- 1Key Laboratory of Diagnostic Medicine Designated by the Ministry of Education, Chongqing Medical University, Chongqing, China
- 2School of Laboratory Medicine, Chongqing Medical University, Chongqing, China
- 3Department of Ultrasound, The First Affiliated Hospital of Chongqing Medical University, Chongqing, China
- 4Department of Clinical Laboratory, Mianyang Central Hospital, Mianyang, Sichuan, China
- 5Department of Laboratory Medicine, The First Affiliated Hospital of Chongqing Medical University, Chongqing, China
Vaccine effectiveness is mainly determined by the mechanism mediating protection, emphasizing the importance of unraveling the protective mechanism for novel pneumococcal vaccine development. We previously demonstrated that the regulatory T cell (Treg) immune response has a protective effect against pneumococcal infection elicited by the live-attenuated pneumococcal vaccine SPY1. However, the mechanism underlying this protective effect remains unclear. In this study, a short synthetic peptide (P17) was used to downregulate Tregs during immunization and subsequent challenges in a mouse model. In immunized mice, increase in immune cytokines (IL-12p70, IL-4, IL-5, and IL-17A) induced by SPY1 were further upregulated by P17 treatment, whereas the decrease in the infection-associated inflammatory cytokine TNF-α by SPY1 was reversed. P17 also inhibited the increase in the immunosuppressive cytokine IL-10 and inflammatory mediator IL-6 in immunized mice. More severe pulmonary injuries and more dramatic inflammatory responses with worse survival in P17-treated immunized mice indicated the indispensable role of the Treg immune response in protection against pneumococcal infection by maintaining a balance among acquired immune responses stimulated by SPY1. Further studies revealed that the significant elevation of active transforming growth factor β (TGF-β)1 by SPY1 vaccination activated FOXP3, leading to increased frequencies of CD4+CD25+Foxp3+ T cells. Moreover, SPY1 vaccination elevated the levels of Smad2/3 and phosphor-Smad2/3 and downregulated the negative regulatory factor Smad7 in a time-dependent manner during pneumococcal infection, and these changes were reversed by P17 treatment. These results illustrate that SPY1-stimulated TGF-β1 induced the generation of SPY1-specific Tregs via the Smad2/3 signaling pathway. In addition, SPY1-specific Tregs may participate in protection via the enhanced expression of PD-1 and CTLA-4. The data presented here extend our understanding of how the SPY1-induced acquired Treg immune response contributes to protection elicited by live-attenuated vaccines and may be helpful for the evaluation of live vaccines and other mucosal vaccine candidates.
Introduction
Streptococcus pneumoniae, an important opportunistic pathogen that colonizes the human oral and nasopharyngeal cavities, is the leading cause of pneumonia in the elderly, immunocompromised, and children younger than 5 years (1, 2). Vaccination is an effective means of preventing pneumococcal disease (3). Currently, both injection and the intranasal administration of pneumococcal vaccines are recommended by the WHO (4). However, intranasal administration can stimulate mucosal immune responses, unlike the conventional systemic delivery of vaccines using a needle and syringe. Furthermore, the mucosal delivery of vaccines can induce systemic immunity, similar to that induced by injection-based vaccination. Mucosal vaccination is pain-free, reduces the risk of needle reuse, and reduces the burden on healthcare professionals, among other benefits (5). However, commercial pneumococcal polysaccharide vaccines and conjugate vaccines are constrained by limited serotype coverage, high-cost, and vaccine serotype replacement (6, 7); accordingly, it is necessary to develop novel vaccine candidates who can overcome these disadvantages. Due to the strong antigenicity and comprehensive serotype coverage promised by a wide range of antigenic molecules, whole-cell pneumococcal vaccines are regarded as ideal vaccine candidates (8, 9). Importantly, vaccine effectiveness is mainly dependent on the underlying protective mechanism; therefore, unraveling this mechanism is critical for novel pneumococcal vaccine development.
Streptococcus pneumoniae strain SPY1 is a live-attenuated pneumococcal vaccine. We have systematically described the extremely reduced virulence, reliable genetic stability, high safety, and excellent protection against pneumococcal infection in a mouse model (10). Humoral and Th2–Th17 T cell immune responses are indispensable for the protection induced by SPY1 (11). We have also surprisingly detected a protective role of the regulatory T cell (Treg) immune response elicited by SPY1, which has not previously been described for S. pneumoniae vaccines (11). These findings highlight the importance of the development of novel pneumococcal vaccines that can induce a protective Treg response, which is vital for the maintenance of immune homeostasis as well as for limiting infection-associated inflammation and facilitating the resolution of tissue damage post-infection. However, the mechanism underlying the activation of the SPY1-induced Treg response is still unknown.
As a pleiotropic cytokine, transforming growth factor β (TGF-β) is essential for the differentiation of Tregs in innate immunity (12). TGF-β could induce the expression of Foxp3 and the conversion of CD4+CD25− T cells to CD4+CD25+ T cells, promoting Treg proliferation and subsequent immunosuppression (13–15). In our previous study, a short peptide P17 (KRIWFIPRSSWYERA) was introduced to inhibit the production of TGF-β1 and to downregulate Tregs (11, 16, 17). As expected, treatment with P17 significantly impairs the effectiveness of SPY1 in colonization and invasive infection models, suggesting the importance of SPY1-induced TGF-β1 for the protective Treg immune response in acquired immunity. Several signaling pathways, including Smad-dependent and Smad-independent pathways, have potential roles in the activation of Tregs mediated by activated TGF-β1, and the specific functional pathway varies among experimental models (18–21). Previous research has shown that the percentage of Tregs is elevated in SPY1-vaccinated mice; however, signaling mechanism by which TGF-β1 mediates the differentiation of Tregs is unknown.
In this study, the mechanism underlying the protective Treg response activated by vaccination with SPY1 was explored. The inhibition of TGF-β1 dramatically attenuated the SPY1-induced protection against pulmonary injuries caused by pneumococcal colonization. In addition, SPY1-induced TGF-β1 is essential for the balance among systemic protective immune responses triggered by SPY1 vaccination. The activation of the TGF-β1-Smad2/3 signaling pathway is responsible for the generation of Tregs, which are involved in SPY1 protection via the elevated expression of CTLA-4 and PD-1. These findings and those of our previous studies provide insight into the proximal mechanism mediating the protection elicited by the SPY1-induced acquired Treg immune response and may contribute to a more comprehensive evaluation of live vaccines and other mucosal vaccines.
Materials and Methods
Mice
Female C57BL/6 mice (6–8 weeks) were purchased from the animal center of Chongqing Medical University, Chongqing, China. Mice were kept under specific pathogen-free conditions at the animal facilities of Chongqing Medical University during the time of the experiments. All animal experiments were performed in accordance with the guidelines of the Institutional Animal Care and Use Committee of Chongqing Medical University.
Bacterial Strains and Immunogen Preparation
Streptococcus pneumoniae strain NCTC 7466 (D39, serotype 2) was obtained from the National Collection of Type Cultures (NCTC; London, UK). The S. pneumoniae clinical isolate CMCC 31693 (serotype 19F) was obtained from the National Center for Medical Culture Collections (CMCC; Beijing, China). SPY1 is a novel live-attenuated S. pneumoniae vaccine candidate strain with definite serotype-independent protection against pneumococcal infection (10), and its basic protective mechanism has been described in our previous research (11). All pneumococcal strains were grown in casein-based medium with yeast extract (C + Y medium) or on Columbia sheep blood agar plates at 37°C in 5% CO2. To prepare the immunogens, SPY1 was grown at 37°C in 5% CO2 in C + Y medium to approximately 2 × 108 CFU/ml. After centrifugation and washing (twice), sediments were resuspended in sterile phosphate-buffered saline (PBS). The final vaccine mixture for routine immunization contained 1 × 108 CFU of SPY1 and 1 µg of adjuvant cholera toxin (CT; Sigma-Aldrich, St. Louis, MO, USA) per 20-µl dose.
Immunization of Mice and Challenge
C57BL/6 mice were anesthetized with ethyl ether and then intranasally administered the vaccine (group CT + SPY1) or adjuvant alone (group CT) four times at 1-week intervals. One week after the last vaccination, mice were intranasally challenged with either strain 19F (1 × 108 CFU) or strain D39 (1 × 108 CFU). To investigate the roles and related mechanisms of the SPY1-induced Treg immune response, as shown in Figure 1A, during the whole vaccination period of 28 days, half of the mice in the CT + SPY1 group were randomly chosen and treated with an intraperitoneal injection of 100 µg of peptide P17 daily to downregulate Tregs (group CT + SPY1 + P17), as described previously (11). The other half of mice in the CT + SPY1 group received sterile PBS as a control. Similarly, mice from CT group were also injected with P17 or PBS daily. In addition to 28 days of P17 treatment, on the day of challenge, the P17-treated mice received 500 µg of P17 twice at 2 and 4 h before challenge (Figure 1A). The peptide P17 (KRIWFIPRSSWYERA; purity >95%, as determined by high performance liquid chromatography) was synthesized by GL Biochem (Shanghai, China).
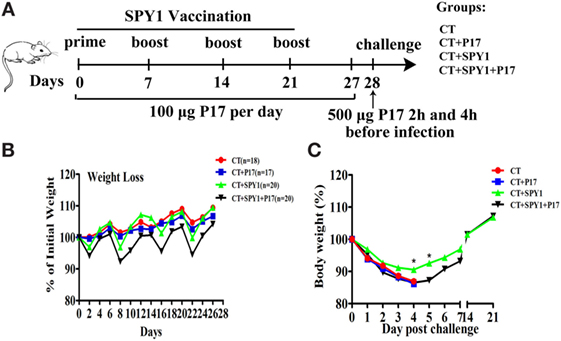
Figure 1. Regulatory T cell (Treg) downregulator P17 eliminates SPY1-elicited protection against invasive S. pneumonia infection. (A) Mice were intranasally immunized with SPY1 plus CT or with CT alone for four times at 1-week intervals. During the whole vaccination progress of 28 days in all, half of mice from group CT + SPY1 were randomly chosen to be intraperitoneally injected with 100 µg of peptide P17 daily and the other half of mice in group CT + SPY1 received sterile phosphate-buffered saline (PBS) as control. Similarly, mice from CT group were also injected with P17 or PBS daily. On day 28, mice were intranasally challenged with pneumococcal strains. (B) Mice body weight were monitored every other day during the vaccination procedure, and the weight data were expressed as the percentages of initial mice weights measured at day 0 of vaccination. On day 28, mice were intranasal challenged with 1 × 108 CFU of pneumococcal strain D39, and the body weights of live mice were monitored for consecutive 21 days after infection (C). *p < 0.05.
