- Department of Biomedical Sciences, Institute of Translational Pharmacology, National Research Council, Rome, Italy
The innate immune system provides the first line of defense against pathogen infection though also influences pathways involved in cancer immunosurveillance. The innate immune system relies on a limited set of germ line-encoded sensors termed pattern recognition receptors (PRRs), signaling proteins and immune response factors. Cytosolic receptors mediate recognition of danger damage-associated molecular patterns (DAMPs) signals. Once activated, these sensors trigger multiple signaling cascades, converging on the production of type I interferons and proinflammatory cytokines. Recent studies revealed that PRRs respond to nucleic acids (NA) released by dying, damaged, cancer cells, as danger DAMPs signals, and presence of signaling proteins across cancer types suggests that these signaling mechanisms may be involved in cancer biology. DAMPs play important roles in shaping adaptive immune responses through the activation of innate immune cells and immunological response to danger DAMPs signals is crucial for the host response to cancer and tumor rejection. Furthermore, PRRs mediate the response to NA in several vaccination strategies, including DNA immunization. As route of double-strand DNA intracellular entry, DNA immunization leads to expression of key components of cytosolic NA-sensing pathways. The involvement of NA-sensing mechanisms in the antitumor response makes these pathways attractive drug targets. Natural and synthetic agonists of NA-sensing pathways can trigger cell death in malignant cells, recruit immune cells, such as DCs, CD8+ T cells, and NK cells, into the tumor microenvironment and are being explored as promising adjuvants in cancer immunotherapies. In this minireview, we discuss how cGAS–STING and RIG-I–MAVS pathways have been targeted for cancer treatment in preclinical translational researches. In addition, we present a targeted selection of recent clinical trials employing agonists of cytosolic NA-sensing pathways showing how these pathways are currently being targeted for clinical application in oncology.
Introduction
The innate immune system provides the first line of defense against pathogen infection. It relies on a small set of germ line-encoded sensors named pattern recognition receptors (PRRs), which are deputized to detection of pathogen-associated molecular patterns (PAMPs) and danger damage-associated molecular patterns (DAMPs) signals.
Nucleic acid (NA)-sensing is an essential mechanism of the innate immunity that utilizes cytosolic receptors to detect extranuclear DNA or extracellular RNA as DAMPs signals (1).
In mammalian cells, two paradigmatic cytosolic NA-sensing pathways are the cyclic GMP-AMP synthase (cGAS)–stimulator of interferon genes (STING) and the RIG-I-like receptors (RLRs)-MAVS pathways, which are responsible for cytosolic DNA and RNA sensing, respectively (2, 3). The cGAS is a DNA sensor protein, which, upon binding double-strand (ds) DNA independently of DNA sequence, and catalyzes the synthesis of 2′-3′-cyclic GMP-AMP (cGAMP) (4). cGAMP functions as a second messenger that, in turn, engages the endoplasmic reticulum (ER)-membrane adaptor protein STING. After its activation STING traffics from the ER via the Golgi to perinuclear endosomes recruiting tank-binding kinase 1 (TBK1). A phosphorylation cascade allows signal transmission leading to activation of interferon regulatory factor (IRF) 3 and nuclear factor κB (NF-κB) that translocate into the nucleus to drive transcription of type-I interferons (IFNs), interferon-stimulated genes (ISGs), proinflammatory cytokines and chemokines (5, 6). Cytosolic dsRNA sensing involves three sensor proteins, namely retinoic acid-induced gene-I (RIG-I), melanoma differentiation-associated gene 5 (MDA5) and laboratory of physiology and genetics 2 (LGP2) (7), collectively referred to as RLRs. RIG-I sensor preferentially detects 5′-triphosphate (5′-3p)-ending RNA and short dsRNA, while MDA5 recognize long dsRNA (8). The signaling pathway proceeds with interaction of RIG-I or MDA5 with the adaptor mitochondrial antiviral-signaling protein (MAVS) located in the outer mitochondrial membrane and activation of IRF3/IRF7 and NF-κB. The activation usually results in IFNs production, consequent induction of ISGs and activation of NF-kB target genes.
Cancer cells share key hallmarks such as oxidative stress, genome instability and mutations, and altered metabolic rate that can generate nuclear and/or mitochondrial DNA damage (9). Recent studies revealed that damaged NAs released by dying cancer cells can be sensed as DAMP danger signals by PRRs present on CD8α dendritic cells (DCs) in tumor microenvironment (TME), leading to activation of cGAS-STING and/or RIG-I/MDA5 signaling pathways. The consequent type I IFN secretion activates DCs in an autocrine or paracrine manner, resulting in their migration to tumor-draining lymph nodes, where DCs cross-prime naïve CD8+ T lymphocytes (10–13) (Figure 1).
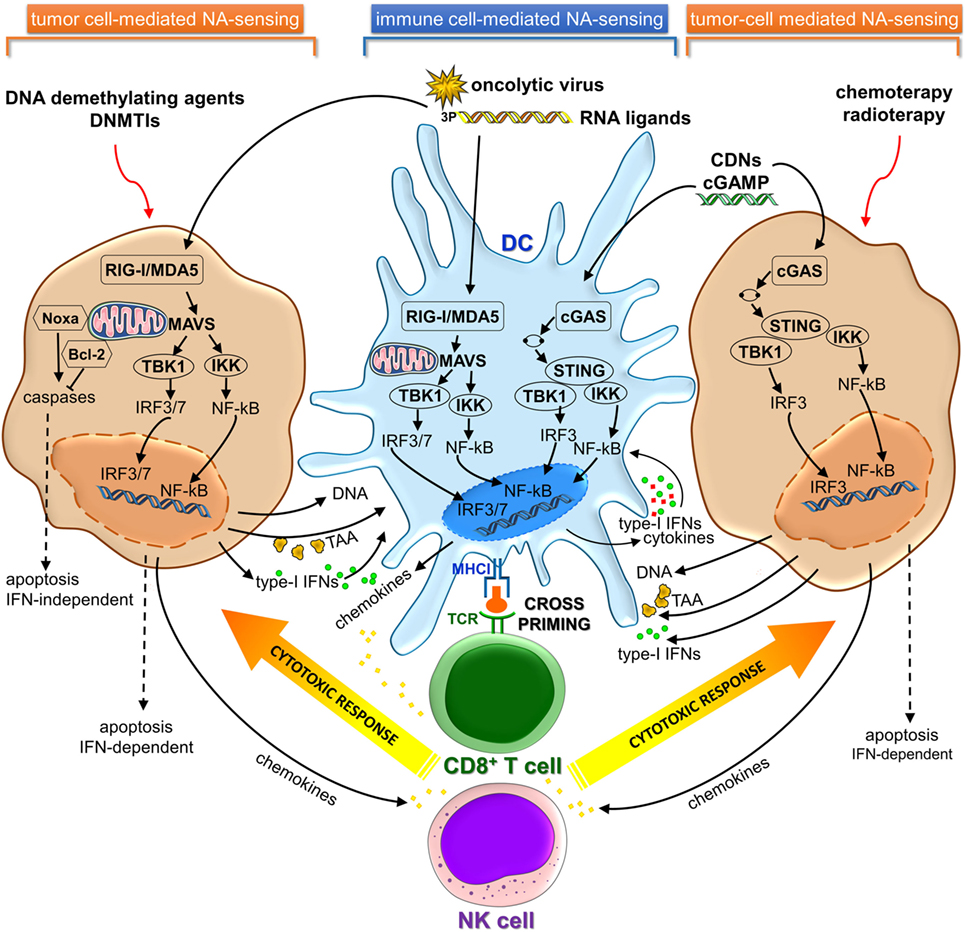
Figure 1. cGAS-STING and RIG-I/MDA5 signaling pathways in immune and cancer cells involved in cancer immunosurveillance and immunotherapy. cGAS, cyclic GMP-AMP synthase; STING, stimulator of interferon genes; RIG-I, retinoic acid-induced gene-I; MDA5, melanoma differentiation-associated gene 5; NA, nucleic acid; TAA, tumor-associated antigen; DNMTIs, DNA methyltransferase inhibitors.
