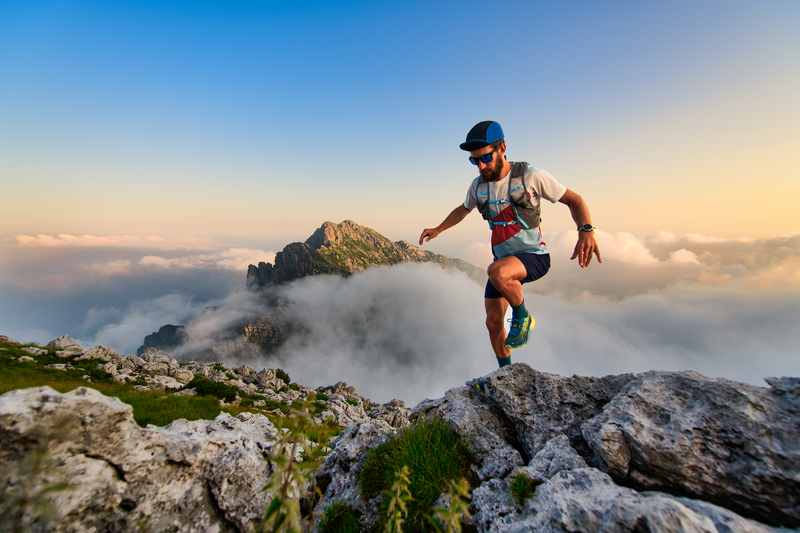
95% of researchers rate our articles as excellent or good
Learn more about the work of our research integrity team to safeguard the quality of each article we publish.
Find out more
REVIEW article
Front. Immunol. , 25 January 2018
Sec. Molecular Innate Immunity
Volume 9 - 2018 | https://doi.org/10.3389/fimmu.2018.00044
This article is part of the Research Topic Apoptotic Cell Clearance in Health and Disease View all 25 articles
Toxic substances and microbial or food-derived antigens continuously challenge the liver, which is tasked with their safe neutralization. This vital organ is also important for the removal of apoptotic immune cells during inflammation and has been previously described as a “graveyard” for dying lymphocytes. The clearance of apoptotic and necrotic cells is known as efferocytosis and is a critical liver function to maintain tissue homeostasis. Much of the research into this form of immunological control has focused on Kupffer cells, the liver-resident macrophages. However, hepatocytes (and other liver resident cells) are competent efferocytes and comprise 80% of the liver mass. Little is known regarding the mechanisms of apoptotic and necrotic cell capture by epithelia, which lack key receptors that mediate phagocytosis in macrophages. Herein, we discuss recent developments that increased our understanding of efferocytosis in tissues, with a special focus on the liver parenchyma. We discuss the impact of efferocytosis in health and in inflammation, highlighting the role of phagocytic epithelia.
• Efferocytosis is a vital process in tissues that can be carried out by multiple cell types, including blood derived and tissue resident phagocytes.
• Hepatocytes are competent efferocytes and play an important role in the clearance of dead cells in health and in inflammation.
• Epithelial cell efferocytosis is understudied and involves distinct mechanisms to professional phagocytes.
• Defects in efferocytosis have been linked to diseases such as autoimmunity, failure to prevent metastasis, failure to limit infection.
• Understanding molecular mechanisms of efferocytosis may reveal new pathways for therapeutic intervention to alleviate inflammation.
Efferocytosis, the clearance of dead and dying cells, is important to prevent tissue damage and promote the resolution of inflammation (1). The liver has evolved into an expert in defusing biochemical threats emanating from food or microbial antigens, which reach the organ along with 75% of its blood supply that arrives through venous blood from the gut. Hepatocytes comprise 80% of liver cells and constitute the biochemical powerhouses of the liver parenchyma, and as a result they often perish in their duties to absorb toxic substances. To cope with loss of hepatic epithelia, the liver has evolved the remarkable ability to regenerate.
To perform their detoxification roles, hepatocytes are strategically organized roughly into two hepatocyte-thick cords, flanked by a thin layer of fenestrated endothelia (Figure 1). Nutrient-rich blood enters the liver via the portal vein and oxygen-rich blood via the hepatic artery, which, together with a bile duct, form the liver portal triad (Figure 1A). Blood from both sources mixes in the specialized hepatic capillaries termed sinusoids, and drains toward the central vein. Hepatocytes near the portal triads (designated zone 1) can be damaged by the inflammatory infiltrate during interface hepatitis, when immune cells cross the sinusoidal endothelia and reach the parenchyma. Zone 2 is found mid-distance from a portal triad and the draining central vein (zone 3). Periportal hepatocytes near zone 1 have access to oxygenated blood from the hepatic artery, and nutrients from the portal blood supply that arrives from the gut. Oxygen and nutrient levels reduce toward the central vein and hepatocytes in zone 3 are found in hypoxic conditions. Fenestrations in the sinusoids allow hepatocytes access to solutes and immune cells reaching through the fenestrations from the circulation (2, 3), but prevent unregulated migration of immune cells to the parenchyma (4).
Figure 1. Organization of liver-resident and recirculating efferocytes. (A) Hepatocytes are spread over three zones, exposed to different levels of oxygen and nutrients. Hepatocytes in zone 1 proximal to the portal triad (portal vein, hepatic artery, bile duct) have access to arterial and venous blood entering the liver through the circulation. Hepatocytes in zone 3 have less access to oxygen and nutrients and are exposed to blood draining into the central vein. Hematoxylin-eosin stain, scale bar represents 50 µm. (B) A plethora of liver resident and recirculating cells are able to engulf apoptotic and necrotic cells and clear them to maintain tissue homeostasis. Kupffer cells, monocytes and macrophages (mϕ) are the best-characterized efferocytes in the liver.
Cells that perish in the sinusoidal spaces are cleared by circulating phagocytes (monocytes, dendritic cells, neutrophils), liver-resident macrophages termed Kupffer cells, and by sinusoidal endothelia (Figure 1B). The best-characterized liver efferocytes are macrophages, both those derived from monocytes infiltrating from the circulation, and the self-renewing populations of Kupffer cells. It is understood that professional phagocytes are activated during injury and adapt their phenotype following the encounter of cellular debris, danger signals, and soluble mediators of the inflammatory milieu. The critical role of liver macrophages including Kupffer cells in the ebb and flow of inflammation was recently reviewed by Tacke’s group (5, 6).
Activated hepatic stellate cells can also engulf apoptotic hepatocytes, which in turn lead to increases in tumor growth factor-β (TGF-β) secretion (7). Biliary epithelial cells (BECs) also take part in efferocytosis of neighboring apoptotic cells; an important adaptation for diseases associated with increased BEC apoptosis such as primary biliary cholangitis (8). The phagocytic activity of hepatocytes was noted in 1992 (9). Hepatocyte efferocytosis assists in parenchymal housekeeping to rapidly dispose of cell remnants and prevent excessive inflammation.
Hepatocyte death from biochemical toxicity (necrosis) occurs in health as part of normal homeostasis, however, liver damage is exacerbated in infection or in alcoholic, drug or ischemia-induced liver injury where large areas of necrotic lesions are evident (Figure 2). Acute-on-chronic liver failure is a syndrome associated with exacerbation of hepatitis B infection (HBV) and characterized by broad areas of hepatic necrosis in cirrhotic patients (Figure 2A). Lymphocyte infiltration is often seen in the parenchyma in chronic liver diseases. Crispe and others have elegantly put forward the “graveyard theory” where the liver is primary site for the disposal of spent immune cells (10). Figure 2B shows hepatic epithelia in the process of engulfing immune cells that have perished in the parenchyma, and this is seen predominantly near the portal regions. Conversely, in cases of acute liver injury such as paracetamol overdose (POD), hepatocyte necrosis due to loss of ATP is noted around the centrilobular regions (zone 3, Figure 2C). Histological features of necrotic hepatocytes include eosinophilic degradation and pyknotic nuclei, which are readily detectable by hematoxylin-eosin staining (inset, Figure 2C).
Figure 2. Hepatocytes engulf necrotic and apoptotic cells in acute-on-chronic liver injury caused by hepatitis B infection (HBV) and in paracetamol injury (POD). (A) Hematoxylin–eosin staining of acute-on-chronic liver injury in a patient with HBV infection. Large areas of hepatocyte necrosis are evident. Inset image shows dark stained hepatocyte nuclei in live hepatocytes (L) and pyknotic or karyolytic nuclei in necrotic hepatocytes (N). (B) Healthy hepatocytes with clearly marked nuclei are seen phagocytosing small apoptotic cells (arrows). Note hepatocyte invaginations which have formed to enable capture of apoptotic cells. (C) Hematoxylin-eosin staining of liver with paracetamol-induced injury, which causes centrilobular necrosis. Inset shows pink cytoplasm in necrotic hepatocytes (N) compared to surviving non-discolored hepatocytes with clearly defined nuclei (L). (D) In situ end labeling (ISEL) of apoptotic cell nuclei is seen here in pink, in a liver with ischemia-reperfusion injury. The marked hepatocyte has a non-apoptotic nucleus seen in blue, and has engulfed an apoptotic cell with a pink nucleus. Neighboring apoptotic hepatocytes can be seen with pink nuclei, and non-apoptotic cells with blue nuclei. The bars show 20 µm.