Flow Cytometry
Mouse lungs were removed and cell suspensions were prepared. For Treg (CD4+CD25+Foxp3+) detection, lung cells were first incubated with CD16/CD32 and stained with anti-mouse CD4-FITC (clone RM4-5; eBioscience, San Diego, CA, USA) and anti-mouse CD25-APC (clone PC61.5; eBioscience). Then, these cells were fixed and permeabilized in Fix/Perm buffer and subsequently incubated with anti-mouse Foxp3-PE (clone FJK-16S; eBioscience), according to the instructions provided with the Mouse Regulatory T Cell Staining Kit (eBioscience) for the intracellular Foxp3 analysis. To analyze the expression of PD-1 and CTLA-4, cells were stained with anti-mouse CD279 (PD-1)-APC/Cy7 (clone 29F.1A12; BioLegend, San Diego, CA, USA) and anti-mouse CD152 (CTLA-4)-PerCP/Cy5.5 (clone UC10-4B9; BioLegend). All the samples were analyzed using a Becton Dickinson FACSCalibur flow cytometer (Franklin Lakes, NJ, USA).
RNA Extraction and Quantitative Real-Time PCR
Mouse lungs were removed at different time points after pneumococcal 19F challenge, and total RNA was extracted using RNAiso Plus reagent (Takara Bio, Dalian, China) following the manufacturer’s instructions. For reverse transcription, 1 µg of total RNA was reverse-transcribed into cDNA, and the PrimeScript™ RT Reagent Kit (Takara Bio) was used for reverse transcription. PCR amplification was performed on the Bio-Rad CFX96 Real-Time system using SYBR Premix Ex Taq™ (Takara Bio). The 2−ΔΔCt method was used to determine the specific Ct value of each target gene. Quantitative real-time PCR for each target gene were repeated three times. The PCR primers were synthesized by Sangon (Shanghai, China) and the sequences are listed in Table S1 in Supplementary Material. All genes were murine in origin.
Protein Extraction and Western Blot Analysis
Lungs were collected at different time points after pneumococcal 19F challenge, and proteins were extracted by adding the appropriate volume of whole-cell lysates [RIPA buffer (Beyotime, Shanghai, China): PMSF: phosphatase inhibitor (BioTools, Jupiter, FL, USA) = 100:10:1]. For the detection of Smad2/3, phosphor-Smad2/3, and Smad7 at the protein levels, the same concentrations of total cellular extracts were separated by 10% SDS-PAGE, subjected to electrophoresis, and transferred to PVDF membranes (Millipore, Bedford, MA, USA) by electroblotting. After they were blocked with 5% skim milk for 2 h at room temperature, membranes were incubated overnight at 4°C with primary antibodies, including anti-mouse β-actin, anti-mouse Smad2/3, anti-mouse phosphor-Smad2/3 (Cell Signaling Technology, Danvers, MA, USA), and anti-mouse Smad7 antibodies (Abcam, Cambridge, UK), followed by incubation with an HRP-conjugated secondary antibody for 1 h at room temperature. The Enhanced Chemiluminescence (ECL) Western Blotting System (GE Healthcare, Little Chalfont, UK) was used to detect the target bands. The band intensities were quantified using Quantity One (Bio-Rad Laboratories, Hercules, CA, USA).
Cytokine Assays
Mouse lungs and spleens were fully homogenized and centrifuged, and the supernatants were collected for cytokine measurement. In addition, mouse splenocytes were plated in 24-well tissue culture plates in 1 ml of DMEM with 10% fetal calf serum (HyClone, Logan, UT, USA) (2 × 107 cells/well) after red blood cells were removed by hemolysis. Cultured splenocytes were stimulated with 70% ethanol-inactivated SPY1 (equivalent to 107 CFU/ml), and the supernatants were harvested at different time points for cytokine measurement. Levels of IL-6, TNF-α, IL-12p70, IFN-γ, IL-4, IL-5, IL-17A, and IL-10 in homogenate supernatants and splenocyte supernatants were measured using an enzyme-linked immunosorbent assay (ELISA) kit (BioLegend) in accordance with the manufacturer’s protocols. Samples were diluted when required.
Immunofluorescence Assay
At 24 h post-pneumococcal 19F challenge, mouse lungs were removed and fixed in 4% paraformaldehyde for 24 h and permeated in 20% sucrose for 24 h. Then, the tissues were frozen in OCT at −20°C and cryo-cut for slides. For Smad2/3 staining, after thawing and blocking with goat serum and subsequent washing with PBS, slides were covered with an anti-Smad2/3 antibody (Cell Signaling Technology) at 4°C overnight, followed by incubation with Fluorescein-Conjugated Goat Anti-Rat IgG (ZSGB-bio, Beijing, China). DAPI was used to stain cell nuclei for 2 h at room temperature in the dark. The cell morphology and fluorescence intensities were observed using an ECLIPSE 80i microscope equipped with a Nikon INTENSILIGHT C-HGFI. The percentages of lung cells with positive Smad2/3 expression were calculated by counting 100 cells per slide (three mice per group).
Lung Histology and Immunohistochemistry
On day 7 after the last immunization, mice were intranasally challenged with 1 × 108 CFU of pneumococcal strain 19F. Mice were sacrificed and lung tissues were removed at 6, 12, and 24 h post-infection. After fixation in buffered 10% formalin, lungs were sectioned and embedded in paraffin, and 5-µm sections were cut. The sections were stained with hematoxylin and eosin (Sigma-Aldrich) and then examined using a light microscope. The degrees of peribronchial inflammation were graded semi-quantitatively following previously described methods (22).
For immunohistochemistry, sections were retrieved in citrate buffer for 5 min. After natural cooling, sections were incubated with 3% H2O2 and washed three times with PBS, followed by incubation with an anti-FOXP3 antibody (BioLegend) and Rabbit anti-TGF-β1 polyclonal antibody (OmnimAbs, Alhambra, CA, USA) and treatment with streptavidin horseradish peroxidase chemistry according to standard protocols. The mean IODs (integral optical density) of TGF-β1 expression were measured using Image-Pro Plus (Media Cybernetics, Silver Spring, MD, USA).
Data Analysis and Statistics
Statistical analyses were performed using GraphPad Prism 5 (GraphPad Software, La Jolla, CA, USA). Unpaired Student’s t-tests were used to compare two independent groups. One-way ANOVA was utilized for multiple comparisons. Differences at p < 0.05 were considered statistically significant.
Results
Treg Downregulator P17 Eliminates SPY1-Elicited Protection Against Invasive S. pneumoniae Infection
In this study, female C57BL/6 mice were intranasally immunized according to the vaccination schedule described in Figure 1A. During the vaccination process, half of the immunized mice were intraperitoneally injected with 100 µg of the short synthetic peptide P17 to downregulate Treg activity, and the body weights of all mice were observed every other day. Compared with the CT group, mice in the SPY1 group lost more body weight in the first several days after the first vaccination, and body weight in the of SPY1 group recovered quickly to the level of the CT group (Figure 1B). However, we observed greater body weight loss in mice in the CT + SPY1 + P17 group than in the CT + SPY1 group during the vaccination period (Figure 1B), which may be due to the disruption of the immune regulatory response caused by P17 administration.
On day 28, mice were intranasally challenged with S. pneumoniae strain D39. Greater body weight loss was observed in non-immunized mice than in immunized mice on day 4 post-challenge (Figure 1C). Beginning at day 5 post-D39 infection, body weights of mice belonging to the SPY1-immunized group began to increase, finally recovering to the weights recorded before infection, illustrating the protection elicited by SPY1, to some extent (Figure 1C). The body weights of mice in the P17-treated SPY1-immunized group also recovered on day 5 post-infection; however, the degree of body weight increase was significantly lower than that in the SPY1-immunized group (Figure 1C). More importantly, compared with other mouse groups, SPY1-immunized mice survived for longer after lethal S. pneumoniae strain D39 infection (Figure S1 in Supplementary Material), consistent with our previous results (11). Collectively, these data demonstrated that the Treg downregulator P17 can markedly eliminate SPY1-elicited protection against invasive S. pneumoniae infection, highlighting the importance of the protective Treg immune response.
P17 Treatment Disturbs the Immune Responses Triggered by SPY1 Vaccination
To further investigate the influence of P17 administration on the specific immune responses induced by SPY1, concentrations of immune and inflammatory cytokines in mouse splenocyte supernatants after stimulation by inactivated SPY1 were determined on day 7 after the last immunization. Coincident with our previous results (11), stimulation with inactivated SPY1 induced higher levels of IL-6, IL-12p70, IL-4, IL-5, and IL-17A in immunized mice than in non-immunized mouse (Figure 2). Elevated levels of IL-10 in immunized mice indicated the activation of an immunoregulatory response elicited by SPY1 (Figure 2). We detected a disturbance in the SPY1-activated immune responses in P17-treated immunized mice, which was attributed to the inhibition of immunosuppressive Tregs by P17. The increase in immune cytokines (IL-12p70, IL-4, IL-5, and IL-17A) induced by SPY1 were further upregulated by P17 treatment, whereas the decrease in the infection-associated inflammatory cytokine TNF-α by SPY1 was reversed. Furthermore, P17 also inhibited the increase in the immunosuppressive cytokine IL-10 and inflammatory mediator IL-6 (23) in immunized mice (Figure 2).
