- 1Finnish Red Cross Blood Service, Helsinki, Finland
- 2Molecular and Integrative Biosciences Research Programme, Faculty of Biological and Environmental Sciences, University of Helsinki, Helsinki, Finland
- 3Minerva Foundation Institute for Medical Research, Biomedicum, Helsinki, Finland
- 4Institute of Clinical Medicine, Division of Surgery, University of Oulu, Oulu, Finland
- 5Department of Anatomy and Cell Biology, Institute of Biomedicine, University of Oulu, Oulu, Finland
- 6Clinical Research Center, Department of Surgery and Intensive Care, Oulu University Hospital, Oulu, Finland
Resolution-phase macrophage population orchestrates active dampening of the inflammation by secreting anti-inflammatory and proresolving products including interleukin (IL)-10 and lipid mediators (LMs). We investigated the effects of both human bone marrow-derived mesenchymal stromal cells (MSCs) and MSC-derived extracellular vesicles (MSC-EVs) on mature human regulatory macrophages (Mregs). The cytokines and LMs were determined from cell culture media of Mregs cultivated with MSCs and MSC-EVs. In addition, the alterations in the expression of cell surface markers and the phagocytic ability of Mregs were investigated. Our novel findings indicate that both MSC coculture and MSC-EVs downregulated the production of IL-23 and IL-22 enhancing the anti-inflammatory phenotype of Mregs and amplifying proresolving properties. The levels of prostaglandin E2 (PGE2) were substantially upregulated in MSC coculture media, which may endorse proresolving LM class switching. In addition, our results manifest, for the first time, that MSC-EVs mediate the Mreg phenotype change via PGE2. These data suggest that both human MSC and MSC-EVs may potentiate tolerance-promoting proresolving phenotype of human Mregs.
Introduction
Inflammation is a crucial component of host tissue response, and controlling its initiation, progress, resolution, and post-resolution phases is essential for recovering tissue homeostasis. The overlapping stages of the cascade are moderated by macrophages, which are highly versatile and dynamic cells responding to various microenvironmental stimuli (1, 2). Macrophages exist as a heterogeneous population and display a spectrum of phenotypes both in vivo and in vitro depending on the provided signals. Conventional terms for two paradigmatic populations include classically activated “host defense” M1 and alternatively activated “wound-healing” M2. Additional concepts of “regulatory macrophages” or “Mregs” have emerged within the last decade (3–6).
At the resolution phase, the macrophage population shifts toward a resolving phenotype (7). These immune regulatory macrophages (Mregs) are characterized by immunosuppressive properties, such as high production of interleukin (IL)-10 and transforming growth factor (TGF)-β, and a downregulated production of pro-inflammatory IL-12 (3, 8, 9). The induction of Mreg populations may follow both innate and adaptive immune responses and arise from various stimuli including glucocorticoids, immune complexes, prostaglandins (PGs), IL-10, and apoptotic cells, combined with a second stimulus, such as a toll-like receptor ligand (3, 9–12). In recent years, Hutchinson and coworkers have established an experimental method for the preparation of ex vivo-manipulated regulatory macrophages. These cells suppress mitogen-stimulated T-cell proliferation in vitro and have been used as a promising immunosuppressive agent in early-phase clinical trials in renal transplantations (6, 13).
In addition to anti-inflammatory cytokines, lipid mediators (LMs) play an important role in the resolution phase. The resolution is initiated with LM class switching, in which PGs act as a cue for the conversion of pro-inflammatory to proresolving LM production. PGE2 and PGD2 induce neutrophils to produce fewer pro-inflammatory 5-lipoxygenase (5-LOX)-derived LMs, such as leukotrienes, and increase the production of 15-LOX products, such as lipoxins (LXs), through cyclic adenosine monophosphate induction and regulation of the gene transcription of 15-LOX (14). Proresolving LMs, termed specialized proresolving mediators (SPMs), reduce inflammation by decreasing neutrophil recruitment and increasing macrophage-mediated phagocytosis and efferocytosis (15). Macrophages are known to produce SPMs such as LXs, resolvins (Rvs), protectins, and maresins (16).
Mesenchymal stromal cells (MSCs) are multipotent adult stem cells that have been widely used in experimental cell therapy due to their immunosuppressive and anti-inflammatory properties (17). Key players in MSC immunomodulation include the tryptophan-degrading enzyme indoleamine 2,3-dioxygenase, adenosine-producing CD73, and PGE2 (18–22). MSCs are able to polarize macrophages toward a more anti-inflammatory phenotype in a PGE2-mediated manner (23–25). MSCs may improve the phagocytosis of macrophages by transporting mitochondria to macrophages via tunneling nanotube-like structures (26). MSCs have also been reported to produce SPMs in a murine model (27), but the evidence on SPM biosynthesis in human MSCs is limited, and only the production of an important proresolving mediator LXA4 has been described (28). In addition to secreted soluble molecules, paracrine activity via extracellular vesicles (EVs) is an important function of MSCs. MSC-derived EVs (MSC-EVs) mediate the immunosuppressive effect of MSCs (29, 30) and may also elicit a similar therapeutic response as the cells themselves (31–33). Lo Sicco et al. recently reported that human MSC-EVs are able to trigger polarization from the M1 to M2 phenotype in a murine model both in vitro and in vivo (34).
Mregs are considered an important proresolving cell population during the later stages of the immune response. Despite this prominent role, the cooperation between Mregs and other well-known immunomodulatory agents, such as MSCs, is sparsely studied. The majority of previous research on the effects of MSCs has been executed in murine models or by observing M2-type switch using polarized monocytes. Especially, the effect of MSCs or MSC-EVs on the properties of mature Mregs has not been addressed before. In this study, we focused on interplay in resolution and investigated the effects of human MSC coculture and MSC-EVs on the human Mreg population. The levels of cytokines and LMs were analyzed from conditioned media. In addition, we evaluated phagocytic ability and the alterations of phenotype marker expression of the Mreg population. Our novel findings indicate that both MSC coculture and MSC-EVs enhance the anti-inflammatory phenotype of Mregs by downregulating the production of IL-23 and IL-22. We identified several LMs and pathway markers from human Mreg-, MSC-, and EV-conditioned media. The results manifest that MSC-EVs may also mediate the discovered changes in cytokine levels via PGE2, and thus promote the resolution of inflammation.
Materials and Methods
MSC Culture
Human bone marrow-derived MSCs from two donors (35, 36) at passage 4 were thawed, and 1,200 cells/cm2 were plated on 10 cm plates (Nunclon™ Delta Surface, Thermo Fisher Scientific) in 10 ml α-MEM (Gibco) supplemented with 10% fetal bovine serum (FBS) (Gibco), 20 mM HEPES (Gibco), 100 U/ml penicillin, and 100 µg/ml streptomycin (Gibco). The cells were incubated at 37°C, 5% CO2 for 5 days, and the medium was renewed after 24 h. The cells were washed with 5 ml warm endotoxin-free phosphate-buffered saline (PBS) with or without (w/o) Ca2+/Mg2+ (Gibco DPBS CTS™) and detached with 1.5 ml TrypLE™ Express (Gibco). The detachment process was stopped with 5 ml warm 10% FBS (Sigma-Aldrich) in RPMI Medium 1640, GlutaMAX™ Supplement (Gibco), and the cells were centrifuged at 500 g for 5 min. The pellet was suspended with 10% FBS in RPMI Medium 1640, GlutaMAX™ Supplement, and cells were counted with NucleoCounter® NC-100™ (ChemoMetec).
MSC-Derived Extracellular Vesicle Extraction
Human bone marrow-derived MSC from two donors (35, 36) at passage 4 were thawed, and 1,200 cells/cm2 were plated on two 15 cm plates in 30 ml α-MEM supplemented with 10% FBS, 20 mM HEPES, 100 U/ml penicillin, and 100 µg/ml streptomycin. The cells were incubated at 37°C, 5% CO2 for 6 days, and the medium was renewed after 24 h. The cells were detached with TrypLE™ Express, and 1,300 cells/cm2 were plated on two-chamber type of Corning® CellSTACK® cell culture chambers (Sigma-Aldrich) in 250 ml medium. The chamber cultivation continued at 37°C, 5% CO2 for 3 days, and the medium was renewed after 24 h. Before starvation, the cells were washed three times with 100 ml PBS and once with 75 ml α-MEM. During starvation, the cells were incubated in 200 ml serum-free starvation medium α-MEM at 37°C, 5% CO2 for 2 days. The media were collected and centrifuged at 2,000 g for 10 min to remove cell debris. The supernatant was ultracentrifuged with Optima™ MAX-XP Ultracentrifuge (Beckman Coulter) at 100,000 g 1.5 h +4°C with MLA-50 rotor (k-factor = 92, Beckman Coulter), and the pelleted EVs were combined. For the second EV collection, the cell starvation was continued in 200 ml α-MEM at 37°C, 5% CO2 for 2 days followed by replication of EV centrifugation steps.
