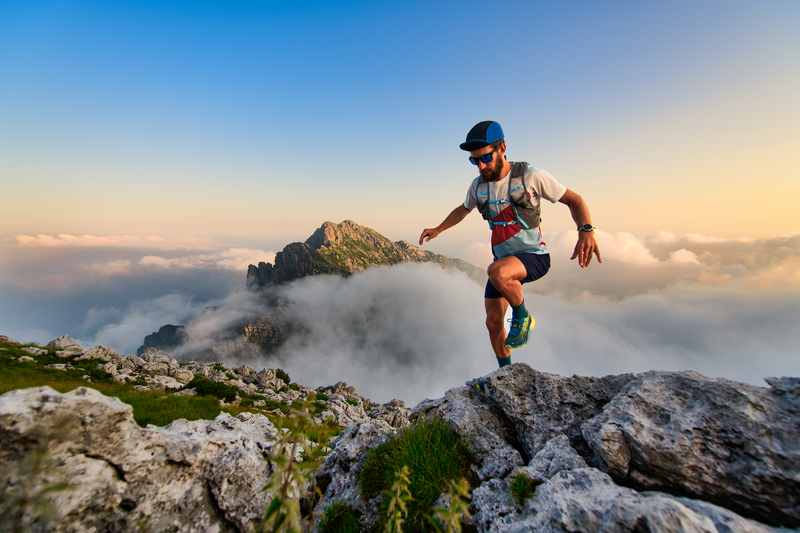
95% of researchers rate our articles as excellent or good
Learn more about the work of our research integrity team to safeguard the quality of each article we publish.
Find out more
MINI REVIEW article
Front. Immunol. , 04 April 2018
Sec. Vaccines and Molecular Therapeutics
Volume 9 - 2018 | https://doi.org/10.3389/fimmu.2018.00649
This article is part of the Research Topic Development of Humanized Mouse Models for Infectious Diseases and Cancer View all 17 articles
Immunodeficient mice transplanted with human cell populations or tissues, also known as human immune system (HIS) mice, have emerged as an important and versatile tool for the in vivo study of human immunodeficiency virus-type 1 (HIV-1) pathogenesis, treatment, and persistence in various biological compartments. Recent work in HIS mice has demonstrated their ability to recapitulate critical aspects of human immune responses to HIV-1 infection, and such studies have informed our knowledge of HIV-1 persistence and latency in the context of combination antiretroviral therapy. The central nervous system (CNS) is a unique, immunologically privileged compartment susceptible to HIV-1 infection, replication, and immune-mediated damage. The unique, neural, and glia-rich cellular composition of this compartment, as well as the important role of infiltrating cells of the myeloid lineage in HIV-1 seeding and replication makes its study of paramount importance, particularly in the context of HIV-1 cure research. Current work on the replication and persistence of HIV-1 in the CNS, as well as cells of the myeloid lineage thought to be important in HIV-1 infection of this compartment, has been aided by the expanded use of these HIS mouse models. In this review, we describe the major HIS mouse models currently in use for the study of HIV-1 neuropathogenesis, recent insights from the field, limitations of the available models, and promising advances in HIS mouse model development.
Infection with human immunodeficiency virus-type 1 (HIV-1) results in CD4+ T cell destruction and progressive debilitation of the immune system (1). Although combination antiretroviral therapy (cART) can effectively suppress HIV-1 RNA to undetectable levels in the peripheral blood (2), the ability of replication-competent HIV-1 to persist in cellular and tissue reservoirs despite suppressive therapy is a barrier to cure (3–5). Penetration of the central nervous system (CNS) by HIV-1 occurs early in infection (6, 7). HIV is postulated to cross the blood–brain barrier (BBB) via the infiltration of infected monocytes, CD4+ T lymphocytes (8, 9), or as cell-free virus (10, 11). Resulting CNS immune activation; the infection and activation of monocytes, perivascular macrophages, and resident microglia; and indirect mechanisms are all thought to play a critical role in the pathogenesis of HIV-1 in the CNS (12–16). Early neuropathological characterization of the CNS in those with advanced untreated HIV-1 and HIV-associated dementia (HAD) revealed encephalitis marked by inflammation, microglial activation, astrogliosis, and neuronal loss (17, 18). Use of highly effective cART has significantly reduced the incidence of HAD (19). Nonetheless, HIV-1-associated neurocognitive disorders (HANDs) persist as an important clinical complication of HIV-1 infection in the cART era and can result in an array of cognitive, behavioral, and motor deficits (20). Murine models that mimic human immune systems (HIS) have been extremely valuable tools for the elucidation of a number of pathophysiological mechanisms responsible for HIV-1 CNS pathogenesis. However, no adjunctive therapies for HAND exist beyond cART, and a combination of novel and more physiologically relevant HIS mouse models is now being evaluated to advance our knowledge of the complex immunological and pathological features of HIV-1 neuropathogenesis in the cART era (21, 22).
Animal models provide an important complementary approach to the study of HIV-1 pathogenesis (23). To varying degrees, these in vivo models replicate the intricacies of complex immunological interactions between multiple cell types to an extent not possible in vitro. In addition, they are free from many of the experimental constraints imposed by the inaccessibility/limited availability of human tissue (24). Commonly used non-human primate (NHP) animal models include rhesus, pigtail, and cynomolgus macaques that can be infected with a simian or chimeric simian/human immunodeficiency virus. NHP models have provided great insight into HIV-1 neuropathogenesis. In particular, rhesus macaques have been shown to develop HIV encephalitis (HIVE) and microglial infection (25), and a highly neurovirulent (although not physiologic) challenge model has been developed in pigtail macaques (26). However, studies using NHPs are limited by high cost, special housing requirements, and small experimental groups. In response to these constraints, small animal models of human disease have been developed and widely employed (27). However, their ability to recapitulate human disease can be limited as some important human pathogens (including HIV) display tropism unique to humans (28, 29). The transgenic expression of select HIV-1 proteins such as HIV-1 envelope and trans-activator of transcription, human receptors and co-receptors in mice result in animals with a broad range of HIV-1-related pathologies (30–34). These include a spectrum of neurotoxicity, defective neurogenesis, and glial abnormalities in mouse CNS that resemble those seen in the brains of HIV-1-infected humans (35–39). Although these transgenic models mirror specific components of the pathophysiological effects of select HIV-1 proteins on the CNS (40), as well as some of the cognitive and behavioral features of HAND (41), they are unable to model critical aspects of HIV-1 CNS infection in the human host, such as viral CNS invasion (42). For these reasons, the use of small animal models that can more accurately mimic the HIS is of great value.