Activation of cytosolic DNA sensing pathways impacts on autophagy and tumor antigens (Ags) cross-presentation in DCs. Type-I IFNs production by DCs actually represents the link between NAs sensing and effective Ags cross-presentation to CD8+ T cells, therefore linking innate and adaptive immunity (14). Type-I IFNs stimulate upregulation and consequent surface expression of MHC class I genes. Furthermore, type-I IFNs directly promote Ags intracellular retention in DCs that have engulfed apoptotic tumor cells through slowing the endosomal-lysosomal acidification rate, thus enhancing capacity to cross-present Ag by DCs (15–17). Since MHC class I cross-presentation depends on the time of persistence of Ag within the phagolysosomal compartment (16, 18), autophagy possibly provides an intracellular depot where Ag is stored, rather than degraded and represents an alternative pathway for MHC class I presentation (19–21).
Endosomal tumor-derived NAs escape into the DC cytosol through a yet not completely understood mechanism. Specific internalizing receptors such as CLEC9A and CD205 and high-mobility group box 1 protein can mediate uptake of genetic material from dying tumor cells and affect subsequent endosomal trafficking (22–24). Likewise tumor-derived Ags, released DNA could be retained in the endolysosomal compartment where it is preserved before it gains access to the cytosol where it can be recognized by cGAS and other sensor proteins such as intereferon-γ-inducible protein 16 (IFI16), absent in melanoma 2 (AIM2), and Z-DNA-binding protein 1 (ZBP1) (10, 11, 14). The delayed endosomal acidification may further contribute to reduce DNA degradation by DNAse II protease.
Presence of NA sensor proteins across cancer types suggests that these signaling mechanisms may be involved in cancer biology. Actually the expression of RIG-I was significantly downregulated in human hepatic carcinoma (HCC) tissues (25, 26).
Clinical studies revealed that the expression of STING was significantly reduced in HCC tissues compared to the controls, and lower expression of STING was associated with a more advanced tumor stages and a worse survival (27) as well with poor prognosis for patients with gastric cancer (28). Collectively, these studies suggest that STING and RIG-I sensors may serve as tumor suppressors and have clinical values against certain types of tumors as prognostic/predictive biomarkers.
Furthermore, PRRs mediate the response to NAs in several vaccination approaches, including DNA immunization. As route of dsDNA intracellular entry, DNA immunization leads to expression of key components of the cytosolic NA-sensing pathways.
Recent data showed that natural and synthetic agonists of NA-sensing pathways could trigger cell death in malignant cells and recruit immune cells, such as DCs, CD8+ T cells, and NK cells into the TME.
In this minireview, we will highlight the newest insights from preclinical studies demonstrating the relevance of manipulating the cGAS-STING and RLRs-MAVS signaling pathways for cancer treatment and how these pathways are currently being targeted pharmacologically (Figure 1).
Clinical evaluation of these innate immune modulators, with agonists alone and in combination with other immunomodulatory agents demonstrates the high translational potential for cGAS-STING and RLRs-MAVS signaling pathway engagement. In Table 1 and Table S1 in Supplementary Material, we present a selection of very recent and novel therapies employing agonists of cytosolic NA-sensing pathways in oncology and provide detailed information concerning mechanisms of action, assessments, and outcomes of reported clinical trials.
Results from completed early phase clinical trials with human STING and RIG-I agonists showed biologic and therapeutic effects in patients, leading to combination clinical trials with checkpoint inhibitors (31, 43).
Preclinical Evaluation of Sting Agonists for Cancer Treatment
Regulation and function of the cGAS-STING pathway has been reviewed elsewhere (3, 46–50), so we will briefly consider newest insights into the topic of STING agonists as potent anticancer agents in preclinical models.
STING pathway has been mostly characterized in APCs, meanwhile in the TME, T cells, endothelial cells, and fibroblasts, stimulated with STING agonists ex vivo, have been found to produce type-I IFNs (29). By contrast, most studies indicated that tumor cells developed strategies to inhibit STING pathway activation, likely for immune evasion during carcinogenesis (51, 52).
Recent pieces of evidence have indicated that activation of the STING pathway was correlated to the induction of a spontaneous antitumor T-cell response involving the expression of type-I IFN genes (3, 10, 53). Furthermore, host STING pathway is required for efficient cross-priming of tumor-Ag specific CD8+ T cells mediated by DCs (10, 54) (Figure 1).
Based on these findings, direct pharmacologic stimulation of the STING pathway has been explored as a cancer therapy.
Demaria et al. demonstrated CD8+ T and type-I IFNs dependent antitumor effect of cGAMP, a natural STING ligand, in melanoma and colon cancer mice models (55).
In 2016, Li et al. confirmed the potent antitumor effect of intratumoral (i.t.) injection of cGAMP in CT26 colon adenocarcinoma-bearing mice. The antitumor activity of cGAMP relied on DC activation and CD8+T cell cross-priming (56). More recently, Ohkuri et al. demonstrated accumulation and antitumor effect of potent macrophages in mouse TME of breast cancer, squamous cell carcinomas, colon cancer, and melanoma tissues (57) after i.t. injection of cGAMP.
Canonical cyclic-dinucleotides (CDNs), as direct agonists for STING, show a poor ability to activate human STING. Therefore, an increasing number of synthetic CDNs that potently activate all human STING variants have been designed in recent years (29, 58).