Macrophage Polarization Assay
The schematic overview of macrophage polarization assay is presented in Figure S1 in Supplementary Material, and the detailed method is described in Supplementary Methods in Supplementary Material.
Briefly, human peripheral blood mononuclear cells (PBMC) were extracted from buffy coats using Ficoll-Pague™ Plus (GE Healthcare Life Sciences) density gradient centrifugation at day 0. Monocyte selection was performed by plating 2 × 106 PBMC/well in Nunclon™ Delta Surface 24-well plates (Thermo Fisher Scientific) in RPMI Medium 1640 (Gibco), incubating at 37°C, 5% CO2 for 2 h. The attached monocytes were incubated at 37°C, 5% CO2 for 6 days in the following Polarization Media: 5 ng/ml M-colony stimulating factor (CSF) (PromoCell), 10% FBS (Sigma-Aldrich) in RPMI Medium 1640, GlutaMAX™ Supplement for Mreg polarization; 50 ng/ml GM-CSF (PromoCell), 10% FBS in RPMI Medium 1640, GlutaMAX™ Supplement for M1 polarization; and 50 ng/ml M-CSF, 10% FBS in RPMI Medium 1640, GlutaMAX™ Supplement for M2 polarization.
At day 6, the media were replaced with the following Activation Media: 25 ng/ml interferon (IFN)-γ, 10 ng/ml lipopolysaccharide (LPS), 5 ng/ml M-CSF, 10% FBS in RPMI Medium 1640, GlutaMAX™ Supplement for Mreg polarization; 50 ng/ml IFN-γ, 10 ng/ml LPS, 50 ng/ml GM-CSF, 10% FBS in RPMI Medium 1640, GlutaMAX™ Supplement for M1 polarization; and 20 ng/ml IL-4, 50 ng/ml M-CSF, 10% FBS in RPMI Medium 1640, GlutaMAX™ Supplement for M2 polarization. The added media volume was 500 µl in treatment wells and 600 µl in control wells. The incubation was continued at 37°C, 5% CO2.
At day 7, 20,000 MSCs in 100 µl Mreg/M1/M2 Activation media were added into representative treatment wells. The final media volume was 600 μl/well, and the cells were incubated at 37°C, 5% CO2 for 3 days. Alternatively, in MSC-EV supplementation experiments, at day 7, isolated EVs from one two-chamber were suspended in Mreg Activation Media, and 50 µl was added into 30 representative treatment wells. The supplementation was repeated at day 9, and isolated EVs in 50 µl representative Mreg Activation Media were added into each well. The final media volume was 600 μl/well, and the cells were incubated at 37°C, 5% CO2 for 3 days.
At day 10, media samples were collected from each well and centrifuged at 300 g for 15 min at RT. The supernatants were snap frozen on dry ice and stored at −70°C. Cell samples for flow cytometry analysis were detached with 0.5 ml/well cold Macrophage Detachment Solution DFX (PromoCell).
Phagocytosis Assay
The phagocytic ability of Mregs was determined at day 10 of Macrophage assay using a Phagocytosis Assay Kit (IgG FITC) (Cayman Chemicals) according to the manufacturer’s instructions. Briefly, latex beads coated with FITC-labeled rabbit IgG were added in 1:300 dilution in 200 µl Polarization media II on Mregs and incubated at +37°C, 5% CO2 for 4 h in Cell-IQ® automated cell culture and analysis system (CM Technologies Oy). After incubation, the cells were washed with 1 ml warm PBS and detached with 0.5 ml/well cold Macrophage Detachment Solution DFX. The cells were suspended up to 15 ml PBS and centrifuged at 350 g for 10 min. To quench cell-bound external fluorescence, the pellets were suspended with 45 µl Phagocytosis Kit Assay Buffer (Cayman Chemicals) and 5 µl 10× Trypan Blue Quenching Solution (Cayman Chemicals) was added followed by 1–2 min incubation at RT. The cells were washed with 2 ml Phagocytosis Kit Assay Buffer, centrifuged at 350 g for 10 min, and suspended in 50 µl 0.3% bovine serum albumin, 2 mM EDTA in PBS, pH 7.2.
Macrophage Phenotyping Using Flow Cytometry Analysis
The antibody staining was performed with PE-CD80 (clone 2D10.4, mouse IgG1 k, eBioscience), PE-Cy7-CD86 [clone 2331 (FUN-1), mouse IgG1 k, BD Biosciences], BV421-CD163 (clone GHI/61, mouse IgG1 k, BD Biosciences), and APC-CD206 (clone 19.2, mouse IgG1 k, BD Biosciences) according to the manufacturers’ instructions. Respectively, conjugated isotype control antibodies were used as negative control for background staining. Macrophages were suspended in 50 µl staining buffer (0.3% bovine serum albumin, 2 mM EDTA in PBS, pH 7.2) and incubated with 2.5 µg of Human BD Fc Block™ (BD Biosciences) for 10 min at RT. The pre-mixed fluorescent antibody cocktail was added, and the cells were incubated on ice in darkness for 30 min. After staining, the cells were washed with 2 ml staining buffer, pelleted by centrifuging at 350 g for 10 min, and suspended in 100 µl staining buffer.
Cell data were acquired with BD FACSAria IIU (BD Biosciences) flow cytometer using FACSDiva™ version 8.0.1 software (BD Biosciences) and analyzed with FlowJo® version 10.0.7 software (FlowJo, LLC). Macrophages were gated based on forward (FSC) and side (SSC) scatter patterns. Doublets and aggregates were excluded using FSC area versus FSC height. The fluorescence positive cells were gated based on isotype controls and populations. The results are represented as median fluorescence intensity (FRI) and frequency of positive cells. The representative gating strategy is presented in Figure S2 in Supplementary Material.
Cytokine Measurements
The cell culture media from Macrophage polarization assay was analyzed for 18 cytokines using human Th1/Th2/Th9/Th17/Th22/Treg 18 Plex ProcartaPlex Immunoassay (eBioscience) according to the manufacturer’s instructions. The media samples were thawed on ice, and three technical replicates from each experiment were pooled together. Briefly, 50 µl of samples and standards was incubated with magnetic beads followed by washing steps and the detection antibody mixture. After addition of streptavidin-PE, the signal data were acquired on Luminex® 100 system (Luminex). The data were analyzed with ProcartaPlex Analyst 1.0 Software (Thermo Fisher Scientific). Measures within the cytokine-specific detection range were included in the analysis, except the tumor necrosis factor (TNF)-α analysis, in which, for certain M1 samples, the upper limit of the detection range was used.
Identification of LMs and Pathway Markers
The levels of LMs and pathway markers were determined using liquid chromatography–tandem mass spectrometry (LC–MS/MS). The media samples were analyzed for LMs thromboxane (Tx)B2, PGE2, PGD2, 15-deoxy-Δ12,14-PGJ2, leukotriene B4, LXA4, RvD1, RvD2, RvD3, maresin 1, 10S,17S-dihydroxydocosahexaenoic acid (diHDHA) (also known as protectin DX), and monohydroxy pathway markers 15-hydroxyeicosatetraenoic acid (HETE), 18-hydroxyeicosapentaenoic acid (HEPE), 17-HDHA, and 14S-HDHA.
The cell culture samples were thawed on ice, and three volumes of methanol containing 500 pg of internal standards d4-PGE2 and d5-RvD2 were added to the sample. Then, samples were incubated for 45 min at −20°C for protein precipitation. The samples were centrifuged at 700 g for 15 min, and the supernatant was filtered using Captiva ND Lipids filtration device (Agilent Technologies), which trapped phospholipids but allowed LMs to pass through. The eluate was then concentrated to 75 µl, and 5 µl was injected to the LC–MS/MS system. The LMs and pathway markers were analyzed by using 6490 Triple Quadrupole LC/MS equipment with Agilent Jet Stream and iFunnel Technology coupled with 1290 Infinity LC (Agilent Technologies). For chromatographic separation, a ZorBAX Eclipse Plus C18 RRHD analytical column (2.1 mm × 50 mm, 1.8 µm, Agilent Technologies) was used. The LC–MS/MS method employed multiple reaction monitoring (MRM) detection for each LM (Table S1 in Supplementary Material) with the LC phases and optimized source parameters described by Le Faouder et al. (37).
Samples were analyzed as triplicates. The criteria used to identify a peak were as follows: (1) retention time of the peak was matched with the standard, (2) a peak eluted at the correct retention time in both Quantifier and Qualifier MRM scans of a single mediator, and (3) all of the triplicate sample runs contained a peak. The data were analyzed with MassHunter Quantitative Analysis software version 6.00 (Agilent Technologies) using the Quantifier MRM for quantitative analysis (Q3 quant, Table 3). Concentrations were normalized against the respective internal standards (Table S1 in Supplementary Material), and the detection limit was 0.2 ng/ml.