In contrast to transgenic or chimeric mice, the creation of mice with human immune system components (HIS mice) provide an in vivo environment that allows for the study of HIV-1 and its interaction with cells of the human immune system (24). HIS mouse production initiates with the choice of an immunodeficient mouse strain that can accommodate the engraftment of human cells and tissues without rejection (43). Early immunodeficient mice used for human tissue or cell xenografts included “nude” mice, which lack mature CD4+ and CD8+ T cells (44) and severe combined immunodeficiency (SCID) mice, which harbor a mutation in the protein kinase, DNA-activated, catalytic polypeptide gene (Prkdcscid) and lack mature T and B cells (45). The ability of these strains to support long-term, systemic reconstitution with human cells were, however, limited by relatively high residual levels of innate immune responses, such as those mediated by natural killer (NK) cells resulting in the rejection of human bone-marrow allographs (46). Improved levels of immunodeficiency were found in strains lacking mature B and T lymphocytes due to disruptions in the recombination-activating genes Rag1 and Rag2 (47, 48), that were further augmented in mice also harboring a complete null mutation of the common cytokine receptor γ chain (IL2Rγ, or γc), resulting in the absence of mouse NK cells (49–51). As a result, modern HIS mouse models are typically produced by engrafting human hematopoietic stem cells (hHSCs), human peripheral mononuclear cells, and/or human tissues into these highly immunodeficient strains following their preconditioning with sublethal irradiation or chemotherapy. The main platforms in use include NSG (NOD-scid IL2Rγnull and NOD.Cg-PrkdcscidIL-2Rγtm1Wjll/Sz) (52), NRG (NOD-Rag1−/−IL2RγC-null), NOG (NOD.Cg-Prkdcscid IL-2Rγtm1Sug), and BRG (BALB/c-Rag2null IL-2Rγnull) strains (24, 53). Although important differences in the extent of humanization and functional quality of the populating human cells exist between models, multilineage reconstitution with hHSCs can include all major human lymphocyte classes (CD4+ and CD8+ T cells, B cells, and NK cells) as well as various myeloid cells (monocytes, macrophages, and dendritic cells). In those strains of mice that support human T-cell development when transplanted with human CD34+ hHSCs, T cell maturation occurs in the murine thymus (52, 54, 55). When humanized mice are engineered by implanting human thymus and liver tissue, developing T cells are educated on human thymic epithelial cells, allowing for restriction by human leukocyte antigens (HLAs) I and II (56, 57). The bone marrow–liver–thymus (BLT) mouse model, which combines the implantation of fetal liver and thymus under the kidney capsule of NOD/SCID, NSG, or C57BL/6 Rag2−/− IL2γ−/− mice, along with the transplant of autologous CD34+ hHSCs is the most complete and well explored (58–60). The technical demands of this system are associated with considerable expense, and the need to surgically implant each mouse can result in significant variation in HIS repopulation (61). However, with their strong lymph node and intestinal reconstitution, BLT mice are particularly useful for the study of HIV-1 infection at mucosal surfaces (62–64). Modern HIS mouse models provide stable human cellular reconstitution that can support HIV-1 replication in the peripheral blood and multiple organs (27), allowing them to provide insights into many aspects of HIV-1 biology including viral life cycle and innate and adaptive immune responses to HIV-1 (59, 62, 65–68). Viral suppression with clinically relevant cART (69–73) has been demonstrated in HIS mice, and they have proven effective for the investigation of multiple immune-based approaches for the in vivo control of viral replication and elimination of HIV-infected cells (74–78).
Early neuroAIDS mouse models involved the generation of HIVE through the direct injection of human microglia or macrophages into the brain of SCID mice (79, 80). While the resultant SCID-HIVE model recapitulates some of the neuropathological features of human HIVE, these approaches are traumatic and result in xenoreactivity induced-inflammation through the artificial insertion of human cells into a foreign mouse cellular environment (81). Despite these caveats, studies investigating the impact of cART in this model have demonstrated reductions in neuropathological features of HIVE including decreased astro- and micro-gliosis and reductions in HIV-1 brain viral loads (82–84). Subsequent development of the humanized mouse model, in which NSG mice are engrafted with CD34+ hHSCs (CD34+-NSG mice), has allowed for more detailed, prolonged studies of HIV-1 CNS infection and neurodegeneration in the context of unchecked HIV-1 replication (85). Systemic HIV-1 infection in this model is characterized by low CNS viral burdens and the transmigration of HIV-infected human monocytes and macrophages into the mouse CNS. These human cells localize predominantly to the meninges, perivascular spaces, and, to a lesser extent, brain parenchyma (85–88). Regional activation of resident murine microglia and astrocytes, neuroinflammation, and neurodegeneration are also among the salient findings in this model (85, 86, 89). Some of these changes were reversible by long-acting nanoparticle-based cART (90). More recently, pre- and post-infection dosing with a novel sonic hedgehog mimetic was found to increase BBB integrity in acutely infected CD34+-NSG mice, resulting in decreased leukocyte extravasation into CNS during and pathologic evidence of neuroprotection (91). Finally, a simplified HIS model generated by the intraperitoneal (IP) injection of human PBMCs into non-irradiated NSG mice (NSG-huPBL) has recently been described (92). In this model, IP challenge with HIV-1 resulted in systemic viral infection and CNS invasion with infected CD4+ T cells. The presence of neuropathology—characterized by neurodegeneration, activated microglia, and astrocytes—was found to be dependent on the infecting viral strain (93). A brief summary of currently available HIS mouse models with published data on HIV-1 CNS infection can be found in Table 1.
Table 1. HIS mouse models with published studies of human immunodeficiency virus-type 1 (HIV-1) infection of the central nervous system (CNS).
Monocytes and macrophages can be infected with HIV-1 both in vitro and in vivo (94–96). However, the question of whether cells of myeloid lineage serve as true HIV-1 reservoirs in the context of suppressive cART remains of great interest (97). This question is central to the study of HIV-1 persistence in the CNS as perivascular monocyte-derived macrophages and parenchymal microglia are the most important cellular targets of HIV-1 in the CNS (98), and infection of these cell types is critical to HIV-1 CNS pathogenesis and HAND (99). Recent evidence suggesting that macrophages may become positive for viral DNA through the capture and phagocytosis of infected CD4+ T cells implies a mechanism of infection distinct from virological synapse formation and furthers the debate (100, 101). Recent study in the T cell only mouse in which implantation of autologous human fetal liver and thymus under the kidney capsule of an NSG mouse results in systemic reconstitution almost exclusively with human T cells predictably demonstrates the development of latent T cell reservoirs of HIV-1 (102). Complementary studies by Honeycutt et al. in myeloid-only mice (MoM) in which NOD/SCID mice transplanted with CD34+ hHSCs are reconstituted with human myeloid and B cells in the absence of human T cells have proven informative. Using this novel HIS model, Honeycutt et al. demonstrated that macrophages can support efficient HIV-1 replication in vivo in multiple compartments in the absence of T cells following infection with certain macrophage-tropic (M-tropic) HIV-1 strains such as HIV-1 ADA. HIV-1 DNA and RNA as well as macrophages expressing HIV-1 p24 were detected in the brains of infected MoM (60). In addition, cessation of suppressive cART in MoM resulted in measurable in vivo viral rebound after 7 weeks (103) supporting infection of long-lived tissue macrophage populations (104). Another recent study in CD34+-NSG mice infected with M-tropic HIV-1 found evidence for CD14+CD16+ monocyte/macrophage cells with HIV-1 RNA and integrated proviral DNA in the spleen and bone marrow. Consistent with previous reports in this model, viral RNA was detected in the brains in a few animals at low copy numbers (105). As a result, HIS mouse models have proven utility in defining cellular sites for HIV-1 infection and hold promise for further elucidating the viral dynamics of the establishment and recrudescence of potential CNS-based HIV-1 reservoirs.