Hepatocytes also clear away cells that have triggered the molecular cascade of events of programmed cell death (apoptosis) (Figures 2B,D), but can actively destroy live autoreactive immune cells by direct engulfment as noted for CD8+ T cells undergoing suicidal emperipolesis (11). Immune cell death and liver damage are exacerbated in chronic liver inflammation of multiple etiologies, including autoimmune, metabolic, viral, and genetic diseases (12, 13). The rapid processing of dead and dying cells is vital to moderate inflammation (12, 14, 15).
It is remarkable how little we know about the molecular mechanisms that govern the ability of the largest internal organ in the body to mediate the clearance of damaged or dying cells, given that this is one of the liver’s major functions. Herein, we bring together research on hepatocyte efferocytosis and place it into context with current molecular knowledge on the clearance of dead cells by immune phagocytes.
Cells die through a wide array of processes, each situational and requiring their own dedicated cascade of signaling events. The most frequent forms of cell death are attributed to apoptosis or necrosis. Apoptosis, an active form of programmed cell death, is characterized by the initiation of specific inducible pathways (16, 17). This includes the extrinsic pathway; the engagement of extracellular signals, including Fas ligand (FasL) (18) and tumor necrosis factor family cytokines (TNF) (19), amongst others, to their respective death receptors which initiates intracellular death signaling. Apoptosis can also be triggered intrinsically; certain signals, such as a lack of growth factors, endoplasmic reticulum stress or DNA damage, can induce a shift in expression of Bcl-2 family mitochondrial proteins (20). Increased activity of proapoptotic proteins lead to cytochrome C release and caspase 9 activation. Apoptosis pathways result in the activation of effector caspases (3, 6, and 7), which in turn begin to proteolytically degrade the cell’s components. Apoptotic cells are generally smaller than live cells and can be identified by the formation of surface blebs (16).
Necrosis is considered a passive, unprogrammed type of cell death and is often incurred accidentally, although active mechanisms of necrosis have also been reported (21). While multiple mechanisms can induce necrosis, the major causes are attributed to compromising of the plasma membrane, or depletion of energy (22). Furthermore, apoptotic cells can be converted to necrotic cells (also known as secondary necrosis) if ATP levels fall below the quantity required to complete the active apoptotic process (23). The appearance of necrotic cells is often swollen with disrupted organelle and plasma membranes (24). The nucleus is often broken down and will be unstained by hematoxylin (Figure 2). As necrosis often occurs in areas of tissues, rather than the single cell death hallmark of apoptosis, often multiple necrotic cells can be identified in one area. Due to their lack of integrity, necrotic cells will often form cell debris, which can induce liver damage if not cleared swiftly, as we discuss in later sections.
Upon the death of a cell, its corpse must be cleared through efferocytosis. This is a specialist form of phagocytosis, whereby fragments of the dying cells are engulfed by other cells, which in turn degrade and recycle their components. Although both apoptotic and necrotic cells are often captured by the same efferocytes, each are recognized through different means and yield differing response in the predatory cell (25). Apoptotic cells are most commonly recognized through the display of the phospholipid phosphatidylserine (PtdSer) on the outer leaf of the plasma membrane that can be recognized by many receptors [phosphatidylserine receptors (PSRs)] directly (26) or via association with low-density lipoprotein (27, 28). Of note, in a rat liver model, it was shown that recognition of apoptotic cells from mice or humans was reduced compared to rat cells; it is therefore possible that species-specific recognition molecules can mediate efferocytosis (29).
A phenotypic aspect of apoptotic cells is that, although shriveled, the cell remains intact as a singular body. This allows for a clean removal of the dying cells by efferocytes, usually without provoking an inflammatory response. How intact a necrotic cell remains is reflected through the manner in which cell death was induced. As such, multiple modalities for necrotic cell recognition are necessary to guarantee their clearance. Some reports have suggested that necrotic cells can also be recognized by PSRs (30). However, due to the lack of integrity of most necrotic cells, they are often recognized through molecules exposed by necrotic death (25). The same mechanisms are also used to detect pathogens. For example, complement receptors and Fc receptors detect opsonized necrotic cells, and this recognition can trigger signaling events that activate the phagocyte (31–35). As such, necrotic cells are engulfed through the detection of autoantigens, which often increases the risk for autoimmune disease. Necrotic cells can also be indirectly recognized through opsonin engagement of other cellular components. For example, ficolin-2 and -3 have been shown to bind DNA, facilitating the clearance of late-apoptotic/necrotic cells through interactions with calreticulin (33, 36). A ubiquitous mechanism for clearance of necrotic cells remains uncertain.
The differences between recognition, and thus further downstream signaling of apoptotic and necrotic cells, result in conversing consequences for the efferocyte (37). Apoptotic cell clearance generally leads to the production of anti-inflammatory stimuli and pro-resolution signals for inflammation such as interleukin 10 (IL-10) and TGF-β (38). Conversely, necrotic clearance generally results in pro-inflammatory signaling, as many of the recognition receptors are also required for pathogen recognition. In the liver, the signals associated with hepatocyte death were recently reviewed by Brenner and colleagues (39). In this work the importance of the extent and duration of dead cell accumulation was highlighted, as mild and localized cell death can aid regeneration and exert hepatoprotective effects. Equally, prolonged and wide-spread cell death can exacerbate liver injury.
Multiple techniques have been described for both in vivo and in vitro studies of efferocytosis. Fluorescent dye-labeled efferocytes can be “fed” alternatively labeled dead cells under varying conditions and time courses. Early apoptosis can be confirmed by Annexin V labeling of the cell surface as it binds directly to PtdSer, although care must be taken when studying certain activated cell types or using calcium-sensitive protocols (40). Later stages of apoptosis or necrosis are often confirmed with cell impermeable DNA dyes such as 7AAD or TOPRO-3 iodide, which can enter cells once the membrane is compromised. Combined labeling with Annexin V and a membrane-impermeable DNA label was developed to identify the stages of apoptotic cells in more detail (41). Cells can then be assessed by flow cytometry, or imaged by fluorescent microscopy. Complete internalization of dead cells can be confirmed by lack of access to membrane dyes added to the culture media (such as CellMask Plasma Membrane Stains, Thermo Fisher Scientific) or demonstration of efferosome acidification using pH indicator dyes (Figure 3). Quantitative analyses by confocal and time-lapse microscopy can be useful to determine the frequency and kinetic of efferocytosis in vitro.
Figure 3. Visualizing efferocytosis by confocal microscopy. Hepatic epithelia were cocultured with violet-labeled staurosporin-treated apoptotic Jurkat T cells in the presence of pHrodo red, which only fluoresces in conditions of low pH (Thermo Fisher Scientific). CellMask Plasma Membrane stain was added to the culture media to label all exposed cell membranes before imaging. (A) Non-internalized apoptotic cells (blue) attached to hepatocytes were labeled by CellMask Plasma Membrane in white, and they were not labeled by pHrodo red dye (white arrow). (B) Internalized dead cells were not accessible to the membrane dye, confirming internalization (yellow arrow). Complete internalization into an acidic compartment was confirmed by pHrodo red, which detected efferosome acidification as early as 3 hours following engulfment. The scale bar indicates 5 µm.
Fluorescent labeling of dead cells and efferocytes may also be adapted for flow cytometry-based studies, whereby double-positive cells represent efferocytes containing cargo. This form of analysis has been used to study the clearance of neuraminidase-treated red blood cells in mice (42). Other studies opt to analyze efferocytosis using downstream secreted molecules as proxy to utilize alternative techniques such as reporter assays. The capacity of Scavenger Receptor Class F Member 1 (SCARF1) to act as a dead-cell receptor on transfected HEK293T cells, for example, was confirmed using IL-8 mRNA production as a marker of NF-κB activation following apoptotic and necrotic cell efferocytosis (43).
