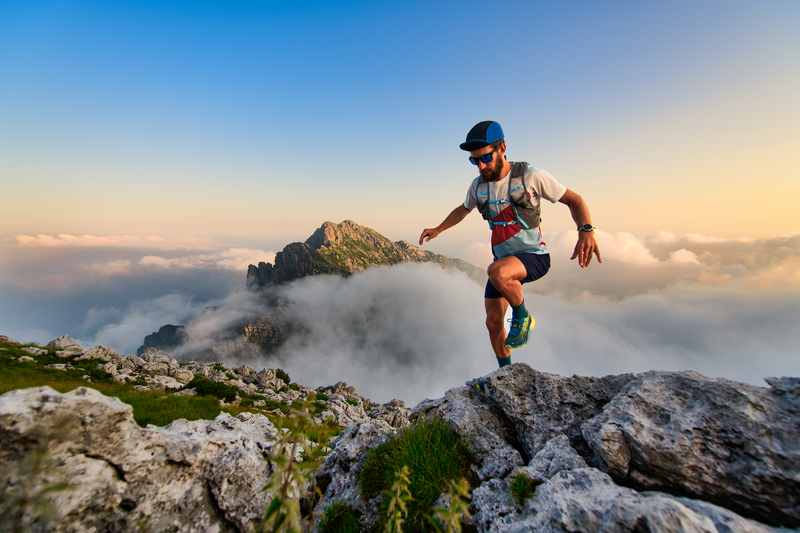
94% of researchers rate our articles as excellent or good
Learn more about the work of our research integrity team to safeguard the quality of each article we publish.
Find out more
ORIGINAL RESEARCH article
Front. Immunol. , 02 March 2018
Sec. Vaccines and Molecular Therapeutics
Volume 9 - 2018 | https://doi.org/10.3389/fimmu.2018.00325
This article is part of the Research Topic Nanoparticle Vaccines Against Infectious Diseases View all 16 articles
Streptococcus pneumoniae is a major causative agent of pneumonia, a debilitating disease particularly in young and elderly populations, and is the leading worldwide cause of death in children under the age of five. While there are existing vaccines against S. pneumoniae, none are protective across all serotypes. Pneumococcal surface protein A (PspA), a key virulence factor of S. pneumoniae, is an antigen that may be incorporated into future vaccines to address the immunological challenges presented by the diversity of capsular antigens. PspA has been shown to be immunogenic and capable of initiating a humoral immune response that is reactive across approximately 94% of pneumococcal strains. Biodegradable polyanhydrides have been studied as a nanoparticle-based vaccine (i.e., nanovaccine) platform to stabilize labile proteins, to provide adjuvanticity, and enhance patient compliance by providing protective immunity in a single dose. In this study, we designed a room temperature stable PspA-based polyanhydride nanovaccine that eliminated the need for a free protein component (i.e., 100% encapsulated within the nanoparticles). Mice were immunized once with the lead nanovaccine and upon challenge, presented significantly higher survival rates than animals immunized with soluble protein alone, even with a 25-fold reduction in protein dose. This lead nanovaccine formulation performed similarly to protein adjuvanted with Alum, however, with much less tissue reactogenicity at the site of immunization. By eliminating the free PspA from the nanovaccine formulation, the lead nanovaccine was efficacious after being stored dry for 60 days at room temperature, breaking the need for maintaining the cold chain. Altogether, this study demonstrated that a single dose PspA-based nanovaccine against S. pneumoniae induced protective immunity and provided thermal stability when stored at room temperature for at least 60 days.
Community-acquired pneumonia is a debilitating disease and is globally responsible for 16% of deaths in children under the age of five annually (1). Streptococcus pneumoniae, a causative agent of bacterial pneumonia, is a Gram-positive bacterial pathogen of humans, which colonizes the upper respiratory tract and leads to the development of potentially life-threatening diseases, such as otitis media, sinusitis, and pneumonia, with pneumonia being the most serious of these conditions (2, 3). While otitis media and sinusitis are not as severe, they still account for seven million cases in the United States annually (2). S. pneumoniae is asymptomatically carried in approximately 25% of healthy individuals and is spread between individuals through respiratory droplets, with children serving as the primary transmission source to adults (4–7). Pneumococcal infections are most prevalent in infants, young children, and the elderly, accounting for over $3 billion spent annually in direct healthcare spending; in addition, 60–87% of all pneumococcal bacteremia cases are associated with pneumonia in adults (2). While control of S. pneumoniae by antibiotics has been beneficial, up to 50% of all strains are resistant to erythromycin, with 97% of those also being resistant to azithromycin, making intervention strategies such as vaccination a necessary component of health care management (8). To date, the single most effective advance in the field of pneumonia prevention has been through immunization, though existing vaccines are still not completely effective (9, 10).
Current preventive strategies involve the use of pneumococcal polysaccharide vaccines (PPVs) and pneumococcal conjugate vaccines (PCVs). PPVs (T-independent antigens) comprise capsular polysaccharides, which are poorly immunogenic in very young and elderly individuals, while PCVs (T-dependent antigens) are more effective in these at-risk populations because of the adjoined protein component. While the introduction of PPV23, PCV7, and PCV13 have had significant contributions in reducing the overall global burden of pneumococcal pneumonia, there is still room for improvement (11). As it currently stands, infection with strains not covered by vaccine serotype infections account for approximately 50% of all deaths in individuals over 55 years of age (12). In addition, it has been observed that introduction of new vaccine strategies against S. pneumoniae, such as the introduction of PCV10 immunization program for children in the Netherlands or use of PCV7 in Spain, may be directly correlated to an increased emergence of disease caused by non-vaccine serotype strains cases (13–15). It was demonstrated that PCV7 immunization, which contains the 19F serotype antigen, actually caused an increase in pneumococcal infections by the closely related 19A serotype (16). Globally, this is of particular concern when considering the development of a universal vaccine, as it is now clear that the prevalence of certain serotypes differs greatly between age, geographic region, and ethnicity (3, 17, 18). PPV23 encompasses the most pneumococcal serotypes of the commercially available vaccines. However, it is not recommended for use in children under the age of 18 months because of their poor antibody response to T-independent polysaccharide antigens, explaining why PCV13 is recommended for this age group. While the use of PCV and PPV vaccine formulations have effectively reduced the incidence of pneumococcal pneumonia, there is a need for newer vaccines to protect against the emergence of non-vaccine strains of S. pneumoniae (19). Coupled with the phase variation in expression of capsular and surface protein antigens, broadly protective vaccines against colonization and invasive pneumococcal disease are likely to require the inclusion of more conserved antigens that will also facilitate the induction of antigen-specific CD4+ T cells (20, 21).
S. pneumoniae has several key virulence factors that play a critical role in colonization, transmission, and tissue damage, including pneumolysin, two neuraminidases, and pneumococcal surface protein A (PspA). PspA is a choline binding protein, one of the most abundant proteins located on the pneumococcal cell surface, and has been shown to be of particular importance in facilitating nasopharangyl colonization through inhibiting host complement responses (22). In the design of new vaccines against S. pneumoniae, it is important to consider a target immunogen that will be capable of inducing a broad, serotype-independent, protective immune response. PspA has been of recent interest as a potential vaccine candidate due to its location on the cell surface of all 95 strains of S. pneumoniae currently known and its critical role in pneumococcal pathogenesis (3, 23, 24). Anti-PspA antibodies to clades 1 and 2 of PspA have been shown to be cross-protective against S. pneumoniae strains encompassing all six clades of PspA, and provide protection when passively transferred to naïve mice as a therapeutic intervention following septic S. pneumoniae challenge (17, 25–27). The ability to provide protection across many serotypes of S. pneumoniae using only two clades of PspA allows for the design of a broadly cross-protective vaccine when compared to vaccines containing numerous capsular polysaccharides.