New synthetic CDNs agonist has shown potent antitumor efficiency in various tumor models such as B16F10 melanoma, 4T1 mammary adenocarcinoma, and CT26 colon carcinoma, with regression of established tumor, metastasis rejection, and establishment of long-term immune memory (29).
Recently, many preclinical studies draw a blueprint for the application of STING agonists in tumor therapy in the context of combination therapies.
Strategies that combine STING immunotherapy with other immunomodulatory agents are being explored in mouse models. The antitumor efficacy of cGAMP administered by i.t. injection into B16.F10 tumors was enhanced when combined with anti-programmed death-1 (PD-1) and anti-cytotoxic T-lymphocyte associated-4 (CTLA-4) antibodies (55). In other studies, CDNs together with anti-PD-1 incited much stronger antitumor effects than monotherapy in a mouse model of squamous cell carcinoma model as well of melanoma (59, 60). Luo et al. showed great synergy by combining a STING-activating nanovaccine and an anti-PD1 antibody, and suggested generation of long-term antitumor memory in TC-1 tumor model (61). STING agonists can enhance antitumor responses when combining with tumor vaccines. CDN ligands formulated with granulocyte-macrophage colony-stimulating factor-producing cellular cancer vaccines, termed STINGVAX, showed strong in vivo therapeutic efficacy in several models of established cancer. Antitumor activity was STING dependent and corresponded to activation of DCs and tumor antigen-specific CD8+ T cells. STINGVAX combined with PD-1 blockade induced regression of poorly immunogenic tumors that did not respond to PD-1 blockade alone (62). STING agonists in combination with traditional chemotherapeutic agents or radiotherapy can work synergistically to trigger antitumor response (56, 63).
The focus of STING pathway agonists for clinical use has thus far centered on their role as vaccine adjuvants and as cancer immunotherapeutic agents for treatment of solid tumors. However, induction of type-I IFNs and other inflammatory cytokines through STING pathway activation results in potent leukemia-specific immunity, culminating in impressive improvements in survival of preclinical acute myeloid leukemia models. Thus, Curran et al. provided solid rationale for clinical translation of STING agonists as immune therapy for leukemia and other hematologic cancers (64).
The intricate STING role may be associated with cell type and activated intensity of downstream signaling. Agonist-mediated activation of STING induces apoptosis in malignant B-cells through specific cytotoxicity, suggesting the potential therapeutic use of STING agonists in treating B-cell malignancies (65). Meanwhile, STING activation reverses lymphoma-mediated resistance to antibody immunotherapy through macrophage activation and modulation of intratumoral macrophage phenotype, as showed by Dahal et al. (66). The induction of apoptosis seems to be a general effector response of the STING pathway in lymphocytes. Gulen et al. reported that overt stimulation of the STING pathway in primary and malignant T cells elicits apoptosis through induction of IRF-3-dependent and p53-dependent proapoptotic genes. This phenomenon, which is evident upon strong stimulus delivery, reveals that the signaling strength determines proapoptotic functions of STING (67). In agreement, low and short in vivo activation of STING in T cells provokes type-I IFNs production and ISGs expression mimicking the response of innate cells (68).
Targeting RIG-I/MDA5 Pathway for Cancer Therapy
RIG-I-like receptors are expressed in most tissues, including cancer cells (69). Recent studies have demonstrated that promising druggable targets against cancer may be represented by components of antiviral immune response. Tumor cells and virus-infected cells can be regarded as injured host cells sharing common features (70, 71). In fact, cancer cells can be induced to mimic a viral infection using RLRs ligands to activate cytosolic RNA-sensing pathway and IFN response (44, 72). This activation also can result in stimulation of cytotoxic immune cells, such as NK and CD8+ T cells, which kill cancer cells via extrinsic and intrinsic apoptosis (73–75). Consequently, activation of RLRs by using synthetic ligands or oncolytic virus in tumor cells can induce cell death in an IFN-dependent or IFN-independent manner (44, 72–74, 76–78) (Figure 1). Several types of bifunctional small interfering RNAs (siRNAs) with 5′-3p ends conferring a non-self RNA PAMP (79) were developed for both silencing oncogenic or immunosuppressive genes and inducing cell death mediated by viral mimicry (12, 13, 73, 77, 80, 81). Systemic administration of a siRNA designed to trigger RIG-I and silence Bcl2 induced DC-dependent production of IFNs and strongly inhibited tumor growth in B16 melanoma model. These RIG-I-mediated immune responses synergized with siRNA-mediated Bcl2 silencing to promote massive tumor apoptosis in lung metastases in vivo (73). Likewise, in human drug-resistant leukemia cell lines treatment with multifunctional 5′-3P-siRNA downregulated multi-drug resistance 1 (MDR1) expression and triggered RIG-I-dependent intrinsic apoptosis pathway involving upregulation of Noxa protein, cytochrome-C, and effector caspases (81). On the other hand, small endogenous non-coding RNAs gave rise to RIG-I:RNA complexes and initiated downstream signaling events, after ionizing radiation treatment (82). Antitumor DNA-demethylating agent, 5-AZA-CdR, and DNA methyltransferase inhibitors (DNMTis) triggered cytosolic sensing of dsRNA in cancer cells activating endogenous retroviruses and, thus, mimicking a viral infection. The increased viral defense gene expression induced a type-I IFN signaling and apoptosis (44, 72). Furthermore, DNMTi treatment potentiated anti-CTLA-4 immune checkpoint therapy in a pre-clinical melanoma model (44).
Besides the dual tumoricidal property, there are several advantages of targeting RIG-I/MDA5 signaling pathway for cancer immunotherapy. It has been reported that malignant cells are highly sensitive to RIG-I/MDA-5 proapoptotic signaling pathway (74, 83), whereas normal cells are less susceptible as they are rescued from apoptosis by upregulation or activation of endogenous Bcl-xL, which prevents RIG-I/MDA5-induced cell death (74). Furthermore, RIG-I and MDA5 are able to trigger a p53-independent alternative pathway for the induction of proapoptotic Noxa. Hence, RIG-I/MDA5-driven apoptosis is not mediated by the tumor suppressor p53 mutational status in cancer cells (74), which strongly contributes to resistance to chemo- and radiotherapy (84).
In Table 1 and Table S1 in Supplementary Material are reported some RIG-I/MDA5 ligands that are being utilized in clinical trials. Overall, triggering RIG-I/MDA5 pathway results in eliciting both immunostimulatory and proapoptotic activity conferring to RIG-I/MDA5 a pivotal role in tumor evasion from immune surveillance. Yet it is noteworthy that stimulation of cancer cells by RIG-I ligands not only cause apoptosis but also enhance DCs Ag cross-priming through type-I IFNs release and upregulation of MHC class I gene expression (12, 75, 76).