Efferocytosis is not often as straightforward to detect in vivo. Fluorescent labeling can enable temporal measurements in mouse models by intravital imaging of the liver (44), but the technique remains to be adapted successfully for use in human tissues ex vivo. Molecular markers of cell death for use with fixed tissue are often important for the confirmation of efferocytosis. Caspase 3/7 activation or their effects can be measured to delineate apoptotic bodies by immunohistochemistry (IHC) or immunofluorescence (IF) (45). DNA end-labeling is frequently used to confirm the death of cells in tissues. End-labeling involves the addition of labeled nucleotides to DNA breaks induced throughout multiple modalities of death, using a DNA polymerase. This was historically used for in situ end labeling (ISEL) of fixed tissue sections as part of IHC chromagen staining (Figure 2D) (46). This was then adapted for the creation of terminal deoxynucleotidyl transferase (TdT) dUTP Nick-End Labeling (TUNEL) (47) which substitutes a polymerase for TdT. This adaptation allows for the use of many different modified forms of labeled nucleotides (often dUTP), such as non-reactive protein tags or fluorophores. TUNEL staining has been altered to specifically identify cells in late-stage apoptosis.
Further stains for cell membrane proteins or specific nucleic proteins can be used to determine complete engulfment of dead cells. Many of these techniques were exemplified in a recent study of macrophage/monocyte efferocytosis in models of acute liver injury (48). In this work, Antoniades and colleagues studied the mechanism of resolution of liver inflammation through apoptotic cell clearance by macrophages/monocytes via Mer tyrosine kinase receptor (MerTK). Staining for myeloperoxidase (activated neutrophils) combined with TUNEL allowed for the identification of apoptotic neutrophils in human liver, both through IHC and IF staining. Additionally, fluorescent monocytes were cocultured in vitro with alternatively fluorescent apoptotic hepatic cells or neutrophils. The ability of these monocytes to clear apoptotic cells was then assessed through fluorescent microscopy and flow cytometry. Both techniques were used to show the increased capacity of monocytes for dead cell clearance following stimulation with secretory leukocyte protease inhibitor.
Phagocytes express several receptors to recognize and subsequently clear dying cells from the tissues (49–51). In the case of professional phagocytes (e.g., macrophages) multiple apoptotic and necrotic cell receptors have been characterized and these remain relevant in the liver (37, 52) (Table 1). First described in 1992, it is now widely accepted that apoptotic cells are recognized through their expression of PtdSer on the outer leaf of the plasma membrane (53, 54). Several receptors directly recognize PtdSer, many of which are expressed by professional phagocytes (55). These include stabilin-1, stabilin-2, brain-specific angiogenesis inhibitor 1 (BAI1), and RAGE, as well as the TIM family of transmembrane glycoproteins, including TIM-1, -3, and -4 (56–61). Mammary, alveolar and mesangial epithelia recognize apoptotic cells via the PSR, CD36, the vitronectin receptor αvβ3, and CD91 (62–64). Of note, molecules that bind PtdSer such as high-mobility group box 1 (HMGB1) can also downregulate apoptotic cell clearance (65, 66).
It is common for PtdSer to be recognized in complex with certain bridging molecules. Some of the most well-studied PtdSer receptors, the TAM tyrosine kinases (Tyro3, Axl, and MerTK) work in this manner (102); notably, hepatocytes express Axl but not Tyro3 or MerTK (103). The earliest known examples of these are Gas6 and Protein S (104, 105). Gas6 is universally recognized by TAM receptors, whereas Protein S, which is expressed in hepatocytes, is not recognized by Axl. Similarly, integrins αvβ3 and αvβ5 have been shown to promote efferocytosis through the recognition of PtdSer in complex with lactadherin, also known as milk fat globule EGF factor 8 (MFG-E8) (67, 68, 106).
The entirety of apoptotic cell recognition does not lie with the detection of PtdSer expression. It was shown that Tubby protein and its relative Tubby-like protein 1 (TuLP1), which do not bind PtdSer, specifically localize at the surface of apoptotic cells and could act as TAM receptor bridging molecules in a similar manner to Gas6, which in turn promoted apoptotic cell clearance (89). All TAM tyrosine kinases recognized TuLP1, whereas Tubby was exclusively recognized by MerTK on macrophages and retinal pigment cells. Mechanisms of immune surveillance and signaling have also been shown to contribute to apoptotic cell clearance. Components of the complement pathway have been shown to induce phagocytosis in macrophages and DCs by opsonizing apoptotic cells, including C1q and C3 (34, 78, 107). Furthermore, SIGN-R1, a mouse analog of human mannose receptor DC-SIGN, was shown to bind apoptotic cells and induce their labeling with C3 and subsequent clearance by marginal zone macrophages (92).
Recognition of apoptotic cells, although important, is not sufficient for macrophages to engulf and clear them. Downstream intracellular signaling is necessary for load-processing following capture. An important, highly-conserved signaling pathway has been described downstream from most common PtdSer-receptors, involving GTPase Rac1 and ELMO1-DOCK180 interactions (50). TAM-family molecules, αvβ5 integrins and BAI1 act as docks for apoptotic cells, leading to intracellular signaling via this pathway (108, 109). Upon engagement of an apoptotic cell by these receptors, DOCK180 is recruited by ELMO1 (110, 111). In complex, these proteins act as guanine exchange factors, allowing for Rac1 activation, which induces necessary cytoskeletal arrangements required for complete engulfment of the prey cell. Stabilin 1 and 2 have also been shown to activate this pathway through the adaptor protein GULP (109, 112, 113). Completion of apoptotic cell engulfment also commonly involves the activation of nuclear receptors. Loose nucleotides released from dying cells commonly act as “eat-me” signals, and can engage purigenic P2 receptors (P2X and P2Y), leading to an increased capacity for efferocytosis in macrophages (114, 115). It was recently shown that liver X receptor (LXR) was necessary for the capture and processing of apoptotic cells by macrophages and dendritic cells (116, 117). LXR responds to oxysterols found in engulfed apoptotic cells. Stimulation of LXR upregulated MerTK and anti-inflammatory cytokines IL-10 and TGF-β, while also leading to the downregulation of proinflammatory cytokines such as IL-1β, CCL2, and MARCO. A-Gonzalez and Hidalgo reviewed nuclear receptors and their role in macrophage efferocytosis recently (118). LXRα mediates fatty acid regulation in hepatocytes (119), but its role in hepatocyte efferocytosis remains to be determined.
Non-professional phagocytes, such as epithelial cells express multifunctional scavenger receptors, or molecules that exert alternative functions in other cell types. For example, TIM-1, also known as kidney injury molecule 1 (KIM-1), is known to possess multiple immune functions, including CD4+ T-cell and mast cell activation (59). However, TIM-1 was also upregulated in kidney epithelia following injury, allowing for a temporary efferocytic capability (99). Certain cell-exclusive receptors and modulators associated with apoptotic cell clearance have also been described. Apoptotic cell clearance in the liver has been shown to involve asialoglycoprotein receptor (ASGPR) on hepatocytes (96). This ASGPR1 and ASGPR2 complex is critical for receptor-mediated endocytosis of terminally desialylated glycoproteins and is restricted to the liver. Autoantibodies to ASGPR have been found in patients and models of autoimmune hepatitis (120–122). Resolvin D1 is also important in liver protection from ischemia/reperfusion injury, by enhancing efferocytosis by M2-polarizing macrophages (123). Furthermore, phagocytosis by retinal pigment cells, although mostly conducted through MerTK, was shown to be increased through recognition of ATP-binding cassette subfamily F member 1 (ABCF1) released from apoptotic photoreceptor outer segments (124). Overall, it appears that although the broad function of recognition and engulfment of apoptotic cells is conserved throughout many cell types, multiple mechanisms exist that conduct these processes across phagocytes, both homo- and heterotypically.
External stimuli are pertinent for regulation of dead cell clearance by efferocytosis. As such, “find-me” signals released by apoptotic cells are often necessary for the guidance of efferocytes to their prey (53). The best-characterized examples of these are extracellular nucleotides (115). It was shown that upon caspase 3/7 activation in apoptotic cells, ATP and UTP released from apoptotic cells could recruit monocytes/macrophages through recognition by P2Y2. Conversely, molecules with the opposite effect known as “don’t eat-me” signals have also been described. CD47 is the most notable, having been shown to provide resistance to clearance by macrophages on malignant cells and more recently on atherosclerotic plaques (125, 126). Similar “find-me” signals may be utilized by non-motile phagocytes, which extend protrusions to collect apoptotic cells for clearance but are restricted to targets within their tissue niche.
Due to the influence of dying cells on the immune response, cytokine and growth factor stimulation of both professional and non-professional phagocytes can regulate their capacity to clear dead cells. Apoptotic T-cell lymphomas release sphingosine-1-phosphate, a bioactive lipid often involved in immune cell recruitment, leading to the recruitment of macrophages and monocytes (127). Similarly, certain chemokines, tasked with immune cell recruitment have also been shown to increase phagocyte recruitment to areas of apoptotic cells. CX3CL1 (fractalkine) was shown to recruit macrophages to its source, apoptotic Burkitt lymphoma cells (128).