Although they represent powerful research tools, limitations to the use of HIS mice for the in vivo study of HIV-1 exist. HIS models do not perfectly recapitulate human hematopoiesis and can display a relatively short lifespan, particularly after the approximately 8- to 18-week period needed for appropriate engraftment (43). Variability in the efficiency of human cell engraftment is an important challenge to robust experimentation. In addition, despite the fact that most HIS mouse models have demonstrated highly effective adaptive T-cell immune responses, the majority of models display an absence of species-specific human cytokines and impaired B-cell function and humoral immune responses (55, 106–108). This is important, as one proposed mechanism for the pathology induced in the CNS in response to HIV-1 infection is an abnormal cytokine/chemokine response (16). Another important limitation of currently available HIS mouse models is the frequent development of graft-versus-host disease (GVHD), characterized by multiorgan lymphocytic infiltration and sclerosis in the weeks following hHSC transplant (109). This is an important limitation to the study of HIV-1 in the CNS in particular, as longer-term experiments are necessary to demonstrate productive infection of the CNS by HIV-1 and CNS pathology in animals naïve to and under cART and/or putative adjunct therapeutics. Several research groups are working to improve the functionality of HIS mouse models in response to these limitations. Lavender et al. have described the evaluation of GVHD-resistant triple knockout (TKO) mice, which lack CD47 in addition to Rag 1 and IL2rg. These TKO-BLT mice reportedly remained healthy for 45 weeks post-humanization and could be virally suppressed on cART (110). Additional efforts to improve HIS mouse platforms have included the depletion of endogenous mouse macrophages (111) and the development of strains expressing human cytokines for improved human NK-cell development (112, 113). HIS models with improved development of HLA-restricted human T cells have been achieved through engraftment of HLA-matched hHSC into immunodeficient mice with transgenic expression of human HLA molecules (114). Huang et al. have reported the development of a novel HIS mouse model utilizing recombinant adeno-associated virus-based gene transfer technologies (115) to introduce genes encoding HLA-A2/DR and selected human cytokines into NSG mice. The ability of this resultant HIS mouse model to endogenously encode for human MHC constitutively during its lifespan and key human cytokines during development of lymphoid and myeloid progenitor cells allows for an accurate recapitulation of many aspects of the human immune system. This is reflected in highly functional human CD4+ and CD8+T-cell and B-cell responses (116, 117) as well as the successful reconstitution of human monocytes (CD14+) and macrophages (CD14+/CD11b+) (117). These HIS mice can be productively infected with HIV-1 (118) and have the ability to secrete measurable human IFN-γ, IL-2, CCL3, and IL-1β in vivo in response to parasitic and viral pathogens (117–120). With high rates of engraftment and low rates of GVHD, this model can be a useful tool for the study of potentially important viral reservoirs of HIV-1 in the CNS. In a similar vein, Kim et al. have recently reported the use of immunodeficient mice expressing HLA class II (DR4) (DRAG mice) engrafted with HLA-matched hHSCs to study early HIV-1 infection. The authors report HIV-1 replication in various tissues, including bone marrow, lymph nodes, and the brain, which on day 21 following mucosal infection, was the last tissue examined to become HIV-1 viral RNA positive (121). Finally, infiltrating human myeloid cells and lymphocytes have been demonstrated in the brains of HIV-1 infected HIS mice (85). However, the generation of models harboring functional human myeloid cells in percentages approximating those seen in humans has been challenging. In several HIS platforms, strategies to improve human myeloid cell reconstitution include the administration of exogenous human Flt3 ligand (122), exogenous delivery of human granulocyte-macrophage colony-stimulating factor (GM-CSF) and IL-4 (123, 124), and human GM-CSF and IL-3 knock-in (125, 126).
Limitations of HIS mouse models that are of unique interest to the study of HIV-1 in the CNS exist as well. Common to all HIS mouse models is the absence of human microglia in the CNS (127)—a major deficiency as microglia represent one of the most important cellular targets of HIV-1 in the brain (98). Unfortunately, engrafted CD34+ hHSCs are unlikely to repopulate human microglial cells within the brains of HIS mice, as microglial cells are derived early during development from yolk sack precursors (127). Additionally, human glia (astrocytes and oligodendrocytes)—the most abundant cell types in the human CNS—are absent in the majority of HIS mouse models (128). As a result, these platforms are unable to recapitulate innate glial cell responses resulting from the complex interactions between human glia and infected mononuclear phagocytes during progressive HIV-1 infection (129, 130). In response, several groups have attempted to reconstitute HIS mouse brain with neonatally transplanted human glial progenitor cells (131, 132). Following such interventions, Li et al. reported the detection of human glia in diverse brain regions of HIS mice including periventricular areas, white matter tracts, and brain stem. RNA-sequencing in the selected brain regions of such mice infected with M-tropic HIV-1 reportedly display glial transcriptional signatures and viral defense signaling pathways that mirror human disease (133–136). Although this approach does not repopulate the brain with human microglia, such experimental improvements are welcome and will allow for the improved modeling of human HIV-1 CNS neuropathological disease.
Human immune system (HIS) mouse models have proven to be extremely valuable tools for the study of HIV-1 infection of the CNS, its resulting neuropathology and the potential for HIV-1 persistence in this immunologically privileged compartment. As with all model systems, experimental and biologic limitations exist. These include the absence of human CNS cell types that in response to HIV-1 invasion play key roles in the development of the neuroinflammatory milieu and impaired immune, glial, and neural cell functions leading to HAND. However, model improvements are ongoing, with the general aims of preventing GVHD and enhancing the levels, reproducibility, and quality of human immune cell reconstitution. The rational evolution of these models will continue to foster authentic human immune responses in HIS mouse models and will further facilitate development of diagnostic, novel therapeutic, and viral eradication strategies for HIV-1 in the CNS.
TE and MT contributed to the conception, writing, and discussion of this review manuscript. TE wrote the initial draft of the manuscript. The final version of the manuscript was approved by both authors.
The authors declare that the research was conducted in the absence of any commercial or financial relationships that could be construed as a potential conflict of interest.
This work was supported by the Mark S. Bertuch AIDS Research Fund (#554400), Leidos, Inc. (P010148091 and P010173450) and The Rockefeller University Bernard L. Schwartz Program for Physician Scientists.
1. Gottlieb MS, Schroff R, Schanker HM, Weisman JD, Fan PT, Wolf RA, et al. Pneumocystis carinii pneumonia and mucosal candidiasis in previously healthy homosexual men: evidence of a new acquired cellular immunodeficiency. N Engl J Med (1981) 305(24):1425–31. doi:10.1056/NEJM198112103052401
3. Chun TW, Stuyver L, Mizell SB, Ehler LA, Mican JA, Baseler M, et al. Presence of an inducible HIV-1 latent reservoir during highly active antiretroviral therapy. Proc Natl Acad Sci U S A (1997) 94(24):13193–7. doi:10.1073/pnas.94.24.13193
4. Finzi D, Hermankova M, Pierson T, Carruth LM, Buck C, Chaisson RE, et al. Identification of a reservoir for HIV-1 in patients on highly active antiretroviral therapy. Science (1997) 278(5341):1295–300. doi:10.1126/science.278.5341.1295
5. Wong JK, Hezareh M, Gunthard HF, Havlir DV, Ignacio CC, Spina CA, et al. Recovery of replication-competent HIV despite prolonged suppression of plasma viremia. Science (1997) 278(5341):1291–5. doi:10.1126/science.278.5341.1291
6. Pilcher CD, Shugars DC, Fiscus SA, Miller WC, Menezes P, Giner J, et al. HIV in body fluids during primary HIV infection: implications for pathogenesis, treatment and public health. AIDS (2001) 15(7):837–45. doi:10.1097/00002030-200105040-00004
7. Schacker T, Collier AC, Hughes J, Shea T, Corey L. Clinical and epidemiologic features of primary HIV infection. Ann Intern Med (1996) 125(4):257–64. doi:10.7326/0003-4819-125-4-199608150-00001
8. Haase AT. Pathogenesis of lentivirus infections. Nature (1986) 322(6075):130–6. doi:10.1038/322130a0
9. Dahiya S, Irish BP, Nonnemacher MR, Wigdahl B. Genetic variation and HIV-associated neurologic disease. Adv Virus Res (2013) 87:183–240. doi:10.1016/B978-0-12-407698-3.00006-5
10. Collman R, Balliet JW, Gregory SA, Friedman H, Kolson DL, Nathanson N, et al. An infectious molecular clone of an unusual macrophage-tropic and highly cytopathic strain of human immunodeficiency virus type 1. J Virol (1992) 66(12):7517–21.
