (67). Nevertheless, the design of new or improved vaccines that confer sustained sterilizing immunity at mucosal surface will be greatly facilitate by the identification of immunization approaches that induce potent pathogen-specific TRM at the mucosal site of infection.
KM and MW co-authored this article.
The authors declare that the research was conducted in the absence of any commercial or financial relationships that could be construed as a potential conflict of interest.
This work was supported by a Science Foundation Ireland (awards 11/PI/1036 and 16/IA/4468 to KM).
1. Masopust D, Vezys V, Marzo AL, Lefrancois L. Preferential localization of effector memory cells in nonlymphoid tissue. Science (2001) 291(5512):2413–7. doi:10.1126/science.1058867
2. Mackay LK, Braun A, Macleod BL, Collins N, Tebartz C, Bedoui S, et al. Cutting edge: CD69 interference with sphingosine-1-phosphate receptor function regulates peripheral T cell retention. J Immunol (2015) 194(5):2059–63. doi:10.4049/jimmunol.1402256
3. Shiow LR, Rosen DB, Brdickova N, Xu Y, An J, Lanier LL, et al. CD69 acts downstream of interferon-alpha/beta to inhibit S1P1 and lymphocyte egress from lymphoid organs. Nature (2006) 440(7083):540–4. doi:10.1038/nature04606
4. Cepek KL, Shaw SK, Parker CM, Russell GJ, Morrow JS, Rimm DL, et al. Adhesion between epithelial cells and T lymphocytes mediated by E-cadherin and the alpha E beta 7 integrin. Nature (1994) 372(6502):190–3. doi:10.1038/372190a0
5. Collins N, Jiang X, Zaid A, Macleod BL, Li J, Park CO, et al. Skin CD4(+) memory T cells exhibit combined cluster-mediated retention and equilibration with the circulation. Nat Commun (2016) 7:11514. doi:10.1038/ncomms11514
6. Park CO, Fu X, Jiang X, Pan Y, Teague JE, Collins N, et al. Staged development of long-lived T-cell receptor alphabeta TH17 resident memory T-cell population to Candida albicans after skin infection. J Allergy Clin Immunol (2018) 142(2):647–62. doi:10.1016/j.jaci.2017.09.042
7. Wilk MM, Misiak A, McManus RM, Allen AC, Lynch MA, Mills KHG. Lung CD4 tissue-resident memory T cells mediate adaptive immunity induced by previous infection of mice with Bordetella pertussis. J Immunol (2017) 199(1):233–43. doi:10.4049/jimmunol.1602051
8. Bankovich AJ, Shiow LR, Cyster JG. CD69 suppresses sphingosine 1-phosophate receptor-1 (S1P1) function through interaction with membrane helix 4. J Biol Chem (2010) 285(29):22328–37. doi:10.1074/jbc.M110.123299
9. Mackay LK, Minnich M, Kragten NA, Liao Y, Nota B, Seillet C, et al. Hobit and Blimp1 instruct a universal transcriptional program of tissue residency in lymphocytes. Science (2016) 352(6284):459–63. doi:10.1126/science.aad2035
10. Skon CN, Lee JY, Anderson KG, Masopust D, Hogquist KA, Jameson SC. Transcriptional downregulation of S1pr1 is required for the establishment of resident memory CD8+ T cells. Nat Immunol (2013) 14(12):1285–93. doi:10.1038/ni.2745
11. Ruf BR, Knuf M. The burden of seasonal and pandemic influenza in infants and children. Eur J Pediatr (2014) 173(3):265–76. doi:10.1007/s00431-013-2023-6
12. Crowcroft NS, Stein C, Duclos P, Birmingham M. How best to estimate the global burden of pertussis? Lancet Infect Dis (2003) 3(7):413–8. doi:10.1016/S1473-3099(03)00669-8
13. Thome JJ, Bickham KL, Ohmura Y, Kubota M, Matsuoka N, Gordon C, et al. Early-life compartmentalization of human T cell differentiation and regulatory function in mucosal and lymphoid tissues. Nat Med (2016) 22(1):72–7. doi:10.1038/nm.4008
14. Zens KD, Chen JK, Guyer RS, Wu FL, Cvetkovski F, Miron M, et al. Reduced generation of lung tissue-resident memory T cells during infancy. J Exp Med (2017) 214(10):2915–32. doi:10.1084/jem.20170521
15. Mueller SN, Mackay LK. Tissue-resident memory T cells: local specialists in immune defence. Nat Rev Immunol (2016) 16(2):79–89. doi:10.1038/nri.2015.3