Multiple cytokines have varying effects on efferocytosis (129). Most notably, secretion of IL-3 and IL-14 increased efferocytosis in macrophages through activation of PPAR and increase in CD36 expression (130, 131). IL-4 has been reported to upregulate expression of other PtdSer-receptors such as stabilin 1and 2 (61). IL-10 and TGF-β can also increase efferocytes by macrophages (132, 133). In contrast, pro-inflammatory cytokines reduce the capacity for dead cell engulfment: TNF-α has been shown to inhibit efferocytosis in macrophages (134) and both IFN-γ secretion and receptiveness were reversely correlated with anti-inflammatory cytokines and receptors including IL-4 and TIM receptors (59, 129, 135). However, this was not always the case for these cytokines. Both TNF-α and IFN-γ have been shown to increase LOX-1, which may recognize apoptotic cells by LDL-labeled PtdSer. Furthermore, IFN-γ activation of macrophages, in the absence of other pro-inflammatory stimuli, was shown to increase apoptotic uptake (135).
The ability of phagocytes to clear dead cells is also subject to regulation. This is the result of alterations in gene expression, which can function as negative feedback following initial engulfment of dying cells. For example, it has been shown that macrophages, upon engulfing apoptotic cells can undergo a form of activation and reprograming (136). As well as skewing the macrophage to a more anti-inflammatory phenotype, which in turn promotes inflammatory resolution, both mouse and human macrophages can upregulate CXCR4 during efferocytosis, which in turn encourages their recruitment to draining lymph nodes (137). These macrophages were also shown to subsequently reduce their efferocytosis capacity. Thus, apoptotic cells can reduce local levels of efferocytosis as well as promote them.
More recently macrophages were shown to regulate efferocytosis in surrounding non-circulating phagocytes, such as phagocytic airway epithelial cells (138). In response to IL-4 and IL-13, which are secreted by epithelia and stimulated Th2 cells, macrophages upregulated secretion of both insulin-like growth factor 1 (IGF-1) and microvesicles containing anti-inflammatory signals. Both microvesicles and IGF-1, in turn, fed back to epithelia, causing a reduction of apoptotic cell clearance in favor of microvesicle uptake.
In the context of the liver, some of the mechanisms described for the regulation of efferocytosis apply to circulating and resident macrophages/monocytes. Further, the neuronal guidance protein netrin-1 has been shown to promote resolution of ischemia/reperfusion injury, in part by increasing the capacity of Kupffer cells to engulf apoptotic cells (139). The same molecule was shown to promote liver regeneration (139). In a mouse model of colon carcinoma metastasis in the liver, intercellular cell adhesion molecule 1-deficient macrophages cocultured with tumor cells showed increased efferocytosis dependent on phosphatidylinositol 3 kinase (140).
Environmental factors can also affect phagocytosis, and this extends to the clearance of dead cells; studies in human skin have demonstrated that ethanol can reduce phagocytic function (141), and there have been reports on increased phagocytosis in ethanol-fed rats, which was modulated by diet (142). Hepatocyte phagocytosis of apoptotic cells was decreased in ethanol-fed rats compared to controls, therefore the effects of ethanol on efferocytosis may be cell type-dependent (97). It is unclear whether professional phagocytes play a role in the regulation of efferocytosis by hepatocytes and liver endothelial cells.
Non-professional efferocytes are important throughout all developmental stages of an organism and can take over the clearance of apoptotic cells in the absence of professional phagocytes (143). This was confirmed in PU.1 knockout mice that lack macrophages, and the removal of apoptotic cells required for foot-limb development was instead performed by mesenchymal cells (144). Non-professional phagocytes therefore contribute to efferocytosis, even at the earliest stages of development.
Some of the best-studied phagocytic epithelia are bronchial and alveolar epithelial cells (138, 145, 146). Epithelial cells lining the respiratory tract make first contact with airborne allergens such as house dust mite antigens. Subsequent inflammatory stimuli, including the recruitment of basophils, mast cells and lymphocytes, result in epithelial cell injury. Lung epithelia clear their dying neighbors through PtdSer and Rac1-dependent mechanisms, which can be modified experimentally (146). As with macrophages, apoptotic cell clearance by lung epithelia induced anti-inflammatory cytokines such as IL-10 and TGF-β. Conditional Rac1 deletion in mouse lung epithelia resulted in an exacerbated immune response and greater epithelial damage. These studies demonstrated the efficiency and importance for lung epithelial cell efferocytosis in the regulation of lung inflammation (146, 147).
Retinal epithelial cell efferocytosis has also been well characterized (148–151). Light-sensing cells of the retina are frequently turned over via programmed-cell death, often succumbing to autophagy-associated death, called autolysis (152, 153). Dysregulation of autophagy in these cells has been frequently reported to increase retinal pigment cell death (152, 154). Although a normal part of age-related macular degeneration, failure to clear these dying cells can accelerate retinal damage. Together with professional phagocytes, retinal pigment cells are also charged with the removal of dead cells, in a manner dependent on MerTK.
Throughout the lifecycle of an organism, the removal of immature cells or those with high turnover is necessary to maintain tissue homeostasis. Intravital microscopy has revealed how hair follicles in mice regress through programmed cell death of hair-producing basal epithelial cells (155). Neighboring cells of the same type then clear apoptotic cells through mechanisms requiring TGF-β signaling. In response to kidney damage, epithelial cells recognize and engulf PtdSer-positive apoptotic cells via KIM-1 or TIM-1 (99). Colonic epithelial cells have also been shown to engulf their apoptotic neighbors, which aids in maintaining low levels of inflammation (156).
Studies in multiple progenitor types have recently identified their importance in efferocytosis. Skeletal muscle progenitors recognizing PtdSer on neighboring apoptotic cells, receive the signal to differentiate and fuse into multinuclear myofibers (157). Mesenchymal stem cells take their cues from bone marrow apoptotic cells via efferocytosis and undergo osteogenic differentiation (158). Chondrogenic progenitor cells display macrophage-like abilities in that they react to “find-me” signals from apoptotic cells (159), and non-motile chondrocytes also have a role in efferocytosis (160). As previously discussed, neuronal progenitors which apoptose following failure to complete neural circuits throughout neurogenesis, were recognized and cleared by other progenitor cells via Rac1 activation following ELMO-1 signaling (161). Of note, neuronal and hepatic epithelia can be derived from common progenitor cells.
Through its cardinal role in the neutralization of toxic substances, to its frequent influx and arresting of leukocytes, the liver has evolved to cope well with cell death (10, 162). Although hepatocytes are somewhat resistant to intrinsic apoptotic pathways (163–165), many death receptors are ubiquitously expressed throughout the liver, increasing their susceptibility to extrinsic apoptosis by exposure to pro-inflammatory cytokines such as TNF family molecules including TNF-related apoptosis-inducing ligand (TRAIL) (166–170). Clearance of apoptotic cells by macrophages is a pro-resolution process, however, liver-infiltrating macrophages and Kupffer cells can upregulate death ligands in the liver, including FasL, TNF-α, and TRAIL, increasing the rate of local hepatocyte death and the risk of further inflammation (171, 172). Acute injury such as ischemia and the resulting trauma from hypoxia/reoxygenation can also result in similar sudden increases in necrotic cell death (164, 165, 169). Furthermore, steatosis—accumulation of lipids associated with a multitude of fatty liver diseases—can cause wide hepatocyte cell death via lipoapoptosis induced by ER stress-mediated intrinsic pathways (173, 174). Ethanol-induced injury can also have an impact on receptor-mediated endocytosis by the ASGPR and efferocytosis (175–178).
Failure to clear dead cells from the parenchyma is accumulatively detrimental to the liver; clearance of necrotic cells—both primary and secondary, resulting from uncleared apoptotic cells—results in increase in pro-inflammatory cell influx and cytokine secretion, leading to further damage to the liver (14). HMGB1 is important in liver protection from ischemia/reperfusion injury (179), yet in a sterile model it acted as a damage-associated molecular pattern that enhanced liver injury in both ischemia/reperfusion and POD models (180). Interactions between ASGPR on hepatocytes and B220 epitope of CD45 assist in the capture and trapping of apoptotic cells in the liver (96, 181, 182). The impact of hepatocyte efferocytosis on the inflammatory milieu remains to be established.
Beyond the capacity of hepatocytes for erythrocytosis (183), further evidence or insights into the mechanisms or anti-inflammatory impact of hepatocyte efferocytosis have not been elucidated. Hepatocytes express an array of immunomodulatory cytokines, including TNF-α and IL-10 (184–186); it is not known whether these are modulated during efferocytosis as in lung epithelia and in professional phagocytes. Understanding the molecular mechanisms, purpose and regulation of dead cell clearance by hepatocytes is vital to estimate its impact on the onset and resolution of inflammation, as elevation in hepatocyte apoptosis is key to the pathogenesis and progression of most forms of liver disease (14). Outstanding questions on hepatocyte efferocytosis include:
– What are the molecules that mediate recognition and engulfment of apoptotic and/or necrotic cells by hepatocytes? ASGPR is thus far the only receptor restricted to hepatocyte efferocytosis; despite its multiple roles in receptor-mediated efferocytosis, ASGPR-deficient mice develop normally yet have exacerbated pathology in liver injury models (177, 187).