11. Spudich S, Gonzalez-Scarano F. HIV-1-related central nervous system disease: current issues in pathogenesis, diagnosis, and treatment. Cold Spring Harb Perspect Med (2012) 2(6):a007120. doi:10.1101/cshperspect.a007120
12. Koenig S, Gendelman HE, Orenstein JM, Dal Canto MC, Pezeshkpour GH, Yungbluth M, et al. Detection of AIDS virus in macrophages in brain tissue from AIDS patients with encephalopathy. Science (1986) 233(4768):1089–93. doi:10.1126/science.3016903
13. Hagberg L, Fuchs D, Rosengren L, Gisslen M. Intrathecal immune activation is associated with cerebrospinal fluid markers of neuronal destruction in AIDS patients. J Neuroimmunol (2000) 102(1):51–5. doi:10.1016/S0165-5728(99)00150-2
14. Williams KC, Hickey WF. Central nervous system damage, monocytes and macrophages, and neurological disorders in AIDS. Annu Rev Neurosci (2002) 25:537–62. doi:10.1146/annurev.neuro.25.112701.142822
15. Garden GA. Microglia in human immunodeficiency virus-associated neurodegeneration. Glia (2002) 40(2):240–51. doi:10.1002/glia.10155
16. Kaul M, Garden GA, Lipton SA. Pathways to neuronal injury and apoptosis in HIV-associated dementia. Nature (2001) 410(6831):988–94. doi:10.1038/35073667
17. Budka H. Multinucleated giant cells in brain: a hallmark of the acquired immune deficiency syndrome (AIDS). Acta Neuropathol (1986) 69(3–4):253–8. doi:10.1007/BF00688301
18. Petito CK, Cho ES, Lemann W, Navia BA, Price RW. Neuropathology of acquired immunodeficiency syndrome (AIDS): an autopsy review. J Neuropathol zExp Neurol (1986) 45(6):635–46. doi:10.1097/00005072-198611000-00003
19. McArthur JC. HIV dementia: an evolving disease. J Neuroimmunol (2004) 157(1–2):3–10. doi:10.1016/j.jneuroim.2004.08.042
20. Heaton RK, Franklin DR, Ellis RJ, McCutchan JA, Letendre SL, Leblanc S, et al. HIV-associated neurocognitive disorders before and during the era of combination antiretroviral therapy: differences in rates, nature, and predictors. J Neurovirol (2011) 17(1):3–16. doi:10.1007/s13365-010-0006-1
21. Jaeger LB, Nath A. Modeling HIV-associated neurocognitive disorders in mice: new approaches in the changing face of HIV neuropathogenesis. Dis Model Mech (2012) 5(3):313–22. doi:10.1242/dmm.008763
22. Gelman BB, Endsley J, Kolson D. When do models of NeuroAIDS faithfully imitate “the real thing”? J Neurovirol (2017). doi:10.1007/s13365-017-0601-5
23. Hatziioannou T, Evans DT. Animal models for HIV/AIDS research. Nat Rev Microbiol (2012) 10(12):852–67. doi:10.1038/nrmicro2911
24. Marsden MD, Zack JA. Humanized mouse models for human immunodeficiency virus infection. Annu Rev Virol (2017) 4(1):393–412. doi:10.1146/annurev-virology-101416-041703
25. Harbison C, Zhuang K, Gettie A, Blanchard J, Knight H, Didier P, et al. Giant cell encephalitis and microglial infection with mucosally transmitted simian-human immunodeficiency virus SHIVSF162P3N in rhesus macaques. J Neurovirol (2014) 20(1):62–72. doi:10.1007/s13365-013-0229-z
26. Zink MC, Amedee AM, Mankowski JL, Craig L, Didier P, Carter DL, et al. Pathogenesis of SIV encephalitis. Selection and replication of neurovirulent SIV. Am J Pathol (1997) 151(3):793–803.
27. Masse-Ranson G, Mouquet H, Di Santo JP. Humanized mouse models to study pathophysiology and treatment of HIV infection. Curr Opin HIV AIDS (2018) 13(2):143–51. doi:10.1097/COH.0000000000000440
28. Shibata R, Kawamura M, Sakai H, Hayami M, Ishimoto A, Adachi A. Generation of a chimeric human and simian immunodeficiency virus infectious to monkey peripheral blood mononuclear cells. J Virol (1991) 65(7):3514–20.
29. Morrow WJ, Wharton M, Lau D, Levy JA. Small animals are not susceptible to human immunodeficiency virus infection. J Gen Virol (1987) 68(Pt 8):2253–7. doi:10.1099/0022-1317-68-8-2253
30. Kopp JB, Klotman ME, Adler SH, Bruggeman LA, Dickie P, Marinos NJ, et al. Progressive glomerulosclerosis and enhanced renal accumulation of basement membrane components in mice transgenic for human immunodeficiency virus type 1 genes. Proc Natl Acad Sci U S A (1992) 89(5):1577–81. doi:10.1073/pnas.89.5.1577
31. Browning J, Horner JW, Pettoello-Mantovani M, Raker C, Yurasov S, DePinho RA, et al. Mice transgenic for human CD4 and CCR5 are susceptible to HIV infection. Proc Natl Acad Sci U S A (1997) 94(26):14637–41. doi:10.1073/pnas.94.26.14637
32. Hanna Z, Kay DG, Rebai N, Guimond A, Jothy S, Jolicoeur P. Nef harbors a major determinant of pathogenicity for an AIDS-like disease induced by HIV-1 in transgenic mice. Cell (1998) 95(2):163–75. doi:10.1016/S0092-8674(00)81748-1
33. Leonard JM, Abramczuk JW, Pezen DS, Rutledge R, Belcher JH, Hakim F, et al. Development of disease and virus recovery in transgenic mice containing HIV proviral DNA. Science (1988) 242(4886):1665–70. doi:10.1126/science.3201255
34. Seay K, Qi X, Zheng JH, Zhang C, Chen K, Dutta M, et al. Mice transgenic for CD4-specific human CD4, CCR5 and cyclin T1 expression: a new model for investigating HIV-1 transmission and treatment efficacy. PLoS One (2013) 8(5):e63537. doi:10.1371/journal.pone.0063537
35. Toggas SM, Masliah E, Rockenstein EM, Rall GF, Abraham CR, Mucke L. Central nervous system damage produced by expression of the HIV-1 coat protein gp120 in transgenic mice. Nature (1994) 367(6459):188–93. doi:10.1038/367188a0
36. Kim BO, Liu Y, Ruan Y, Xu ZC, Schantz L, He JJ. Neuropathologies in transgenic mice expressing human immunodeficiency virus type 1 Tat protein under the regulation of the astrocyte-specific glial fibrillary acidic protein promoter and doxycycline. Am J Pathol (2003) 162(5):1693–707. doi:10.1016/S0002-9440(10)64304-0
37. Potash MJ, Chao W, Bentsman G, Paris N, Saini M, Nitkiewicz J, et al. A mouse model for study of systemic HIV-1 infection, antiviral immune responses, and neuroinvasiveness. Proc Natl Acad Sci U S A (2005) 102(10):3760–5. doi:10.1073/pnas.0500649102
38. Okamoto S, Kang YJ, Brechtel CW, Siviglia E, Russo R, Clemente A, et al. HIV/gp120 decreases adult neural progenitor cell proliferation via checkpoint kinase-mediated cell-cycle withdrawal and G1 arrest. Cell Stem Cell (2007) 1(2):230–6. doi:10.1016/j.stem.2007.07.010
39. Lee MH, Wang T, Jang MH, Steiner J, Haughey N, Ming GL, et al. Rescue of adult hippocampal neurogenesis in a mouse model of HIV neurologic disease. Neurobiol Dis (2011) 41(3):678–87. doi:10.1016/j.nbd.2010.12.002
40. Mucke L, Masliah E, Campbell IL. Transgenic models to assess the neuropathogenic potential of HIV-1 proteins and cytokines. Curr Top Microbiol Immunol (1995) 202:187–205.