Stimulation of Innate Immune PRRs by DNA Vaccines
In the last decade, several DNA vaccines products have been licensed for animal use demonstrating the wide applications of the DNA-based vaccine, such as Apex®-IHN, West Nile-Innovator®, and ONCEPT® (85–88). DNA vaccines can induce both humoral and cellular immune responses. When used in humans, however, DNA vaccines suffer from lower immunogenicity profiles (89).
Several studies confirmed that immunogenicity of DNA vaccines is regulated by critical components of the innate immune system via plasmid DNA recognition through the STING–TBK1 pathway.
The DNA vaccine “adjuvant effect” is not TLR9 dependent, indeed, both TLR9- and MyD88-deficient treated mice mount immune responses comparable to wild-type mice (90). Such immunogenicity leads to the production of type-I IFNs that were found to be crucial for both direct- and indirect-antigen presentation via distinct cell types (i.e. DCs and muscle cells, respectively), resulting in the adjuvant effect for the encoded antigen (91, 92). However, the requirement for IFN-αβ in generating high-level antibody responses has yielded contradictory results. The necessity for IRF3 in cellular-mediated immune responses was previously demonstrated, but with a more limited impact, as described by Suschak et al. (90). Indeed, the temporary defect in immune priming provided by Irf3 deletion is overcome by the induction of Irf7, allowing for rescue of DNA vaccine immunogenicity.
The acknowledged versatility of plasmid DNA facilitates the co-delivery of genetic adjuvants encoding immune-stimulatory molecules that need to be overexpressed and selected antigen/s, producing a new generation of vaccines that stand out for their safety and feasibility. In a preclinical study, the co-administration of two plasmid vectors one encoding the DNA sensor DAI and the other one the melanoma-associated antigen tyrosinase-related protein-2 (TRP2) resulted in enhanced tumor rejection and protection against B16 melanoma challenge (93).
The concerted stimulation of innate immune PRRs by DNA vaccines can achieve a more potent and broader activation of the immune responses and a long-lasting protective adaptive immunity.
Co-expressing TBK1 and the serine repeat antigen, a candidate vaccine antigen expressed in the blood-stages of Plasmodium falciparum, in the same plasmid backbone enhanced the antigen-specific humoral immune responses, but failed to improve cellular immune responses (91).
Favorable safety profile and potential clinical benefit were achieved after the phase I clinical trial on the activity of CYL-02, a non-viral gene therapy product that sensitizes pancreatic cancer cells to gemcitabine, a chemotherapic acting as a STING pathway agonist, and a non-invasive biomarkers for patient selection was identified (32).
RIG-I and MDA5-activating DNA vaccines can elicit apoptotic and immunostimulatory effects and, thus, could induce growth inhibition or apoptosis of multiple types of cancer cells. Plasmid vector backbones expressing composite immunostimulatory RNAs that act as synergic RIG-I agonists lead to type-I IFNs production (92, 94).
Conclusion and Perspectives
The spatiotemporal orchestration of innate stimulation with antigen cross-presentation in APCs represents a crucial challenge in reaching a strong tumor specific T-cell response, which in turn is crucial for cancer immunotherapy.
Several studies suggest that cytosolic NA-sensing plays a central role in inducing and bridging innate immunity and adaptive immune responses against tumors and that triggering innate immune system contribute to counteract tumor-induced immunosuppression. Employment of RIG-I/MDA5 and cGAS-STING agonists could represent a novel strategy for cancer immunotherapy.
The role of cGAS-STING and RLRs-MAVS pathways in tumor immunity remains complex and numerous questions still remain unanswered.
Noteworthy, some studies suggest that STING activation may induce a suppressive TME, contributing to tumor growth and metastasis and that STING agonists may not be effective against all tumors, particularly those with tolerogenic responses to DNA and low tumor antigenicity (95, 96). Sensing of DNA by specific innate immune or other cell types and different routes of acute or chronic DNA exposure influence immune responses to DNA.
In head and neck squamous cell carcinoma, dose-dependent activation of RIG-I resulted in divergent effects on cancer cell proliferation. Actually, low dose of dsRNA promoted NF-kB- and Akt-dependent cell proliferation and metastasis (97).
The functional consequences of the cGAS-STING and RIG-I-MAVS pathways regulation/activation in different cells within the TME require deeper characterization. Targeting multiple pathways may be required for efficacious therapeutic responses in some patients and the crosstalk between RIG-I and STING pathways through direct interactions between downstream signaling components may amplify the innate response.
The complex role of STING and RIG-I signaling in cancer underlines how innate immune pathways in the TME alter tumorigenesis in distinct tumors, with effects in designing efficacious immunotherapy trials.
Author Contributions
SI: searched for literature articles, conceived and wrote the manuscript, conceived the figure, and revised and approved the final version of the manuscript. DF: performed search for clinical trials, collected and analyzed clinical trials data, prepared the tables, conceived and prepared the figure, contributed to the manuscript preparation and editing, and revised and approved the final version of the manuscript. MR: searched for literature articles, conceived and wrote the manuscript, edited the manuscript, and revised and approved the final version of the manuscript.
Conflict of Interest Statement
The authors declare that the research was conducted in the absence of any commercial or financial relationships that could be construed as a potential conflict of interest.
Acknowledgments
The authors thank Dr. Ivano Condò (University Tor Vergata, Rome, Italy) for critical reading of the manuscript and helpful discussion. The authors would like to acknowledge all the contributions in the field that could not be included in the review. This work was supported by the grant from the National Italian Research Council (CNR) “Progetto CNR–DSB.AD007.107” to MR and “Progetto CNR–DSB.AD007.090” to SI.
Supplementary Material
The Supplementary Material for this article can be found online at https://www.frontiersin.org/articles/10.3389/fimmu.2018.00711/full#supplementary-material.