16. Sathaliyawala T, Kubota M, Yudanin N, Turner D, Camp P, Thome JJ, et al. Distribution and compartmentalization of human circulating and tissue-resident memory T cell subsets. Immunity (2013) 38(1):187–97. doi:10.1016/j.immuni.2012.09.020
17. Holmkvist P, Roepstorff K, Uronen-Hansson H, Sanden C, Gudjonsson S, Patschan O, et al. A major population of mucosal memory CD4+ T cells, coexpressing IL-18Ralpha and DR3, display innate lymphocyte functionality. Mucosal Immunol (2015) 8(3):545–58. doi:10.1038/mi.2014.87
18. Mackay LK, Wynne-Jones E, Freestone D, Pellicci DG, Mielke LA, Newman DM, et al. T-box transcription factors combine with the cytokines TGF-beta and IL-15 to control tissue-resident memory T cell fate. Immunity (2015) 43(6):1101–11. doi:10.1016/j.immuni.2015.11.008
19. Hondowicz BD, An D, Schenkel JM, Kim KS, Steach HR, Krishnamurty AT, et al. Interleukin-2-dependent allergen-specific tissue-resident memory cells drive asthma. Immunity (2016) 44(1):155–66. doi:10.1016/j.immuni.2015.11.004
20. Turner DL, Goldklang M, Cvetkovski F, Paik D, Trischler J, Barahona J, et al. Biased generation and in situ activation of lung tissue-resident memory CD4 T cells in the pathogenesis of allergic asthma. J Immunol (2018) 200(5):1561–9. doi:10.4049/jimmunol.1700257
21. Laidlaw BJ, Zhang N, Marshall HD, Staron MM, Guan T, Hu Y, et al. CD4+ T cell help guides formation of CD103+ lung-resident memory CD8+ T cells during influenza viral infection. Immunity (2014) 41(4):633–45. doi:10.1016/j.immuni.2014.09.007
22. McKinstry KK, Strutt TM, Kuang Y, Brown DM, Sell S, Dutton RW, et al. Memory CD4+ T cells protect against influenza through multiple synergizing mechanisms. J Clin Invest (2012) 122(8):2847–56. doi:10.1172/JCI63689
23. Swain SL, McKinstry KK, Strutt TM. Expanding roles for CD4(+) T cells in immunity to viruses. Nat Rev Immunol (2012) 12(2):136–48. doi:10.1038/nri3152
24. Teijaro JR, Turner D, Pham Q, Wherry EJ, Lefrancois L, Farber DL. Cutting edge: tissue-retentive lung memory CD4 T cells mediate optimal protection to respiratory virus infection. J Immunol (2011) 187(11):5510–4. doi:10.4049/jimmunol.1102243
25. Turner DL, Bickham KL, Thome JJ, Kim CY, D’Ovidio F, Wherry EJ, et al. Lung niches for the generation and maintenance of tissue-resident memory T cells. Mucosal Immunol (2014) 7(3):501–10. doi:10.1038/mi.2013.67
26. Strutt TM, Dhume K, Finn CM, Hwang JH, Castonguay C, Swain SL, et al. IL-15 supports the generation of protective lung-resident memory CD4 T cells. Mucosal Immunol (2018) 11(3):668–80. doi:10.1038/mi.2017.101
27. McKinstry KK, Strutt TM, Bautista B, Zhang W, Kuang Y, Cooper AM, et al. Effector CD4 T-cell transition to memory requires late cognate interactions that induce autocrine IL-2. Nat Commun (2014) 5:5377. doi:10.1038/ncomms6377
28. Hondowicz BD, Kim KS, Ruterbusch MJ, Keitany GJ, Pepper M. IL-2 is required for the generation of viral-specific CD4(+) Th1 tissue-resident memory cells and B cells are essential for maintenance in the lung. Eur J Immunol (2018) 48(1):80–6. doi:10.1002/eji.201746928
29. Jones CA, Taylor TJ, Knipe DM. Biological properties of herpes simplex virus 2 replication-defective mutant strains in a murine nasal infection model. Virology (2000) 278(1):137–50. doi:10.1006/viro.2000.0628
30. Nakanishi Y, Lu B, Gerard C, Iwasaki A. CD8(+) T lymphocyte mobilization to virus-infected tissue requires CD4(+) T-cell help. Nature (2009) 462(7272):510–3. doi:10.1038/nature08511
31. Iijima N, Iwasaki A. T cell memory. A local macrophage chemokine network sustains protective tissue-resident memory CD4 T cells. Science (2014) 346(6205):93–8. doi:10.1126/science.1257530
32. Mills KH, Barnard A, Watkins J, Redhead K. Cell-mediated immunity to Bordetella pertussis: role of Th1 cells in bacterial clearance in a murine respiratory infection model. Infect Immun (1993) 61(2):399–410.