Polyanhydride nanoparticle-based vaccines (i.e., nanovaccines) represent next-generation vaccine platforms against pathogens such as S. pneumoniae. These nanovaccines are formulated using random copolymers based on 1,8-bis-(p-carboxyphenoxy)-3,6-dioxaoctane (CPTEG), 1,6-bis-(p-carboxyphenoxy)hexane (CPH), and sebacic acid (SA) and have been extensively studied as a nanovaccine platform against infectious pathogens, such as influenza virus, Yersinia pestis, and Bacillus anthracis (28–31).
Polyanhydrides provide vaccine delivery benefits and adjuvant properties that make them well suited as a vaccine delivery platform. These materials exhibit high biocompatibility with minimal injection site reactivity (32–34) (i.e., tenderness, swelling, and pain) in comparison to traditional adjuvants, such as Alum, which has been associated with immunization site tenderness and pain, and are currently FDA-approved for use to treat malignant gliomas in the brain (35, 36). In addition, polyanhydrides are hydrophobic and exhibit surface erosion characteristics, which helps stabilize labile proteins, protects protein denaturation from enzymatic cleavage and acidic degradation products, and allows for the prolonged release of antigen (28, 37–41). The sustained release of antigen allows for enhanced bioavailability of antigen to drive adaptive immune responses and allows for single dose administration and dose-sparing capabilities (30, 42–44). Previous work has shown that mice immunized with a single dose of nanovaccine encapsulating Y. pestis fusion protein F1-V were completely protected against Y. pestis lethal challenge after at least 280 days post-immunization (DPI) (31). Varying polyanhydride copolymer composition has shown to modulate internalization and persistence within APCs in vitro, as well as induction of both cellular and humoral immune responses in vivo indicating the ability to tailor polymer chemistry in order to rationally design nanovaccines that optimally inducing antigen-specific protective immunity (31, 42, 45–54).
Previous work from our laboratories has demonstrated that PspA encapsulated into nanoparticles maintained its stability, conformational structure, as well as biological activity upon release, which was measured using an apolactoferrin binding assay (37). In this same work, mice immunized with a single dose of the nanovaccine generated robust antibody titer and avidity to PspA, equivalent to that of antigen adjuvanted with MPLA. However, the protective efficacy of a PspA-based nanovaccine against lethal challenge has not been demonstrated. In this study, the ability of various polyanhydride nanovaccine chemistries as vaccine candidates was evaluated and it was demonstrated that a lead PspA-based nanovaccine protected animals against lethal challenge with a 25-fold reduction in overall PspA dose. Furthermore, this lead formulation was shown to be capable of storage at room temperature for at least 2 months without any loss of efficacy.
Chemicals used for monomer and polymer synthesis included sodium hydroxide, hydrobenzoic acid, dibromohexane, 1-methyl-2-pyrrolidinone, SA monomer, and triethylene glycol purchased from Sigma Aldrich (St. Louis, MO, USA). Acetic anhydride, ethyl ether, petroleum ether, chloroform, methylene chloride, hexane, acetone, sulfuric acid, potassium carbonate, dimethyl formamide, toluene, acetonitrile, N,N-dimethylacetamide, and acetic acid were purchased from Fisher Scientific (Fairlawn, NJ, USA); 4-p-fluorobenzonitrile was purchased from Apollo Scientific (Cheshire, UK). For 1H NMR analysis of the copolymers, deuterated chloroform and dimethyl sulfoxide were purchased from Cambridge Isotope Laboratories (Andover, MA, USA). The N-terminal region of a recombinant PspA (UAB055, PspA/Rx1 AA1 to 303, clade 2 PspA of the PspA family 1) was produced by Dr. David McPherson (University of Alabama at Birmingham) as described previously (55). Prior to immunization, endotoxin was removed from the protein using endotoxin removal beads (Miltenyi Biotec, Bergisch Gladbach, Germany) according to the manufacturer’s instructions followed by dialysis and lyophilization. The final endotoxin content of the protein was determined to be less than 1.9 EU/mg as determined by a limulus amebocyte lysate chromogenic endotoxin quantification kit (Thermo Fisher Scientific).
Monomers of CPH and CPTEG were synthesized as previously described (28, 49). A 50:50 molar composition copolymer of CPH and CPTEG and a 20:80 molar composition copolymer of CPH and SA was synthesized using melt condensation as described by Torres et al. (28). PspA protein, 1% (w/w), was encapsulated into polyanhydride nanoparticles using solid/oil/oil nanoprecipitation, as previously described (37). Excess buffer was removed from PspA protein solution using a 5 kDa MWCO dialysis microcentrifuge tube and the resulting protein solution was lyophilized overnight at −40°C under vacuum. The lyophilized protein and the respective copolymer was then dissolved in methylene chloride at a concentration of 20 mg/mL of solvent. The solution was sonicated using the VibraCell ultrasonic probe (Sonics & Materials, Inc., Newtown, CT, USA) to ensure complete dissolution and homogenization of the protein and the copolymer. The resulting solution was then added to pentane at a 1–200 (v/v) ratio of methylene chloride to pentane at 20°C for the 20:80 CPH:SA nanoparticle formulation or at a 1–250 (v/v) ratio of methylene chloride to pentane at −40°C and for the 50:50 CPTEG:CPH nanoparticle formulation. Nanoparticles were collected using vacuum filtration. Surface charge of representative 20:80 CPH:SA and 50:50 CPTEG:CPH nanoparticle formulations were measured using quasi-elastic light scattering (Zetasizer Nano, Malvern Instruments Ltd., Worcester, UK).
CBA/CaHN-Btkxid/J (CBA/N) mice were purchased from Jackson Laboratory (Bar Harbor, ME, USA) and used for the immunization studies. These mice have a mutation in their Bruton’s tyrosine kinase gene. Due to this mutation, these mice are unable to initiate a proper antibody response to Type II thymic-independent antigens (e.g., capsular polysaccharides), and are, thus, able to better mimic natural antibody responses in at-risk elderly or young populations. Mice were housed under specific pathogen-free conditions where all bedding, caging, water, and feed were sterilized prior to use. All studies were conducted with the approval of the Iowa State University Institutional Animal Care and Use Committee.
Groups of 8–10 CBA/N mice were immunized subcutaneously at the nape of the neck with 0–20 µg PspA, 0–5 µg PspA encapsulated into 50–500 µg polyanhydride nanoparticles, and/or 50 µL of Imject Alum Adjuvant (ThermoFisher Scientific) and delivered in a total volume of 100 µL. Immunization groups for the individual studies (see Tables 1 and 2) comprised the following : (i) 25 µg soluble PspA alone, (ii) 25 µg PspA administered with 50 µL Imject Alum, (iii) 5 µg PspA encapsulated into 250 µg 50:50 CPTEG:CPH nanoparticles + 20 µg soluble PspA, (iv) 5 µg PspA encapsulated into 250 µg 20:80 CPH:SA nanoparticles + 20 µg soluble PspA, (v) 1 µg soluble PspA alone, (vi) 1 µg PspA administered with 50 µL Imject Alum, (vii) 1 µg PspA encapsulated into 50 µg 50:50 CPTEG:CPH nanoparticles, (viii) 0.5 µg PspA encapsulated into 25 µg 50:50 CPTEG:CPH nanoparticles + 25 µg blank 50:50 CPTEG:CPH nanoparticles + 0.5 µg soluble PspA, or (ix) saline control. Blood was collected from mice via saphenous vein and serum was isolated following centrifugation (10,000 rcf for 10 min) at days 17 or 21 DPI. Serum was stored at −20°C until analysis could be performed.