Statistical Analyses
Statistical analyses were performed with GraphPad PRISM® version 7.02 (GraphPad Software). Due to a relatively low number of biological replicates and non-normal distribution of variables, non-parametric statistical methods were applied. The values of cell culture media cytokines were log10-transformed. The variation of cytokines and flow cytometry results between M1-, M2-, and Mreg-conditioned media groups was analyzed by the Kruskal–Wallis test, and pairwise analyses were executed using the Mann–Whitney U tests. When assessing the variation of the levels of cytokines and LMs in Mreg-conditioned media w/o MSC coculture or MSC-EVs, the Wilcoxon matched-pairs signed-rank test was used. The results are expressed as median with interquartile ranges. p-Values < 0.05 were considered significant.
Ethical Permits
BM MSC donors gave their voluntary, informed, and written consent before sample collection, and the study protocols were approved by the Ethical Committee of Northern Ostrobothnia Hospital District or Ethical Committee of Hospital District of Helsinki and Uusimaa, Finland. The utilization of anonymized PBMCs from blood donors in research is in accordance with the rules of the Finnish Supervisory Authority for Welfare and Health (Valvira).
Results
Characteristics of Mregs and Other Macrophage Subtypes
Phenotypes of Mreg, M1, and M2 were assessed by the flow cytometry analysis of T cell activation co-stimulatory molecules CD80 and CD86, scavenger receptor CD163, and mannose receptor CD206, and the results are presented in Table 1. The observed Mreg phenotype was CD80low/intermediate, CD86+, CD163low, and CD206low. Approximately 40% of Mregs were positive for CD80, 70% for CD86, 25% for CD163, and 14% for CD206 expression. All the median FRIs and the frequencies of positive cells significantly differed between the studied macrophage subtypes, except the median FRI of CD80 (Table 1). When analyzing the subtypes pairwise (Figure 1), we observed that the median FRI of CD80 was higher among M1 than among Mreg (p = 0.027). All macrophage subtypes were highly positive for CD86. Both the median FRI of CD86 (p = 0.032) and frequency of CD86 positive cells (p = 0.006) were higher among M1 than Mreg.
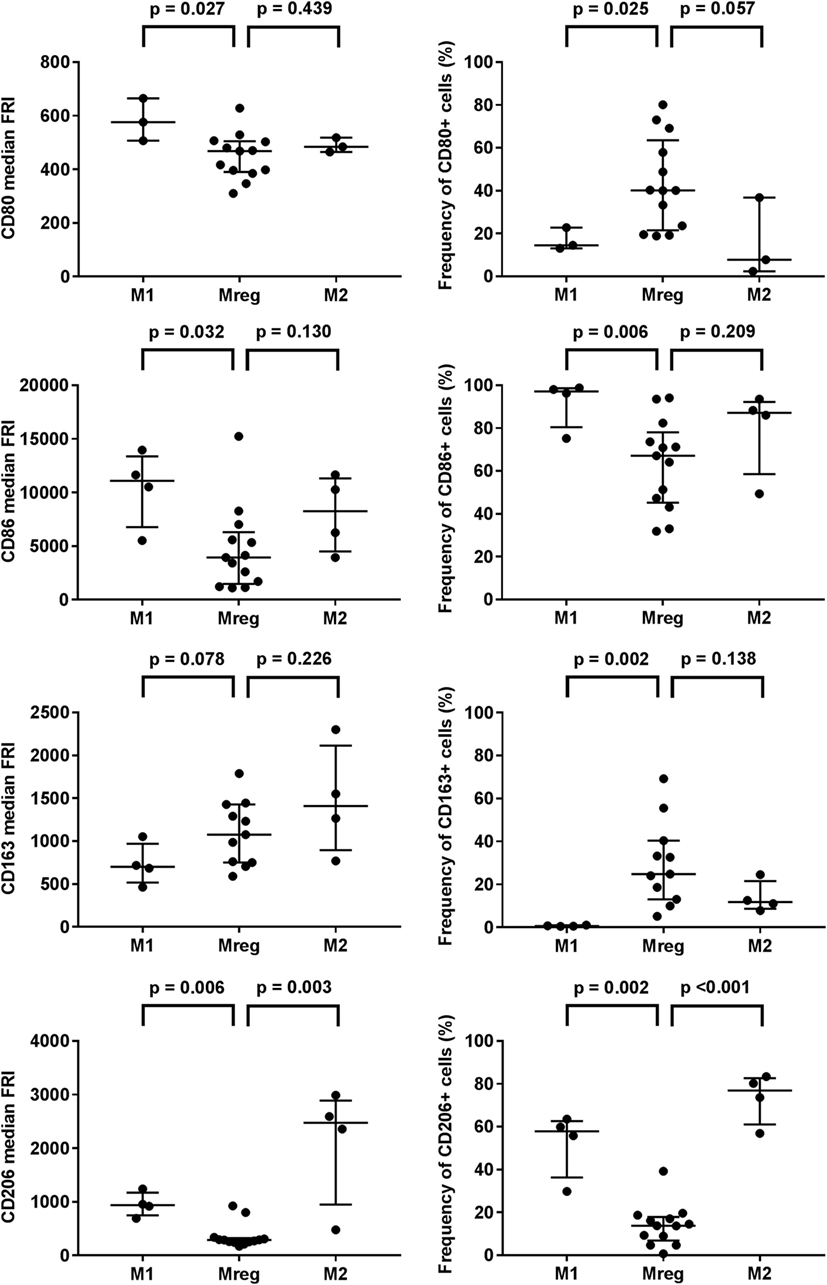
Figure 1. Phenotypes of M1, M2, and Mreg subtypes. The antibody staining was performed with PE-CD80, PE-Cy7-CD86, BV421-CD163, and APC-CD206 according to the manufacturers’ instructions. The median fluorescence intensities (left panel) and frequencies of positive cells (right panel) were determined with flow cytometry analysis. The significance in pairwise variation between M1 and Mreg, and M2 and Mreg was analyzed using the Mann–Whitney U test. The results are presented as median with interquartile range. The number of biological replicates varied from 3 to 13. Abbreviations: FRI, fluorescence intensity; M1, classically activated “host defense” macrophage; M2, alternatively activated “wound-healing” macrophage; Mreg, regulatory macrophage.
The observed phenotype differences were more prominent between Mreg and M1 than between Mreg and M2. The major exception was CD206; when analyzed pairwise, both median FRI (p = 0.003) and frequency of CD206+ cells (p < 0.001) were higher among M2 than Mreg.
The results of cytokines in Mreg-, M1-, and M2-conditioned media are presented in Figure 2. The levels of IL-1β, IL-2, IL-5, TNF-α, IL-10, IL-22, and IL-23 significantly varied between the macrophage-conditioned media groups. When compared pairwise, compared with M1-conditioned media, the levels of IL-1β (p = 0.003), IL-2 (p = 0.010), IL-5 (p = 0.002), TNF-α (p = 0.003), IL-22 (p = 0.005), and IL-23 (p = 0.003) in Mreg-conditioned media were significantly lower. By contrast, the level of IL-10 was higher in Mreg-conditioned media than in M1- and M2-conditioned media (p = 0.003 for both comparisons). In addition, the levels of IL-22 and IL-23 were higher in Mreg-conditioned media than in M2-conditioned media (p = 0.017 and p = 0.016, respectively).