33. Sakai S, Kauffman KD, Schenkel JM, McBerry CC, Mayer-Barber KD, Masopust D, et al. Cutting edge: control of Mycobacterium tuberculosis infection by a subset of lung parenchyma-homing CD4 T cells. J Immunol (2014) 192(7):2965–9. doi:10.4049/jimmunol.1400019
34. Smith NM, Wasserman GA, Coleman FT, Hilliard KL, Yamamoto K, Lipsitz E, et al. Regionally compartmentalized resident memory T cells mediate naturally acquired protection against pneumococcal pneumonia. Mucosal Immunol (2018) 11(1):220–35. doi:10.1038/mi.2017.43
35. Romagnoli PA, Fu HH, Qiu Z, Khairallah C, Pham QM, Puddington L, et al. Differentiation of distinct long-lived memory CD4 T cells in intestinal tissues after oral Listeria monocytogenes infection. Mucosal Immunol (2017) 10(2):520–30. doi:10.1038/mi.2016.66
36. Johnson RM, Brunham RC. Tissue-resident T cells as the central paradigm of Chlamydia immunity. Infect Immun (2016) 84(4):868–73. doi:10.1128/IAI.01378-15
37. Morrison SG, Morrison RP. In situ analysis of the evolution of the primary immune response in murine Chlamydia trachomatis genital tract infection. Infect Immun (2000) 68(5):2870–9. doi:10.1128/IAI.68.5.2870-2879.2000
38. Johnson RM, Yu H, Strank NO, Karunakaran K, Zhu Y, Brunham RC. B cell presentation of Chlamydia antigen selects out protective CD4γ13 T cells: implications for genital tract tissue-resident memory lymphocyte clusters. Infect Immun (2018) 86(2):e00614-17. doi:10.1128/IAI.00614-17
39. Kopf M, Le Gros G, Bachmann M, Lamers MC, Bluethmann H, Kohler G. Disruption of the murine IL-4 gene blocks Th2 cytokine responses. Nature (1993) 362(6417):245–8. doi:10.1038/362245a0
40. Thawer SG, Horsnell WG, Darby M, Hoving JC, Dewals B, Cutler AJ, et al. Lung-resident CD4(+) T cells are sufficient for IL-4Ralpha-dependent recall immunity to Nippostrongylus brasiliensis infection. Mucosal Immunol (2014) 7(2):239–48. doi:10.1038/mi.2013.40
41. Steinfelder S, Rausch S, Michael D, Kuhl AA, Hartmann S. Intestinal helminth infection induces highly functional resident memory CD4(+) T cells in mice. Eur J Immunol (2017) 47(2):353–63. doi:10.1002/eji.201646575
42. Glennie ND, Yeramilli VA, Beiting DP, Volk SW, Weaver CT, Scott P. Skin-resident memory CD4+ T cells enhance protection against Leishmania major infection. J Exp Med (2015) 212(9):1405–14. doi:10.1084/jem.20142101
43. Glennie ND, Volk SW, Scott P. Skin-resident CD4+ T cells protect against Leishmania major by recruiting and activating inflammatory monocytes. PLoS Pathog (2017) 13(4):e1006349. doi:10.1371/journal.ppat.1006349
44. Conti HR, Peterson AC, Brane L, Huppler AR, Hernandez-Santos N, Whibley N, et al. Oral-resident natural Th17 cells and gammadelta T cells control opportunistic Candida albicans infections. J Exp Med (2014) 211(10):2075–84. doi:10.1084/jem.20130877
45. Stockinger B, Bourgeois C, Kassiotis G. CD4+ memory T cells: functional differentiation and homeostasis. Immunol Rev (2006) 211:39–48. doi:10.1111/j.0105-2896.2006.00381.x
46. Lees JR, Farber DL. Generation, persistence and plasticity of CD4 T-cell memories. Immunology (2010) 130(4):463–70. doi:10.1111/j.1365-2567.2010.03288.x