Table 1. 25 µg Pneumococcal surface protein A (PspA)-containing single dose vaccine treatments groups.
Table 2. One-microgram pneumococcal surface protein A (PspA)-containing single dose vaccine treatments groups.
S. pneumoniae strain A66.1, of PspA family 1, clade 2 was used for challenge and grown as previously described (56, 57). Briefly, the bacteria was grown at 37°C for 18 h in filter-sterilized Todd-Hewitt broth with 0.5% yeast extract, and stored at −80°C at a concentration of 1 × 108 CFU/mL. Inoculum was diluted to a concentration of 2.5 × 104 CFU/mL in sterile PBS and 100 µL was administered intraveneously at 24 DPI. Mice were monitored three times per day for the duration of the challenge (14 days). Body temperatures and body condition scores were also recorded once per day. Mice were sacrificed when determined to be moribund or at 14 days post-challenge.
Anti-PspA antibody titers were determined via an ELISA as described previously (37). Briefly, high-binding Costar 590 EIA/RIA microtiter plates (Corning) were coated overnight at 4°C with 0.5 µg/mL PspA. The blocking buffer for this assay comprised 2.5% (w/v) powdered milk dissolved in PBS-T and incubated for 2 h at 56°C to inactivate any endogenous phosphatase activity. PspA-coated plates were incubated with blocking solution for 2 h at room temperature before being washed three times with PBS-T. Serum obtained from immunized mice was added to the first well of a row at a dilution of 1:200 and serially diluted in PBS-T containing 1% (v/v) goat serum. Each serum sample was tested in duplicate. Following incubation overnight at 4°C, plates were washed three times with PBS-T after which secondary antibody was added at a dilution of 1 µg/mL. The secondary antibody used in these studies was alkaline phosphatase-conjugated goat anti-mouse IgG heavy and light chain (Jackson ImmunoResearch) and was incubated on the plates for 2 h at room temperature. Plates were then washed three times with PBS-T and alkaline phospatase substrate (Fisher Scientific, Pittsburgh, PA, USA) was added at a concentration of 1 mg/mL dissolved in 50 mM sodium carbonate, 2 mM magnesium chloride buffer at pH 9.3 for colorimetric development. Plates were analyzed after 30 min using a SpectraMax M3 microplate reader at a wavelength of 405 nm. Titer is reported as the reciprocoal of serum dilution at which optical density value was at least twice that of the saline group average plus two SDs.
Shelf stability of the PspA nanovaccine was assessed via ELISA and lethal challenge. 50:50 CPTEG:CPH nanoparticles encapsulating PspA were taken out of the freezer (−80°C) and stored as a dry powder formulation at 25°C (room temperature) in a glass desiccator containing drierite for 60 days prior to immunization. Soluble PspA was stored at 4°C and combined with Imject Alum prior to immunization. A separate group of nanoparticles were kept in the freezer over the course of this experiment as a freezer-stored standard control. Following room temperature storage, vaccine efficacy was evaluated by immunizing mice with a nanovaccine dose equivalent to 1 µg PspA per animal from one of the storage conditions above, soluble PspA adsorbed to Alum, or the saline control and assessing antibody titer via ELISA 21 DPI (see “Anti-PspA Antibody Titer” for details). Following lethal challenge with 2,500 CFU of S. pneumoniae (see S. pneumoniae challenge for details), survival was assessed over the next 24 days.
Statistical analyses were performed using the Gehan–Breslow–Wilcoxon test and the one-way analysis of variance using Tukey’s multiple comparisons test. A p-value ≤0.05 was considered statistically significant. All analyses were performed using GraphPad Prism v. 7.0 software.
The nanoparticle chemistries chosen for the current study were based on 50:50 CPTEG:CPH and 20:80 CPH:SA copolymers, consistent with our previous work, in which these chemistries were shown to provide protein structural stability and maintain the functional activity of PspA following release (37). The nanoparticles were synthesized using solid/oil/oil emulsion (54). The PspA release kinetics from these formulations are described in our previous work (37). Scanning electron photomicrographs of the nanoparticles revealed similar sizes for the two formulations, with sizes of 455 ± 175 and 422 ± 163 nm for 50:50 CPTEG:CPH and 20:80 CPH:SA nanoparticles, respectively (Figure 1). Zeta potential measurements of empty 20:80 CPH:SA and 50:50 CPTEG:CPH nanoparticles revealed that the particles had a negative surface charge, with zeta potentials of −41.9 ± 0.9 and −33.1 ± 5.1 mV, respectively, consistent with previous publications (45, 48, 58).
Figure 1. Pneumococcal surface protein A nanovaccine characterization. Representative scanning electron photomicrographs of (A) 2% PspA-loaded (w/w) 20:80 CPH:SA nanoparticles and (B) 2% PspA-loaded (w/w) 50:50 CPTEG:CPH nanoparticles (scale bar = 5 µm). (C) Particle size distribution of 2% PspA-loaded (w/w) 20:80 CPH:SA nanoparticles (422 ± 163 nm). (D) Particle size distribution of 2% PspA-loaded (w/w) 50:50 CPTEG:CPH nanoparticles (455 ± 175 nm).
Previously, it was demonstrated that mice vaccinated with PspA-based nanovaccines induced a robust humoral response; however, protective efficacy was not evaluated (37). In the current studies, separate groups of CBA/CaHN mice (n = 8 per group) were immunized subcutaneously as follows: (i) 25 µg soluble PspA alone, (ii) 25 µg PspA administered with Imject Alum, (iii) PspA-containing 50:50 CPTEG:CPH nanoparticles, (iv) PspA-containing 20:80 CPH:SA nanoparticles, or (v) saline (Table 1). Both the nanovaccine formulations contained 5 µg of encapsulated PspA along with 20 µg of soluble protein, as shown in Table 1. Anti-PspA total IgG titers were evaluated at 17 DPI and there were no significant differences in titer between vaccinated groups (Figure 2A). Mice were challenged with a lethal dose of S. pneumoniae strain A66.1 at 24 DPI and survival was assessed over a period of 2 weeks. All PspA naïve (saline) mice succumbed to infection within 4 days, while all the immunized animals showed prolonged survival following challenge. At 14 days post-challenge, only 25% of the (non-adjuvanted) soluble PspA-immunized animals survived, whereas adjuvanting the protein with alum provided 100% protection. Immunization with the 20:80 CPH:SA nanovaccine resulted in 87% survival, while 100% of the animals immunized with 50:50 CPTEG:CPH nanovaccine were protected, as shown in Figure 2B. The survival data, in correlation with the antibody titers indicate that not only is PspA an effective immunogen, but induces protective immunity when delivered with an adjuvant. Though both nanoparticle chemistries protected animals from lethal challenge compared to saline, the 50:50 CPTEG:CPH PspA nanovaccine performed significantly (p ≤ 0.008) better than soluble PspA (i.e., sPspA) alone. Based on these results, we selected the 50:50 CPTEG:CPH nanovaccine as our lead candidate for further evaluation.