41. Fitting S, Ignatowska-Jankowska BM, Bull C, Skoff RP, Lichtman AH, Wise LE, et al. Synaptic dysfunction in the hippocampus accompanies learning and memory deficits in human immunodeficiency virus type-1 Tat transgenic mice. Biol Psychiatry (2013) 73(5):443–53. doi:10.1016/j.biopsych.2012.09.026
42. Saylor D, Dickens AM, Sacktor N, Haughey N, Slusher B, Pletnikov M, et al. HIV-associated neurocognitive disorder – pathogenesis and prospects for treatment. Nat Rev Neurol (2016) 12(4):234–48. doi:10.1038/nrneurol.2016.27
43. Denton PW, Sogaard OS, Tolstrup M. Using animal models to overcome temporal, spatial and combinatorial challenges in HIV persistence research. J Transl Med (2016) 14:44. doi:10.1186/s12967-016-0807-y
44. Flanagan SP. ’Nude’, a new hairless gene with pleiotropic effects in the mouse. Genet Res (1966) 8(3):295–309. doi:10.1017/S0016672300010168
45. Bosma GC, Custer RP, Bosma MJ. A severe combined immunodeficiency mutation in the mouse. Nature (1983) 301(5900):527–30. doi:10.1038/301527a0
46. Murphy WJ, Kumar V, Bennett M. Rejection of bone marrow allografts by mice with severe combined immune deficiency (SCID). Evidence that natural killer cells can mediate the specificity of marrow graft rejection. J Exp Med (1987) 165(4):1212–7. doi:10.1084/jem.165.4.1212
47. Mombaerts P, Iacomini J, Johnson RS, Herrup K, Tonegawa S, Papaioannou VE. RAG-1-deficient mice have no mature B and T lymphocytes. Cell (1992) 68(5):869–77. doi:10.1016/0092-8674(92)90030-G
48. Shinkai Y, Rathbun G, Lam KP, Oltz EM, Stewart V, Mendelsohn M, et al. RAG-2-deficient mice lack mature lymphocytes owing to inability to initiate V(D)J rearrangement. Cell (1992) 68(5):855–67. doi:10.1016/0092-8674(92)90029-C
49. Cao X, Shores EW, Hu-Li J, Anver MR, Kelsall BL, Russell SM, et al. Defective lymphoid development in mice lacking expression of the common cytokine receptor gamma chain. Immunity (1995) 2(3):223–38. doi:10.1016/1074-7613(95)90047-0
50. DiSanto JP, Muller W, Guy-Grand D, Fischer A, Rajewsky K. Lymphoid development in mice with a targeted deletion of the interleukin 2 receptor gamma chain. Proc Natl Acad Sci U S A (1995) 92(2):377–81. doi:10.1073/pnas.92.2.377
51. Ohbo K, Suda T, Hashiyama M, Mantani A, Ikebe M, Miyakawa K, et al. Modulation of hematopoiesis in mice with a truncated mutant of the interleukin-2 receptor gamma chain. Blood (1996) 87(3):956–67.
52. Ishikawa F, Yasukawa M, Lyons B, Yoshida S, Miyamoto T, Yoshimoto G, et al. Development of functional human blood and immune systems in NOD/SCID/IL2 receptor {gamma} chain(null) mice. Blood (2005) 106(5):1565–73. doi:10.1182/blood-2005-02-0516
53. Shultz LD, Brehm MA, Garcia-Martinez JV, Greiner DL. Humanized mice for immune system investigation: progress, promise and challenges. Nat Rev Immunol (2012) 12(11):786–98. doi:10.1038/nri3311
54. Shultz LD, Lyons BL, Burzenski LM, Gott B, Chen X, Chaleff S, et al. Human lymphoid and myeloid cell development in NOD/LtSz-scid IL2R gamma null mice engrafted with mobilized human hemopoietic stem cells. J Immunol (2005) 174(10):6477–89. doi:10.4049/jimmunol.174.10.6477
55. Traggiai E, Chicha L, Mazzucchelli L, Bronz L, Piffaretti JC, Lanzavecchia A, et al. Development of a human adaptive immune system in cord blood cell-transplanted mice. Science (2004) 304(5667):104–7. doi:10.1126/science.1093933
56. Olesen R, Wahl A, Denton PW, Garcia JV. Immune reconstitution of the female reproductive tract of humanized BLT mice and their susceptibility to human immunodeficiency virus infection. J Reprod Immunol (2011) 88(2):195–203. doi:10.1016/j.jri.2010.11.005
57. Wege AK, Melkus MW, Denton PW, Estes JD, Garcia JV. Functional and phenotypic characterization of the humanized BLT mouse model. Curr Top Microbiol Immunol (2008) 324:149–65. doi:10.1007/978-3-540-75647-7_10
58. Lan P, Tonomura N, Shimizu A, Wang S, Yang YG. Reconstitution of a functional human immune system in immunodeficient mice through combined human fetal thymus/liver and CD34+ cell transplantation. Blood (2006) 108(2):487–92. doi:10.1182/blood-2005-11-4388
59. Melkus MW, Estes JD, Padgett-Thomas A, Gatlin J, Denton PW, Othieno FA, et al. Humanized mice mount specific adaptive and innate immune responses to EBV and TSST-1. Nat Med (2006) 12(11):1316–22. doi:10.1038/nm1431
60. Honeycutt JB, Wahl A, Baker C, Spagnuolo RA, Foster J, Zakharova O, et al. Macrophages sustain HIV replication in vivo independently of T cells. J Clin Invest (2016) 126(4):1353–66. doi:10.1172/JCI84456