47. Harcourt GC, Garrard S, Davenport MP, Edwards A, Phillips RE. HIV-1 variation diminishes CD4 T lymphocyte recognition. J Exp Med (1998) 188(10):1785–93. doi:10.1084/jem.188.10.1785
48. Zens KD, Chen JK, Farber DL. Vaccine-generated lung tissue-resident memory T cells provide heterosubtypic protection to influenza infection. JCI Insight (2016) 1(10):e85832. doi:10.1172/jci.insight.85832
49. Zhao J, Zhao J, Mangalam AK, Channappanavar R, Fett C, Meyerholz DK, et al. Airway memory CD4(+) T cells mediate protective immunity against emerging respiratory coronaviruses. Immunity (2016) 44(6):1379–91. doi:10.1016/j.immuni.2016.05.006
50. Milligan GN, Dudley-McClain KL, Chu CF, Young CG. Efficacy of genital T cell responses to herpes simplex virus type 2 resulting from immunization of the nasal mucosa. Virology (2004) 318(2):507–15. doi:10.1016/j.virol.2003.10.010
51. Sato A, Suwanto A, Okabe M, Sato S, Nochi T, Imai T, et al. Vaginal memory T cells induced by intranasal vaccination are critical for protective T cell recruitment and prevention of genital HSV-2 disease. J Virol (2014) 88(23):13699–708. doi:10.1128/JVI.02279-14
52. Wang L, Zhu C, Zhang T, Tian Q, Zhang N, Morrison S, et al. Nonpathogenic colonization with Chlamydia in the gastrointestinal tract as oral vaccination for inducing transmucosal protection. Infect Immun (2018) 86(2):e00630-17. doi:10.1128/IAI.00630-17
53. Stary G, Olive A, Radovic-Moreno AF, Gondek D, Alvarez D, Basto PA, et al. VACCINES. A mucosal vaccine against Chlamydia trachomatis generates two waves of protective memory T cells. Science (2015) 348(6241):aaa8205. doi:10.1126/science.aaa8205
54. Eliasson DG, Omokanye A, Schon K, Wenzel UA, Bernasconi V, Bemark M, et al. M2e-tetramer-specific memory CD4 T cells are broadly protective against influenza infection. Mucosal Immunol (2018) 11(1):273–89. doi:10.1038/mi.2017.14
55. Lapuente D, Storcksdieck Genannt Bonsmann M, Maaske A, Stab V, Heinecke V, Watzstedt K, et al. IL-1β as mucosal vaccine adjuvant: the specific induction of tissue-resident memory T cells improves the heterosubtypic immunity against influenza A viruses. Mucosal Immunol (2018) 11(4):1265–78. doi:10.1038/s41385-018-0017-4
56. Klein NP, Bartlett J, Fireman B, Aukes L, Buck PO, Krishnarajah G, et al. Waning protection following 5 doses of a 3-component diphtheria, tetanus, and acellular pertussis vaccine. Vaccine (2017) 35(26):3395–400. doi:10.1016/j.vaccine.2017.05.008
57. Warfel JM, Zimmerman LI, Merkel TJ. Acellular pertussis vaccines protect against disease but fail to prevent infection and transmission in a nonhuman primate model. Proc Natl Acad Sci U S A (2014) 111(2):787–92. doi:10.1073/pnas.1314688110
58. Locht C, Papin JF, Lecher S, Debrie AS, Thalen M, Solovay K, et al. Live attenuated pertussis vaccine BPZE1 protects baboons against Bordetella pertussis disease and infection. J Infect Dis (2017) 216(1):117–24. doi:10.1093/infdis/jix254
59. Ross PJ, Sutton CE, Higgins S, Allen AC, Walsh K, Misiak A, et al. Relative contribution of Th1 and Th17 cells in adaptive immunity to Bordetella pertussis: towards the rational design of an improved acellular pertussis vaccine. PLoS Pathog (2013) 9(4):e1003264. doi:10.1371/journal.ppat.1003264