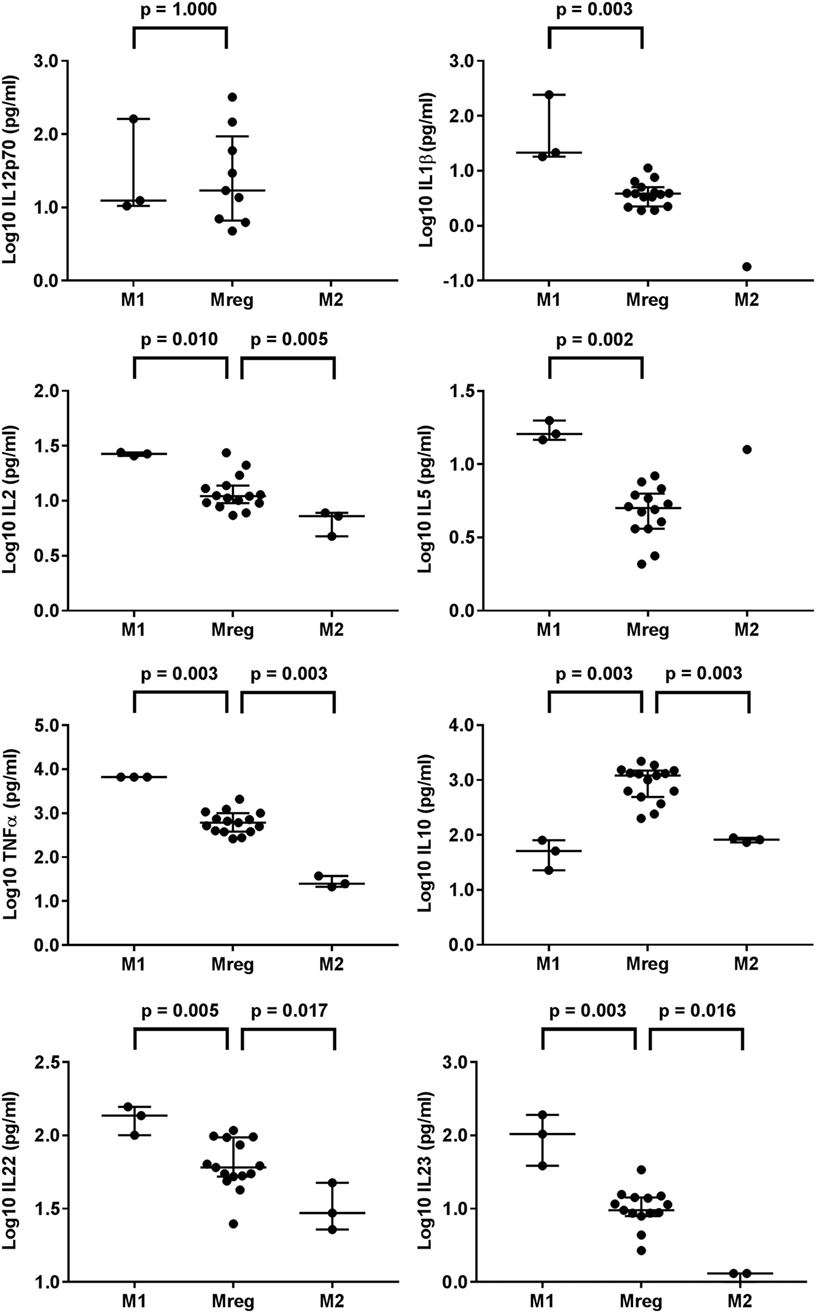
Figure 2. Level of cytokines in Mreg-, M1-, and M2-conditioned media. The media were analyzed for 18 cytokines, and measures within the cytokine-specific detection range were included in the analysis. The values of cell culture media cytokines were log10-transformed. The significance in pairwise variation between M1 and Mreg, and M2 and Mreg was analyzed using the Mann–Whitney U test, and the results are expressed as median with interquartile range. The number of biological replicates varies from 3 (for M1 and M2) to 15 for Mreg. Abbreviations: IL, interleukin; M1, classically activated “host defense” macrophage; M2, alternatively activated “wound-healing” macrophage; Mreg, regulatory macrophage.
Effect of MSC Coculture or MSC-EVs on Mreg Phenotype
The effects of MSC coculture or MSC-EVs on Mreg CD80, CD86, CD163, and CD206 phenotype were assessed by flow cytometry analysis and the representative raw data overlays are presented in Figures S3 and S5 in Supplementary Material, respectively. The pairwise analyses are depicted in Table 2 and Figures S4 and S6 in Supplementary Material. The MSC coculture did not affect the median FRI of CD80 in Mregs; however, the frequency of CD80+ Mregs was lower in the coculture than in Mreg culture alone (p = 0.024). We observed no significant change in CD86 expression and frequency among Mregs. The median FRI of scavenger receptor CD163 expression significantly decreased in Mregs when cocultured with MSC (p = 0.003). In addition, the frequency of CD163+ Mregs was lower in the coculture than in Mreg culture alone (p = 0.002). The same trend was observed in mannose receptor CD206 expression and the frequency of positive Mregs, even though only the reduction in frequency was significant (p = 0.060 for CD206 median FRI; p = 0.008 for frequency of CD206+ Mregs).
In concordance with the MSC coculture results, MSC-EVs decreased the median FRI of CD163 (p = 0.016), and compared with Mregs cultured without EVs, reduced the frequency of CD163+ Mregs (p = 0.016) (Table 2; Figure S6 in Supplementary Material). Again, the same trend was observed in CD206 expression and frequency of positive Mregs, even though only the reduction of median FRI of CD206 reached significance (p = 0.031 for CD206 median FRI; p = 0.078 for frequency of CD206+ Mregs).
Effect of MSC Coculture and MSC-EVs on the Cytokine Levels of Mreg-Conditioned Media
The cytokine levels were measured from Mreg-conditioned media without MSC coculture, and the results are presented in Figure 3. The levels of IL-10, IL-22, and IL-23 were significantly lower in Mreg-conditioned media with MSC coculture than without it (p = 0.003, p < 0.001, and p < 0.001, respectively). In addition, there was a positive trend toward decreased level of TNF-α after MSC coculture, even though the result did not reach significance (p = 0.064). This effect was more prominent among M1 subtype where the TNF-α production was more abundant (data not shown).
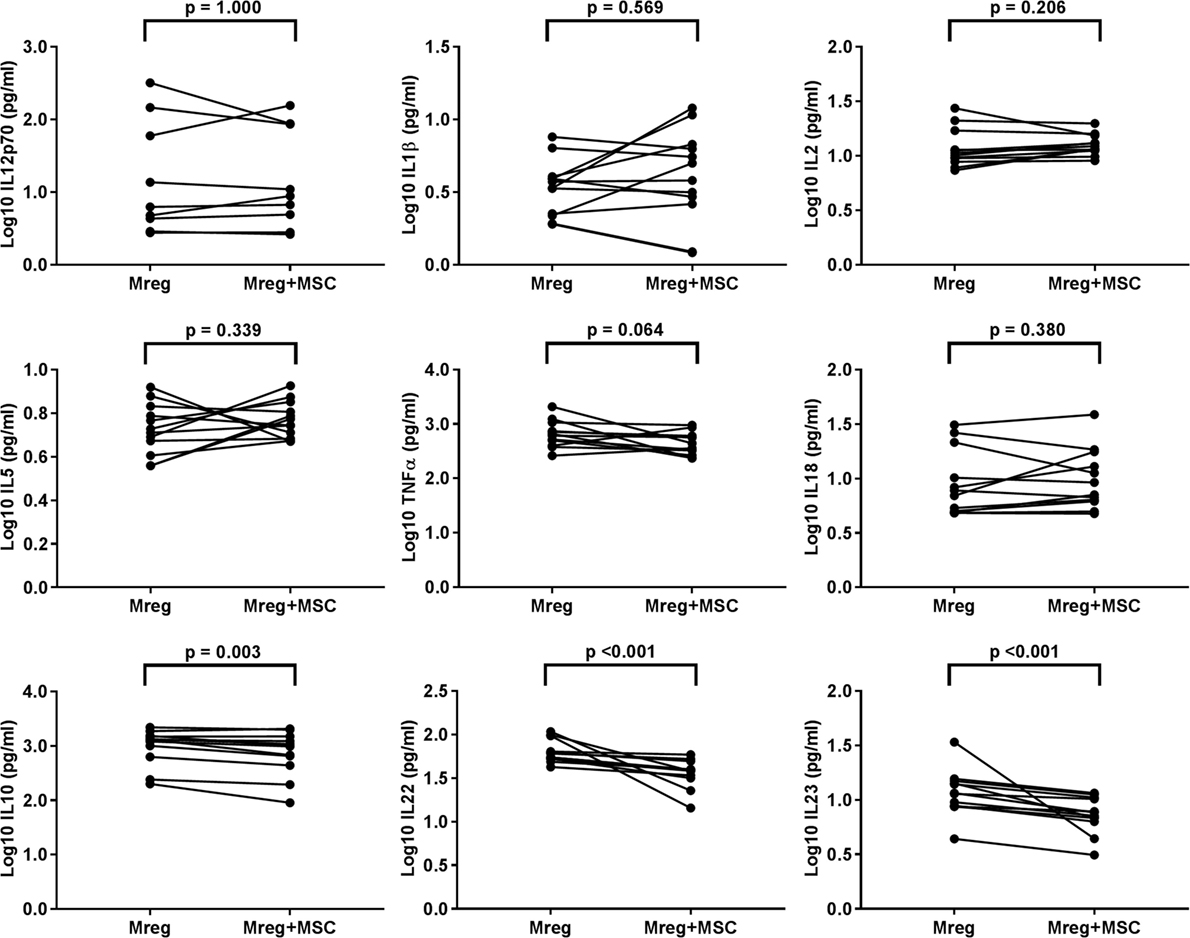
Figure 3. Effect of MSC coculture on the level of cytokines in Mreg-conditioned media. The media were analyzed for 18 cytokines and measures within the cytokine-specific detection range were included in the analysis. The values of cell culture media cytokines were log10-transformed. The variation of cytokines between Mreg-conditioned media with and without MSC coculture was analyzed by the Wilcoxon matched-pairs signed-rank test. The number of biological replicates varied from 9 to 12. Abbreviations: IL, interleukin; Mreg, regulatory macrophage; MSC, mesenchymal stromal cell.