61. Nixon CC, Mavigner M, Silvestri G, Garcia JV. In vivo models of human immunodeficiency virus persistence and cure strategies. J Infect Dis (2017) 215(Suppl_3):S142–51. doi:10.1093/infdis/jiw637
62. Sun Z, Denton PW, Estes JD, Othieno FA, Wei BL, Wege AK, et al. Intrarectal transmission, systemic infection, and CD4+ T cell depletion in humanized mice infected with HIV-1. J Exp Med (2007) 204(4):705–14. doi:10.1084/jem.20062411
63. Denton PW, Estes JD, Sun Z, Othieno FA, Wei BL, Wege AK, et al. Antiretroviral pre-exposure prophylaxis prevents vaginal transmission of HIV-1 in humanized BLT mice. PLoS Med (2008) 5(1):e16. doi:10.1371/journal.pmed.0050016
64. Wahl A, Swanson MD, Nochi T, Olesen R, Denton PW, Chateau M, et al. Human breast milk and antiretrovirals dramatically reduce oral HIV-1 transmission in BLT humanized mice. PLoS Pathog (2012) 8(6):e1002732. doi:10.1371/journal.ppat.1002732
65. Gorantla S, Sneller H, Walters L, Sharp JG, Pirruccello SJ, West JT, et al. Human immunodeficiency virus type 1 pathobiology studied in humanized BALB/c-Rag2-/-gammac-/- mice. J Virol (2007) 81(6):2700–12. doi:10.1128/JVI.02010-06
66. Brainard DM, Seung E, Frahm N, Cariappa A, Bailey CC, Hart WK, et al. Induction of robust cellular and humoral virus-specific adaptive immune responses in human immunodeficiency virus-infected humanized BLT mice. J Virol (2009) 83(14):7305–21. doi:10.1128/JVI.02207-08
67. Dudek TE, No DC, Seung E, Vrbanac VD, Fadda L, Bhoumik P, et al. Rapid evolution of HIV-1 to functional CD8(+) T cell responses in humanized BLT mice. Sci Transl Med (2012) 4(143):143ra98. doi:10.1126/scitranslmed.3003984
68. Rongvaux A, Takizawa H, Strowig T, Willinger T, Eynon EE, Flavell RA, et al. Human hemato-lymphoid system mice: current use and future potential for medicine. Annu Rev Immunol (2013) 31:635–74. doi:10.1146/annurev-immunol-032712-095921
69. Denton PW, Krisko JF, Powell DA, Mathias M, Kwak YT, Martinez-Torres F, et al. Systemic administration of antiretrovirals prior to exposure prevents rectal and intravenous HIV-1 transmission in humanized BLT mice. PLoS One (2010) 5(1):e8829. doi:10.1371/journal.pone.0008829
70. Denton PW, Olesen R, Choudhary SK, Archin NM, Wahl A, Swanson MD, et al. Generation of HIV latency in humanized BLT mice. J Virol (2012) 86(1):630–4. doi:10.1128/JVI.06120-11
71. Horwitz JA, Halper-Stromberg A, Mouquet H, Gitlin AD, Tretiakova A, Eisenreich TR, et al. HIV-1 suppression and durable control by combining single broadly neutralizing antibodies and antiretroviral drugs in humanized mice. Proc Natl Acad Sci U S A (2013) 110(41):16538–43. doi:10.1073/pnas.1315295110
72. Kovarova M, Council OD, Date AA, Long JM, Nochi T, Belshan M, et al. Nanoformulations of rilpivirine for topical pericoital and systemic coitus-independent administration efficiently prevent HIV transmission. PLoS Pathog (2015) 11(8):e1005075. doi:10.1371/journal.ppat.1005075
73. Nischang M, Sutmuller R, Gers-Huber G, Audige A, Li D, Rochat MA, et al. Humanized mice recapitulate key features of HIV-1 infection: a novel concept using long-acting anti-retroviral drugs for treating HIV-1. PLoS One (2012) 7(6):e38853. doi:10.1371/journal.pone.0038853
74. Sango K, Joseph A, Patel M, Osiecki K, Dutta M, Goldstein H. Highly active antiretroviral therapy potently suppresses HIV infection in humanized Rag2-/-gammac-/- mice. AIDS Res Hum Retroviruses (2010) 26(7):735–46. doi:10.1089/aid.2009.0136
75. Diskin R, Klein F, Horwitz JA, Halper-Stromberg A, Sather DN, Marcovecchio PM, et al. Restricting HIV-1 pathways for escape using rationally designed anti-HIV-1 antibodies. J Exp Med (2013) 210(6):1235–49. doi:10.1084/jem.20130221
76. Denton PW, Long JM, Wietgrefe SW, Sykes C, Spagnuolo RA, Snyder OD, et al. Targeted cytotoxic therapy kills persisting HIV infected cells during ART. PLoS Pathog (2014) 10(1):e1003872. doi:10.1371/journal.ppat.1003872
77. Halper-Stromberg A, Lu CL, Klein F, Horwitz JA, Bournazos S, Nogueira L, et al. Broadly neutralizing antibodies and viral inducers decrease rebound from HIV-1 latent reservoirs in humanized mice. Cell (2014) 158(5):989–99. doi:10.1016/j.cell.2014.07.043
78. Klein F, Halper-Stromberg A, Horwitz JA, Gruell H, Scheid JF, Bournazos S, et al. HIV therapy by a combination of broadly neutralizing antibodies in humanized mice. Nature (2012) 492(7427):118–22. doi:10.1038/nature11604
79. Persidsky Y, Limoges J, McComb R, Bock P, Baldwin T, Tyor W, et al. Human immunodeficiency virus encephalitis in SCID mice. Am J Pathol (1996) 149(3):1027–53.