References
1. Desmet CJ, Ishii KJ. Nucleic acid sensing at the interface between innate and adaptive immunity in vaccination. Nat Rev Immunol (2012) 12(7):479–91. doi:10.1038/nri3247
2. Wu J, Chen ZJ. Innate immune sensing and signaling of cytosolic nucleic acids. Annu Rev Immunol (2014) 32:461–88. doi:10.1146/annurev-immunol-032713-120156
3. Chen Q, Sun L, Chen ZJ. Regulation and function of the cGAS-STING pathway of cytosolic DNA sensing. Nat Immunol (2016) 17(10):1142–9. doi:10.1038/ni.3558
4. Wu J, Sun L, Chen X, Du F, Shi H, Chen C, et al. Cyclic GMP-AMP is an endogenous second messenger in innate immune signaling by cytosolic DNA. Science (2013) 339(6121):826–30. doi:10.1126/science.1229963
5. Ishikawa H, Ma Z, Barber GN. STING regulates intracellular DNA-mediated, type I interferon-dependent innate immunity. Nature (2009) 461(7265):788–92. doi:10.1038/nature08476
6. Abe T, Barber GN. Cytosolic-DNA-mediated, STING-dependent proinflammatory gene induction necessitates canonical NF-kappaB activation through TBK1. J Virol (2014) 88(10):5328–41. doi:10.1128/JVI.00037-14
7. Yoneyama M, Onomoto K, Jogi M, Akaboshi T, Fujita T. Viral RNA detection by RIG-I-like receptors. Curr Opin Immunol (2015) 32:48–53. doi:10.1016/j.coi.2014.12.012
8. Schlee M. Master sensors of pathogenic RNA – RIG-I like receptors. Immunobiology (2013) 218(11):1322–35. doi:10.1016/j.imbio.2013.06.007
9. Luo J, Solimini NL, Elledge SJ. Principles of cancer therapy: oncogene and non-oncogene addiction. Cell (2009) 136(5):823–37. doi:10.1016/j.cell.2009.02.024
10. Woo SR, Fuertes MB, Corrales L, Spranger S, Furdyna MJ, Leung MY, et al. STING-dependent cytosolic DNA sensing mediates innate immune recognition of immunogenic tumors. Immunity (2014) 41(5):830–42. doi:10.1016/j.immuni.2014.10.017
11. Klarquist J, Hennies CM, Lehn MA, Reboulet RA, Feau S, Janssen EM. STING-mediated DNA sensing promotes antitumor and autoimmune responses to dying cells. J Immunol (2014) 193(12):6124–34. doi:10.4049/jimmunol.1401869
12. Duewell P, Steger A, Lohr H, Bourhis H, Hoelz H, Kirchleitner SV, et al. RIG-I-like helicases induce immunogenic cell death of pancreatic cancer cells and sensitize tumors toward killing by CD8(+) T cells. Cell Death Differ (2014) 21(12):1825–37. doi:10.1038/cdd.2014.96
13. Ellermeier J, Wei J, Duewell P, Hoves S, Stieg MR, Adunka T, et al. Therapeutic efficacy of bifunctional siRNA combining TGF-beta1 silencing with RIG-I activation in pancreatic cancer. Cancer Res (2013) 73(6):1709–20. doi:10.1158/0008-5472.CAN-11-3850
14. Vatner RE, Janssen EM. STING, DCs and the link between innate and adaptive tumor immunity. Mol Immunol (2017). doi:10.1016/j.molimm.2017.12.001
15. Reboulet RA, Hennies CM, Garcia Z, Nierkens S, Janssen EM. Prolonged antigen storage endows merocytic dendritic cells with enhanced capacity to prime anti-tumor responses in tumor-bearing mice. J Immunol (2010) 185(6):3337–47. doi:10.4049/jimmunol.1001619
16. Lorenzi S, Mattei F, Sistigu A, Bracci L, Spadaro F, Sanchez M, et al. Type I IFNs control antigen retention and survival of CD8alpha(+) dendritic cells after uptake of tumor apoptotic cells leading to cross-priming. J Immunol (2011) 186(9):5142–50. doi:10.4049/jimmunol.1004163
17. Diamond MS, Kinder M, Matsushita H, Mashayekhi M, Dunn GP, Archambault JM, et al. Type I interferon is selectively required by dendritic cells for immune rejection of tumors. J Exp Med (2011) 208(10):1989–2003. doi:10.1084/jem.20101158
18. van Montfoort N, Camps MG, Khan S, Filippov DV, Weterings JJ, Griffith JM, et al. Antigen storage compartments in mature dendritic cells facilitate prolonged cytotoxic T lymphocyte cross-priming capacity. Proc Natl Acad Sci U S A (2009) 106(16):6730–5. doi:10.1073/pnas.0900969106
19. Li Y, Wang LX, Yang G, Hao F, Urba WJ, Hu HM. Efficient cross-presentation depends on autophagy in tumor cells. Cancer Res (2008) 68(17):6889–95. doi:10.1158/0008-5472.CAN-08-0161
20. Chemali M, Radtke K, Desjardins M, English L. Alternative pathways for MHC class I presentation: a new function for autophagy. Cell Mol Life Sci (2011) 68(9):1533–41. doi:10.1007/s00018-011-0660-3
21. Mintern JD, Macri C, Chin WJ, Panozza SE, Segura E, Patterson NL, et al. Differential use of autophagy by primary dendritic cells specialized in cross-presentation. Autophagy (2015) 11(6):906–17. doi:10.1080/15548627.2015.1045178
22. Sancho D, Joffre OP, Keller AM, Rogers NC, Martinez D, Hernanz-Falcon P, et al. Identification of a dendritic cell receptor that couples sensing of necrosis to immunity. Nature (2009) 458(7240):899–903. doi:10.1038/nature07750
23. Shrimpton RE, Butler M, Morel AS, Eren E, Hue SS, Ritter MA. CD205 (DEC-205): a recognition receptor for apoptotic and necrotic self. Mol Immunol (2009) 46(6):1229–39. doi:10.1016/j.molimm.2008.11.016
24. Yanai H, Ban T, Wang Z, Choi MK, Kawamura T, Negishi H, et al. HMGB proteins function as universal sentinels for nucleic-acid-mediated innate immune responses. Nature (2009) 462(7269):99–103. doi:10.1038/nature08512
25. Hou J, Zhou Y, Zheng Y, Fan J, Zhou W, Ng IO, et al. Hepatic RIG-I predicts survival and interferon-alpha therapeutic response in hepatocellular carcinoma. Cancer Cell (2014) 25(1):49–63. doi:10.1016/j.ccr.2013.11.011
26. Liu Z, Dou C, Yao B, Xu M, Ding L, Wang Y, et al. Ftx non coding RNA-derived miR-545 promotes cell proliferation by targeting RIG-I in hepatocellular carcinoma. Oncotarget (2016) 7(18):25350–65. doi:10.18632/oncotarget.8129
27. Bu Y, Liu F, Jia QA, Yu SN. Decreased expression of TMEM173 predicts poor prognosis in patients with hepatocellular carcinoma. PLoS One (2016) 11(11):e0165681. doi:10.1371/journal.pone.0165681
28. Song S, Peng P, Tang Z, Zhao J, Wu W, Li H, et al. Decreased expression of STING predicts poor prognosis in patients with gastric cancer. Sci Rep (2017) 7:39858. doi:10.1038/srep39858
29. Corrales L, Glickman LH, McWhirter SM, Kanne DB, Sivick KE, Katibah GE, et al. Direct activation of STING in the tumor microenvironment leads to potent and systemic tumor regression and immunity. Cell Rep (2015) 11(7):1018–30. doi:10.1016/j.celrep.2015.04.031
30. ClinicalTrials.gov. Study of the Safety and Efficacy of MIW815 (ADU-S100) in Patients with Advanced/Metastatic Solid Tumors or Lymphomas (NCT02675439). (2016). Available from: https://clinicaltrials.gov/ct2/show/NCT02675439?term=NCT02675439&rank=1. (Accessed: October 25, 2016).