Figure 2. Pneumococcal surface protein A (PspA) nanovaccines are capable of initiating protective immune responses. (A) Serum was collected from immunized mice (see Table 1) at 17 days post-immunization (DPI) and analyzed for total anti-PspA IgG (data is represented as log10) using ELISA. Dashed line represents background anti-PspA IgG levels in serum from the saline-immunized mice. (B) S. pneumoniae challenge was performed at 24 DPI by i.v. administration of 2,500 CFUs of A66.1 strain and survival was monitored for 14 days post-challenge. * indicates significance (p ≤ 0.0025) compared to sPspA alone and # indicates significance (p ≤ 0.001) compared to saline-treated mice. Data are representative of one independent experiment containing n = 8 mice per treatment group.
Rational design of vaccines using subunit proteins involves considering total cost of the end product, as well as protein availability that potentially impacts vaccine shortages. Our previous work has shown that encapsulating a lowered dose of antigen into polyanhydride nanoparticles can induce a robust immune response (43). In the current studies, the ability of the nanovaccine to provide dose-sparing (i.e., 25-fold reduction) was evaluated by immunizing separate groups of CBA/N mice (n = 8 per group; see Table 2) subcutaneously using the following regimens: (i) 1 µg PspA encapsulated into 50:50 CPTEG:CPH nanoparticles (PspA Nanovaccine), (ii) 0.5 µg PspA encapsulated into 50:50 CPTEG:CPH nanoparticles plus 0.5 µg soluble PspA (PspA Nanovaccine + Soluble PspA), (iii) 1 µg soluble PspA administered with Imject Alum, (iv) 1 µg soluble PspA alone, and (v) saline alone (i.e., naïve control). Serum was collected at 21 DPI and analyzed for total anti-PspA IgG. Mice immunized with the nanovaccines or PspA + Alum showed significantly (p ≤ 0.008) higher titers to PspA compared to soluble PspA alone (Figure 3A). Survival rates following lethal challenge showed significantly (p ≤ 0.001) higher survival (87.5%) in mice immunized with both the nanovaccine formulations compared to animals immunized with soluble PspA (12%) (Figure 3B). Animals immunized with PspA adjuvanted with Alum performed similarly to the animals receiving the nanovaccine formulations. There were no observable differences in disease severity between the immunized animals, as measured by body condition scores and temperature (data not shown). In addition, soluble PspA administered in conjunction with 100 µg of empty 50:50 CPTEG:CPH nanoparticles, performed similarly to other adjuvanted groups, further indicating the ability for PspA to initiate a protective immune response when adjuvanted properly (data not shown). No significant differences in survival were observed between mice immunized with PspA fully encapsulated within the nanoparticles (i.e., PspA Nanovaccine) and animals that received half of the protein as a soluble bolus (i.e., PspA Nanovaccine + Soluble PspA). Eliminating the use of soluble PspA in the nanovaccine is beneficial when designing a shelf-stable dry powder formulation for the long-term storage of a PspA nanovaccine.
Figure 3. Nanovaccines with 25-fold reduction in total protein provided protection against lethal challenge. (A) Serum was collected from immunized mice (see Table 2) at 21 days post-immunization (DPI) and analyzed for total anti-PspA IgG (data is represented as log10) using ELISA. Dashed line represents background anti-PspA IgG levels in the serum from the saline-immunized mice. * indicates significance (p ≤ 0.008) compared to sPspA alone. (B) S. pneumoniae challenge was performed at 24 DPI by i.v. administration of 2,500 CFUs of A66.1 strain and survival was monitored for 14 days. * indicates significance (p ≤ 0.003) compared to sPspA alone and # indicates significance (p ≤ 0.0002) compared to saline-treated mice. Data are representative of one independent experiment containing n = 8 mice per treatment group.
To evaluate the potential for a room temperature stored (i.e., non-refrigerated) PspA nanovaccine, 1 µg of PspA was encapsulated within 50 µg of 50:50 CPTEG:CPH nanoparticles (i.e., 2 wt.% loading) and stored at room temperature (25°C) and standard storage conditions (−80°C), as detailed in the Section “Materials and Methods” and as previously described (28). The PspA encapsulated nanovaccine was stored at −80°C (freezer stored) or at 25°C for 60 days. The soluble PspA was stored at 4°C and mixed with Alum prior to immunization. Following storage, separate groups of mice (n = 8–10 per group) were immunized subcutaneously with the PspA nanovaccine stored at the different conditions or with alum-adjuvanted PspA and serum was collected and analyzed at 21 DPI. Storage conditions did not have a significant effect on anti-PspA IgG titers, with all of the PspA nanovaccines performing similarly (Figure 4A). Mice were then challenged with a lethal dose of S. pneumoniae at 24 DPI and survival was monitored for 2 weeks. All of the non-immunized mice succumbed to challenge within 4 days post challenge, while the nanovaccine-immunized mice presented with 60–70% survival with a single dose (Figure 4B). Once again, no significant differences were observed between the survival rates of mice immunized with the PspA nanovaccine stored at various conditions and those immunized with soluble PspA adjuvanted with Alum. These data indicate that the PspA nanovaccine is efficacious when stored both in the freezer or at room temperature for time periods up to 60 days and performs similarly to refrigerated PspA adjuvanted with Alum.
Figure 4. Room temperature-stored Pneumococcal surface protein A (PspA) nanovaccine maintains efficacy after 60 days. (A) Serum was collected from groups of immunized mice at 21 days post-immunization (DPI) with vaccine stored at different conditions as indicated and analyzed for total anti-PspA IgG (data are represented as log10) using ELISA. Dashed line represents background anti-PspA IgG levels in the serum from the saline-immunized mice. (B) S. pneumoniae challenge was performed at 24 DPI by i.v. administration of 2,500 CFUs of A66.1 strain and survival was monitored for 14 days. * indicates significance (p ≤ 0.001) compared to saline-treated mice. Data are representative of one independent experiment containing n = 8–10 mice per treatment group.
Streptococcus pneumoniae is a causative agent of debilitating bacterial pneumonia. While many existing vaccines have proven to be valuable in reducing overall global disease burden, none are without limitations. Newly developed vaccine strategies against S. pneumoniae have identified several novel antigens, including PspA. Anti-PspA antibodies have been observed, following natural colonization or infection in children (59, 60). Other studies have shown success against lethal intranasal or septic S. pneumoniae challenge by vaccinating with PspA, which further indicates its potential as an efficacious vaccine immunogen (22, 61).
Aluminum hydroxide (i.e., Alum)-based adjuvants are widely used because of their ability to promote Th2 immune responses (62). However, Alum-based vaccines are associated with higher levels of immunization site tissue reactogenicity (63, 64). Immunization-site tenderness and pain are the most commonly reported symptoms following immunization with vaccines containing Alum. Prevalence of these adverse reactions has been found to be directly associated with increased protein dose administered and decreased age of the vaccinated individuals (65–67). Previous work with polyanhydride nanovaccines has shown low-level tissue site inflammation and toxicity making them a safe alternative to traditional vaccine adjuvants (32, 33, 41). Therefore, nanovaccines may represent a safe and efficacious alternative to Alum-based vaccines, which have limitations associated with administration site reactogenicity and only promote humoral immune responses against PspA.