The cytokine levels were measured from Mreg-conditioned media w/o MSC-EVs and the results are presented in Figure 4. The levels of IL-22 and IL-23 were significantly lower in Mreg-conditioned media with MSC coculture than without it (p = 0.008 and p = 0.031, respectively).
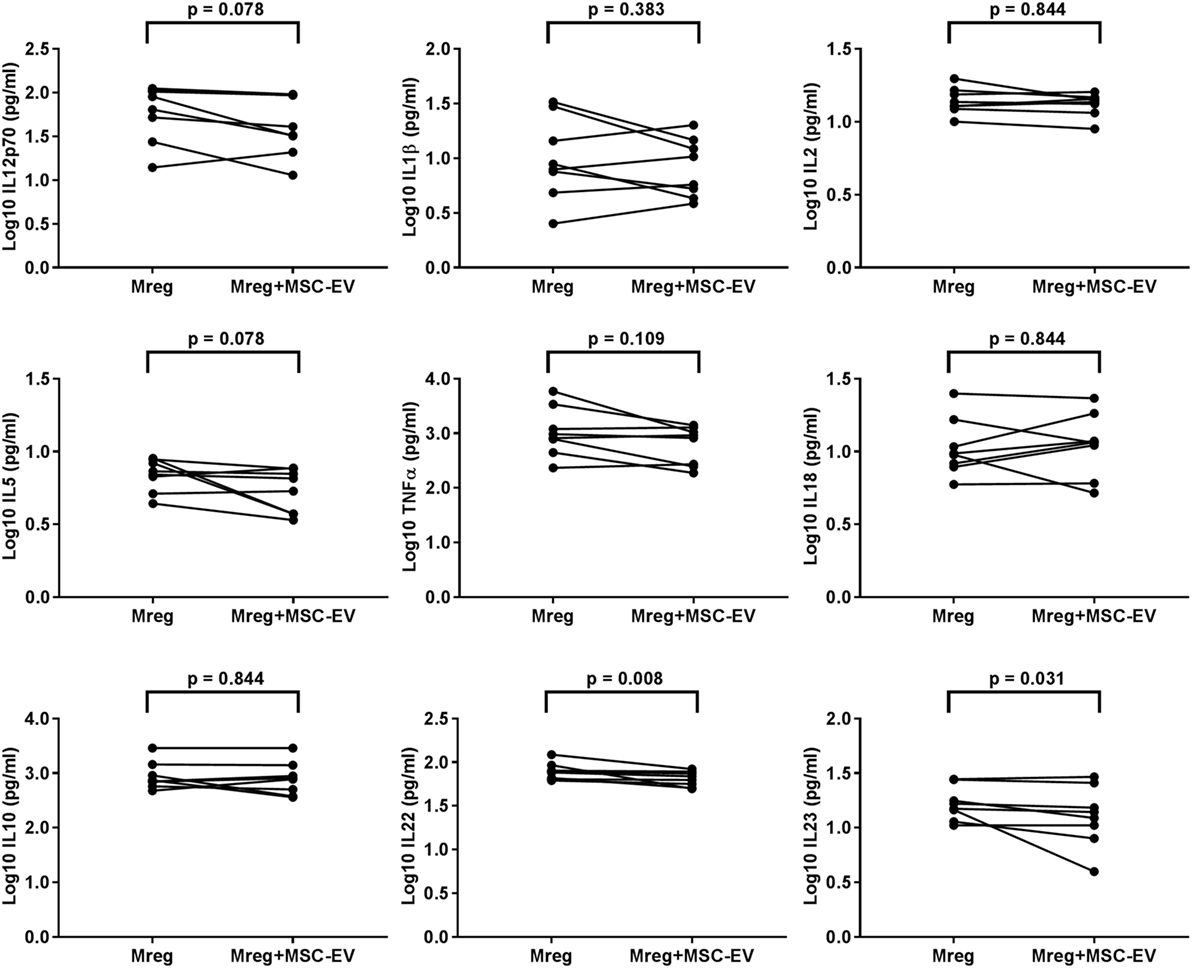
Figure 4. Effect of MSC-derived extracellular vesicles on the level of cytokines in Mreg-conditioned media. The media were analyzed for 18 cytokines, and measures within the cytokine-specific detection range were included in the analysis. The values of cell culture media cytokines were log10-transformed. The variation of cytokines between Mreg-conditioned media with and without MSC-EVs was analyzed by the Wilcoxon matched-pairs signed-rank test. The number of biological replicates is 8. Abbreviations: EV, extracellular vesicle; IL, interleukin; Mreg, regulatory macrophage; MSC, mesenchymal stromal cell; MSC-EVs, MSC-derived extracellular vesicles.
Effect of MSC Coculture and MSC-EVs on the LMs and Pathway Markers of Mreg-Conditioned Media
The levels of LMs and pathway markers were determined using LC–MS/MS, and identification of 7 out of the 15 metabolites addressed was successful. Table 3 displays the results for Mreg-conditioned media w/o MSC coculture or MSC-EVs. The levels of arachidonic acid-derived PGE2 (p = 0.002) and 15-HETE (p = 0.020), and docosahexaenoic acid-derived 17-HDHA (p = 0.009) were higher in Mreg-conditioned media with MSC coculture than in Mreg culture alone. When treated with MSC-EVs, compared with non-treated Mreg culture, the Mreg-conditioned media showed a significant increase in the level of PGE2 (p = 0.031).
TxB2, PGE2, 15-HETE, 18-HEPE, 17-HDHA, and 14S-HDHA were also detected both from MSC-conditioned media and MSC-EVs (Figure S7 in Supplementary Material).
Effect of MSC Coculture on the Phagocytic Ability of Mreg
The phagocytic ability of Mregs was assessed using latex beads coated with FITC-labeled rabbit IgG. It was noted that mannose receptor CD206 expression of Mregs was highly upregulated by the addition of the immune complex in all experimental conditions, and after 4-h incubation, the median frequencies of CD206+ Mregs were >90% (data not shown). However, there were neither significant differences in median FRI of FITC-IgG (p = 1.000) nor in frequency of FITC-IgG+ Mreg cells (p = 0.313) between Mregs culture alone and with MSC coculture (Figure 5).
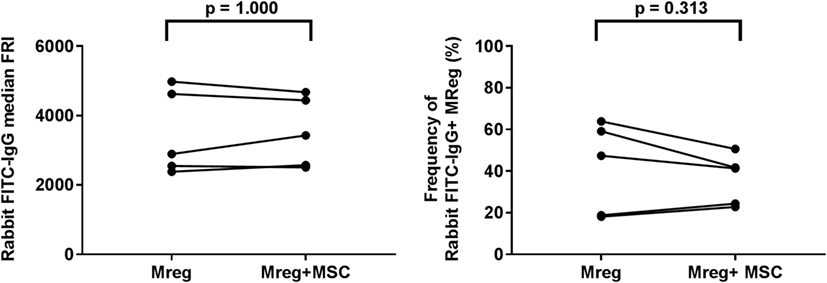
Figure 5. Effect of MSC coculture on the phagocytic ability of Mreg. The phagocytic ability of Mregs was assessed using latex beads coated with FITC-labeled rabbit IgG. The median FRI (left panel) and frequencies of positive cells (right panel) were determined with flow cytometry analysis. The variations between Mreg with and without MSC coculture were analyzed by the Wilcoxon matched-pairs signed-rank test. The number of biological replicates is 5. Abbreviations: FRI, fluorescence intensity; IgG, immunoglobulin G; Mreg, regulatory macrophage; MSC, mesenchymal stromal cell.
Discussion
Active dampening of the inflammatory response is a key phenomenon in the restoration of tissue homeostasis after infection or tissue damage. Resolution-phase macrophages orchestrate anti-inflammatory actions by secreting various factors, including IL-10 and SPMs (7). In this study, we investigated the effects of immunomodulative human bone marrow-derived MSCs and MSC-EVs on human Mregs. We observed that both MSC coculture and MSC-EVs enhanced the anti-inflammatory phenotype of Mreg by downregulating the production of IL-23 and IL-22. Several LMs and pathway markers were identified from Mreg-, MSC-, and EV-conditioned media. Interestingly, our results indicate that MSC-EVs may also mediate the discovered changes in cytokine levels via PGE2, and thus promote the resolution of inflammation.