31. ClinicalTrials.gov. Study of the Safety and Efficacy of MIW815 with PDR001 to Patients with Advanced/Metastatic Solid Tumors or Lymphoma (NCT03172936). (2017). Available from: https://clinicaltrials.gov/ct2/show/NCT03172936. (Accessed: November 17, 2017).
32. Buscail L, Bournet B, Vernejoul F, Cambois G, Lulka H, Hanoun N, et al. First-in-man phase 1 clinical trial of gene therapy for advanced pancreatic cancer: safety, biodistribution, and preliminary clinical findings. Mol Ther (2015) 23(4):779–89. doi:10.1038/mt.2015.1
33. ClinicalTrials.gov. Gene Therapy of Pancreatic Ductal Adenocarcinoma (TherGAP) (NCT01274455). (2010). Available from: https://clinicaltrials.gov/ct2/show/NCT01274455?term=NCT01274455&rank=1. (Accessed: March 11, 2016).
34. ClinicalTrials.gov. Effect of Intratumoral Injection of Gene Therapy for Locally Advanced Pancreatic Cancer (THERGAP-02) (NCT02806687). (2016). Available from: https://clinicaltrials.gov/ct2/show/NCT02806687?term=NCT02806687&rank=1. (Accessed: February 13, 2017).
35. Trial U-CC. Phase I/II Clinical Trial of Inactivated HVJ-E Administration for Advanced Malignant Melanoma Patients (UMIN000002376). (2009). Available from: https://upload.umin.ac.jp/cgi-open-bin/ctr_e/ctr_view.cgi?recptno=R000002889. (Accessed: January 1, 2012).
36. Nakajima T, Itai T, Wada H, Yamauchi T, Kiyohara E and Kaneda Y. A novel therapy for melanoma and prostate cancer using a non-replicating sendai virus particle (HVJ-E). In: Wei M, Good D, editors. Novel gene therapy approaches. InTech, (2013). doi:10.5772/55014
37. Tanemura A, Kiyohara E, Katayama I, Kaneda Y. Recent advances and developments in the antitumor effect of the HVJ envelope vector on malignant melanoma: from the bench to clinical application. Cancer Gene Ther (2013) 20(11):599–605. doi:10.1038/cgt.2013.61
38. Trial U-CC. Phase I/II clinical trial to assess safety and efficacy of intratumoral and subcutaneous injection of HVJ-E to castration resistant prostate cancer patients (UMIN000006142). (2011). Available from: https://upload.umin.ac.jp/cgi-open-bin/ctr_e/ctr_view.cgi?recptno=R000007153. (Accessed: July 1, 2014).
39. Fujita K, Nakai Y, Kawashima A, Ujike T, Nagahara A, Nakajima T, et al. Phase I/II clinical trial to assess safety and efficacy of intratumoral and subcutaneous injection of HVJ-E in castration-resistant prostate cancer patients. Cancer Gene Ther (2017) 24(7):277–81. doi:10.1038/cgt.2017.15
40. ClinicalTrials.gov. Azacitidine and Entinostat in Treating Patients with Metastatic Colorectal Cancer (NCT01105377). (2010). Available from: https://clinicaltrials.gov/ct2/show/NCT01105377. (Accessed: August 1, 2014).
41. Li H, Chiappinelli KB, Guzzetta AA, Easwaran H, Yen RW, Vatapalli R, et al. Immune regulation by low doses of the DNA methyltransferase inhibitor 5-azacitidine in common human epithelial cancers. Oncotarget (2014) 5(3):587–98. doi:10.18632/oncotarget.1782
42. ClinicalTrials.gov. Azacitidine and Entinostat in Treating Patients with Advanced Breast Cancer (NCT01349959). (2011). Available from: https://clinicaltrials.gov/ct2/show/NCT01349959. (Accessed: December 30, 2016).
43. ClinicalTrials.gov. Phase II Anti-PD1 Epigenetic Therapy Study in NSCLC. (NA_00084192) (NCT01928576). (2013). Available from: https://clinicaltrials.gov/ct2/show/NCT01928576. (Accessed: October 6, 2017).
44. Chiappinelli KB, Strissel PL, Desrichard A, Li H, Henke C, Akman B, et al. Inhibiting DNA methylation causes an interferon response in cancer via dsRNA including endogenous retroviruses. Cell (2015) 162(5):974–86. doi:10.1016/j.cell.2015.07.011
45. Wrangle J, Wang W, Koch A, Easwaran H, Mohammad HP, Vendetti F, et al. Alterations of immune response of non-small cell lung cancer with azacytidine. Oncotarget (2013) 4(11):2067–79. doi:10.18632/oncotarget.1542
46. Corrales L, Matson V, Flood B, Spranger S, Gajewski TF. Innate immune signaling and regulation in cancer immunotherapy. Cell Res (2017) 27(1):96–108. doi:10.1038/cr.2016.149
47. Corrales L, McWhirter SM, Dubensky TW Jr, Gajewski TF. The host STING pathway at the interface of cancer and immunity. J Clin Invest (2016) 126(7):2404–11. doi:10.1172/JCI86892
48. Rivera Vargas T, Benoit-Lizon I, Apetoh L. Rationale for stimulator of interferon genes-targeted cancer immunotherapy. Eur J Cancer (2017) 75:86–97. doi:10.1016/j.ejca.2016.12.028
49. Qiao J, Tang H, Fu YX. DNA sensing and immune responses in cancer therapy. Curr Opin Immunol (2017) 45:16–20. doi:10.1016/j.coi.2016.12.005
50. He L, Xiao X, Yang X, Zhang Z, Wu L, Liu Z. STING signaling in tumorigenesis and cancer therapy: a friend or foe? Cancer Lett (2017) 402:203–12. doi:10.1016/j.canlet.2017.05.026
51. Xia T, Konno H, Ahn J, Barber GN. Deregulation of STING signaling in colorectal carcinoma constrains DNA damage responses and correlates with tumorigenesis. Cell Rep (2016) 14(2):282–97. doi:10.1016/j.celrep.2015.12.029
52. Xia T, Konno H, Barber GN. Recurrent loss of STING signaling in melanoma correlates with susceptibility to viral oncolysis. Cancer Res (2016) 76(22):6747–59. doi:10.1158/0008-5472.CAN-16-1404
53. Barber GN. STING: infection, inflammation and cancer. Nat Rev Immunol (2015) 15(12):760–70. doi:10.1038/nri3921
54. Deng L, Liang H, Xu M, Yang X, Burnette B, Arina A, et al. STING-dependent cytosolic DNA sensing promotes radiation-induced type I interferon-dependent antitumor immunity in immunogenic tumors. Immunity (2014) 41(5):843–52. doi:10.1016/j.immuni.2014.10.019