– Is efferocytosis by hepatocytes in portal and centrilobular regions mediated by the same molecular mechanisms? These regions have differential access to apoptotic and necrotic cells, respectively, as well as nutrient, inflammatory infiltrate and oxygenation levels that may all influence the capacity for efferocytosis.
– How is hepatocyte efferocytosis regulated in health, infection, inflammation and cancer?
– Can hepatocyte efferocytosis be modulated by pharmacological interventions?
– Does efferocytosis affect the ability of hepatocytes to regenerate during injury?
Failure to remove dying cells, both apoptotic and necrotic, have been connected to disease exacerbation (49). Accumulation of dying cells increases the availability of proimmunogenic factors and can increase the risk of autoimmunity, especially as death-recognition becomes skewed to proinflammatory recognition of secondary-necrotic cells. This topic was explored in a recent special issue in Frontiers in Immunology (188).
Defects in efferocytosis have also been shown to be beneficial for the longevity of tumors. Upregulation in the “don’t-eat me” signal CD47 was reported in myeloid leukemia (189, 190) which was associated with increased tumor survival and poorer prognosis. Similar pathogenic consequences of aberrant efferocytosis have been exemplified through deficiencies in death receptors (1). Loss of axl, MerTK, and its associated ligand, Gas6, have all been shown to promote the growth of colon cancers (191, 192). Conversely, loss of stabilin-1 has shown to reduce growth of implanted tumors in knockout mice, due to reduced recruitment of tumor-associated lymphocytes and macrophages (193). As such, loss of death-receptor expression is not always beneficial for cancer vitality. However, loss of other receptors for dying cells has displayed varying phenotypes associated with the lack of apoptotic cell clearance. Loss of SCARF1 and axl has been reported to promote autoimmunity (43, 194).
Similar dangers to those mentioned above regarding deficiencies in dying cell clearance are apparent for many liver diseases. In the context of the liver, the effects of efferocytosis in autoimmune family disorders have not been established directly. Reports on efferocytosis in liver diseases are listed in Table 2. Clearance of dying cells in the liver is thought to reduce the risk of autoimmune hepatitis and promote reversal of fibrosis by macrophages (195, 196). In primary biliary cholangitis, efferocytosis by biliary epithelia may be important in defining the tissue specificity of the autoimmune response (8, 197). It is worth considering that standard of care treatments for autoimmune conditions include corticosteroid regimens, which have been shown to upregulate efferocytosis (49, 198). Prevention of efferocytosis may therefore exacerbate liver diseases.
As well as causing hepatocyte necrosis, chronic alcohol exposure was reported to reduce macrophage efferocytosis through diminishing MFG-E8 expression (209). Prevention of efferocytosis by macrophages in the liver could increase further inflammatory stimuli, although it is not clear how hepatocyte efferocytosis would be affected. Contrarily, reduced efferocytosis in certain disease models has been shown to be beneficial. Loss of the dead-cell receptor TIM4, for example, in a mouse model of ischemia/reperfusion injury reduced immune cell infiltration and hepatocyte damage (210). Understanding the protein-specific and situational benefits or detriments to reduced efferocytosis in diseases of the liver and other organs can give insights into possible therapeutics for tissue damage and autoimmunity.
Recent advances in epithelial cell efferocytosis have highlighted the importance of tissue epithelia in the everyday clearance of billions of apoptotic cells. Compared to professional efferocytes, there is little known regarding the receptors and molecular processes involved in the recognition of apoptotic and necrotic cells by non-professional phagocytes, including molecules that may confer tissue-specific function. Given the impact of efferocytosis on the pathogenesis of autoimmunity, tissue injury and tumor biology (211), molecules driving efficient clearance of dead cells are valid therapeutic targets. Hepatocyte efferocytosis, accomplished at least in part by the liver-restricted ASGPR, is an attractive target for therapeutic intervention for a multitude of liver diseases.
This study was carried out in accordance with the recommendations of LREC 06/Q2708/11, South Birmingham, Birmingham, UK. All subjects gave written informed consent in accordance with the Declaration of Helsinki.
SPD, GMR, and ZS performed stains for immunohistochemistry and immunofluorescence for illustrative purposes, and wrote the manuscript.
This article/paper/report presents independent research funded by the NIHR Birmingham Biomedical Research Centre at the University Hospitals Birmingham NHS Foundation Trust and the University of Birmingham. The views expressed are those of the author(s) and not necessarily those of the NHS, the NIHR or the Department of Health.
The authors declare that the research was conducted in the absence of any commercial or financial relationships that could be construed as a potential conflict of interest.
We thank Janine Fear and Bridget Gunson for excellent laboratory and patient data management, and the staff and patients at the Queen Elizabeth Hospital Liver and Hepatobiliary Unit, Birmingham, UK.
SPD is supported by a PhD studentship from the Medical Research Council (MRC) Centre for Immune Regulation, University of Birmingham, UK. GMR is supported by the National Institute for Health Research (NIHR) Birmingham Liver Biomedical Research Unit, University of Birmingham, UK. ZS is supported by a Royal Society Dorothy Hodgkin Fellowship, the Wellcome Trust Institutional Strategic Support Fund, and MRC Confidence in Concept award.
1. Arandjelovic S, Ravichandran KS. Phagocytosis of apoptotic cells in homeostasis. Nat Immunol (2015) 16:907–17. doi:10.1038/ni.3253
2. Guidotti LG, Inverso D, Sironi L, Di Lucia P, Fioravanti J, Ganzer L, et al. Immunosurveillance of the liver by intravascular effector CD8(+) T cells. Cell (2015) 161:486–500. doi:10.1016/j.cell.2015.03.005
3. Warren A, Le Couteur DG, Fraser R, Bowen DG, McCaughan GW, Bertolino P. T lymphocytes interact with hepatocytes through fenestrations in murine liver sinusoidal endothelial cells. Hepatology (2006) 44:1182–90. doi:10.1002/hep.21378
4. Edwards S, Lalor PF, Nash GB, Rainger GE, Adams DH. Lymphocyte traffic through sinusoidal endothelial cells is regulated by hepatocytes. Hepatology (2005) 41:451–9. doi:10.1002/hep.20585
5. Krenkel O, Tacke F. Liver macrophages in tissue homeostasis and disease. Nat Rev Immunol (2017) 17:306–21. doi:10.1038/nri.2017.11
6. Tacke F. Targeting hepatic macrophages to treat liver diseases. J Hepatol (2017) 66:1300–12. doi:10.1016/j.jhep.2017.02.026
7. Canbay A, Taimr P, Torok N, Higuchi H, Friedman S, Gores GJ. Apoptotic body engulfment by a human stellate cell line is profibrogenic. Lab Invest (2003) 83:655–63. doi:10.1097/01.LAB.0000069036.63405.5C
8. Rong GH, Yang GX, Ando Y, Zhang W, He XS, Leung PS, et al. Human intrahepatic biliary epithelial cells engulf blebs from their apoptotic peers. Clin Exp Immunol (2013) 172:95–103. doi:10.1111/cei.12046
9. Soji T, Murata Y, Ohira A, Nishizono H, Tanaka M, Herbert DC. Evidence that hepatocytes can phagocytize exogenous substances. Anat Rec (1992) 233:543–6. doi:10.1002/ar.1092330408
10. Crispe IN, Dao T, Klugewitz K, Mehal WZ, Metz DP. The liver as a site of T-cell apoptosis: graveyard, or killing field? Immunol Rev (2000) 174:47–62. doi:10.1034/j.1600-0528.2002.017412.x
11. Benseler V, Warren A, Vo M, Holz LE, Tay SS, Le Couteur DG, et al. Hepatocyte entry leads to degradation of autoreactive CD8 T cells. Proc Natl Acad Sci U S A (2011) 108:16735–40. doi:10.1073/pnas.1112251108
12. Guicciardi ME, Malhi H, Mott JL, Gores GJ. Apoptosis and necrosis in the liver. Compr Physiol (2013) 3:977–1010. doi:10.1002/cphy.c120020
13. Malhi H, Gores GJ, Lemasters JJ. Apoptosis and necrosis in the liver: a tale of two deaths? Hepatology (2006) 43:S31–44. doi:10.1002/hep.21062
14. Malhi H, Guicciardi ME, Gores GJ. Hepatocyte death: a clear and present danger. Physiol Rev (2010) 90:1165–94. doi:10.1152/physrev.00061.2009
15. Green DR. The end and after: how dying cells impact the living organism. Immunity (2011) 35:441–4. doi:10.1016/j.immuni.2011.10.003
16. Elmore S. Apoptosis: a review of programmed cell death. Toxicol Pathol (2007) 35:495–516. doi:10.1080/01926230701320337
17. Kerr JF, Wyllie AH, Currie AR. Apoptosis: a basic biological phenomenon with wide-ranging implications in tissue kinetics. Br J Cancer (1972) 26:239–57. doi:10.1038/bjc.1972.33
18. Strasser A, Jost PJ, Nagata S. The many roles of FAS receptor signaling in the immune system. Immunity (2009) 30:180–92. doi:10.1016/j.immuni.2009.01.001
19. Flusberg DA, Sorger PK. Surviving apoptosis: life-death signaling in single cells. Trends Cell Biol (2015) 25:446–58. doi:10.1016/j.tcb.2015.03.003
20. Wong WW, Puthalakath H. Bcl-2 family proteins: the sentinels of the mitochondrial apoptosis pathway. IUBMB Life (2008) 60:390–7. doi:10.1002/iub.51
21. Proskuryakov SY, Gabai VL, Konoplyannikov AG. Necrosis is an active and controlled form of programmed cell death. Biochemistry (Mosc) (2002) 67:387–408. doi:10.1023/A:1015289521275
22. Trump BF, Berezesky IK, Chang SH, Phelps PC. The pathways of cell death: oncosis, apoptosis, and necrosis. Toxicol Pathol (1997) 25:82–8. doi:10.1177/019262339702500116
23. Leist M, Single B, Castoldi AF, Kuhnle S, Nicotera P. Intracellular adenosine triphosphate (ATP) concentration: a switch in the decision between apoptosis and necrosis. J Exp Med (1997) 185:1481–6. doi:10.1084/jem.185.8.1481