80. Poluektova LY, Munn DH, Persidsky Y, Gendelman HE. Generation of cytotoxic T cells against virus-infected human brain macrophages in a murine model of HIV-1 encephalitis. J Immunol (2002) 168(8):3941–9. doi:10.4049/jimmunol.168.8.3941
81. Persidsky Y, Buttini M, Limoges J, Bock P, Gendelman HE. An analysis of HIV-1-associated inflammatory products in brain tissue of humans and SCID mice with HIV-1 encephalitis. J Neurovirol (1997) 3(6):401–16. doi:10.3109/13550289709031186
82. Cook JE, Dasgupta S, Middaugh LD, Terry EC, Gorry PR, Wesselingh SL, et al. Highly active antiretroviral therapy and human immunodeficiency virus encephalitis. Ann Neurol (2005) 57(6):795–803. doi:10.1002/ana.20479
83. Cook-Easterwood J, Middaugh LD, Griffin WC III, Khan I, Tyor WR. Highly active antiretroviral therapy of cognitive dysfunction and neuronal abnormalities in SCID mice with HIV encephalitis. Exp Neurol (2007) 205(2):506–12. doi:10.1016/j.expneurol.2007.03.007
84. Koneru R, Olive MF, Tyor WR. Combined antiretroviral therapy reduces brain viral load and pathological features of HIV encephalitis in a mouse model. J Neurovirol (2014) 20(1):9–17. doi:10.1007/s13365-013-0223-5
85. Gorantla S, Makarov E, Finke-Dwyer J, Castanedo A, Holguin A, Gebhart CL, et al. Links between progressive HIV-1 infection of humanized mice and viral neuropathogenesis. Am J Pathol (2010) 177(6):2938–49. doi:10.2353/ajpath.2010.100536
86. Boska MD, Dash PK, Knibbe J, Epstein AA, Akhter SP, Fields N, et al. Associations between brain microstructures, metabolites, and cognitive deficits during chronic HIV-1 infection of humanized mice. Mol Neurodegener (2014) 9:58. doi:10.1186/1750-1326-9-58
87. Marsden MD, Zack JA. Studies of retroviral infection in humanized mice. Virology (2015) 479-480:297–309. doi:10.1016/j.virol.2015.01.017
88. Akkina R, Allam A, Balazs AB, Blankson JN, Burnett JC, Casares S, et al. Improvements and limitations of humanized mouse models for HIV research: NIH/NIAID “meet the experts” 2015 workshop summary. AIDS Res Hum Retroviruses (2016) 32(2):109–19. doi:10.1089/AID.2015.0258
89. Dash PK, Gorantla S, Gendelman HE, Knibbe J, Casale GP, Makarov E, et al. Loss of neuronal integrity during progressive HIV-1 infection of humanized mice. J Neurosci (2011) 31(9):3148–57. doi:10.1523/JNEUROSCI.5473-10.2011
90. Dash PK, Gendelman HE, Roy U, Balkundi S, Alnouti Y, Mosley RL, et al. Long-acting nanoformulated antiretroviral therapy elicits potent antiretroviral and neuroprotective responses in HIV-1-infected humanized mice. AIDS (2012) 26(17):2135–44. doi:10.1097/QAD.0b013e328357f5ad
91. Singh VB, Singh MV, Piekna-Przybylska D, Gorantla S, Poluektova LY, Maggirwar SB. Sonic hedgehog mimetic prevents leukocyte infiltration into the CNS during acute HIV infection. Sci Rep (2017) 7(1):9578. doi:10.1038/s41598-017-10241-0
92. Kim KC, Choi BS, Kim KC, Park KH, Lee HJ, Cho YK, et al. A simple mouse model for the study of human immunodeficiency virus. AIDS Res Hum Retroviruses (2016) 32(2):194–202. doi:10.1089/AID.2015.0211
93. Wu X, Liu L, Cheung KW, Wang H, Lu X, Cheung AK, et al. Brain invasion by CD4(+) T cells infected with a transmitted/founder HIV-1BJZS7 during acute stage in humanized mice. J Neuroimmune Pharmacol (2016) 11(3):572–83. doi:10.1007/s11481-016-9654-0
94. Embretson J, Zupancic M, Ribas JL, Burke A, Racz P, Tenner-Racz K, et al. Massive covert infection of helper T lymphocytes and macrophages by HIV during the incubation period of AIDS. Nature (1993) 362(6418):359–62. doi:10.1038/362359a0
95. Gendelman HE, Orenstein JM, Martin MA, Ferrua C, Mitra R, Phipps T, et al. Efficient isolation and propagation of human immunodeficiency virus on recombinant colony-stimulating factor 1-treated monocytes. J Exp Med (1988) 167(4):1428–41. doi:10.1084/jem.167.4.1428
96. Ho DD, Rota TR, Hirsch MS. Infection of monocyte/macrophages by human T lymphotropic virus type III. J Clin Invest (1986) 77(5):1712–5. doi:10.1172/JCI112491
97. Merino KM, Allers C, Didier ES, Kuroda MJ. Role of monocyte/macrophages during HIV/SIV infection in adult and pediatric acquired immune deficiency syndrome. Front Immunol (2017) 8:1693. doi:10.3389/fimmu.2017.01693
98. Persidsky Y, Poluektova L. Immune privilege and HIV-1 persistence in the CNS. Immunol Rev (2006) 213:180–94. doi:10.1111/j.1600-065X.2006.00440.x
99. Joseph SB, Arrildt KT, Sturdevant CB, Swanstrom R. HIV-1 target cells in the CNS. J Neurovirol (2015) 21(3):276–89. doi:10.1007/s13365-014-0287-x
100. Baxter AE, Russell RA, Duncan CJ, Moore MD, Willberg CB, Pablos JL, et al. Macrophage infection via selective capture of HIV-1-infected CD4+ T cells. Cell Host Microbe (2014) 16(6):711–21. doi:10.1016/j.chom.2014.10.010
101. Calantone N, Wu F, Klase Z, Deleage C, Perkins M, Matsuda K, et al. Tissue myeloid cells in SIV-infected primates acquire viral DNA through phagocytosis of infected T cells. Immunity (2014) 41(3):493–502. doi:10.1016/j.immuni.2014.08.014
102. Honeycutt JB, Wahl A, Archin N, Choudhary S, Margolis D, Garcia JV. HIV-1 infection, response to treatment and establishment of viral latency in a novel humanized T cell-only mouse (TOM) model. Retrovirology (2013) 10:121. doi:10.1186/1742-4690-10-121
103. Honeycutt JB, Thayer WO, Baker CE, Ribeiro RM, Lada SM, Cao Y, et al. HIV persistence in tissue macrophages of humanized myeloid-only mice during antiretroviral therapy. Nat Med (2017) 23(5):638–43. doi:10.1038/nm.4319
105. Arainga M, Su H, Poluektova LY, Gorantla S, Gendelman HE. HIV-1 cellular and tissue replication patterns in infected humanized mice. Sci Rep (2016) 6:23513. doi:10.1038/srep23513
106. Brehm MA, Shultz LD, Luban J, Greiner DL. Overcoming current limitations in humanized mouse research. J Infect Dis (2013) 208(Suppl 2):S125–30. doi:10.1093/infdis/jit319
107. Karpel ME, Boutwell CL, Allen TM. BLT humanized mice as a small animal model of HIV infection. Curr Opin Virol (2015) 13:75–80. doi:10.1016/j.coviro.2015.05.002
108. Martinez-Torres F, Nochi T, Wahl A, Garcia JV, Denton PW. Hypogammaglobulinemia in BLT humanized mice – an animal model of primary antibody deficiency. PLoS One (2014) 9(10):e108663. doi:10.1371/journal.pone.0108663
109. Greenblatt MB, Vrbanac V, Tivey T, Tsang K, Tager AM, Aliprantis AO. Graft versus host disease in the bone marrow, liver and thymus humanized mouse model. PLoS One (2012) 7(9):e44664. doi:10.1371/journal.pone.0044664
110. Lavender KJ, Pace C, Sutter K, Messer RJ, Pouncey DL, Cummins NW, et al. An advanced BLT-humanized mouse model for extended HIV-1 cure studies. AIDS (2018) 32(1):1–10. doi:10.1097/QAD.0000000000001674
111. Hu Z, Van Rooijen N, Yang YG. Macrophages prevent human red blood cell reconstitution in immunodeficient mice. Blood (2011) 118(22):5938–46. doi:10.1182/blood-2010-11-321414
112. Huntington ND, Alves NL, Legrand N, Lim A, Strick-Marchand H, Mention JJ, et al. IL-15 transpresentation promotes both human T-cell reconstitution and T-cell-dependent antibody responses in vivo. Proc Natl Acad Sci U S A (2011) 108(15):6217–22. doi:10.1073/pnas.1019167108
113. Huntington ND, Legrand N, Alves NL, Jaron B, Weijer K, Plet A, et al. IL-15 trans-presentation promotes human NK cell development and differentiation in vivo. J Exp Med (2009) 206(1):25–34. doi:10.1084/jem.20082013
114. Shultz LD, Saito Y, Najima Y, Tanaka S, Ochi T, Tomizawa M, et al. Generation of functional human T-cell subsets with HLA-restricted immune responses in HLA class I expressing NOD/SCID/IL2r gamma(null) humanized mice. Proc Natl Acad Sci U S A (2010) 107(29):13022–7. doi:10.1073/pnas.1000475107