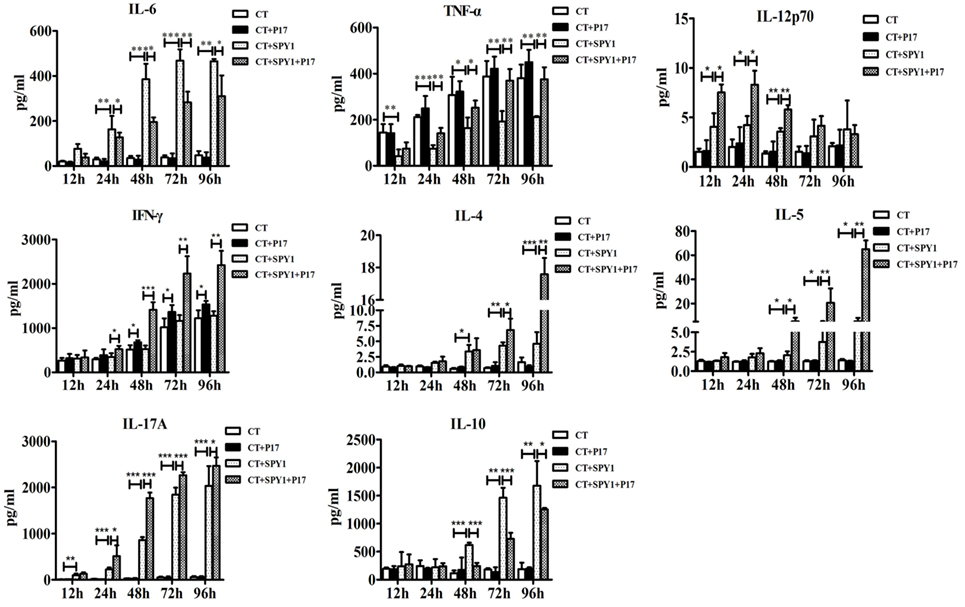
Figure 2. P17 treatment strikes the balance among systemic protective immune responses triggered by SPY1 vaccination. On day 7 after the last immunization, mice splenocytes were isolated and stimulated with 70% ethanol-inactivated SPY1. Splenocytes treated with Concanavalin A served as positive control. Concentrations of cytokines including IL-6, TNF-α, IL-12p70, IFN-γ, IL-4, IL-5, IL-17A, and IL-10 in supernatants of stimulated splenocytes were analyzed by enzyme-linked immunosorbent assay. All data were presented as the mean ± SD of three independent experiments. *p < 0.05; **p < 0.01; ***p < 0.001.
P17 Treatment Significantly Impairs SPY1-Induced Protection Against Pulmonary Injury Caused by Pneumococcal Colonization
Previously, we established the negative effect of P17 on SPY1-specific protection against S. pneumoniae infection, including improved survival rates and reduced bacterial loads in the nasopharynx and lungs (11). In this study, to explore the protective roles of the SPY1-stimulated Treg immune response in more detail, differences in mouse pulmonary damage after pneumococcal colonization among groups were evaluated based on lung morphological observations and histopathological analyses. Moderate inflammatory cell recruitment to the pulmonary interstitium around airways and blood vessels and slight damage to alveolar structural integrity were observed in SPY1-immunized mice post-intranasal challenge with pneumococcal strain 19 F (Figures 3A,B; Figure S2 in Supplementary Material). By contrast, more severe pulmonary injuries were found in the CT control group, with extensive inflammatory cell infiltration in peribronchial as well as perivascular spaces and in the alveoli, disappearance of alveolar structural integrity, and obvious hemorrhage. Similar serious pulmonary injuries were also detected in P17-treated immunized mice, since the immune modulatory response was markedly suppressed. Consistent with these results, compared with other groups, a remarkably lower peribronchial inflammation score was observed in SPY1-immunized mice (Figure 3C). Simultaneously, pulmonary levels of cytokines representing different immune responses triggered by SPY1 vaccination were evaluated. As shown in Figure 3D, compared with non-immunized mice, a decrease in the inflammatory cytokine TNF-α and increase in immune cytokines, including IL-6, IL-12p70, IL-4, IL-5, IL-17A, and IL-10, were found in immunized mouse lung homogenates, indicating elevated, but controlled SPY1-induced immune responses, which are beneficial for fighting against pneumococcal colonization. By contrast, due to the downregulation of the immune-suppressive cytokine IL-10 and consequent immune imbalance caused by P17 treatment, mice showed drastic and uncontrolled pulmonary immune responses as well as infection-associated inflammation, characterized by even higher concentrations of TNF-α, IL-12p70, IFN-γ, IL-4, IL-5, and IL-17A (Figure 3D), consistent with the degrees of pulmonary injury described above.
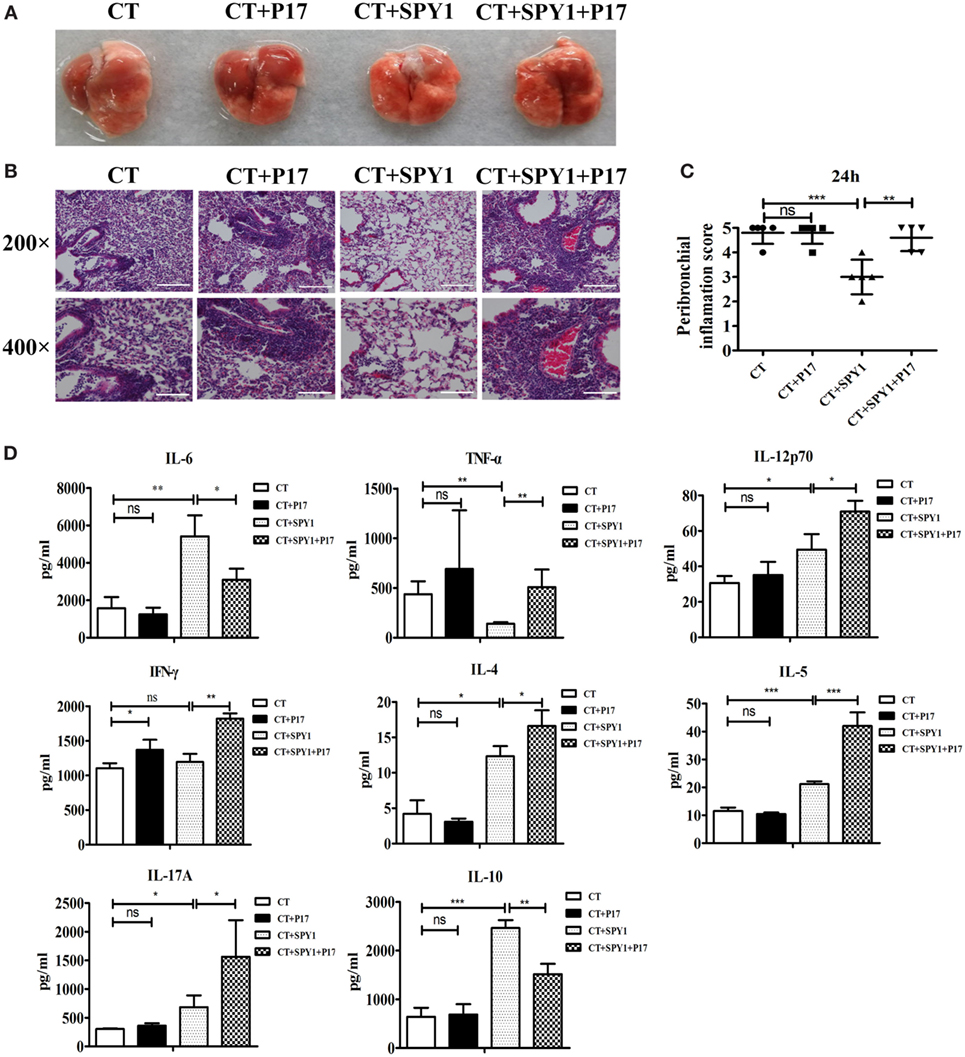
Figure 3. P17 treatment significantly impairs the SPY1-induced acquired protection against pulmonary injury caused by pneumococcal colonization. On day 7 after the last immunization, mice were intranasal challenged with 1 × 108 CFU of pneumococcal strain 19F. Mice were sacrificed and lung tissues were removed at 24 h post-intranasal infection with pneumococcal strain 19F. (A) Lungs appearance post-infection. (B) Pathological analyses were done by hematoxylin and eosin staining, with lung sections examined under light microscopy at 200× (scale bar = 100 µm) and 400× (scale bar = 50 µm) magnification. (C) Scores of peribronchial inflammations were semi-quantitatively graded, and data were shown as mean ± SD of scores of five mice per group. (D) Concentrations of cytokines including IL-6, TNF-α, IL-12p70, IFN-γ, IL-4, IL-5, IL-17A, and IL-10 in lung homogenates collected at 48 h post-infection were detected by enzyme-linked immunosorbent assay, and the data were shown as mean ± SD (each experiment was individually performed three times). *p < 0.05; **p < 0.01; ***p < 0.001.
SPY1 Vaccination Activates the Expression of the Treg Molecule FOXP3
In our previous study, we were surprised to detect a protective role of the SPY1-specific Treg immune response during pneumococcal infection (11). In this study, to comprehensively analyze the mechanism underlying the activation of Tregs by vaccination, the expression of the transcription factor Foxp3, a characteristic molecule in the Treg immune pathway, was first examined. Flow cytometry results showed a significant increase (p < 0.01) in CD4+CD25+Foxp3+ T cells in immunized mouse lungs; however, P17 treatment could reverse the increase in Foxp3-positive cells to a level similar to that in the CT control group (Figure 4A). More importantly, the foxp3 mRNA level was significantly higher in SPY1-immunized mice than in the CT control group, and the upregulation was reversed by P17 treatment (Figure 4B). The inhibition of FOXP3 in SPY1-vaccinated mice by P17 treatment was also verified by immunohistochemical staining of lung tissues (Figure 4C). Thus, these results demonstrated that SPY1 triggers a FOXP3 response.