Work from other laboratories has shown limited success using nanotechnology-based vaccine platforms with PspA in various animal models though, to date, none of these studies have shown protection against lethal challenge with single-dose immunization (68–70). Previous work from our laboratories has indicated that encapsulation of PspA into polyanhydride nanoparticles is capable of retaining protein structure and function, which enabled initiation of a humoral immune response (37). Building upon these studies, PspA was encapsulated within both 50:50 CPTEG:CPH and 20:80 CPH:SA nanoparticle formulations in the current work. Both formulations produced nanoparticles of similar size and size distribution (Figure 1). The size, morphology, and size distribution of these particles were similar to those previously reported for these formulations (28, 30, 37, 42). Following subcutaneous immunization of mice with a single dose of either formulation, anti-PspA IgG antibodies were produced, in agreement with our previous findings (37). Following lethal septic challenge, both nanovaccine formulations (containing 25 µg PspA) were capable of conferring protective immunity in mice, with 87.5% survival in 20:80 CPH:SA nanovaccine-immunized mice and 100% protection in those that received the 50:50 CPTEG:CPH nanovaccine (Figure 2). Based on the increased protection and higher antibody titers observed following immunization with a single dose of the 50:50 CPTEG:CPH nanovaccine formulation, this chemistry was selected as the lead candidate nanovaccine.
Reducing the amount of protein necessary per dose can greatly reduce the cost associated with using a recombinant protein vaccine, thereby allowing for the production and storage of more doses. Most of the published reports evaluating PspA-based vaccine success immunized mice with a minimum of 5 µg of antigen plus an adjuvant and required multiple boosts (56, 61, 71, 72). Indeed, a recent report demonstrated complete protection in mice receiving 0.5 µg of PspA adjuvanted with poly(I:C) intranasally; however, the immunization schedule required three doses to provide protection (71). The need for a single dose vaccination regimen cannot be overstated from a patient compliance standpoint because according to the WHO, an estimated 29% of children aged 1 month–5 years die each year from vaccine preventable diseases, citing non-compliance with the immunization regimen as a key reason for lack of successful national vaccination programs (73). Therefore, single-dose vaccines can aid in improving patient compliance, reduce cost, and improve national immunization rates worldwide. Recent work from our laboratories has demonstrated that 25 µg of ovalbumin encapsulated into polyanhydride microparticles was able to elicit robust antibody titers equivalent to a 16× larger soluble dose of ovalbumin while 25 µg of soluble ovalbumin alone was unable to elicit detectable antibody responses (43). In order to corroborate these results with PspA, the dose of antigen evaluated was reduced 25-fold to 1 µg. Immunization with the 1 µg PspA-based 50:50 CPTEG:CPH nanovaccine formulation, both with and without soluble PspA, induced production of high anti-PspA IgG titers (Figure 3A), with very little reduction in overall antibody titer compared to the previous study with a 25 µg dose of PspA (i.e., data in Figure 2). Antibody titers were similar between mice immunized with 1 µg of soluble PspA administered with Alum or with the nanovaccine formulations, with the highest overall titers observed in mice immunized with the fully encapsulated nanovaccine formulation. When compared to soluble protein adjuvanted with Alum, mice immunized with the 50:50 CPTEG:CPH nanovaccine were similarly protected from lethal challenge. Furthermore, 87.5% of the animals challenged with the reduced dose PspA nanovaccine (with or without soluble PspA) were protected (Figure 3B). These data demonstrate that reducing the protein dose by 25-fold had little effect on survival, with both the fully encapsulated PspA nanovaccine and the nanovaccine administered with a soluble bolus providing significantly higher survival following lethal challenge, compared to soluble protein alone.
By eliminating the soluble protein component from vaccine formulations, nanovaccines can be stored as a dry powder outside of refrigeration with the potential for long-term shelf storage, thereby circumventing the cold chain. As it currently stands, all prequalified WHO vaccines require refrigeration (73). Nanovaccines may enable next-generation vaccines to be stored without the need for refrigeration (i.e., eliminating the cold chain), allowing for vaccines to be sent to remote locations in need for vaccination, resulting in reduced medical costs and productivity losses associated with disease (74). Previous work from our laboratories demonstrated that nanovaccines encapsulating PspA were able to retain protein structure and activity following release, and a nanovaccine encapsulating the Bacillus anthracis protective antigen (PA) released biologically and immunologically functional protein following storage at both room temperature and above after 4 months (28, 37). By contrast, aluminum salt-based adjuvants have been reported to alter the structure of proteins and decrease thermal stability following adsorption (75), and previous work from our laboratories has shown that PA adsorbed to alum lost its functional activity when stored for 4 months at refrigerated conditions or above (28). Following storage at room temperature for 60 days, the PspA nanovaccine was shown to be efficacious, as measured by antibody titer and survival (Figure 4). PspA adsorbed to alum performed similarly, however, because PspA is a stable protein with coiled structure, it is likely that differences may be observed between the efficacy of the nanovaccine and alum-based formulations following storage for an extended period of time, or at elevated temperature, and should be evaluated in future studies (22). As such, this work indicates that the PspA nanovaccine formulation can be stored as a dry powder for at least 60 days while maintaining its immunogenicity.
In summary, this work describes the design of a safe next-generation PspA-based pneumonia nanovaccine with dose-sparing, protective immunity, and enhanced room temperature storage. These studies are promising first steps with respect to establishing the polyanhydride nanovaccine platform as an alternative to traditional vaccines in terms of reduced cost (enabled by the dose sparing and single dose capabilities) and difficulties associated with transportation and storage of vaccines in developing countries (enabled by the superior room temperature storage capabilities).
This study was carried out in accordance with the recommendations of the Iowa State University Institutional Animal Care and Use Committee. The protocol was approved by the Iowa State University Institutional Animal Care and Use Committee.
DW-M, SH, and SK performed the experiments and analyzed the data. MW and BN, together with DW-M, SH, and SK, designed the experimental plan. All the authors participated in writing the manuscript.
The authors declare that the research was conducted in the absence of any commercial or financial relationships that could be construed as a potential conflict of interest.
The authors thank Dr. David McPherson of the University of Alabama at Birmingham for producing the recombinant PspA used in this work. The authors acknowledge financial support from NIH-NIAID (R56-AI075026) and the Nanovaccine Institute. BN acknowledges the support of the Vlasta Klima Balloun Faculty Chair.