60. Dunne A, Mielke LA, Allen AC, Sutton CE, Higgs R, Cunningham CC, et al. A novel TLR2 agonist from Bordetella pertussis is a potent adjuvant that promotes protective immunity with an acellular pertussis vaccine. Mucosal Immunol (2015) 8(3):607–17. doi:10.1038/mi.2014.93
61. Pizzolla A, Nguyen THO, Smith JM, Brooks AG, Kedzieska K, Heath WR, et al. Resident memory CD8(+) T cells in the upper respiratory tract prevent pulmonary influenza virus infection. Sci Immunol (2017) 2(12):eaam6970. doi:10.1126/sciimmunol.aam6970
62. Klein NP, Bartlett J, Rowhani-Rahbar A, Fireman B, Baxter R. Waning protection after fifth dose of acellular pertussis vaccine in children. N Engl J Med (2012) 367(11):1012–9. doi:10.1056/NEJMoa1200850
63. Christensen D, Mortensen R, Rosenkrands I, Dietrich J, Andersen P. Vaccine-induced Th17 cells are established as resident memory cells in the lung and promote local IgA responses. Mucosal Immunol (2017) 10(1):260–70. doi:10.1038/mi.2016.28
64. Shin H, Iwasaki A. A vaccine strategy that protects against genital herpes by establishing local memory T cells. Nature (2012) 491(7424):463–7. doi:10.1038/nature11522
65. Kumar BV, Ma W, Miron M, Granot T, Guyer RS, Carpenter DJ, et al. Human tissue-resident memory T cells are defined by core transcriptional and functional signatures in lymphoid and mucosal sites. Cell Rep (2017) 20(12):2921–34. doi:10.1016/j.celrep.2017.08.078
66. Gerlach C, Moseman EA, Loughhead SM, Alvarez D, Zwijnenburg AJ, Waanders L, et al. The chemokine receptor CX3CR1 defines three antigen-experienced CD8 T cell subsets with distinct roles in immune surveillance and homeostasis. Immunity (2016) 45(6):1270–84. doi:10.1016/j.immuni.2016.10.018
Keywords: memory CD4 T cell, tissue-resident memory T cell, infection, immunization, vaccine, protective immunity, Th1 cell, Th17 cell
Citation: Wilk MM and Mills KHG (2018) CD4 TRM Cells Following Infection and Immunization: Implications for More Effective Vaccine Design. Front. Immunol. 9:1860. doi: 10.3389/fimmu.2018.01860
Received: 17 May 2018; Accepted: 27 July 2018;
Published: 10 August 2018
Edited by:
Eric Tartour, Hôpital Européen Georges-Pompidou, FranceReviewed by:
Karl Kai McKinstry, University of Central Florida, United StatesCopyright: © 2018 Wilk and Mills. This is an open-access article distributed under the terms of the Creative Commons Attribution License (CC BY). The use, distribution or reproduction in other forums is permitted, provided the original author(s) and the copyright owner(s) are credited and that the original publication in this journal is cited, in accordance with accepted academic practice. No use, distribution or reproduction is permitted which does not comply with these terms.
*Correspondence: Kingston H. G. Mills, a2luZ3N0b24ubWlsbHNAdGNkLmll
Disclaimer: All claims expressed in this article are solely those of the authors and do not necessarily represent those of their affiliated organizations, or those of the publisher, the editors and the reviewers. Any product that may be evaluated in this article or claim that may be made by its manufacturer is not guaranteed or endorsed by the publisher.
Research integrity at Frontiers
Learn more about the work of our research integrity team to safeguard the quality of each article we publish.