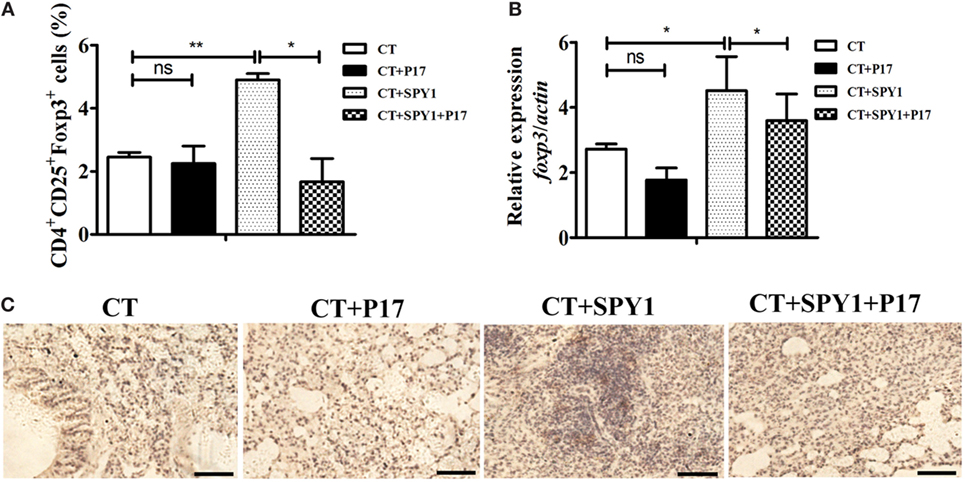
Figure 4. SPY1 vaccination activates the expression of regulatory T cells (Tregs) molecule Foxp3. (A) On day 7 after the last immunization, mice lungs were aseptically removed and homogenized, and single lung cell was stained with anti-mouse CD4-FITC and anti-mouse CD25-APC, followed by anti-mouse Foxp3-APC according to the manufacturer’s instructions. Cells were analyzed using a Becton Dickinson FACSCalibur flow cytometer then. The percentages of CD4+ T cells which were CD25+Foxp3+ Treg were calculated. (B) On day 7 after the last immunization, mice lungs were aseptically removed, and total RNA were extracted. Expression of foxp3 mRNA was analyzed by quantitative real-time PCR. Data were shown as mean ± SD from three independent experiments. (C) On day 7 after the last immunization, mice lungs were aseptically removed, and lung sections were stained with anti-FOXP3 antibody followed by streptavidin horseradish peroxidase chemistry. Then, the sections were examined under light microscopy at 100× magnification. Scale bar = 100 µm. Images were representative of staining observed in the lungs of mice within the group (n = 4–6 mice). *p < 0.05; **p < 0.01.
SPY1 Vaccination Stimulates TGF-β1 Production
Transforming growth factor β is a vital cytokine for the differentiation of Tregs (14). To identify the mechanism underlying the generation of SPY1-specific Tregs, the production of TGF-β1, one of the three isoforms expressed mainly in the immune system in immunized mice, was evaluated. As shown in Figures 5A,B, substantial increases in the concentrations of active TGF-β1 in lung homogenates as well as spleen homogenates in immunized mice were observed by ELISA. Splenocytes from mice immunized with SPY1 expressed significantly more active TGF-β1 in response to inactivated SPY1 in vitro than that of splenocytes from CT-treated control mice (Figure 5C), illustrating the stimulation of TGF-β1. Further verifying the production of SPY1-stimulated TGF-β1, tgf-β1 mRNA, levels were higher in immunized mouse lungs after intranasal infection with pneumococcal strain 19F than in the CT-treated control group, reaching peak levels at 12 h post-infection (Figure 5D). Immunohistochemical staining of mouse lung tissues with TGF-β1 antibodies also proved the activation of TGF-β1 by vaccination with SPY1 (Figures 5E,F; Figure S3 in Supplementary Material), with a remarkable increase in the percentage of TGF-β1-positive lung cells from SPY1-immunized mice in a time-dependent manner (Figure 5G). More importantly, all of the trends indicating an increase in active TGF-β1 in immunized mice were obviously inhibited by the administration of P17, providing further evidence that SPY1 vaccination could stimulate the production of TGF-β1.
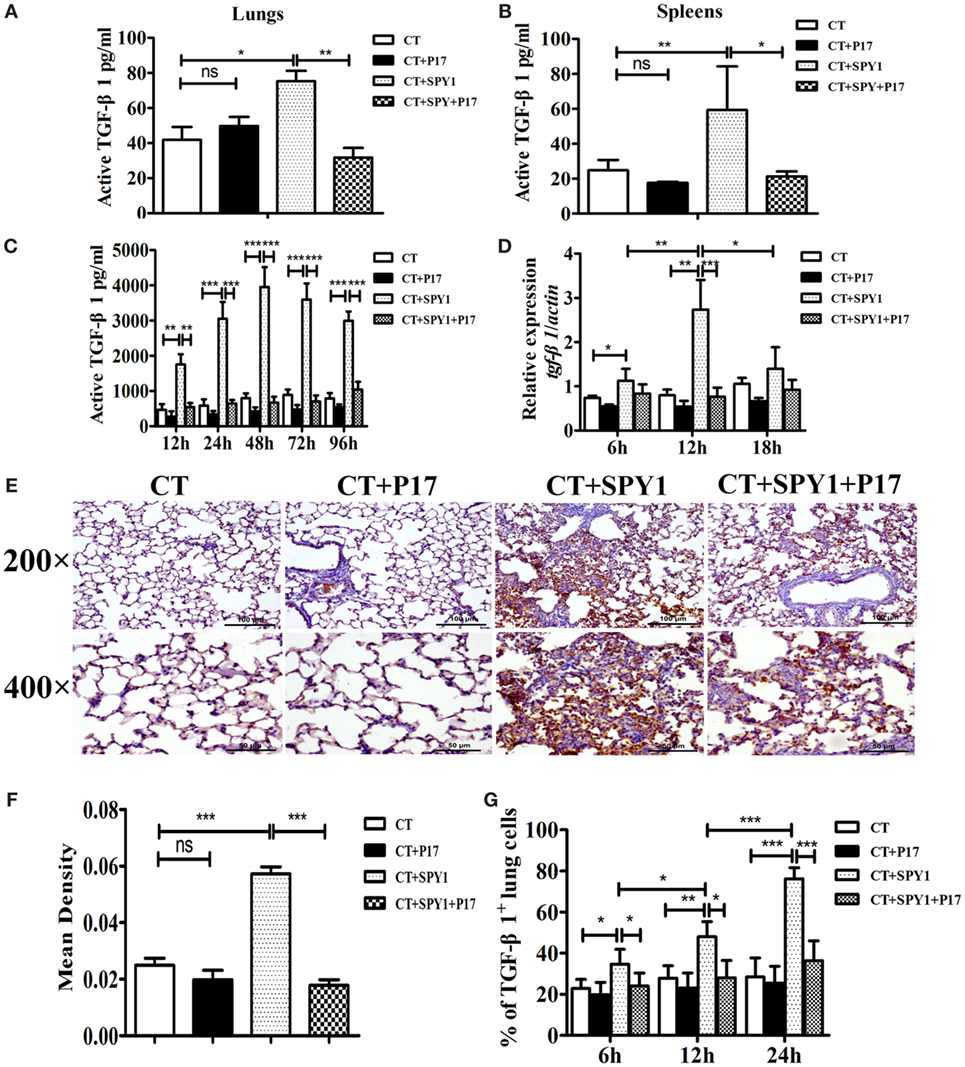
Figure 5. SPY1 vaccination stimulates the production of transforming growth factor β (TGF-β)1. On day 7 after the last immunization, mice were sacrificed and concentrations of active TGF-β1 in lungs (A) and spleens (B) were determined by enzyme-linked immunosorbent assay (ELISA). (C) Splenocytes were isolated and stimulated with 70% ethanol-killed SPY1, and the level of active TGF-β1 in splenocytes supernatants was also detected by ELISA. (D) Mice were intranasal challenged with 1 × 108 CFU of pneumococcal strain 19F on day 7 after the last immunization, and expression of tgf-β1 mRNA in lungs were analyzed by quantitative real-time PCR at 6, 12, and 18 h post-infection, respectively. (E) At 24 h post-pneumococcal 19F infection, immunohistochemical staining with anti-TGF-β1 polyclonal antibody was utilized to detect expression of TGF-β1 in lung tissues. Lung sections were examined under light microscopy at magnification 200× (scale bar = 100 µm) and 400× (scale bar = 50 µm). (F) The mean IODs of TGF-β1 expression were measured and calculated by Image-Pro Plus. Data were shown as mean ± SD of IODs of five mice per group. (G) Expressions of TGF-β1 in mice lungs at 6, 12, and 24 h post 19F infection were determined by immunohistochemical staining, and percentages of lung cells with positive TGF-β1 expressions were calculated by counting 100 cells of each lung section with three slides per time point (three mice per group). Data were shown as the mean ± SD from three independent experiments. *p < 0.05; **p < 0.01; ***p < 0.001.
TGF-β1-Smad2/3 Signaling Participates in the Generation of SPY1-Specific Tregs
Classic TGF-β-inducing intracellular signaling is mediated by SMAD family proteins, and the differentiation of Tregs from naïve T cells is mainly regulated by the TGF-β/Smad pathway (24). To explore the specific signaling pathway involving in the differentiation of SPY1-induced Tregs, dynamic changes in Smad mRNA in mouse lungs post-pneumococcal infection were detected. As shown in Figures 6A–C, compared with CT-treated mice, the expression levels of smad2, smad3, and smad4 mRNA were substantially higher in immunized mice at 6 and 12 h post-pneumococcal strain 19F infection and were also significantly higher than those in P17-treated SPY1-vaccinated mice. A relatively low level of smad7, which is a negative regulator in the TGF-β signaling pathway, was detected in immunized mice (Figure 6D). Moreover, P17 treatment significantly upregulated the expression of smad7 in the immunized group, since P17 injection could block TGF-β signaling. Furthermore, total Smad2/3, phosphorylated Smad2/3, and Smad7 protein expression levels were analyzed by western blotting (Figures 6E–H). Consistent with the observed mRNA expression changes, the expression of Smad2/3 was upregulated in immune mice and downregulated in P17-injected mice at 12 and 18 h post-infection. The stimulation of the TGF-β1/Smad pathway in mouse lungs by SPY1 vaccination was also established by an immunofluorescence assay, indicating significant differences in the percentages of cells expressing Smad2/3 between immunized mice and P17-treated immunized mice (Figures 6I,J). We further evaluated whether other SMAD-independent pathways were involved in the stimulation of SPY1-specific Tregs, but the mRNA expression levels of various signaling molecules including p38 mapk, akt, mtor, and pi3k, did not differ significantly among groups (data not shown). Taken together, these results confirmed that SPY1 vaccination could activate the TGF-β1-Smad2/3 signal pathway to induce a SPY1-specific Treg response and, as a consequence, protection during pneumococcal infection.