1. O’Brien KL, Wolfson LJ, Watt JP, Henkle E, Deloria-Knoll M, McCall N, et al. Burden of disease caused by Streptococcus pneumoniae in children younger than 5 years: global estimates. Lancet (2009) 374:893–902. doi:10.1016/S0140-6736(09)61204-6
2. Huang SS, Johnson KM, Ray GT, Wroe P, Lieu TA, Moore MR, et al. Healthcare utilization and cost of pneumococcal disease in the United States. Vaccine (2011) 29:3398–412. doi:10.1016/j.vaccine.2011.02.088
3. Jedrzejas MJ. Pneumococcal virulence factors : structure and function pneumococcal virulence factors: structure and function. Microbiol Mol Biol Rev (2001) 65:187–207. doi:10.1128/MMBR.65.2.187
4. Althouse BM, Hammitt LL, Grant L, Wagner BG, Reid R, Larzelere-Hinton F, et al. Identifying transmission routes of Streptococcus pneumoniae and sources of acquisitions in high transmission communities. Epidemiol Infect (2017) 145:2750–8. doi:10.1017/S095026881700125X
5. Mendy AL, Agbla SC, Odutola AA, Antonio M, Greenwood M, Sutherland JS, et al. Kinetics of antibodies against pneumococcal proteins and their relationship to nasopharyngeal carriage in the first two months of life. PLoS One (2017) 12(10):e0185824. doi:10.1371/journal.pone.0185824
6. Quintero B, Araque M, Van Der Gaast-De Jongh C, Escalona F, Correa M, Morillo-Puente S, et al. Epidemiology of Streptococcus pneumoniae and Staphylococcus aureus colonization in healthy Venezuelan children. Eur J Clin Microbiol Infect Dis (2011) 30:7–19. doi:10.1007/s10096-010-1044-6
7. Wyllie AL, Pannekoek Y, Bovenkerk S, van Engelsdorp Gastelaars J, Ferwerda B, van de Beek D, et al. Sequencing of the variable region of rpsB to discriminate between Streptococcus pneumoniae and other streptococcal species. Open Biol (2017) 7:170074. doi:10.1098/rsob.170074
8. Song J-H, Chang H-H, Suh JY, Ko KS, Jung S-I, Oh WS, et al. Macrolide resistance and genotypic characterization of Streptococcus pneumoniae in Asian countries: a study of the Asian Network for Surveillance of Resistant Pathogens (ANSORP). J Antimicrob Chemother (2004) 53:457–63. doi:10.1093/jac/dkh118
9. Grabenstein JD, Musey LK. Differences in serious clinical outcomes of infection caused by specific pneumococcal serotypes among adults. Vaccine (2014) 32:2399–405. doi:10.1016/j.vaccine.2014.02.096
10. Jacobs DM, Yung F, Hart E, Nguyen MNH, Shaver A. Trends in pneumococcal meningitis hospitalizations following the introduction of the 13-valent pneumococcal conjugate vaccine in the United States. Vaccine (2017) 35:6160–5. doi:10.1016/j.vaccine.2017.09.050
11. Bonten MJM, Huijts SM, Bolkenbaas M, Webber C, Patterson S, Gault S, et al. Polysaccharide conjugate vaccine against pneumococcal pneumonia in adults. N Engl J Med (2015) 372:1114–25. doi:10.1056/NEJMoa1408544
12. Marrie TJ, Tyrrell GJ, Majumdar SR, Eurich DT. Effect of age on the manifestations and outcomes of invasive pneumococcal disease in adults. Am. J. Med (2017) 131(1):.e1–100. doi:10.1016/j.amjmed.2017.06.039
13. Guevara M, Barricarte A, Gil-Setas A, García-Irure JJ, Beristain X, Torroba L, et al. Changing epidemiology of invasive pneumococcal disease following increased coverage with the heptavalent conjugate vaccine in Navarre. Clin Microbiol Infect (2009) 15:1013–9. doi:10.1111/j.1469-0691.2009.02904.x
14. Pletz MW, Maus U, Krug N, Welte T, Lode H. Pneumococcal vaccines: mechanism of action, impact on epidemiology and adaption of the species. Int J Antimicrob Agents (2008) 32:199–206. doi:10.1016/j.ijantimicag.2008.01.021
15. Vestjens SMT, Wagenvoort GHJ, Grutters JC, Meek B, Aldenkamp AF, Vlaminckx BJM, et al. Changes in pathogens and pneumococcal serotypes causing community-acquired pneumonia in The Netherlands. Vaccine (2017) 35:4112–8. doi:10.1016/j.vaccine.2017.06.049
16. Hausdorff WP, Hoet B, Schuerman L. Do pneumococcal conjugate vaccines provide any cross-protection against serotype 19A? BMC Pediatr (2010) 10:4. doi:10.1186/1471-2431-10-4
17. Kristian SA, Ota T, Bubeck SS, Cho R, Groff BC, Kubota T, et al. Generation and improvement of effector function of a novel broadly reactive and protective monoclonal antibody against pneumococcal surface protein A of Streptococcus pneumoniae. PLoS One (2016) 11:e0154616. doi:10.1371/journal.pone.0154616
18. Rolo D, Ardanuy C, Fleites A, Martín R, Liñares J. Diversity of pneumococcal surface protein A (PspA) among prevalent clones in Spain. BMC Microbiol (2009) 9:80. doi:10.1186/1471-2180-9-80
19. Weil-Olivier C, van der Linden M, de Schutter I, Dagan R, Mantovani L. Prevention of pneumococcal diseases in the post-seven valent vaccine era: a European perspective. BMC Infect Dis (2012) 12:207. doi:10.1186/1471-2334-12-207
20. Khan MN, Xu Q, Pichichero ME. Protection against Streptococcus pneumoniae invasive pathogenesis by a protein-based vaccine is achieved by suppression of nasopharyngeal bacterial density during influenza A virus coinfection. Infect Immun (2017) 85:e00530–16. doi:10.1128/IAI.00530-16
21. Pichichero ME. Pneumococcal whole-cell and protein-based vaccines: changing the paradigm. Expert Rev Vaccines (2017) 16:1181–90. doi:10.1080/14760584.2017.1393335
22. Khan N, Jan AT. Towards identifying protective B-cell epitopes: the PspA story. Front Microbiol (2017) 8:742. doi:10.3389/fmicb.2017.00742
23. Mehr S, Wood N. Streptococcus pneumoniae – a review of carriage, infection, serotype replacement and vaccination. Paediatr Respir Rev (2012) 13:258–64. doi:10.1016/j.prrv.2011.12.001
24. Zafar MA, Hamaguchi S, Zangari T, Cammer M, Weiser JN. Capsule type and amount affect shedding and transmission of Streptococcus pneumoniae. mBio (2017) 8:e989–917. doi:10.1128/mBio.00989-17
25. Darrieux M, Moreno AT, Ferreira DM, Pimenta FC, de Andrade ALSS, Lopes APY, et al. Recognition of pneumococcal isolates by antisera raised against PspA fragments from different clades. J Med Microbiol (2008) 57:273–8. doi:10.1099/jmm.0.47661-0
26. Goulart C, Darrieux M, Rodriguez D, Pimenta FC, Cristina Brandileone MC, Lucia de Andrade AS, et al. Selection of family 1 PspA molecules capable of inducing broad-ranging cross-reactivity by complement deposition and opsonophagocytosis by murine peritoneal cells. Vaccine (2010) 29:1634–42. doi:10.1016/j.vaccine.2010.12.074
27. Piao Z, Akeda Y, Takeuchi D, Ishii KJ, Ubukata K, Briles DE, et al. Protective properties of a fusion pneumococcal surface protein A (PspA) vaccine against pneumococcal challenge by five different PspA clades in mice. Vaccine (2014) 32:5607–13. doi:10.1016/j.vaccine.2014.07.108
28. Petersen LK, Phanse Y, Ramer-Tait AE, Wannemuehler MJ, Narasimhan B. Amphiphilic polyanhydride nanoparticles stabilize bacillus anthracis protective antigen. Mol. Pharm (2012) 9:874–82. doi:10.1021/mp2004059
29. Ross K, Adams J, Loyd H, Ahmed S, Sambol A, Broderick S, et al. Combination nanovaccine demonstrates synergistic enhancement in efficacy against influenza. ACS Biomater Sci Eng (2016) 2:368–74. doi:10.1021/acsbiomaterials.5b00477
30. Ulery BD, Kumar D, Ramer-Tait AE, Metzger DW, Wannemuehler MJ, Narasimhan B. Design of a protective single-dose intranasal nanoparticle-based vaccine platform for respiratory infectious diseases. PLoS One (2011) 6:e17642. doi:10.1371/journal.pone.0017642
31. Ulery BD, Petersen LK, Phanse Y, Kong CS, Broderick SR, Kumar D, et al. Rational design of pathogen-mimicking amphiphilic materials as nanoadjuvants. Sci Rep (2011) 1:198. doi:10.1038/srep00198
32. Adler AF, Petersen LK, Wilson JH, Torres MP, Thorstenson JB, Gardner SW, et al. High throughput cell-based screening of biodegradable polyanhydride libraries. Comb Chem High Throughput Screen (2009) 12:634–45.