24. Majno G, Joris I. Apoptosis, oncosis, and necrosis. An overview of cell death. Am J Pathol (1995) 146:3–15.
25. Poon IK, Hulett MD, Parish CR. Molecular mechanisms of late apoptotic/necrotic cell clearance. Cell Death Differ (2010) 17:381–97. doi:10.1038/cdd.2009.195
26. Li MO, Sarkisian MR, Mehal WZ, Rakic P, Flavell RA. Phosphatidylserine receptor is required for clearance of apoptotic cells. Science (2003) 302:1560–3. doi:10.1126/science.1087621
27. Fernandez-Castaneda A, Arandjelovic S, Stiles TL, Schlobach RK, Mowen KA, Gonias SL, et al. Identification of the low density lipoprotein (LDL) receptor-related protein-1 interactome in central nervous system myelin suggests a role in the clearance of necrotic cell debris. J Biol Chem (2013) 288:4538–48. doi:10.1074/jbc.M112.384693
28. Murphy JE, Tacon D, Tedbury PR, Hadden JM, Knowling S, Sawamura T, et al. LOX-1 scavenger receptor mediates calcium-dependent recognition of phosphatidylserine and apoptotic cells. Biochem J (2006) 393:107–15. doi:10.1042/BJ20051166
29. Chionna A, Panzarini E, Pagliara P, De Luca A, Caforio S, Abbro L, et al. Hepatic clearance of apoptotic lymphocytes: simply removal of waste cells? Eur J Histochem (2003) 47:97–104. doi:10.4081/813
30. Brouckaert G, Kalai M, Krysko DV, Saelens X, Vercammen D, Ndlovu MN, et al. Phagocytosis of necrotic cells by macrophages is phosphatidylserine dependent and does not induce inflammatory cytokine production. Mol Biol Cell (2004) 15:1089–100. doi:10.1091/mbc.E03-09-0668
31. Guilliams M, Bruhns P, Saeys Y, Hammad H, Lambrecht BN. The function of Fcgamma receptors in dendritic cells and macrophages. Nat Rev Immunol (2014) 14:94–108. doi:10.1038/nri3666
32. Fraser DA, Pisalyaput K, Tenner AJ. C1q enhances microglial clearance of apoptotic neurons and neuronal blebs, and modulates subsequent inflammatory cytokine production. J Neurochem (2010) 112:733–43. doi:10.1111/j.1471-4159.2009.06494.x
33. Honore C, Hummelshoj T, Hansen BE, Madsen HO, Eggleton P, Garred P. The innate immune component ficolin 3 (Hakata antigen) mediates the clearance of late apoptotic cells. Arthritis Rheum (2007) 56:1598–607. doi:10.1002/art.22564
34. Ogden CA, deCathelineau A, Hoffmann PR, Bratton D, Ghebrehiwet B, Fadok VA, et al. C1q and mannose binding lectin engagement of cell surface calreticulin and CD91 initiates macropinocytosis and uptake of apoptotic cells. J Exp Med (2001) 194:781–95. doi:10.1084/jem.194.6.781
35. Paidassi H, Tacnet-Delorme P, Verneret M, Gaboriaud C, Houen G, Duus K, et al. Investigations on the C1q-calreticulin-phosphatidylserine interactions yield new insights into apoptotic cell recognition. J Mol Biol (2011) 408:277–90. doi:10.1016/j.jmb.2011.02.029
36. Jensen ML, Honore C, Hummelshoj T, Hansen BE, Madsen HO, Garred P. Ficolin-2 recognizes DNA and participates in the clearance of dying host cells. Mol Immunol (2007) 44:856–65. doi:10.1016/j.molimm.2006.04.002
37. Lawrence T, Willoughby DA, Gilroy DW. Anti-inflammatory lipid mediators and insights into the resolution of inflammation. Nat Rev Immunol (2002) 2:787–95. doi:10.1038/nri915
38. Szondy Z, Sarang Z, Kiss B, Garabuczi E, Koroskenyi K. Anti-inflammatory mechanisms triggered by apoptotic cells during their clearance. Front Immunol (2017) 8:909. doi:10.3389/fimmu.2017.00909
39. Brenner C, Galluzzi L, Kepp O, Kroemer G. Decoding cell death signals in liver inflammation. J Hepatol (2013) 59:583–94. doi:10.1016/j.jhep.2013.03.033
40. Birge RB, Boeltz S, Kumar S, Carlson J, Wanderley J, Calianese D, et al. Phosphatidylserine is a global immunosuppressive signal in efferocytosis, infectious disease, and cancer. Cell Death Differ (2016) 23:962–78. doi:10.1038/cdd.2016.11
41. Jiang L, Tixeira R, Caruso S, Atkin-Smith GK, Baxter AA, Paone S, et al. Monitoring the progression of cell death and the disassembly of dying cells by flow cytometry. Nat Protoc (2016) 11:655–63. doi:10.1038/nprot.2016.028
42. Bratosin D, Estaquier J, Ameisen JC, Aminoff D, Montreuil J. Flow cytometric approach to the study of erythrophagocytosis: evidence for an alternative immunoglobulin-independent pathway in agammaglobulinemic mice. J Immunol Methods (2002) 265:133–43. doi:10.1016/S0022-1759(02)00076-5
43. Ramirez-Ortiz ZG, Pendergraft WF III, Prasad A, Byrne MH, Iram T, Blanchette CJ, et al. The scavenger receptor SCARF1 mediates the clearance of apoptotic cells and prevents autoimmunity. Nat Immunol (2013) 14:917–26. doi:10.1038/ni.2670
44. Grandjean CL, Montalvao F, Celli S, Michonneau D, Breart B, Garcia Z, et al. Intravital imaging reveals improved Kupffer cell-mediated phagocytosis as a mode of action of glycoengineered anti-CD20 antibodies. Sci Rep (2016) 6:34382. doi:10.1038/srep34382
45. Leers MP, Bjorklund V, Bjorklund B, Jornvall H, Nap M. An immunohistochemical study of the clearance of apoptotic cellular fragments. Cell Mol Life Sci (2002) 59:1358–65. doi:10.1007/s00018-002-8513-8
46. Wheeldon EB, Williams SM, Soames AR, James NH, Roberts RA. Quantitation of apoptotic bodies in rat liver by in situ end labelling (ISEL): correlation with morphology. Toxicol Pathol (1995) 23:410–5. doi:10.1177/019262339502300317
47. Kyrylkova K, Kyryachenko S, Leid M, Kioussi C. Detection of apoptosis by TUNEL assay. Methods Mol Biol (2012) 887:41–7. doi:10.1007/978-1-61779-860-3_5
48. Triantafyllou E, Pop OT, Possamai LA, Wilhelm A, Liaskou E, Singanayagam A, et al. MerTK expressing hepatic macrophages promote the resolution of inflammation in acute liver failure. Gut (2018) 67(2):333–47. doi:10.1136/gutjnl-2016-313615
49. Poon IK, Lucas CD, Rossi AG, Ravichandran KS. Apoptotic cell clearance: basic biology and therapeutic potential. Nat Rev Immunol (2014) 14:166–80. doi:10.1038/nri3607
50. Penberthy KK, Ravichandran KS. Apoptotic cell recognition receptors and scavenger receptors. Immunol Rev (2016) 269:44–59. doi:10.1111/imr.12376
51. Armstrong A, Ravichandran KS. Phosphatidylserine receptors: what is the new RAGE? EMBO Rep (2011) 12:287–8. doi:10.1038/embor.2011.41
52. Elliott MR, Ravichandran KS. The dynamics of apoptotic cell clearance. Dev Cell (2016) 38:147–60. doi:10.1016/j.devcel.2016.06.029
53. Ravichandran KS. Find-me and eat-me signals in apoptotic cell clearance: progress and conundrums. J Exp Med (2010) 207:1807–17. doi:10.1084/jem.20101157
54. Fadok VA, Voelker DR, Campbell PA, Cohen JJ, Bratton DL, Henson PM. Exposure of phosphatidylserine on the surface of apoptotic lymphocytes triggers specific recognition and removal by macrophages. J Immunol (1992) 148:2207–16.