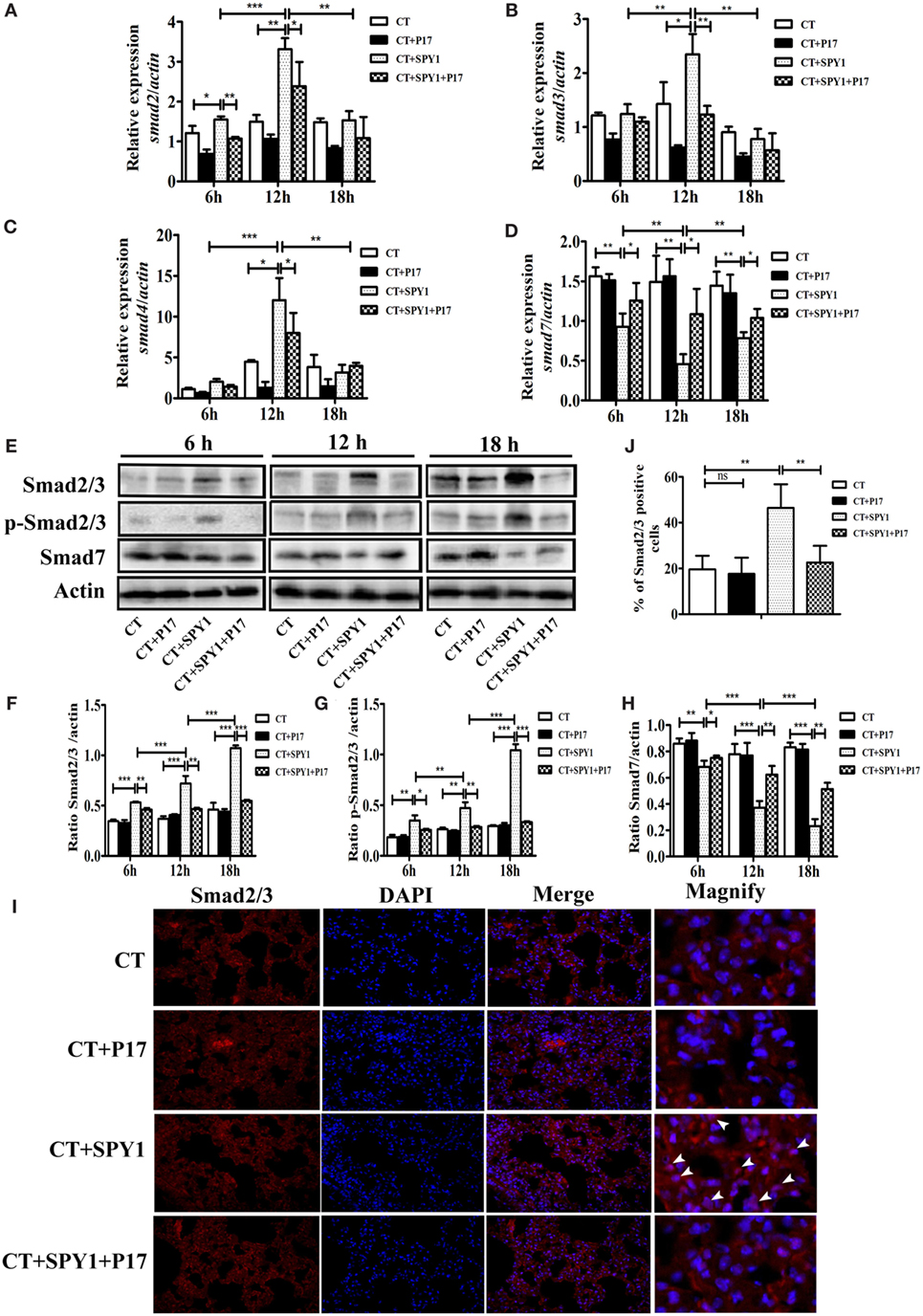
Figure 6. Transforming growth factor β (TGF-β)1-Smad2/3 signaling participates in generation of SPY1-specific regulatory T cells (Tregs). Mice were intranasal challenged with pneumococcal strain 19F on day 7 after the last immunization, and the lungs were aseptically removed at 6, 12, and 18 h post-infection. (A–D) Expression of smad2, smad3, smad4, and smad7 in lungs were analyzed by quantitative real-time PCR. The productions of Smad2/3, phosphor-Smad2/3, and Smad7 in lungs were, respectively, determined by western blot (E) and the related band intensities were shown in histograms (F–H). (I) The expression of Smad2/3 in lungs at 24 h post-infection was examined by immunofluorescence assay with anti-Smad2/3 antibody. Lung sections were examined under light microscopy at magnification 400×. (J) Statistical analysis of the percentages of lung cells with positive Smad2/3 expression was performed by counting 100 cells per slide (three mice per group). Data were shown as the mean ± SD from three independent experiments. *p < 0.05; **p < 0.01; ***p < 0.001.
SPY1 Vaccination Stimulates the Elevated Expression of PD-1 and CTLA-4 on Tregs in Immunized Mice
As an immune response balance factor, Tregs play roles in immunosuppression via multiple Treg-associated cell surface molecules and secreted molecules (25). Therefore, to investigate the specific effectors of SPY1-induced Tregs, the expression of PD-1 and CTLA-4 on CD4+CD25+Foxp3+ cells was examined by flow cytometry (Figure 7A). As shown in Figures 7B,C, the percentages of PD-1+CD4+CD25+Foxp3+ and CTLA-4+CD4+CD25+Foxp3+ cells were both significantly higher in SPY1-immunized mice than in the CT control group, while treatment with P17 (CT + SPY1 + P17 group) reversed the upregulation of immunosuppressive factors. Taken together, these results indicated that PD-1 and CTLA-4 are involved in the SPY1-specific immune modulatory response.
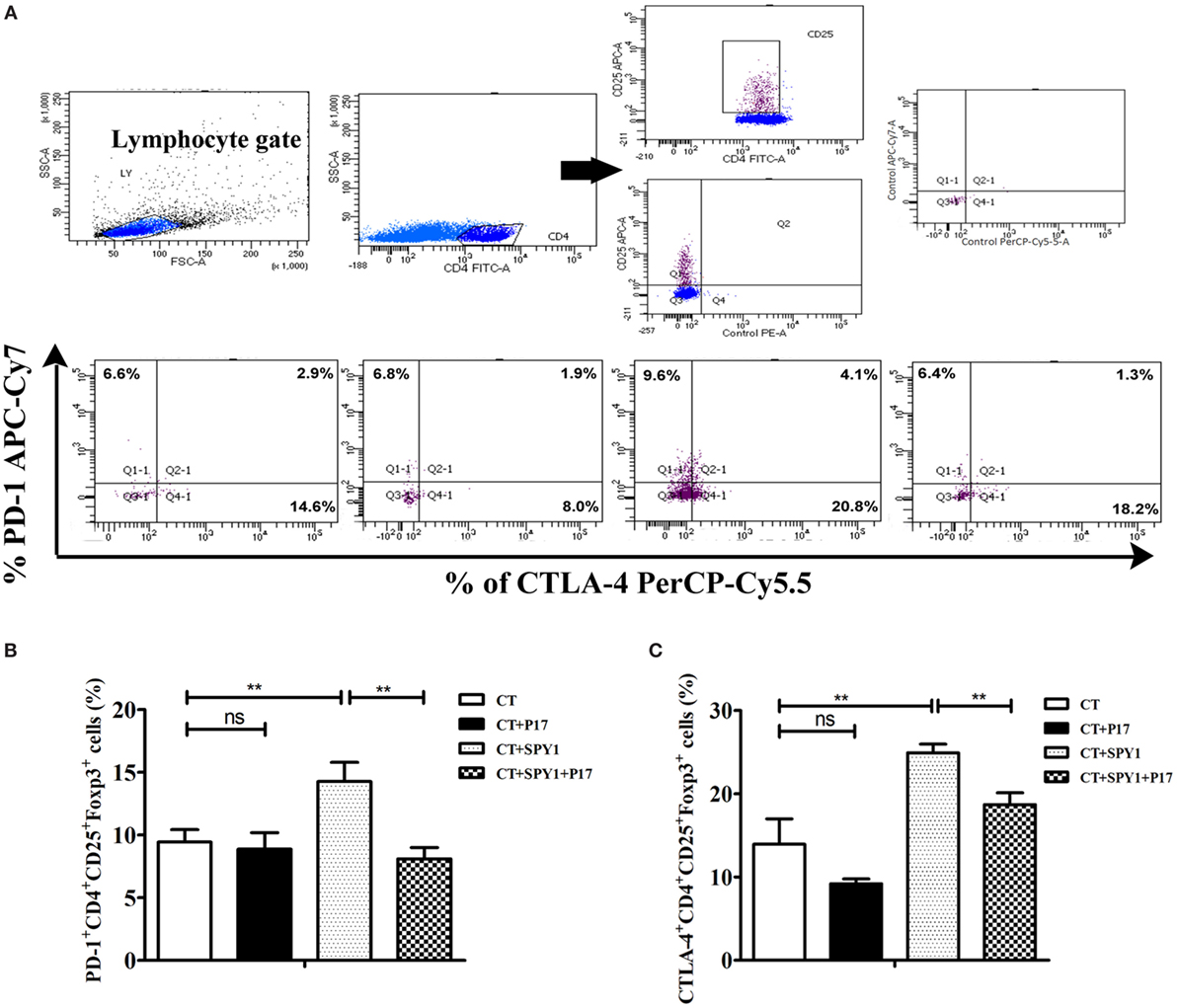
Figure 7. SPY1 vaccination stimulates elevating expression of PD-1 and CTLA-4 on regulatory T cells (Tregs) in immune mice. (A) Mice were intranasal challenged with pneumococcal strain 19F on day 7 after the last immunization, and mice lungs were removed on day 3 post-infection. The relative expression of PD-1 and CTLA-4 on CD4+CD25+Foxp3+ cells were analyzed by flow cytometry with antibodies including anti-mouse CD279(PD-1)-APC/Cy7, anti-mouse CD152(CTLA-4)-PerCP/Cy5.5, anti-mouse CD4-FITC, anti-mouse CD25-APC, and anti-mouse Foxp3-PE, respectively. The procedure was as describe in Section “Materials and Methods.” Percentages of PD-1+CD4+CD25+Foxp3+ cells (B) and CTLA-4+CD4+CD25+Foxp3+ cells (C) in mice lung of triplicate samples were calculated. Data were shown as the mean ± SD. **p < 0.01.
Discussion
Investigations of the mechanism underlying immune protection are crucial for novel vaccine development. We have previously detected protective roles of the acquired Treg response induced by the novel live-attenuated pneumococcal vaccine SPY1 during pneumococcal infection. In this study, we clarified the mechanism mediating the protective Treg response.