115. Flotte TR, Carter BJ. Adeno-associated virus vectors for gene therapy. Gene Ther (1995) 2(6):357–62.
116. Huang J, Li X, Coelho-dos-Reis JG, Wilson JM, Tsuji M. An AAV vector-mediated gene delivery approach facilitates reconstitution of functional human CD8+ T cells in mice. PLoS One (2014) 9(2):e88205. doi:10.1371/journal.pone.0088205
117. Huang J, Li X, Coelho-dos-Reis JG, Zhang M, Mitchell R, Nogueira RT, et al. Human immune system mice immunized with Plasmodium falciparum circumsporozoite protein induce protective human humoral immunity against malaria. J Immunol Methods (2015) 427:42–50. doi:10.1016/j.jim.2015.09.005
118. Saito A, Henning MS, Serrao E, Dubose BN, Teng S, Huang J, et al. Capsid-CPSF6 interaction is dispensable for HIV-1 replication in primary cells but is selected during virus passage in vivo. J Virol (2016) 90(15):6918–35. doi:10.1128/JVI.00019-16
119. Li X, Huang J, Zhang M, Funakoshi R, Sheetij D, Spaccapelo R, et al. Human CD8+ T cells mediate protective immunity induced by a human malaria vaccine in human immune system mice. Vaccine (2016) 34(38):4501–6. doi:10.1016/j.vaccine.2016.08.006
120. Sharma A, Wu W, Sung B, Huang J, Tsao T, Li X, et al. Respiratory syncytial virus (RSV) pulmonary infection in humanized mice induces human anti-RSV immune responses and pathology. J Virol (2016) 90(10):5068–74. doi:10.1128/JVI.00259-16
121. Kim J, Peachman KK, Jobe O, Morrison EB, Allam A, Jagodzinski L, et al. Tracking human immunodeficiency virus-1 infection in the humanized DRAG mouse model. Front Immunol (2017) 8:1405. doi:10.3389/fimmu.2017.01405
122. Li Y, Mention JJ, Court N, Masse-Ranson G, Toubert A, Spits H, et al. A novel Flt3-deficient HIS mouse model with selective enhancement of human DC development. Eur J Immunol (2016) 46(5):1291–9. doi:10.1002/eji.201546132
123. Chen Q, Khoury M, Chen J. Expression of human cytokines dramatically improves reconstitution of specific human-blood lineage cells in humanized mice. Proc Natl Acad Sci U S A (2009) 106(51):21783–8. doi:10.1073/pnas.0912274106
124. Chen Q, He F, Kwang J, Chan JK, Chen J. GM-CSF and IL-4 stimulate antibody responses in humanized mice by promoting T, B, and dendritic cell maturation. J Immunol (2012) 189(11):5223–9. doi:10.4049/jimmunol.1201789
125. Willinger T, Rongvaux A, Takizawa H, Yancopoulos GD, Valenzuela DM, Murphy AJ, et al. Human IL-3/GM-CSF knock-in mice support human alveolar macrophage development and human immune responses in the lung. Proc Natl Acad Sci U S A (2011) 108(6):2390–5. doi:10.1073/pnas.1019682108
126. Saito Y, Ellegast JM, Rafiei A, Song Y, Kull D, Heikenwalder M, et al. Peripheral blood CD34(+) cells efficiently engraft human cytokine knock-in mice. Blood (2016) 128(14):1829–33. doi:10.1182/blood-2015-10-676452
127. Ginhoux F, Lim S, Hoeffel G, Low D, Huber T. Origin and differentiation of microglia. Front Cell Neurosci (2013) 7:45. doi:10.3389/fncel.2013.00045
128. Windrem MS, Schanz SJ, Morrow C, Munir J, Chandler-Militello D, Wang S, et al. A competitive advantage by neonatally engrafted human glial progenitors yields mice whose brains are chimeric for human glia. J Neurosci (2014) 34(48):16153–61. doi:10.1523/JNEUROSCI.1510-14.2014
129. Gorantla S, Poluektova L, Gendelman HE. Rodent models for HIV-associated neurocognitive disorders. Trends Neurosci (2012) 35(3):197–208. doi:10.1016/j.tins.2011.12.006
130. Oberheim NA, Takano T, Han X, He W, Lin JH, Wang F, et al. Uniquely hominid features of adult human astrocytes. J Neurosci (2009) 29(10):3276–87. doi:10.1523/JNEUROSCI.4707-08.2009
131. Han X, Chen M, Wang F, Windrem M, Wang S, Shanz S, et al. Forebrain engraftment by human glial progenitor cells enhances synaptic plasticity and learning in adult mice. Cell Stem Cell (2013) 12(3):342–53. doi:10.1016/j.stem.2012.12.015
132. Uchida N, Buck DW, He D, Reitsma MJ, Masek M, Phan TV, et al. Direct isolation of human central nervous system stem cells. Proc Natl Acad Sci U S A (2000) 97(26):14720–5. doi:10.1073/pnas.97.26.14720
133. Li W, Gorantla S, Gendelman HE, Poluektova LY. Systemic HIV-1 infection produces a unique glial footprint in humanized mouse brains. Dis Model Mech (2017) 10(12):1489–502. doi:10.1242/dmm.031773
134. Gelman BB, Chen T, Lisinicchia JG, Soukup VM, Carmical JR, Starkey JM, et al. The national NeuroAIDS tissue consortium brain gene array: two types of HIV-associated neurocognitive impairment. PLoS One (2012) 7(9):e46178. doi:10.1371/journal.pone.0046178
135. Sanfilippo C, Pinzone MR, Cambria D, Longo A, Palumbo M, Di Marco R, et al. OAS gene family expression is associated with HIV-related neurocognitive disorders. Mol Neurobiol (2018) 55(3):1905–14. doi:10.1007/s12035-017-0460-3
Keywords: human immunodeficiency virus, central nervous system, human immune system mice, myeloid cells, HIV-associated neurocognitive disorders
Citation: Evering TH and Tsuji M (2018) Human Immune System Mice for the Study of Human Immunodeficiency Virus-Type 1 Infection of the Central Nervous System. Front. Immunol. 9:649. doi: 10.3389/fimmu.2018.00649
Received: 20 January 2018; Accepted: 16 March 2018;
Published: 04 April 2018
Edited by:
Urszula Krzych, Walter Reed Army Institute of Research, United StatesReviewed by:
Johannes S. Gach, University of California, Irvine, United StatesCopyright: © 2018 Evering and Tsuji. This is an open-access article distributed under the terms of the Creative Commons Attribution License (CC BY). The use, distribution or reproduction in other forums is permitted, provided the original author(s) and the copyright owner are credited and that the original publication in this journal is cited, in accordance with accepted academic practice. No use, distribution or reproduction is permitted which does not comply with these terms.
*Correspondence: Teresa H. Evering, dGV2ZXJpbmdAYWRhcmMub3Jn
Disclaimer: All claims expressed in this article are solely those of the authors and do not necessarily represent those of their affiliated organizations, or those of the publisher, the editors and the reviewers. Any product that may be evaluated in this article or claim that may be made by its manufacturer is not guaranteed or endorsed by the publisher.
Research integrity at Frontiers
Learn more about the work of our research integrity team to safeguard the quality of each article we publish.