33. Huntimer L, Ramer-Tait AE, Petersen LK, Ross KA, Walz KA, Wang C, et al. Evaluation of biocompatibility and administration site reactogenicity of polyanhydride-particle-based platform for vaccine delivery. Adv Healthc Mater (2013) 2:369–78. doi:10.1002/adhm.201200181
34. Vela-Ramirez JE, Goodman JT, Boggiatto PM, Roychoudhury R, Pohl NLB, Hostetter JM, et al. Safety and biocompatibility of carbohydrate-functionalized polyanhydride nanoparticles. AAPS J (2015) 17:256–67. doi:10.1208/s12248-014-9699-z
35. Brem H, Kader A, Epstein JI, Tamargo RJ, Domb A, Langer R, et al. Biocompatibility of a biodegradable, controlled-release polymer in the rabbit brain. Sel Cancer Ther (1989) 5:55–65. doi:10.1089/sct.1989.5.55
36. Brem H, Domb A, Lenartz D, Dureza C, Olivi A, Epstein JI. Brain biocompatibility of a biodegradable controlled release polymer consisting of anhydride copolymer of fatty acid dimer and sebacic acid. J Control Release (1992) 19:325–9. doi:10.1016/0168-3659(92)90087-8
37. Haughney SL, Petersen LK, Schoofs AD, Ramer-Tait AE, King JD, Briles DE, et al. Retention of structure, antigenicity, and biological function of pneumococcal surface protein A (PspA) released from polyanhydride nanoparticles. Acta Biomater (2013) 9:8262–71. doi:10.1016/j.actbio.2013.06.006
38. Renukaradhya GJ, Narasimhan B, Mallapragada SK. Respiratory nanoparticle-based vaccines and challenges associated with animal models and translation. J Control Release (2015) 219:622–31. doi:10.1016/j.jconrel.2015.09.047
39. Ross KA, Loyd H, Wu W, Huntimer L, Wannemuehler MJ, Carpenter S, et al. Structural and antigenic stability of H5N1 hemagglutinin trimer upon release from polyanhydride nanoparticles. J Biomed Mater Res A (2014) 102:4161–8. doi:10.1002/jbm.a.35086
40. Torres MP, Vogel BM, Narasimhan B, Mallapragada SK. Synthesis and characterization of novel polyanhydrides with tailored erosion mechanisms. J Biomed Mater Res A (2006) 76:102–10. doi:10.1002/jbm.a.30510
41. Vela Ramirez JE, Roychoudhury R, Habte HH, Cho MW, Pohl NLB, Narasimhan B. Carbohydrate-functionalized nanovaccines preserve HIV-1 antigen stability and activate antigen presenting cells. J Biomater Sci Polym Ed (2014) 25:1387–406. doi:10.1080/09205063.2014.940243
42. Haughney SL, Ross KA, Boggiatto PM, Wannemuehler MJ, Narasimhan B. Effect of nanovaccine chemistry on humoral immune response kinetics and maturation. Nanoscale (2014) 6:13770–8. doi:10.1039/C4NR03724C
43. Huntimer L, Wilson Welder JH, Ross K, Carrillo-Conde B, Pruisner L, Wang C, et al. Single immunization with a suboptimal antigen dose encapsulated into polyanhydride microparticles promotes high titer and avid antibody responses. J Biomed Mater Res B Appl Biomater (2013) 101:91–8. doi:10.1002/jbm.b.32820
44. Vela Ramirez JE, Tygrett LT, Hao J, Habte HH, Cho MW, Greenspan NS, et al. Polyanhydride nanovaccines induce germinal center B cell formation and sustained serum antibody responses. J Biomed Nanotechnol (2016) 12:1303–11. doi:10.1166/jbn.2016.2242
45. Carrillo-Conde B, Song E-H, Chavez-Santoscoy A, Phanse Y, Ramer-Tait AE, Pohl NLB, et al. Mannose-functionalized “pathogen-like” polyanhydride nanoparticles target C-type lectin receptors on dendritic cells. Mol Pharm (2011) 8:1877–86. doi:10.1021/mp200213r
46. Carrillo-Conde BR, Ramer-Tait AE, Wannemuehler MJ, Narasimhan B. Chemistry-dependent adsorption of serum proteins onto polyanhydride microparticles differentially influences dendritic cell uptake and activation. Acta Biomater (2012) 8:3618–28. doi:10.1016/j.actbio.2012.06.001
47. Chavez-Santoscoy AV, Roychoudhury R, Pohl NLB, Wannemuehler MJ, Narasimhan B, Ramer-Tait AE. Tailoring the immune response by targeting C-type lectin receptors on alveolar macrophages using “pathogen-like” amphiphilic polyanhydride nanoparticles. Biomaterials (2012) 33:4762–72. doi:10.1016/j.biomaterials.2012.03.027
48. Goodman JT, Vela Ramirez JE, Boggiatto PM, Roychoudhury R, Pohl NLB, Wannemuehler MJ, et al. Nanoparticle chemistry and functionalization differentially regulates dendritic cell-nanoparticle interactions and triggers dendritic cell maturation. Part Part Syst Charact (2014) 31:1269–80. doi:10.1002/ppsc.201400148
49. Kipper MJ, Wilson JH, Wannemuehler MJ, Narasimhan B. Single dose vaccine based on biodegradable polyanhydride microspheres can modulate immune response mechanism. J Biomed Mater Res A (2006) 76:798–810. doi:10.1002/jbm.a.30545
50. Petersen LK, Xue L, Wannemuehler MJ, Rajan K, Narasimhan B. The simultaneous effect of polymer chemistry and device geometry on the in vitro activation of murine dendritic cells. Biomaterials (2009) 30:5131–42. doi:10.1016/j.biomaterials.2009.05.069
51. Petersen LK, Ramer-Tait AE, Broderick SR, Kong C-S, Ulery BD, Rajan K, et al. Activation of innate immune responses in a pathogen-mimicking manner by amphiphilic polyanhydride nanoparticle adjuvants. Biomaterials (2011) 32:6815–22. doi:10.1016/j.biomaterials.2011.05.063
52. Phanse Y, Carrillo-Conde BR, Ramer-Tait AE, Roychoudhury R, Pohl NLB, Narasimhan B, et al. Functionalization of polyanhydride microparticles with di-mannose influences uptake by and intracellular fate within dendritic cells. Acta Biomater (2013) 9:8902–9. doi:10.1016/J.ACTBIO.2013.06.024
53. Torres MP, Wilson-Welder JH, Lopac SK, Phanse Y, Carrillo-Conde B, Ramer-Tait AE, et al. Polyanhydride microparticles enhance dendritic cell antigen presentation and activation. Acta Biomater (2011) 7:2857–64. doi:10.1016/j.actbio.2011.03.023
54. Ulery BD, Phanse Y, Sinha A, Wannemuehler MJ, Narasimhan B, Bellaire BH. Polymer chemistry influences monocytic uptake of polyanhydride nanospheres. Pharm. Res (2009) 26:683–90. doi:10.1007/s11095-008-9760-7
55. Mirza S, Benjamin WH, Coan PA, Hwang SA, Winslett AK, Yother J, et al. The effects of differences in pspA alleles and capsular types on the resistance of Streptococcus pneumoniae to killing by apolactoferrin. Microb Pathog (2016) 99:209–19. doi:10.1016/j.micpath.2016.08.029
56. Arulanandam BP, Lynch JM, Briles DE, Hollingshead S, Metzger DW. Intranasal vaccination with pneumococcal surface protein A and interleukin-12 augments antibody-mediated opsonization and protective immunity against Streptococcus pneumoniae infection. Infect Immun (2001) 69:6718–24. doi:10.1128/IAI.69.11.6718-6724.2001