Pneumococcal conjugate vaccines reduce the risk of nasopharyngeal colonization by the serotypes included in the vaccine (3). However, their wide application is restricted by the limited serotypes, high cost, serotype replacement, etc. Compared with pneumococcal conjugate vaccines, pneumococcal whole-cell vaccines may be a better choice for developing countries, since they retained whole molecules which act as pathogen-associated molecular patterns recognized by host antigen-presenting cells and are presented in their natural configuration (9). Various procedures are used for vaccination, even for live-attenuated vaccines. In an investigation of the live-attenuated cholera vaccine VCUSM21P, administration twice at an interval of 14 days yielded acceptable protection (26). Other studies have found that live-attenuated hepatitis A vaccines H2 and LA-1 virus strains exert satisfactory protection with a single dose administered by subcutaneous injection (27). Based on our previous exploration of SPY1 vaccination, we observed a robust humoral and cellular immune response after intranasal vaccination with SPY1 four times, inducing robust immune responses (11, 28). In our previous work, the differences in immune responses and protective efficacies of SPY1 by intraperitoneal immunization and intranasal immunization were evaluated. Intraperitoneal immunization and intranasal immunization both induced elevations in IL-10, IL-4, and IL-17A; however, the levels of IL-17A in splenocyte supernatants and nasal washes as well as secretory IgA in saliva in intranasally immunized mice were significantly higher than those in intraperitoneally immunized mice, suggesting better protection against pneumococcal colonization in an intranasal immunization model (10). In addition, intranasal immunization provided increased protection against lethal pneumococcal challenge at 3 months post-vaccination, and the survival rates for the intranasal immunization model and intraperitoneal immunization model were 100 and 75%, respectively, and this difference can probably be explained by the more rapid clearance of the subcutaneously injected vaccine (29).
Vaccine adjuvants can be used to enhance immunogenicity, accelerate the immune response, increase the duration of protection, and so on (30). For live-attenuated vaccines, some studies have shown that the antigenicity and immunogenicity of vaccine strains, such as live-attenuated Yersinia pestis against pneumonic plague are strong enough so that the adjuvant is not necessary (31). However, other studies have shown the indispensable role of adjuvants for vaccination with live-attenuated vaccines, such as the cholera vaccine candidate VCUSM21P (26) and H5N1 live-attenuated influenza vaccines (32). In our previous research, we have evaluated the vaccination of SPY1 without adjuvant CT; however, the protective effect was not as efficient as that obtained by CT (data not published). We used adjuvant CT, as in our other studies of SPY1 (10, 11). However, the toxicity of CT limits its application in humans, making the development of safe and nontoxic adjuvants that induce protective immunity essential for the nasal immunization of SPY1 in humans. We have developed safe and effective mucosal adjuvants as CT alternatives, such as the mast cell activator compound 48/80 (C48/80) (33) and CaPi mineralized shell (34). Coupled with these nontoxic adjuvants, protection induced by SPY1 or its derived vaccine SPY1ΔlytA is as efficient as that obtained with CT, ensuring the future application of SPY1 in humans.
The balance between immune response activation essential for host defense and immune suppression restricting excessive host damage caused by the immune response should be strictly regulated during pathogen invasion. In this study, P17, which downregulates Tregs, influenced the systemic protective immune response elicited by SPY1 immunization. P17 treatment significantly upregulated levels of the infection-associated inflammatory cytokine TNF-α and immune cytokines (IL-12p70, IFN-γ, IL-4, IL-5, and IL-17A) and downregulated concentrations of the immunosuppressive cytokine IL-10 and inflammatory mediator IL-6 (23) secreted by splenocytes of SPY1-immunized mice. Correspondently, compared with SPY1-immunized mice not treated with P17, we observed more severe pulmonary injuries in P17-treated immunized mice during pneumococcal infection. Moreover, the changes in cytokines in immunized mouse lung homogenates were in line with those in splenocytes mentioned above. These data indicated that the SPY1-specific Treg immune response could inhibit uncontrolled pulmonary immune responses and infection-associated inflammation and thereby limit excessive immunopathology in immunized mice, resulting in acquired immune homeostasis, which is necessary for vaccination-induced protection against pneumococcal infection. P17 suppressed the increase in IFN-γ; however, the elevation of IFN-γ in immunized mice was not SPY1-specific (no significant differences in the IFN-γ increase were detected between the CT group and CT + SPY1 group) and were probably caused by CT, consistent with the results of our previous study (11) and other reports (35). As a crucial pro-inflammatory cytokine (36), IL-12p70 is elevated by SPY1-infected dendritic cells co-cultured with CD4+ cells (37), consistent with the results of this study. Several studies have suggested that IL-6 is a pro-inflammatory cytokine in infection (38); however, other studies have shown that IL-6-deficient mice exhibit impaired resistance against S. pneumoniae (23), Listeria monocytogenes (23, 39), and Escherichia coli (40), implying that IL-6 inhibits inflammation. Taken together, these findings suggest that IL-6 is a dual cytokine. As one of the immunosuppressive cytokines secreted by Tregs, the roles of IL-10 in infection are unclear. IL-10 impairs the immunosuppressive activity of Tregs in murine models of schistosomiasis japonica or asthma (41). However, during remission stage in mice with lymphocytic choriomeningitis virus infection, IL-10 produced by Tregs promotes the maturation of memory CD8+ T cells, which is beneficial for host defense against secondary infections by intracellular pathogens (42). Similarly, in this study, dramatically elevated expression of IL-10 and palliative pulmonary injuries was observed in SPY1-immunized mice, indicating the beneficial effect of IL-10 on immunoprotection of SPY1-specific Tregs.
There are three main types of CD4+ regulatory cells, i.e., Tr1, CD4+ Th3, and CD4+CD25+Foxp3+ T cells (43). Most studies have focused on CD4+CD25+Foxp3+ T cells, which are indispensable for the maintenance of the immunologic balance (44). SPY1 could induce the Treg immune response involved in protection against pneumococcal infection (11). In this study, we showed that the expression of Foxp3, a key transcription factor belonging to the Treg immune pathway, mediates the activation of SPY1-specific Tregs. Obviously upregulated Foxp3 attributed to SPY1 vaccination was detected, suggesting that CD4+CD25+Foxp3+ T cells are the major type of SPY1-specific Tregs.
Regulatory T cell differentiation is mainly regulated by TGF-β/Smad signaling (24). Smad2 and Smad3 contribute to Foxp3 induction via different mechanisms, Smad3 directly interacts with an enhancer region of Foxp3, i.e., CNS1 (45), and the interaction is believed to be necessary for sustaining normal Foxp3+ Treg numbers in the mouse gut but not in other organs (46). Smad2 has a relatively lower affinity to DNA than Smad3, and it cannot directly interact with CNS1 (47). However, the absence of Smad2 significantly decreases the upregulation of Foxp3 on T cells (48) and the deletion of both Smad2 and Smad3 could completely abolish the induction of Foxp3 by TGF-β (49), illustrating the synergetic effects of Smad2 and Smad3 in Treg induction. We observed increased levels of Smad2 and Smad3 as well as decreased levels of the negative regulatory factor Smad7 in the SPY1 vaccination group. These changes in Smads were significantly reversed by P17 treatment due to the inhibition of TGF-β1, illustrating that SPY1-stimulated TGF-β1 induced the generation of SPY1-specific Tregs via the Smad2/3 signaling pathway. Given that several Smad-independent pathways are also responsible for the induction of Tregs via TGF-β in different experiment models (18–21), the levels of p38 mapk, akt, mtor, and pi3k in pulmonary tissues were determined by real-time PCR, and no significant differences were detected among the control group, P17-treated SPY1-immunized group, and non-P17-treated immunized group (data not shown). Nevertheless, we cannot rule out the potential roles of other unknown signaling pathways in the generation of SPY1-specific Tregs for the immunoprotection in mice.
As foremost costimulatory inhibitors, PD-1 and CTLA-4 play key roles in the suppressive activity of Tregs (25, 50). We observed enhanced expression of PD-1 and CLTA-4 on SPY1-specific Tregs and their subsequent roles in immunoprotection elicited by SPY1-specific Tregs together with increased IL-10. TGF-β is believed to be another important inhibitory cytokine secreted by Tregs; however, its suppressive function remains contentious (47). Some research has revealed that TGF-β1 is redundant for Treg suppressor functions (51), but Ming et al. (52) showed that Treg-derived TGF-β1 is essential for controlling inflammatory-bowel disease. Therefore, the role of TGF-β1 in the immunoprotective function of SPY1-specific Tregs may be complicated.
Despite their crucial role in immune homeostasis and in the prevention of autoimmunity, the function of Tregs in infection is still controversial. Tregs are detrimental to hosts by restricting the effector T cell immune response during early tuberculosis (53). In some cases, virus or bacteria-specific Tregs not only prevent pathogen elimination but also promote a generalized state of immune suppression in vivo, making the host more susceptible to secondary infections with other pathogens (54). However, growing evidence has suggested that interactions between pathogens and Tregs are mutually beneficial to the pathogen and host, which allows persistent infection, conferring the maintenance of long-term memory and resistance to reinfection in a model of Leishmania major infection (55). Furthermore, emerging evidence suggests that Tregs can be beneficial to the host by restricting an overly robust inflammatory response, which causes excessive collateral damage to self-tissues (56), and by promoting the reparation of damaged tissues (57). Accordingly, Tregs should be established to regulate immune responses by maintaining homeostasis for efficient vaccination (58), highlighting the importance of considering vaccine-induced protective Tregs in the design of vaccine candidates.
In addition to Tregs and the regulatory cytokines IL-10 and IL-6, other cells including myeloid-derived suppressor cells, regulatory B cells (Bregs), regulatory γδ T cells, and immunosuppressive plasmocytes, as well as the cytokine IL-35 have immunosuppressive functions. Bregs have strong immunosuppressive effects and can negatively regulate immune responses in malignant tumors (59), infections (60), and autoimmune diseases (61) by multiple mechanisms. IL-35 is a vital anti-inflammatory cytokine that not only induces Bregs but also serves as an important effector of Bregs, participating in the regulation of the immune response via a positive feedback network. The immunosuppressive effects of Bregs and IL-35 have been found in innate immunity model against infection but not in an acquired immunity model with vaccination, and we cannot exclude the potential roles of other immunosuppressive cells and cytokines in the immune protective effect of SPY1.