55. Demaria O, De Gassart A, Coso S, Gestermann N, Di Domizio J, Flatz L, et al. STING activation of tumor endothelial cells initiates spontaneous and therapeutic antitumor immunity. Proc Natl Acad Sci U S A (2015) 112(50):15408–13. doi:10.1073/pnas.1512832112
56. Li T, Cheng H, Yuan H, Xu Q, Shu C, Zhang Y, et al. Antitumor activity of cGAMP via stimulation of cGAS-cGAMP-STING-IRF3 mediated innate immune response. Sci Rep (2016) 6:19049. doi:10.1038/srep19049
57. Ohkuri T, Kosaka A, Ishibashi K, Kumai T, Hirata Y, Ohara K, et al. Intratumoral administration of cGAMP transiently accumulates potent macrophages for anti-tumor immunity at a mouse tumor site. Cancer Immunol Immunother (2017) 66(6):705–16. doi:10.1007/s00262-017-1975-1
58. Diner EJ, Burdette DL, Wilson SC, Monroe KM, Kellenberger CA, Hyodo M, et al. The innate immune DNA sensor cGAS produces a noncanonical cyclic dinucleotide that activates human STING. Cell Rep (2013) 3(5):1355–61. doi:10.1016/j.celrep.2013.05.009
59. Gadkaree SK, Fu J, Sen R, Korrer MJ, Allen C, Kim YJ. Induction of tumor regression by intratumoral STING agonists combined with anti-programmed death-L1 blocking antibody in a preclinical squamous cell carcinoma model. Head Neck (2017) 39(6):1086–94. doi:10.1002/hed.24704
60. Wang H, Hu S, Chen X, Shi H, Chen C, Sun L, et al. cGAS is essential for the antitumor effect of immune checkpoint blockade. Proc Natl Acad Sci U S A (2017) 114(7):1637–42. doi:10.1073/pnas.1621363114
61. Luo M, Wang H, Wang Z, Cai H, Lu Z, Li Y, et al. A STING-activating nanovaccine for cancer immunotherapy. Nat Nanotechnol (2017) 12(7):648–54. doi:10.1038/nnano.2017.52
62. Fu J, Kanne DB, Leong M, Glickman LH, McWhirter SM, Lemmens E, et al. STING agonist formulated cancer vaccines can cure established tumors resistant to PD-1 blockade. Sci Transl Med (2015) 7(283):283ra52. doi:10.1126/scitranslmed.aaa4306
63. Baird JR, Friedman D, Cottam B, Dubensky TW Jr, Kanne DB, Bambina S, et al. Radiotherapy combined with novel STING-targeting oligonucleotides results in regression of established tumors. Cancer Res (2016) 76(1):50–61. doi:10.1158/0008-5472.CAN-14-3619
64. Curran E, Chen X, Corrales L, Kline DE, Dubensky TW Jr, Duttagupta P, et al. STING pathway activation stimulates potent immunity against acute myeloid leukemia. Cell Rep (2016) 15(11):2357–66. doi:10.1016/j.celrep.2016.05.023
65. Tang CH, Zundell JA, Ranatunga S, Lin C, Nefedova Y, Del Valle JR, et al. Agonist-mediated activation of STING induces apoptosis in malignant B cells. Cancer Res (2016) 76(8):2137–52. doi:10.1158/0008-5472.CAN-15-1885
66. Dahal LN, Dou L, Hussain K, Liu R, Earley A, Cox KL, et al. STING activation reverses lymphoma-mediated resistance to antibody immunotherapy. Cancer Res (2017) 77(13):3619–31. doi:10.1158/0008-5472.CAN-16-2784
67. Gulen MF, Koch U, Haag SM, Schuler F, Apetoh L, Villunger A, et al. Signalling strength determines proapoptotic functions of STING. Nat Commun (2017) 8(1):427. doi:10.1038/s41467-017-00573-w
68. Larkin B, Ilyukha V, Sorokin M, Buzdin A, Vannier E, Poltorak A. Cutting edge: activation of STING in T cells induces type I IFN responses and cell death. J Immunol (2017) 199(2):397–402. doi:10.4049/jimmunol.1601999
69. Kato H, Takahasi K, Fujita T. RIG-I-like receptors: cytoplasmic sensors for non-self RNA. Immunol Rev (2011) 243(1):91–8. doi:10.1111/j.1600-065X.2011.01052.x
70. Tschopp J, Thome M, Hofmann K, Meinl E. The fight of viruses against apoptosis. Curr Opin Genet Dev (1998) 8(1):82–7. doi:10.1016/S0959-437X(98)80066-X
71. Hanahan D, Weinberg RA. The hallmarks of cancer. Cell (2000) 100(1):57–70. doi:10.1016/S0092-8674(00)81683-9
72. Roulois D, Loo Yau H, Singhania R, Wang Y, Danesh A, Shen SY, et al. DNA-demethylating agents target colorectal cancer cells by inducing viral mimicry by endogenous transcripts. Cell (2015) 162(5):961–73. doi:10.1016/j.cell.2015.07.056
73. Poeck H, Besch R, Maihoefer C, Renn M, Tormo D, Morskaya SS, et al. 5’-Triphosphate-siRNA: turning gene silencing and Rig-I activation against melanoma. Nat Med (2008) 14(11):1256–63. doi:10.1038/nm.1887
74. Besch R, Poeck H, Hohenauer T, Senft D, Hacker G, Berking C, et al. Proapoptotic signaling induced by RIG-I and MDA-5 results in type I interferon-independent apoptosis in human melanoma cells. J Clin Invest (2009) 119(8):2399–411. doi:10.1172/JCI37155
75. Duewell P, Beller E, Kirchleitner SV, Adunka T, Bourhis H, Siveke J, et al. Targeted activation of melanoma differentiation-associated protein 5 (MDA5) for immunotherapy of pancreatic carcinoma. Oncoimmunology (2015) 4(10):e1029698. doi:10.1080/2162402X.2015.1029698
76. Kubler K, Gehrke N, Riemann S, Bohnert V, Zillinger T, Hartmann E, et al. Targeted activation of RNA helicase retinoic acid-inducible gene-I induces proimmunogenic apoptosis of human ovarian cancer cells. Cancer Res (2010) 70(13):5293–304. doi:10.1158/0008-5472.CAN-10-0825
77. Meng G, Xia M, Xu C, Yuan D, Schnurr M, Wei J. Multifunctional antitumor molecule 5’-triphosphate siRNA combining glutaminase silencing and RIG-I activation. Int J Cancer (2014) 134(8):1958–71. doi:10.1002/ijc.28416
78. Yu X, Wang H, Li X, Guo C, Yuan F, Fisher PB, et al. Activation of the MDA-5-IPS-1 viral sensing pathway induces cancer cell death and type I IFN-dependent antitumor immunity. Cancer Res (2016) 76(8):2166–76. doi:10.1158/0008-5472.CAN-15-2142