Macrophage polarization and activation status strongly depend on the microenvironmental stimuli. Currently, the nomenclature system in the research field is heterogeneous and further confusion may arise from the fact that many murine markers fail to translate to human macrophages (4, 5). In the literature, “regulatory,” “Mreg,” or “immunosuppressive” macrophages comprise populations with a spectrum of characteristics evoked by different experimental conditions (6, 10). Common features include high IL-10 and TGF-β production. Mregs are also potent antigen-presenting cells and express co-stimulatory molecules needed for T and B cell activation (38). In our study, we characterized the phenotype of human Mregs and compared it with those of the conventionally generated M1 and M2 populations. In concordance with the previous Mreg studies, the Mregs generated in our experiments produced substantially higher levels of IL-10 than M1 and M2 populations (10, 11). Despite the activation by M1-stimulus-like combination of IFN-γ and LPS, the phenotype and cytokine profile of Mregs resembled more M2 than M1. The levels of pro-inflammatory cytokines, such as TNF-α and IL-23, were markedly lower in Mreg-conditioned media than in M1-conditioned media, but higher that in M2-conditioned media. As expected, the variance in biological replicates was rather high due to differences in PBMC donors. However, the observed Mreg phenotype CD80low/intermediate, CD86+, CD163low, and CD206low resembled previous reports for the shared markers (6, 13).
It has been reported that MSCs modify the differentiation of monocyte and macrophage populations toward anti-inflammatory or M2 phenotype, by, e.g., increasing IL-10 production, the expression of scavenger receptors, and phagocytic ability (23–26, 39, 40). However, the majority of the work has been done either with mouse models, cell lines, or in conditions where macrophages are not pre-polarized. Therefore, the previous observations are not directly applicable to our findings due to the different phenotype of Mregs. We are the first to show the effect of MSCs and MSC-EVs on fully differentiated human Mregs in the presence of activation medium (LPS and IFN-γ). MSCs have been shown to decrease TNF-α secretion from macrophages (39). In Mreg population, we observed a tendency for decreasing TNF-α. The reduction was much more prominent in the M1 population (data not shown) that produced this cytokine at a very high level. Furthermore, in contrast to the findings in M1/M2 axis, we detected that MSC coculture actually decreased the very high level of IL-10 in the conditioned media.
Both MSC coculture and MSC-EVs reduced the expression of CD163 and CD206 in Mregs, which differs from the previous M1/M2 axis results showing increased expression of M2 markers CD163 and CD206 (25, 39, 40). This observation is in accordance with the finding that MSCs did not enhance the FcγR-mediated IgG-binding phagocytosis of Mregs. Overall, phagocytosis is carried out by both opsonic (i.e., FcγR) and non-opsonic (i.e., CD206) receptors, and the phagocytosis ability, especially of Mregs, could be reasonably investigated using apoptotic neutrophils, which are highly abundant at the resolution phase (7). Altogether, the differences in the MSC-derived effects reflect the nature of diverse macrophage populations, the variation in species, and other experimental conditions. Most likely, the role of Mreg in vivo is not primarily conventional phagocytosis, but more related to efferocytosis and immune regulation.
Our novel results indicate that both MSC coculture and MSC-EVs are able to induce reduction in the levels of IL-23 and IL-22 and thereby enhance the anti-inflammatory characteristics of Mregs. IL-23 is a pro-inflammatory cytokine that induces and maintains the Th17 effector T cell population and pathogenicity, and many chronic immune-mediated disorders, such as psoriasis, arthritis, and Crohn’s disease, are strongly associated with IL-23 dysregulation (41–44). On the other hand, IL-22 is a major secretory product of Th17, and the function of IL-22 has been reported to mediate IL-23-induced inflammation and crosstalk between tissue barrier cells and the cells of the immune system (45). Thus, reduction of both IL-23 and IL-22 could decrease the induction of highly pathogenic Th17 cells and thus exacerbation of inflammation (46). Intriguingly, it has been recently reported that Th17 cells contribute to the resolution of inflammation by differentiating into T regulatory type 1 cells (47). We can therefore hypothesize that MSC coculture and MSC-EVs treated Mreg populations may promote resolution via reduction of Th17 pathogenicity or Th17 conversion. The hypothesis is supported by findings by Chiossone et al. (25), who reported induction of CD25highFoxp3+ regulatory T cells by MSC-educated macrophages and by Melief et al. (48) presenting that induction of regulatory T cells by MSC involves skewing monocytes toward M2-type macrophages.
Specialized proresolving mediators are synthetized from n-6 and n-3 fatty acids such as arachidonic acid, eicosapentaenoic acid, and docosahexaenoic acid. The biosynthetic pathways of SPMs include multiple intermediates, which may also serve as pathway markers (49). We identified TxB2, PGE2, 15-HETE, 18-HEPE, 17-HDHA, and 14S-HDHA from the Mreg-conditioned media with both MSC coculture and MSC-EVs. These molecules belong to the reported LM profiles produced by human macrophages (16). We could not detect any proresolving end products, the levels of which likely remained under the detection limit of our LC–MS/MS system. Although a well-known macrophage-derived SPM, maresin 1, was not detected with the current method, a maresin pathway marker, 14S-HDHA, was identified. Generally, monohydroxy pathway markers are more stable than SPM end products.
We observed that MSCs and, for the first time, MSC-EVs increased the PGE2 production in the Mreg coculture. Conventionally, the PGE2 has been regarded as a pro-inflammatory molecule participating in the initiation of inflammation. However, PGE2 also possesses immunosuppressive properties (50, 51) and is considered one of the most potent immunosuppressive mechanisms of MSCs (21), shown also in the context of macrophage polarization by MSCs (23–25). The dual function of PGE2 might arise from the differing activities of PGE2 receptors (EPs). Poloso et al. recently reported that PGE2 regulates the IL-23 release in human monocyte-derived dendritic cells and that at low PGE2 concentrations the high-affinity EP4 increased IL-23 production (52). Another receptor, EP2 has been suggested to respond to high PGE2 amounts by downregulating the IL-23 release (52, 53). The mechanism via the EP2 may partly explain the observations of high PGE2 and decreased IL-23 in our study. The fact that MSC-EVs are also able to induce the production of PGE2 from responder cells represents an interesting novel mechanism of action supported by the findings of Liu et al. (54).
Prostaglandin E2 has been shown to induce LM class switching in neutrophils. Modulation of 5- and 15-LOX expressions leads to the inhibition of pro-inflammatory leukotriene B4 and increased production of proresolving LXA4 and its pathway marker 15S-HETE in neutrophils (14). In this study, the level of 15-HETE was increased in the coculture of Mreg and MSC, which may imply that the elevated concentration of PGE2 acted as a cue for LM class switching. During the course of resolution, other SPMs, such as Rvs, protectins, and maresins, are produced (15). Interestingly, RvD1, RvD2, and RvE1 have been reported to reduce IL-23 production in asthma, microbial peritonitis, and allergic airway mouse models, respectively (55–57). These findings suggest a possible association between the PGE2-induced class switching and the observed reduced IL-23 production.
Human Mregs have been investigated in cell-based immunosuppressive therapy in early-phase clinical trials in renal transplantations (6, 13, 58), and altogether, the need for the development of cell-based medicinal products is increasing. Modification of Mregs into enhanced tolerance-promoting phenotype is of interest, and our results displaying the reduction of IL-23 and IL-22, support a potential role for combining therapeutic Mregs with MSC or MSC-EVs. The underlying mechanisms, including the MSC-EV-derived induction of PGE2 production and the possible conversion of Th17 into regulatory T cells, require further studies.
Conclusion
In this study, we demonstrate that human MSCs and MSC-EVs are capable of inducing proresolving changes in mature human Mregs. Both MSCs and MSC-EVs decrease the production of pro-inflammatory IL-23 and IL-22 while increasing immunosuppressive PGE2 production. Our findings suggest that MSCs and MSC-EVs may potentiate the proresolving phenotype of Mregs and supports the rationale of further studying the underlying mechanisms. Priming of Mregs with MSC or MSC-EVs is a potential novel approach for promoting the efficacy of Mreg therapy.
Ethics Statement
BM MSC donors gave their voluntary, informed, and written consent before sample collection, and the study protocols were approved by the Ethical Committee of Northern Ostrobothnia Hospital District or Ethical Committee of Hospital District of Helsinki and Uusimaa, Finland. The utilization of anonymized PBMNCs from blood donors in research is in accordance with the rules of the Finnish Supervisory Authority for Welfare and Health (Valvira).
Author Contributions
KH, MH, SL, and EK designed the study. PL and MK provided human MSCs for the study. KH, VS, and MH completed the laboratory analyses. HR, MH, and RK set up the LC–MS/MS method. MH completed the lipid mediator analyses. KH performed the most of the data analyses. KH and MH interpreted the results and wrote the manuscript. SL, HR, RK, PL, MK, and EK critically revised the manuscript and contributed to discussion. All the authors approved the final manuscript.