As identified using a phage-displayed peptide library, peptide P17 is an effective inhibitor of Tregs by inhibiting TGF-β1, and its effect and specificity on Tregs have been well documented in various models, including in an invasive pneumococcal infection model (17, 62). P17 inhibits TGF-β1, TGF-β2, and TGF-β3 activity according to a previous study (17). Being predominantly expressed in the immune system, TGF-β1 is believed to be a crucial pleiotropic cytokine with potent immunoregulatory properties (24). The binding of P17 to TGF-β1 is stronger than binding to other isoforms of TGF-β (17). Therefore, in this study, we focused on TGF-β1. In follow-up work, the protective effect of SPY1-specific Tregs may be further established by evaluating a co-culture of TGF-β1 with mice splenocytes in vitro or by supplying mice with recombinant TGF-β1 in vivo. In addition, depletion of regulatory T cell mice (63) can be used to further evaluate the protection and related mechanisms of SPY1-induced acquired Treg immune responses in future studies.
To conclude, we characterized the mechanism underlying the protective role of vaccine-specific Treg immune response in response to the novel live-attenuated pneumococcal vaccine SPY1 in a mouse model. Immunization with SPY1 stimulated the activation of TGF-β1 via the Smad2/3 signaling pathway, which led to the production of Foxp3+ Tregs and the subsequent upregulation of the costimulatory inhibitors PD-1 and CTLA-4. The activated SPY1-specific Treg immune response maintained the beneficial immune balance among the infection-associated inflammatory cytokine TNF-α, immune cytokines IL-6, IL-12p70, IL-4, IL-5, and IL-17A, as well as the immunoregulatory cytokine IL-10, and further alleviated excessive pulmonary injury, resulting in decreased bacterial colonization, elevated survival rates, and prolonged survival. Our results indicated that a protective immune response is elicited by vaccine-specific Tregs via the TGF-β1-Smad2/3 pathway. These findings may contribute to the comprehensive assessment of live vaccines and other mucosal vaccine candidates.
Ethics Statement
The research was proved by The Ethics Committee of Chongqing Medical University. All the animal experiments were done in accordance with the guidelines of the Institutional Animal Care and Use Committee of Chongqing Medical University.
Author Contributions
HL, XP, and LG were in charge of the whole project and participated in manuscript drafting. YG, SY, XH, and LG contributed to lab work and data analyses. JF, LZ, HW, YY, and XX revised the paper critically. All the authors have reviewed the manuscript.
Conflict of Interest Statement
The authors declare that the research was conducted in the absence of any commercial or financial relationships that could be construed as a potential conflict of interest.
Acknowledgments
This work was supported by National Natural Science Foundation of China (31500749) and Chongqing Natural Science Foundation (cstc2015jcyjA10102).
Supplementary Material
The Supplementary Material for this article can be found online at https://www.frontiersin.org/articles/10.3389/fimmu.2018.01754/full#supplementary-material.
References
1. Liu L, Oza S, Hogan D, Perin J, Rudan I, Lawn JE, et al. Global, regional, and national causes of child mortality in 2000-13, with projections to inform post-2015 priorities: an updated systematic analysis. Lancet (2015) 385(9966):430–40. doi:10.1016/S0140-6736(14)61698-6
2. Mitchell AM, Mitchell TJ. Streptococcus pneumoniae: virulence factors and variation. Clin Microbiol Infect (2010) 16(5):411–8. doi:10.1111/j.1469-0691.2010.03183.x
3. Cilloniz C, Amaro R, Torres A. Pneumococcal vaccination. Curr Opin Infect Dis (2016) 29(2):187–96. doi:10.1097/QCO.0000000000000246
4. Giudice EL, Campbell JD. Needle-free vaccine delivery. Adv Drug Deliv Rev (2006) 58(1):68–89. doi:10.1016/j.addr.2005.12.003
5. Shakya AK, Chowdhury MYE, Tao W, Gill HS. Mucosal vaccine delivery: current state and a pediatric perspective. J Control Release (2016) 240:394–413. doi:10.1016/j.jconrel.2016.02.014
6. Flasche S, Van Hoek AJ, Sheasby E, Waight P, Andrews N, Sheppard C, et al. Effect of pneumococcal conjugate vaccination on serotype-specific carriage and invasive disease in England: a cross-sectional study. PLoS Med (2011) 8(4):e1001017. doi:10.1371/journal.pmed.1001017
7. Balsells E, Guillot L, Nair H, Kyaw MH. Serotype distribution of Streptococcus pneumoniae causing invasive disease in children in the post-PCV era: a systematic review and meta-analysis. PLoS One (2017) 12(5):e0177113. doi:10.1371/journal.pone.0177113
8. Rosch JW, Iverson AR, Humann J, Mann B, Gao G, Vogel P, et al. A live-attenuated pneumococcal vaccine elicits CD4+ T-cell dependent class switching and provides serotype independent protection against acute otitis media. EMBO Mol Med (2014) 6(1):141–54. doi:10.1002/emmm.201202150
9. Pichichero ME. Pneumococcal whole-cell and protein-based vaccines: changing the paradigm. Expert Rev Vaccines (2017) 16(12):1181–90. doi:10.1080/14760584.2017.1393335
10. Wu K, Yao R, Wang H, Pang D, Liu Y, Xu H, et al. Mucosal and systemic immunization with a novel attenuated pneumococcal vaccine candidate confer serotype independent protection against Streptococcus pneumoniae in mice. Vaccine (2014) 32(33):4179–88. doi:10.1016/j.vaccine.2014.05.019
11. Xu X, Wang H, Liu Y, Wang Y, Zeng L, Wu K, et al. Mucosal immunization with the live attenuated vaccine SPY1 induces humoral and Th2-Th17-regulatory T cell cellular immunity and protects against pneumococcal infection. Infect Immun (2015) 83(1):90–100. doi:10.1128/IAI.02334-14
12. Sanjabi S, Oh SA, Li MO. Regulation of the immune response by TGF-beta: from conception to autoimmunity and infection. Cold Spring Harb Perspect Biol (2017) 9(6):a022236. doi:10.1101/cshperspect.a022236
13. Chen W, Jin W, Hardegen N, Lei KJ, Li L, Marinos N, et al. Conversion of peripheral CD4+CD25- naive T cells to CD4+CD25+ regulatory T cells by TGF-beta induction of transcription factor Foxp3. J Exp Med (2003) 198(12):1875–86. doi:10.1084/jem.20030152
14. Huber S, Schramm C, Lehr HA, Mann A, Schmitt S, Becker C, et al. Cutting edge: TGF-beta signaling is required for the in vivo expansion and immunosuppressive capacity of regulatory CD4+CD25+ T cells. J Immunol (2004) 173(11):6526–31. doi:10.4049/jimmunol.173.11.6526
15. Marie JC, Letterio JJ, Gavin M, Rudensky AY. TGF-beta1 maintains suppressor function and Foxp3 expression in CD4+CD25+ regulatory T cells. J Exp Med (2005) 201(7):1061–7. doi:10.1084/jem.20042276
16. Dotor J, Lopez-Vazquez AB, Lasarte JJ, Sarobe P, Garcia-Granero M, Riezu-Boj JI, et al. Identification of peptide inhibitors of transforming growth factor beta 1 using a phage-displayed peptide library. Cytokine (2007) 39(2):106–15. doi:10.1016/j.cyto.2007.06.004
17. Gil-Guerrero L, Dotor J, Huibregtse IL, Casares N, Lopez-Vazquez AB, Rudilla F, et al. In vitro and in vivo down-regulation of regulatory T cell activity with a peptide inhibitor of TGF-beta1. J Immunol (2008) 181(1):126–35. doi:10.4049/jimmunol.181.1.126
18. Zhao M, Zhang H, Liu X, Jiang Y, Ren L, Hu X. The effect of TGF-beta on Treg cells in adverse pregnancy outcome upon Toxoplasma gondii infection. Front Microbiol (2017) 8:901. doi:10.3389/fmicb.2017.00901
19. Huber S, Schrader J, Fritz G, Presser K, Schmitt S, Waisman A, et al. P38 MAP kinase signaling is required for the conversion of CD4+CD25- T cells into iTreg. PLoS One (2008) 3(10):e3302. doi:10.1371/journal.pone.0003302
20. Derynck R, Zhang YE. Smad-dependent and Smad-independent pathways in TGF-beta family signalling. Nature (2003) 425(6958):577–84. doi:10.1038/nature02006
21. Haxhinasto S, Mathis D, Benoist C. The AKT-mTOR axis regulates de novo differentiation of CD4+Foxp3+ cells. J Exp Med (2008) 205(3):565–74. doi:10.1084/jem.20071477
22. Blanquiceth Y, Rodriguez-Perea AL, Tabares Guevara JH, Correa LA, Sanchez MD, Ramirez-Pineda JR, et al. Increase of frequency and modulation of phenotype of regulatory T cells by atorvastatin is associated with decreased lung inflammatory cell infiltration in a murine model of acute allergic asthma. Front Immunol (2016) 7:620. doi:10.3389/fimmu.2016.00620
23. van der Poll T, Keogh CV, Guirao X, Buurman WA, Kopf M, Lowry SF. Interleukin-6 gene-deficient mice show impaired defense against pneumococcal pneumonia. J Infect Dis (1997) 176(2):439–44. doi:10.1086/514062
24. Yoshimura A, Wakabayashi Y, Mori T. Cellular and molecular basis for the regulation of inflammation by TGF-beta. J Biochem (2010) 147(6):781–92. doi:10.1093/jb/mvq043
25. Kawai K, Uchiyama M, Hester J, Wood K, Issa F. Regulatory T cells for tolerance. Hum Immunol (2017) 79(5):294–303. doi:10.1016/j.humimm.2017.12.013
26. Murugaiah C, Nik Mohd Noor NZ, Mustafa S, Manickam R, Pattabhiraman L. Construction and evaluation of V. cholerae O139 mutant, VCUSM21P, as a safe live attenuated cholera vaccine. PLoS One (2014) 9(2):e81817. doi:10.1371/journal.pone.0081817
27. Xu Z-Y, Wang X-Y. Live attenuated hepatitis A vaccines developed in China. Hum Vaccin Immunother (2013) 10(3):659–66. doi:10.4161/hv.27124
28. Qiu Y, Zhang X, Wang H, Zhang X, Mo Y, Sun X, et al. Heterologous prime-boost immunization with live SPY1 and DnaJ protein of Streptococcus pneumoniae induces strong Th1 and Th17 cellular immune responses in mice. J Microbiol (2017) 55(10):823–9. doi:10.1007/s12275-017-7262-1
29. Tarahomjoo S. Recent approaches in vaccine development against Streptococcus pneumoniae. J Mol Microbiol Biotechnol (2014) 24(4):215–27. doi:10.1159/000365052
30. Mohan T, Verma P, Rao DN. Novel adjuvants & delivery vehicles for vaccines development: a road ahead. Indian J Med Res (2013) 138(5):779–95.