79. Hornung V, Ablasser A, Charrel-Dennis M, Bauernfeind F, Horvath G, Caffrey DR, et al. AIM2 recognizes cytosolic dsDNA and forms a caspase-1-activating inflammasome with ASC. Nature (2009) 458(7237):514–8. doi:10.1038/nature07725
80. Schnurr M, Duewell P. Breaking tumor-induced immunosuppression with 5’-triphosphate siRNA silencing TGFbeta and activating RIG-I. Oncoimmunology (2013) 2(5):e24170. doi:10.4161/onci.24170
81. Li D, Gale RP, Liu Y, Lei B, Wang Y, Diao D, et al. 5’-Triphosphate siRNA targeting MDR1 reverses multi-drug resistance and activates RIG-I-induced immune-stimulatory and apoptotic effects against human myeloid leukaemia cells. Leuk Res (2017) 58:23–30. doi:10.1016/j.leukres.2017.03.010
82. Ranoa DR, Parekh AD, Pitroda SP, Huang X, Darga T, Wong AC, et al. Cancer therapies activate RIG-I-like receptor pathway through endogenous non-coding RNAs. Oncotarget (2016) 7(18):26496–515. doi:10.18632/oncotarget.8420
83. Bhoopathi P, Quinn BA, Gui Q, Shen XN, Grossman SR, Das SK, et al. Pancreatic cancer-specific cell death induced in vivo by cytoplasmic-delivered polyinosine-polycytidylic acid. Cancer Res (2014) 74(21):6224–35. doi:10.1158/0008-5472.CAN-14-0819
84. Akasaka T, Tsujii M, Kondo J, Hayashi Y, Ying J, Lu Y, et al. 5FU resistance abrogates the amplified cytotoxic effects induced by inhibiting checkpoint kinase 1 in p53mutated colon cancer cells. Int J Oncol (2015) 46(1):63–70. doi:10.3892/ijo.2014.2693
85. Long A, Richard J, Hawley L, LaPatra SE, Garver KA. Transmission potential of infectious hematopoietic necrosis virus in APEX-IHN(R)-vaccinated Atlantic salmon. Dis Aquat Organ (2017) 122(3):213–21. doi:10.3354/dao03076
86. Davidson AH, Traub-Dargatz JL, Rodeheaver RM, Ostlund EN, Pedersen DD, Moorhead RG, et al. Immunologic responses to West Nile virus in vaccinated and clinically affected horses. J Am Vet Med Assoc (2005) 226(2):240–5. doi:10.2460/javma.2005.226.240
87. Atherton MJ, Morris JS, McDermott MR, Lichty BD. Cancer immunology and canine malignant melanoma: a comparative review. Vet Immunol Immunopathol (2016) 169:15–26. doi:10.1016/j.vetimm.2015.11.003
88. Lauscher A, Krossoy B, Frost P, Grove S, Konig M, Bohlin J, et al. Immune responses in Atlantic salmon (Salmo salar) following protective vaccination against infectious salmon anemia (ISA) and subsequent ISA virus infection. Vaccine (2011) 29(37):6392–401. doi:10.1016/j.vaccine.2011.04.074
89. Kobiyama K, Jounai N, Aoshi T, Tozuka M, Takeshita F, Coban C, et al. Innate immune signaling by, and genetic adjuvants for DNA vaccination. Vaccines (Basel) (2013) 1(3):278–92. doi:10.3390/vaccines1030278
90. Suschak JJ, Wang S, Fitzgerald KA, Lu S. A cGAS-independent STING/IRF7 pathway mediates the immunogenicity of DNA vaccines. J Immunol (2016) 196(1):310–6. doi:10.4049/jimmunol.1501836
91. Coban C, Kobiyama K, Aoshi T, Takeshita F, Horii T, Akira S, et al. Novel strategies to improve DNA vaccine immunogenicity. Curr Gene Ther (2011) 11(6):479–84. doi:10.2174/156652311798192815
92. Iurescia S, Fioretti D, Rinaldi M. Nucleic acid sensing machinery: targeting innate immune system for cancer therapy. Recent Pat Anticancer Drug Discov (2018) 13(1):2–17. doi:10.2174/1574892812666171030163804
93. Lladser A, Mougiakakos D, Tufvesson H, Ligtenberg MA, Quest AF, Kiessling R, et al. DAI (DLM-1/ZBP1) as a genetic adjuvant for DNA vaccines that promotes effective antitumor CTL immunity. Mol Ther (2011) 19(3):594–601. doi:10.1038/mt.2010.268
94. Smith LR, Yang CK, Inventors. Activation of RIG-I Pathway. United States patent US 20130005028 (2013).
95. Chen Q, Boire A, Jin X, Valiente M, Er EE, Lopez-Soto A, et al. Carcinoma-astrocyte gap junctions promote brain metastasis by cGAMP transfer. Nature (2016) 533(7604):493–8. doi:10.1038/nature18268
96. Lemos H, Mohamed E, Huang L, Ou R, Pacholczyk G, Arbab AS, et al. STING promotes the growth of tumors characterized by low antigenicity via IDO activation. Cancer Res (2016) 76(8):2076–81. doi:10.1158/0008-5472.CAN-15-1456
Keywords: DAMPs, STING, RIG-1, innate immune system, cytosolic nucleic acid receptors, antitumor response, agonist, clinical trials
Citation: Iurescia S, Fioretti D and Rinaldi M (2018) Targeting Cytosolic Nucleic Acid-Sensing Pathways for Cancer Immunotherapies. Front. Immunol. 9:711. doi: 10.3389/fimmu.2018.00711
Received: 16 January 2018; Accepted: 22 March 2018;
Published: 09 April 2018
Edited by:
Bénédicte Manoury, Institut National de la Santé et de la Recherche Médicale (INSERM), FranceReviewed by:
Philippe Georgel, Université de Strasbourg, FranceRaymond B. Birge, Rutgers University, The State University of New Jersey, United States
Copyright: © 2018 Iurescia, Fioretti and Rinaldi. This is an open-access article distributed under the terms of the Creative Commons Attribution License (CC BY). The use, distribution or reproduction in other forums is permitted, provided the original author(s) and the copyright owner are credited and that the original publication in this journal is cited, in accordance with accepted academic practice. No use, distribution or reproduction is permitted which does not comply with these terms.
*Correspondence: Sandra Iurescia, c2FuZHJhLml1cmVzY2lhJiN4MDAwNDA7aWZ0LmNuci5pdA==;
Monica Rinaldi, bW9uaWNhLnJpbmFsZGkmI3gwMDA0MDtpZnQuY25yLml0
†These authors have contributed equally to this work and shared first authorship.