55. Medina CB, Ravichandran KS. Do not let death do us part: ‘find-me’ signals in communication between dying cells and the phagocytes. Cell Death Differ (2016) 23:979–89. doi:10.1038/cdd.2016.13
56. Park D, Tosello-Trampont AC, Elliott MR, Lu M, Haney LB, Ma Z, et al. BAI1 is an engulfment receptor for apoptotic cells upstream of the ELMO/Dock180/Rac module. Nature (2007) 450:430–4. doi:10.1038/nature06329
57. Park SY, Kim SY, Jung MY, Bae DJ, Kim IS. Epidermal growth factor-like domain repeat of stabilin-2 recognizes phosphatidylserine during cell corpse clearance. Mol Cell Biol (2008) 28:5288–98. doi:10.1128/MCB.01993-07
58. Park SY, Jung MY, Kim HJ, Lee SJ, Kim SY, Lee BH, et al. Rapid cell corpse clearance by stabilin-2, a membrane phosphatidylserine receptor. Cell Death Differ (2008) 15:192–201. doi:10.1038/sj.cdd.4402242
59. Freeman GJ, Casasnovas JM, Umetsu DT, DeKruyff RH. TIM genes: a family of cell surface phosphatidylserine receptors that regulate innate and adaptive immunity. Immunol Rev (2010) 235:172–89. doi:10.1111/j.0105-2896.2010.00903.x
60. He M, Kubo H, Morimoto K, Fujino N, Suzuki T, Takahasi T, et al. Receptor for advanced glycation end products binds to phosphatidylserine and assists in the clearance of apoptotic cells. EMBO Rep (2011) 12:358–64. doi:10.1038/embor.2011.28
61. Park SY, Jung MY, Lee SJ, Kang KB, Gratchev A, Riabov V, et al. Stabilin-1 mediates phosphatidylserine-dependent clearance of cell corpses in alternatively activated macrophages. J Cell Sci (2009) 122:3365–73. doi:10.1242/jcs.049569
62. Hughes J, Liu Y, Van Damme J, Savill J. Human glomerular mesangial cell phagocytosis of apoptotic neutrophils: mediation by a novel CD36-independent vitronectin receptor/thrombospondin recognition mechanism that is uncoupled from chemokine secretion. J Immunol (1997) 158:4389–97.
63. Sexton DW, Blaylock MG, Walsh GM. Human alveolar epithelial cells engulf apoptotic eosinophils by means of integrin- and phosphatidylserine receptor-dependent mechanisms: a process upregulated by dexamethasone. J Allergy Clin Immunol (2001) 108:962–9. doi:10.1067/mai.2001.119414
64. Monks J, Rosner D, Geske FJ, Lehman L, Hanson L, Neville MC, et al. Epithelial cells as phagocytes: apoptotic epithelial cells are engulfed by mammary alveolar epithelial cells and repress inflammatory mediator release. Cell Death Differ (2005) 12:107–14. doi:10.1038/sj.cdd.4401517
65. Liu G, Wang J, Park YJ, Tsuruta Y, Lorne EF, Zhao X, et al. High mobility group protein-1 inhibits phagocytosis of apoptotic neutrophils through binding to phosphatidylserine. J Immunol (2008) 181:4240–6. doi:10.4049/jimmunol.181.6.4240
66. Banerjee S, de Freitas A, Friggeri A, Zmijewski JW, Liu G, Abraham E. Intracellular HMGB1 negatively regulates efferocytosis. J Immunol (2011) 187:4686–94. doi:10.4049/jimmunol.1101500
67. Andersen MH, Berglund L, Rasmussen JT, Petersen TE. Bovine PAS-6/7 binds alpha v beta 5 integrins and anionic phospholipids through two domains. Biochemistry (1997) 36:5441–6. doi:10.1021/bi963119m
68. Hanayama R, Tanaka M, Miwa K, Shinohara A, Iwamatsu A, Nagata S. Identification of a factor that links apoptotic cells to phagocytes. Nature (2002) 417:182–7. doi:10.1038/417182a
69. Savill J, Dransfield I, Hogg N, Haslett C. Vitronectin receptor-mediated phagocytosis of cells undergoing apoptosis. Nature (1990) 343:170–3. doi:10.1038/343170a0
70. Akakura S, Singh S, Spataro M, Akakura R, Kim JI, Albert ML, et al. The opsonin MFG-E8 is a ligand for the alphavbeta5 integrin and triggers DOCK180-dependent Rac1 activation for the phagocytosis of apoptotic cells. Exp Cell Res (2004) 292:403–16. doi:10.1016/j.yexcr.2003.09.011
71. Albert ML, Kim JI, Birge RB. alphavbeta5 integrin recruits the CrkII-Dock180-rac1 complex for phagocytosis of apoptotic cells. Nat Cell Biol (2000) 2:899–905. doi:10.1038/35046549
72. Albert ML, Pearce SF, Francisco LM, Sauter B, Roy P, Silverstein RL, et al. Immature dendritic cells phagocytose apoptotic cells via alphavbeta5 and CD36, and cross-present antigens to cytotoxic T lymphocytes. J Exp Med (1998) 188:1359–68. doi:10.1084/jem.188.7.1359
73. Lemke G, Burstyn-Cohen T. TAM receptors and the clearance of apoptotic cells. Ann N Y Acad Sci (2010) 1209:23–9. doi:10.1111/j.1749-6632.2010.05744.x
74. Lu Q, Gore M, Zhang Q, Camenisch T, Boast S, Casagranda F, et al. Tyro-3 family receptors are essential regulators of mammalian spermatogenesis. Nature (1999) 398:723–8. doi:10.1038/19554
75. Nagata K, Ohashi K, Nakano T, Arita H, Zong C, Hanafusa H, et al. Identification of the product of growth arrest-specific gene 6 as a common ligand for Axl, Sky, and Mer receptor tyrosine kinases. J Biol Chem (1996) 271:30022–7.