57. Briles DE, Hollingshead SK, King J, Swift A, Braun PA, Park MK, et al. Immunization of humans with Recombinant Pneumococcal Surface Protein A (rPspA) elicits antibodies that passively protect mice from fatal infection with Streptococcus pneumoniae bearing heterologous PspA. J Infect Dis (2000) 182:1694–701. doi:10.1086/317602
58. Wafa EI, Geary SM, Goodman JT, Narasimhan B, Salem AK. The effect of polyanhydride chemistry in particle-based cancer vaccines on the magnitude of the anti-tumor immune response. Acta Biomater (2017) 50:417–27. doi:10.1016/j.actbio.2017.01.005
59. Lebon A, Verkaik NJ, Labout JAM, de Vogel CP, Hooijkaas H, Verbrugh HA, et al. Natural antibodies against several pneumococcal virulence proteins in children during the pre-pneumococcal-vaccine era: the generation R study. Infect Immun (2011) 79:1680–7. doi:10.1128/IAI.01379-10
60. Melin MM, Hollingshead SK, Briles DE, Lahdenkari MI, Kilpi TM, Käyhty HM. Development of antibodies to PspA families 1 and 2 in children after exposure to Streptococcus pneumoniae. Clin Vaccine Immunol (2008) 15:1529–35. doi:10.1128/CVI.00181-08
61. Daniels CC, Coan P, King J, Hale J, Benton KA, Briles DE, et al. The proline-rich region of pneumococcal surface proteins A and C contains surface-accessible epitopes common to all pneumococci and elicits antibody-mediated protection against sepsis. Infect Immun (2010) 78:2163–72. doi:10.1128/IAI.01199-09
62. Bungener L, Geeraedts F, Ter Veer W, Medema J, Wilschut J, Huckriede A. Alum boosts TH2-type antibody responses to whole-inactivated virus influenza vaccine in mice but does not confer superior protection. Vaccine (2008) 26:2350–9. doi:10.1016/j.vaccine.2008.02.063
63. Chen D, Kristensen D. Opportunities and challenges of developing thermostable vaccines. Expert Rev Vaccines (2009) 8:547–57. doi:10.1586/ERV.09.20
64. Levy O, Goriely S, Kollmann TR. Immune response to vaccine adjuvants during the first year of life. Vaccine (2013) 31(21):2500–5. doi:10.1016/j.vaccine.2012.10.016
65. Brady RC, Treanor JJ, Atmar RL, Keitel WA, Edelman R, Chen WH, et al. Safety and immunogenicity of a subvirion inactivated influenza A/H5N1 vaccine with or without aluminum hydroxide among healthy elderly adults. Vaccine (2009) 27:5091–5. doi:10.1016/j.vaccine.2009.06.057
66. Petrovsky N. Comparative safety of vaccine adjuvants: a summary of current evidence and future needs. Drug Saf (2015) 38(11):1059–74. doi:10.1007/s40264-015-0350-4
67. Willhite CC, Karyakina NA, Yokel RA, Yenugadhati N, Wisniewski TM, Arnold IMF, et al. Systematic review of potential health risks posed by pharmaceutical, occupational and consumer exposures to metallic and nanoscale aluminum, aluminum oxides, aluminum hydroxide and its soluble salts. Crit Rev Toxicol (2014) 44(Suppl 4):1–80. doi:10.3109/10408444.2014.934439
68. Fukuyama Y, Yuki Y, Katakai Y, Harada N, Takahashi H, Takeda S, et al. Nanogel-based pneumococcal surface protein A nasal vaccine induces microRNA-associated Th17 cell responses with neutralizing antibodies against Streptococcus pneumoniae in macaques. Mucosal Immunol (2015) 8(5):1144–53. doi:10.1038/mi.2015.5
69. Gyu Kong I, Sato A, Yuki Y, Nochi T, Takahashi H, Sawada S, et al. Nanogel-based pspa intranasal vaccine prevents invasive disease and nasal colonization by streptococcus pneumoniae. Infect Immun (2013) 81:1625–34. doi:10.1128/IAI.00240-13
70. Kunda NK, Alfagih IM, Miyaji EN, Figueiredo DB, Gonçalves VM, Ferreira DM, et al. Pulmonary dry powder vaccine of pneumococcal antigen loaded nanoparticles. Int J Pharm (2015) 495:903–12. doi:10.1016/J.IJPHARM.2015.09.034
71. Ezoe H, Akeda Y, Piao Z, Aoshi T, Koyama S, Tanimoto T, et al. intranasal vaccination with pneumococcal surface protein A plus poly(I:C) protects against secondary pneumococcal pneumonia in mice. Vaccine (2011) 29:1754–61. doi:10.1016/j.vaccine.2010.12.117
72. Tostes RO, Rodrigues TC, Da Silva JB, Schanoski AS, Oliveira MLS, Miyaji EN. Protection elicited by nasal immunization with recombinant pneumococcal surface protein A (rPspA) adjuvanted with whole-cell pertussis vaccine (wP) against co-colonization of mice with Streptococcus pneumoniae. PLoS One (2017) 12:e0170157. doi:10.1371/journal.pone.0170157
73. Walters AA, Krastev C, Hill AVS, Milicic A. Next generation vaccines: single-dose encapsulated vaccines for improved global immunisation coverage and efficacy. J Pharm Pharmacol (2015) 67:400–8. doi:10.1111/jphp.12367
74. Lee BY, Wedlock PT, Haidari LA, Elder K, Potet J, Manring R, et al. Economic impact of thermostable vaccines. Vaccine (2017) 35:3135–42. doi:10.1016/j.vaccine.2017.03.081
Keywords: pneumococcal infections, pneumococcal surface protein A, polyanhydride, nanovaccine, room temperature stability
Citation: Wagner-Muñiz DA, Haughney SL, Kelly SM, Wannemuehler MJ and Narasimhan B (2018) Room Temperature Stable PspA-Based Nanovaccine Induces Protective Immunity. Front. Immunol. 9:325. doi: 10.3389/fimmu.2018.00325
Received: 07 December 2017; Accepted: 06 February 2018;
Published: 02 March 2018
Edited by:
Rajko Reljic, St George’s, University of London, United KingdomReviewed by:
Giampiero Pietrocola, University of Pavia, ItalyCopyright: © 2018 Wagner-Muñiz, Haughney, Kelly, Wannemuehler and Narasimhan. This is an open-access article distributed under the terms of the Creative Commons Attribution License (CC BY). The use, distribution or reproduction in other forums is permitted, provided the original author(s) and the copyright owner are credited and that the original publication in this journal is cited, in accordance with accepted academic practice. No use, distribution or reproduction is permitted which does not comply with these terms.
*Correspondence: Balaji Narasimhan, bmJhbGFqaUBpYXN0YXRlLmVkdQ==
Disclaimer: All claims expressed in this article are solely those of the authors and do not necessarily represent those of their affiliated organizations, or those of the publisher, the editors and the reviewers. Any product that may be evaluated in this article or claim that may be made by its manufacturer is not guaranteed or endorsed by the publisher.
Research integrity at Frontiers
Learn more about the work of our research integrity team to safeguard the quality of each article we publish.