Conflict of Interest Statement
The authors declare that the research was conducted in the absence of any commercial or financial relationships that could be construed as a potential conflict of interest.
Acknowledgments
This research was partially supported by Tekes—The Finnish Funding Agency for Innovation—to the SalWe GET IT DONE program (grant 3986/31/2013) and Clinical Research Funding (EVO/VTR grant). The authors thank Lotta Sankkila for valuable laboratory work.
Supplementary Material
The Supplementary Material for this article can be found online at https://www.frontiersin.org/articles/10.3389/fimmu.2018.00771/full#supplementary-material.
References
1. Wynn TA, Vannella KM. Macrophages in tissue repair, regeneration, and fibrosis. Immunity (2016) 44:450–62. doi:10.1016/j.immuni.2016.02.015
2. Newson J, Motwani MP, Kendall AC, Nicolaou A, Muccioli GG, Alhouayek M, et al. Inflammatory resolution triggers a prolonged phase of immune suppression through COX-1/mPGES-1-derived prostaglandin E2. Cell Rep (2017) 20:3162–75. doi:10.1016/j.celrep.2017.08.098
3. Mosser DM, Edwards JP. Exploring the full spectrum of macrophage activation. Nat Rev Immunol (2008) 8:958–69. doi:10.1038/nri2448
4. Murray P, Allen J, Biswas S, Fisher E, Gilroy D, Goerdt S, et al. Macrophage activation and polarization: nomenclature and experimental guidelines. Immunity (2014) 41:14–20. doi:10.1016/j.immuni.2014.06.008
5. Murray PJ. Macrophage polarization. Annu Rev Physiol (2016) 79:541–66. doi:10.1146/annurev-physiol-022516-034339
6. Hutchinson JA, Riquelme P, Geissler EK, Fandrich F. Human regulatory macrophages. Methods Mol Biol (2011) 677:181–92. doi:10.1007/978-1-60761-869-0_13
7. Ortega-Gomez A, Perretti M, Soehnlein O. Resolution of inflammation: an integrated view. EMBO Mol Med (2013) 5:661–74. doi:10.1002/emmm.201202382
8. Fadok VA, Bratton DL, Konowal A, Freed PW, Westcott JY, Henson PM. Macrophages that have ingested apoptotic cells in vitro inhibit proinflammatory cytokine production through autocrine/paracrine mechanisms involving TGF-beta, PGE2, and PAF. J Clin Invest (1998) 101:890–8. doi:10.1172/JCI1112
9. Anderson CF, Mosser DM. A novel phenotype for an activated macrophage: the type 2 activated macrophage. J Leukoc Biol (2002) 72:101–6. doi:10.1189/jlb.72.1.101
10. Fleming BD, Chandrasekaran P, Dillon LA, Dalby E, Suresh R, Sarkar A, et al. The generation of macrophages with anti-inflammatory activity in the absence of STAT6 signaling. J Leukoc Biol (2015) 98:395–407. doi:10.1189/jlb.2A1114-560R
11. Gerber JS, Mosser DM. Reversing lipopolysaccharide toxicity by ligating the macrophage Fc gamma receptors. J Immunol (2001) 166:6861–8. doi:10.4049/jimmunol.166.11.6861
12. Schif-Zuck S, Gross N, Assi S, Rostoker R, Serhan CN, Ariel A. Saturated-efferocytosis generates pro-resolving CD11b low macrophages: modulation by resolvins and glucocorticoids. Eur J Immunol (2011) 41:366–79. doi:10.1002/eji.201040801
13. Hutchinson JA, Riquelme P, Sawitzki B, Tomiuk S, Miqueu P, Zuhayra M, et al. Cutting edge: immunological consequences and trafficking of human regulatory macrophages administered to renal transplant recipients. J Immunol (2011) 187:2072–8. doi:10.4049/jimmunol.1100762
14. Levy BD, Clish CB, Schmidt B, Gronert K, Serhan CN. Lipid mediator class switching during acute inflammation: signals in resolution. Nat Immunol (2001) 2:612–9. doi:10.1038/89759
15. Serhan CN, Chiang N. Resolution phase lipid mediators of inflammation: agonists of resolution. Curr Opin Pharmacol (2013) 13:632–40. doi:10.1016/j.coph.2013.05.012
16. Dalli J, Serhan CN. Specific lipid mediator signatures of human phagocytes: microparticles stimulate macrophage efferocytosis and pro-resolving mediators. Blood (2012) 120:e60–72. doi:10.1182/blood-2012-04-423525
17. English K. Mechanisms of mesenchymal stromal cell immunomodulation. Immunol Cell Biol (2013) 91:19–26. doi:10.1038/icb.2012.56
18. Amarnath S, Foley JE, Farthing DE, Gress RE, Laurence A, Eckhaus MA, et al. Bone marrow-derived mesenchymal stromal cells harness purinergenic signaling to tolerize human Th1 cells in vivo. Stem Cells (2015) 33:1200–12. doi:10.1002/stem.1934
19. Meisel R, Zibert A, Laryea M, Gobel U, Daubener W, Dilloo D. Human bone marrow stromal cells inhibit allogeneic T-cell responses by indoleamine 2,3-dioxygenase-mediated tryptophan degradation. Blood (2004) 103:4619–21. doi:10.1182/blood-2003-11-3909
20. Kerkelä E, Laitinen A, Räbinä J, Valkonen S, Takatalo M, Larjo A, et al. Adenosinergic immunosuppression by human mesenchymal stromal cells requires co-operation with T cells. Stem Cells (2016) 34:781–90. doi:10.1002/stem.2280
21. Aggarwal S, Pittenger MF. Human mesenchymal stem cells modulate allogeneic immune cell responses. Blood (2005) 105:1815–22. doi:10.1182/blood-2004-04-1559
22. Saldanha-Araujo F, Ferreira FI, Palma PV, Araujo AG, Queiroz RH, Covas DT, et al. Mesenchymal stromal cells up-regulate CD39 and increase adenosine production to suppress activated T-lymphocytes. Stem Cell Res (2011) 7:66–74. doi:10.1016/j.scr.2011.04.001
23. Németh K, Leelahavanichkul A, Yuen PS, Mayer B, Parmelee A, Doi K, et al. Bone marrow stromal cells attenuate sepsis via prostaglandin E2-dependent reprogramming of host macrophages to increase their interleukin-10 production. Nat Med (2009) 15:42–9. doi:10.1038/nm.1905
24. Ylöstalo JH, Bartosh TJ, Coble K, Prockop DJ. Human mesenchymal stem/stromal cells cultured as spheroids are self-activated to produce prostaglandin E2 that directs stimulated macrophages into an anti-inflammatory phenotype. Stem Cells (2012) 30:2283–96. doi:10.1002/stem.1191
25. Chiossone L, Conte R, Spaggiari GM, Serra M, Romei C, Bellora F, et al. Mesenchymal stromal cells induce peculiar alternatively activated macrophages capable of dampening both innate and adaptive immune responses. Stem Cells (2016) 34:1909–21. doi:10.1002/stem.2369
26. Jackson MV, Morrison TJ, Doherty DF, McAuley DF, Matthay MA, Kissenpfennig A, et al. Mitochondrial transfer via tunneling nanotubes is an important mechanism by which mesenchymal stem cells enhance macrophage phagocytosis in the in vitro and in vivo models of ARDS. Stem Cells (2016) 34:2210–23. doi:10.1002/stem.2372
27. Tsoyi K, Hall SR, Dalli J, Colas RA, Ghanta S, Ith B, et al. Carbon monoxide improves efficacy of mesenchymal stromal cells during sepsis by production of specialized proresolving lipid mediators. Crit Care Med (2016) 44:e1236–45. doi:10.1097/CCM.0000000000001999
28. Fang X, Abbott J, Cheng L, Colby JK, Lee JW, Levy BD, et al. Human mesenchymal stem (stromal) cells promote the resolution of acute lung injury in part through lipoxin A4. J Immunol (2015) 195:875–81. doi:10.4049/jimmunol.1500244
29. Di Trapani M, Bassi G, Midolo M, Gatti A, Kamga PT, Cassaro A, et al. Differential and transferable modulatory effects of mesenchymal stromal cell-derived extracellular vesicles on T, B and NK cell functions. Sci Rep (2016) 6:24120. doi:10.1038/srep24120
30. Zhang B, Yin Y, Lai RC, Tan SS, Choo AB, Lim SK. Mesenchymal stem cells secrete immunologically active exosomes. Stem Cells Dev (2014) 23:1233–44. doi:10.1089/scd.2013.0479