31. Zhang X, Qi Z, Du Z, Bi Y, Zhang Q, Tan Y, et al. A live attenuated strain of Yersinia pestis DeltayscB provides protection against bubonic and pneumonic plagues in mouse model. Vaccine (2013) 31(22):2539–42. doi:10.1016/j.vaccine.2013.03.054
32. Zheng D, Yi Y, Chen Z. Development of live-attenuated influenza vaccines against outbreaks of H5N1 influenza. Viruses (2012) 4(12):3589–605. doi:10.3390/v4123589
33. Zeng L, Liu Y, Wang H, Liao P, Song Z, Gao S, et al. Compound 48/80 acts as a potent mucosal adjuvant for vaccination against Streptococcus pneumoniae infection in young mice. Vaccine (2015) 33(8):1008–16. doi:10.1016/j.vaccine.2015.01.013
34. Zhang X, Cui J, Wu Y, Wang H, Wang J, Qiu Y, et al. Streptococcus pneumoniae attenuated strain SPY1 with an artificial mineral shell induces humoral and Th17 cellular immunity and protects mice against pneumococcal infection. Front Immunol (2017) 8:1983. doi:10.3389/fimmu.2017.01983
35. Meza-Sanchez D, Perez-Montesinos G, Sanchez-Garcia J, Moreno J, Bonifaz LC. Intradermal immunization in the ear with cholera toxin and its non-toxic beta subunit promotes efficient Th1 and Th17 differentiation dependent on migrating DCs. Eur J Immunol (2011) 41(10):2894–904. doi:10.1002/eji.201040997
36. Thompson A, Orr SJ. Emerging IL-12 family cytokines in the fight against fungal infections. Cytokine (2018). doi:10.1016/j.cyto.2018.05.019
37. Gao S, Zeng L, Zhang X, Wu Y, Cui J, Song Z, et al. Attenuated Streptococcus pneumoniae vaccine candidate SPY1 promotes dendritic cell activation and drives a Th1/Th17 response. Immunol Lett (2016) 179:47–55. doi:10.1016/j.imlet.2016.08.008
38. Ataie-Kachoie P, Pourgholami MH, Richardson DR, Morris DL. Gene of the month: interleukin 6 (IL-6). J Clin Pathol (2014) 67(11):932–7. doi:10.1136/jclinpath-2014-202493
39. Kopf M, Baumann H, Freer G, Freudenberg M, Lamers M, Kishimoto T, et al. Impaired immune and acute-phase responses in interleukin-6-deficient mice. Nature (1994) 368(6469):339–42. doi:10.1038/368339a0
40. Dalrymple SA, Slattery R, Aud DM, Krishna M, Lucian LA, Murray R. Interleukin-6 is required for a protective immune response to systemic Escherichia coli infection. Infect Immun (1996) 64(8):3231–5.
41. He L, Zhou S, Qi Q, Chi Y, Zhu J, Xu Z, et al. The regulation of regulation: interleukin-10 increases CD4(+) CD25(+) regulatory T cells but impairs their immunosuppressive activity in murine models with schistosomiasis japonica or asthma. Immunology (2018) 153(1):84–96. doi:10.1111/imm.12813
42. Laidlaw BJ, Cui W, Amezquita RA, Gray SM, Guan T, Lu Y, et al. Production of IL-10 by CD4(+) regulatory T cells during the resolution of infection promotes the maturation of memory CD8(+) T cells. Nat Immunol (2015) 16(8):871–9. doi:10.1038/ni.3224
43. Zhao H, Liao X, Kang Y. Tregs: where we are and what comes next? Front Immunol (2017) 8:1578. doi:10.3389/fimmu.2017.01578
44. Sakaguchi S, Powrie F. Emerging challenges in regulatory T cell function and biology. Science (2007) 317(5838):627–9. doi:10.1126/science.1142331
45. Zheng Y, Josefowicz S, Chaudhry A, Peng XP, Forbush K, Rudensky AY. Role of conserved non-coding DNA elements in the Foxp3 gene in regulatory T-cell fate. Nature (2010) 463(7282):808–12. doi:10.1038/nature08750
46. Schlenner SM, Weigmann B, Ruan Q, Chen Y, von Boehmer H. Smad3 binding to the foxp3 enhancer is dispensable for the development of regulatory T cells with the exception of the gut. J Exp Med (2012) 209(9):1529–35. doi:10.1084/jem.20112646
47. Kelly A, Houston SA, Sherwood E, Casulli J, Travis MA. Regulation of innate and adaptive immunity by TGFbeta. Adv Immunol (2017) 134:137–233. doi:10.1016/bs.ai.2017.01.001
48. Martinez GJ, Zhang Z, Reynolds JM, Tanaka S, Chung Y, Liu T, et al. Smad2 positively regulates the generation of Th17 cells. J Biol Chem (2010) 285(38):29039–43. doi:10.1074/jbc.C110.155820
49. Takimoto T, Wakabayashi Y, Sekiya T, Inoue N, Morita R, Ichiyama K, et al. Smad2 and Smad3 are redundantly essential for the TGF-beta-mediated regulation of regulatory T plasticity and Th1 development. J Immunol (2010) 185(2):842–55. doi:10.4049/jimmunol.0904100
50. Jain N, Nguyen H, Chambers C, Kang J. Dual function of CTLA-4 in regulatory T cells and conventional T cells to prevent multiorgan autoimmunity. Proc Natl Acad Sci U S A (2010) 107(4):1524–8. doi:10.1073/pnas.0910341107
51. Fahlen L, Read S, Gorelik L, Hurst SD, Coffman RL, Flavell RA, et al. T cells that cannot respond to TGF-beta escape control by CD4(+)CD25(+) regulatory T cells. J Exp Med (2005) 201(5):737–46. doi:10.1084/jem.20040685
52. Li MO, Wan YY, Flavell RA. T cell-produced transforming growth factor-beta1 controls T cell tolerance and regulates Th1- and Th17-cell differentiation. Immunity (2007) 26(5):579–91. doi:10.1016/j.immuni.2007.03.014
53. Shafiani S, Tucker-Heard G, Kariyone A, Takatsu K, Urdahl KB. Pathogen-specific regulatory T cells delay the arrival of effector T cells in the lung during early tuberculosis. J Exp Med (2010) 207(7):1409–20. doi:10.1084/jem.20091885
54. Roquilly A, McWilliam HEG, Jacqueline C, Tian Z, Cinotti R, Rimbert M, et al. Local modulation of antigen-presenting cell development after resolution of pneumonia induces long-term susceptibility to secondary infections. Immunity (2017) 47(1):135–47.e5. doi:10.1016/j.immuni.2017.06.021
55. Belkaid Y, Piccirillo CA, Mendez S, Shevach EM, Sacks DL. CD4+CD25+ regulatory T cells control Leishmania major persistence and immunity. Nature (2002) 420(6915):502–7. doi:10.1038/nature01152
56. Fulton RB, Meyerholz DK, Varga SM. Foxp3+ CD4 regulatory T cells limit pulmonary immunopathology by modulating the CD8 T cell response during respiratory syncytial virus infection. J Immunol (2010) 185(4):2382–92. doi:10.4049/jimmunol.1000423
57. Arpaia N, Green JA, Moltedo B, Arvey A, Hemmers S, Yuan S, et al. A distinct function of regulatory T cells in tissue protection. Cell (2015) 162(5):1078–89. doi:10.1016/j.cell.2015.08.021
58. Brezar V, Godot V, Cheng L, Su L, Levy Y, Seddiki N. T-regulatory cells and vaccination “pay attention and do not neglect them”: lessons from HIV and cancer vaccine trials. Vaccines (Basel) (2016) 4(3):30. doi:10.3390/vaccines4030030
59. Shen M, Sun Q, Wang J, Pan W, Ren X. Positive and negative functions of B lymphocytes in tumors. Oncotarget (2016) 7(34):55828–39. doi:10.18632/oncotarget.10094
60. Fillatreau S. Regulatory roles of B cells in infectious diseases. Clin Exp Rheumatol (2016) 34(4 Suppl 98):1–5.
61. Ray A, Dittel BN. Mechanisms of regulatory B cell function in autoimmune and inflammatory diseases beyond IL-10. J Clin Med (2017) 6(1):E12. doi:10.3390/jcm6010012
62. Neill DR, Fernandes VE, Wisby L, Haynes AR, Ferreira DM, Laher A, et al. T regulatory cells control susceptibility to invasive pneumococcal pneumonia in mice. PLoS Pathog (2012) 8(4):e1002660. doi:10.1371/journal.ppat.1002660
Keywords: Streptococcus pneumoniae, vaccine, protective mechanism, transforming growth factor β1, regulatory T cells
Citation: Liao H, Peng X, Gan L, Feng J, Gao Y, Yang S, Hu X, Zhang L, Yin Y, Wang H and Xu X (2018) Protective Regulatory T Cell Immune Response Induced by Intranasal Immunization With the Live-Attenuated Pneumococcal Vaccine SPY1 via the Transforming Growth Factor-β1-Smad2/3 Pathway. Front. Immunol. 9:1754. doi: 10.3389/fimmu.2018.01754
Received: 28 April 2018; Accepted: 16 July 2018;
Published: 02 August 2018
Edited by:
Junkal Garmendia, Consejo Superior de Investigaciones Científicas (CSIC), SpainReviewed by:
Carlos Martin, Universidad de Zaragoza, SpainLuciana Leite, Instituto Butantan, Brazil
Fikri Avci, University of Georgia, United States
Copyright: © 2018 Liao, Peng, Gan, Feng, Gao, Yang, Hu, Zhang, Yin, Wang and Xu. This is an open-access article distributed under the terms of the Creative Commons Attribution License (CC BY). The use, distribution or reproduction in other forums is permitted, provided the original author(s) and the copyright owner(s) are credited and that the original publication in this journal is cited, in accordance with accepted academic practice. No use, distribution or reproduction is permitted which does not comply with these terms.
*Correspondence: Xiuyu Xu, eHV4aXV5dTg1QDEyNi5jb20=
†These authors have contributed equally to this work.