31. Kilpinen L, Impola U, Sankkila L, Ritamo I, Aatonen M, Kilpinen S, et al. Extracellular membrane vesicles from umbilical cord blood-derived MSC protect against ischemic acute kidney injury, a feature that is lost after inflammatory conditioning. J Extracell Vesicles (2013) 2:21927. doi:10.3402/jev.v2i0.21927
32. Lai RC, Arslan F, Lee MM, Sze NS, Choo A, Chen TS, et al. Exosome secreted by MSC reduces myocardial ischemia/reperfusion injury. Stem Cell Res (2010) 4:214–22. doi:10.1016/j.scr.2009.12.003
33. Monsel A, Zhu YG, Gennai S, Hao Q, Hu S, Rouby JJ, et al. Therapeutic effects of human mesenchymal stem cell-derived microvesicles in severe pneumonia in mice. Am J Respir Crit Care Med (2015) 192:324–36. doi:10.1164/rccm.201410-1765OC
34. Lo Sicco C, Reverberi D, Balbi C, Ulivi V, Principi E, Pascucci L, et al. Mesenchymal stem cell-derived extracellular vesicles as mediators of anti-inflammatory effects: endorsement of macrophage polarization. Stem Cells Transl Med (2017) 6:1018–28. doi:10.1002/sctm.16-0363
35. Peura M, Bizik J, Salmenperä P, Noro A, Korhonen M, Pätilä T, et al. Bone marrow mesenchymal stem cells undergo nemosis and induce keratinocyte wound healing utilizing the HGF/c-Met/PI3K pathway. Wound Repair Regen (2009) 17:569–77. doi:10.1111/j.1524-475X.2009.00507.x
36. Leskelä HV, Risteli J, Niskanen S, Koivunen J, Ivaska KK, Lehenkari P. Osteoblast recruitment from stem cells does not decrease by age at late adulthood. Biochem Biophys Res Commun (2003) 311:1008–13. doi:10.1016/j.bbrc.2003.10.095
37. Le Faouder P, Baillif V, Spreadbury I, Motta JP, Rousset P, Chene G, et al. LC-MS/MS method for rapid and concomitant quantification of pro-inflammatory and pro-resolving polyunsaturated fatty acid metabolites. J Chromatogr B Analyt Technol Biomed Life Sci (2013) 932:123–33. doi:10.1016/j.jchromb.2013.06.014
38. Stables MJ, Shah S, Camon EB, Lovering RC, Newson J, Bystrom J, et al. Transcriptomic analyses of murine resolution-phase macrophages. Blood (2011) 118:e192–208. doi:10.1182/blood-2011-04-345330
39. Kim J, Hematti P. Mesenchymal stem cell-educated macrophages: a novel type of alternatively activated macrophages. Exp Hematol (2009) 37:1445–53. doi:10.1016/j.exphem.2009.09.004
40. Vasandan AB, Jahnavi S, Shashank C, Prasad P, Kumar A, Prasanna SJ. Human Mesenchymal stem cells program macrophage plasticity by altering their metabolic status via a PGE2-dependent mechanism. Sci Rep (2016) 6:38308. doi:10.1038/srep38308
41. Amin M, Darji K, No DJ, Wu JJ. Review of phase III trial data on IL-23 inhibitors tildrakizumab and guselkumab for psoriasis. J Eur Acad Dermatol Venereol (2017) 31:1627–32. doi:10.1111/jdv.14451
42. Yago T, Nanke Y, Kawamoto M, Kobashigawa T, Yamanaka H, Kotake S. IL-23 and Th17 disease in inflammatory arthritis. J Clin Med (2017) 6:81. doi:10.3390/jcm6090081
43. Neurath MF. Current and emerging therapeutic targets for IBD. Nat Rev Gastroenterol Hepatol (2017) 14:269–78. doi:10.1038/nrgastro.2016.208
44. Bettelli E, Carrier Y, Gao W, Korn T, Strom TB, Oukka M, et al. Reciprocal developmental pathways for the generation of pathogenic effector TH17 and regulatory T cells. Nature (2006) 441:235–8. doi:10.1038/nature04753
45. Zheng Y, Danilenko DM, Valdez P, Kasman I, Eastham-Anderson J, Wu J, et al. Interleukin-22, a T(H)17 cytokine, mediates IL-23-induced dermal inflammation and acanthosis. Nature (2007) 445:648–51. doi:10.1038/nature05505
46. Lee Y, Awasthi A, Yosef N, Quintana FJ, Xiao S, Peters A, et al. Induction and molecular signature of pathogenic TH17 cells. Nat Immunol (2012) 13:991–9. doi:10.1038/ni.2416
47. Gagliani N, Amezcua Vesely MC, Iseppon A, Brockmann L, Xu H, Palm NW, et al. Th17 cells transdifferentiate into regulatory T cells during resolution of inflammation. Nature (2015) 523:221–5. doi:10.1038/nature14452
48. Melief SM, Schrama E, Brugman MH, Tiemessen MM, Hoogduijn MJ, Fibbe WE, et al. Multipotent stromal cells induce human regulatory T cells through a novel pathway involving skewing of monocytes toward anti-inflammatory macrophages. Stem Cells (2013) 31:1980–91. doi:10.1002/stem.1432
49. Serhan CN, Petasis NA. Resolvins and protectins in inflammation resolution. Chem Rev (2011) 111:5922–43. doi:10.1021/cr100396c
50. Wang M, Honn KV, Nie D. Cyclooxygenases, prostanoids, and tumor progression. Cancer Metastasis Rev (2007) 26:525. doi:10.1007/s10555-007-9096-5
51. Phipps RP, Stein SH, Roper RL. A new view of prostaglandin E regulation of the immune response. Immunol Today (1991) 12:349–52. doi:10.1016/0167-5699(91)90064-Z
52. Poloso NJ, Urquhart P, Nicolaou A, Wang J, Woodward DF. PGE2 differentially regulates monocyte-derived dendritic cell cytokine responses depending on receptor usage (EP2/EP4). Mol Immunol (2013) 54:284–95. doi:10.1016/j.molimm.2012.12.010
53. Kalim KW, Groettrup M. Prostaglandin E2 inhibits IL-23 and IL-12 production by human monocytes through down-regulation of their common p40 subunit. Mol Immunol (2013) 53:274–82. doi:10.1016/j.molimm.2012.08.014
54. Liu X, Qu X, Chen Y, Liao L, Cheng K, Shao C, et al. Mesenchymal stem/stromal cells induce the generation of novel IL-10-dependent regulatory dendritic cells by SOCS3 activation. J Immunol (2012) 189:1182–92. doi:10.4049/jimmunol.1102996
55. Rogerio AP, Haworth O, Croze R, Oh SF, Uddin M, Carlo T, et al. Resolvin D1 and aspirin-triggered resolvin D1 promote resolution of allergic airways responses. J Immunol (2012) 189:1983–91. doi:10.4049/jimmunol.1101665
56. Haworth O, Cernadas M, Yang R, Serhan CN, Levy BD. Resolvin E1 regulates interleukin 23, interferon-gamma and lipoxin A4 to promote the resolution of allergic airway inflammation. Nat Immunol (2008) 9:873–9. doi:10.1038/ni.1627
57. Spite M, Norling LV, Summers L, Yang R, Cooper D, Petasis NA, et al. Resolvin D2 is a potent regulator of leukocytes and controls microbial sepsis. Nature (2009) 461:1287–91. doi:10.1038/nature08541
Keywords: regulatory macrophages, mesenchymal stromal cells, extracellular vesicles, interleukin-23, prostaglandin E2, resolution
Citation: Hyvärinen K, Holopainen M, Skirdenko V, Ruhanen H, Lehenkari P, Korhonen M, Käkelä R, Laitinen S and Kerkelä E (2018) Mesenchymal Stromal Cells and Their Extracellular Vesicles Enhance the Anti-Inflammatory Phenotype of Regulatory Macrophages by Downregulating the Production of Interleukin (IL)-23 and IL-22. Front. Immunol. 9:771. doi: 10.3389/fimmu.2018.00771
Received: 05 December 2017; Accepted: 28 March 2018;
Published: 12 April 2018
Edited by:
Anne Fletcher, Monash University, AustraliaReviewed by:
Fa-Ming Chen, The Fourth Military Medical University, ChinaFarida Djouad, INSERM Délégation Languedoc-Roussillon, France
Copyright: © 2018 Hyvärinen, Holopainen, Skirdenko, Ruhanen, Lehenkari, Korhonen, Käkelä, Laitinen and Kerkelä. This is an open-access article distributed under the terms of the Creative Commons Attribution License (CC BY). The use, distribution or reproduction in other forums is permitted, provided the original author(s) and the copyright owner are credited and that the original publication in this journal is cited, in accordance with accepted academic practice. No use, distribution or reproduction is permitted which does not comply with these terms.
*Correspondence: Kati Hyvärinen, kati.hyvarinen@bloodservice.fi