76. Xiong W, Chen Y, Wang H, Wang H, Wu H, Lu Q, et al. Gas6 and the Tyro 3 receptor tyrosine kinase subfamily regulate the phagocytic function of Sertoli cells. Reproduction (2008) 135:77–87. doi:10.1530/REP-07-0287
77. Das S, Sarkar A, Ryan KA, Fox S, Berger AH, Juncadella IJ, et al. Brain angiogenesis inhibitor 1 is expressed by gastric phagocytes during infection with Helicobacter pylori and mediates the recognition and engulfment of human apoptotic gastric epithelial cells. FASEB J (2014) 28:2214–24. doi:10.1096/fj.13-243238
78. Mevorach D, Mascarenhas JO, Gershov D, Elkon KB. Complement-dependent clearance of apoptotic cells by human macrophages. J Exp Med (1998) 188:2313–20. doi:10.1084/jem.188.12.2313
79. Patel PC, Harrison RE. Membrane ruffles capture C3bi-opsonized particles in activated macrophages. Mol Biol Cell (2008) 19:4628–39. doi:10.1091/mbc.E08-02-0223
80. Devitt A, Moffatt OD, Raykundalia C, Capra JD, Simmons DL, Gregory CD. Human CD14 mediates recognition and phagocytosis of apoptotic cells. Nature (1998) 392:505–9. doi:10.1038/33169
81. Devitt A, Pierce S, Oldreive C, Shingler WH, Gregory CD. CD14-dependent clearance of apoptotic cells by human macrophages: the role of phosphatidylserine. Cell Death Differ (2003) 10:371–82. doi:10.1038/sj.cdd.4401168
82. Navazo MD, Daviet L, Savill J, Ren Y, Leung LL, McGregor JL. Identification of a domain (155–183) on CD36 implicated in the phagocytosis of apoptotic neutrophils. J Biol Chem (1996) 271:15381–5. doi:10.1074/jbc.271.26.15381
83. Savill J, Hogg N, Haslett C. Macrophage vitronectin receptor, CD36, and thrombospondin cooperate in recognition of neutrophils undergoing programmed cell death. Chest (1991) 99:6s–7s. doi:10.1378/chest.99.3_Supplement.6S-a
84. Savill J, Hogg N, Ren Y, Haslett C. Thrombospondin cooperates with CD36 and the vitronectin receptor in macrophage recognition of neutrophils undergoing apoptosis. J Clin Invest (1992) 90:1513–22. doi:10.1172/JCI116019
85. Zhang JG, Czabotar PE, Policheni AN, Caminschi I, Wan SS, Kitsoulis S, et al. The dendritic cell receptor Clec9A binds damaged cells via exposed actin filaments. Immunity (2012) 36:646–57. doi:10.1016/j.immuni.2012.03.009
86. Sancho D, Joffre OP, Keller AM, Rogers NC, Martinez D, Hernanz-Falcon P, et al. Identification of a dendritic cell receptor that couples sensing of necrosis to immunity. Nature (2009) 458:899–903. doi:10.1038/nature07750
87. Sambrano GR, Steinberg D. Recognition of oxidatively damaged and apoptotic cells by an oxidized low density lipoprotein receptor on mouse peritoneal macrophages: role of membrane phosphatidylserine. Proc Natl Acad Sci U S A (1995) 92:1396–400. doi:10.1073/pnas.92.5.1396
88. Wermeling F, Chen Y, Pikkarainen T, Scheynius A, Winqvist O, Izui S, et al. Class A scavenger receptors regulate tolerance against apoptotic cells, and autoantibodies against these receptors are predictive of systemic lupus. J Exp Med (2007) 204:2259–65. doi:10.1084/jem.20070600
89. Caberoy NB, Zhou Y, Li W. Tubby and tubby-like protein 1 are new MerTK ligands for phagocytosis. EMBO J (2010) 29:3898–910. doi:10.1038/emboj.2010.265
90. Caberoy NB, Alvarado G, Li W. Tubby regulates microglial phagocytosis through MerTK. J Neuroimmunol (2012) 252:40–8. doi:10.1016/j.jneuroim.2012.07.009
91. Fadok VA, Bratton DL, Rose DM, Pearson A, Ezekewitz RA, Henson PM. A receptor for phosphatidylserine-specific clearance of apoptotic cells. Nature (2000) 405:85–90. doi:10.1038/35011084
92. Prabagar MG, Do Y, Ryu S, Park JY, Choi HJ, Choi WS, et al. SIGN-R1, a C-type lectin, enhances apoptotic cell clearance through the complement deposition pathway by interacting with C1q in the spleen. Cell Death Differ (2013) 20:535–45. doi:10.1038/cdd.2012.160
93. Nakayama M, Akiba H, Takeda K, Kojima Y, Hashiguchi M, Azuma M, et al. Tim-3 mediates phagocytosis of apoptotic cells and cross-presentation. Blood (2009) 113:3821–30. doi:10.1182/blood-2008-10-185884
94. Miyanishi M, Tada K, Koike M, Uchiyama Y, Kitamura T, Nagata S. Identification of Tim4 as a phosphatidylserine receptor. Nature (2007) 450:435–9. doi:10.1038/nature06307
95. Nandrot EF, Anand M, Almeida D, Atabai K, Sheppard D, Finnemann SC. Essential role for MFG-E8 as ligand for alphavbeta5 integrin in diurnal retinal phagocytosis. Proc Natl Acad Sci U S A (2007) 104:12005–10. doi:10.1073/pnas.0704756104
96. Dini L, Autuori F, Lentini A, Oliverio S, Piacentini M. The clearance of apoptotic cells in the liver is mediated by the asialoglycoprotein receptor. FEBS Lett (1992) 296:174–8. doi:10.1016/0014-5793(92)80373-O
97. McVicker BL, Tuma DJ, Kubik JA, Hindemith AM, Baldwin CR, Casey CA. The effect of ethanol on asialoglycoprotein receptor-mediated phagocytosis of apoptotic cells by rat hepatocytes. Hepatology (2002) 36:1478–87. doi:10.1002/hep.1840360625
98. Ryeom SW, Sparrow JR, Silverstein RL. CD36 participates in the phagocytosis of rod outer segments by retinal pigment epithelium. J Cell Sci (1996) 109(Pt 2):387–95.
99. Ichimura T, Asseldonk EJ, Humphreys BD, Gunaratnam L, Duffield JS, Bonventre JV. Kidney injury molecule-1 is a phosphatidylserine receptor that confers a phagocytic phenotype on epithelial cells. J Clin Invest (2008) 118:1657–68. doi:10.1172/JCI34487
100. Oka K, Sawamura T, Kikuta K, Itokawa S, Kume N, Kita T, et al. Lectin-like oxidized low-density lipoprotein receptor 1 mediates phagocytosis of aged/apoptotic cells in endothelial cells. Proc Natl Acad Sci U S A (1998) 95:9535–40. doi:10.1073/pnas.95.16.9535
101. Lee SJ, Park SY, Jung MY, Bae SM, Kim IS. Mechanism for phosphatidylserine-dependent erythrophagocytosis in mouse liver. Blood (2011) 117:5215–23. doi:10.1182/blood-2010-10-313239
102. Lemke G. Biology of the TAM receptors. Cold Spring Harb Perspect Biol (2013) 5:a009076. doi:10.1101/cshperspect.a009076
103. Qi N, Liu P, Zhang Y, Wu H, Chen Y, Han D. Development of a spontaneous liver disease resembling autoimmune hepatitis in mice lacking tyro3, axl and mer receptor tyrosine kinases. PLoS One (2013) 8:e66604. doi:10.1371/journal.pone.0066604
104. Hafizi S, Dahlback B. Gas6 and protein S. Vitamin K-dependent ligands for the Axl receptor tyrosine kinase subfamily. FEBS J (2006) 273:5231–44. doi:10.1111/j.1742-4658.2006.05529.x
105. Stitt TN, Conn G, Gore M, Lai C, Bruno J, Radziejewski C, et al. The anticoagulation factor protein S and its relative, Gas6, are ligands for the Tyro 3/Axl family of receptor tyrosine kinases. Cell (1995) 80:661–70. doi:10.1016/0092-8674(95)90520-0
106. Andersen MH, Graversen H, Fedosov SN, Petersen TE, Rasmussen JT. Functional analyses of two cellular binding domains of bovine lactadherin. Biochemistry (2000) 39:6200–6. doi:10.1021/bi992221r
107. Nauta AJ, Trouw LA, Daha MR, Tijsma O, Nieuwland R, Schwaeble WJ, et al. Direct binding of C1q to apoptotic cells and cell blebs induces complement activation. Eur J Immunol (2002) 32:1726–36. doi:10.1002/1521-4141(200206)32:6<1726::AID-IMMU1726>3.0.CO;2-R
108. Elliott MR, Zheng S, Park D, Woodson RI, Reardon MA, Juncadella IJ, et al. Unexpected requirement for ELMO1 in clearance of apoptotic germ cells in vivo. Nature (2010) 467:333–7. doi:10.1038/nature09356
109. Kim S, Park SY, Kim SY, Bae DJ, Pyo JH, Hong M, et al. Cross talk between engulfment receptors stabilin-2 and integrin alphavbeta5 orchestrates engulfment of phosphatidylserine-exposed erythrocytes. Mol Cell Biol (2012) 32:2698–708. doi:10.1128/MCB.06743-11
110. Gumienny TL, Brugnera E, Tosello-Trampont AC, Kinchen JM, Haney LB, Nishiwaki K, et al. CED-12/ELMO, a novel member of the CrkII/Dock180/Rac pathway, is required for phagocytosis and cell migration. Cell (2001) 107:27–41. doi:10.1016/S0092-8674(01)00520-7
111. Brugnera E, Haney L, Grimsley C, Lu M, Walk SF, Tosello-Trampont AC, et al. Unconventional Rac-GEF activity is mediated through the Dock180-ELMO complex. Nat Cell Biol (2002) 4:574–82. doi:10.1038/ncb824
112. Park SY, Kang KB, Thapa N, Kim SY, Lee SJ, Kim IS. Requirement of adaptor protein GULP during stabilin-2-mediated cell corpse engulfment. J Biol Chem (2008) 283:10593–600. doi:10.1074/jbc.M709105200
113. Park SY, Kim SY, Kang KB, Kim IS. Adaptor protein GULP is involved in stabilin-1-mediated phagocytosis. Biochem Biophys Res Commun (2010) 398:467–72. doi:10.1016/j.bbrc.2010.06.101
114. Marques-da-Silva C, Burnstock G, Ojcius DM, Coutinho-Silva R. Purinergic receptor agonists modulate phagocytosis and clearance of apoptotic cells in macrophages. Immunobiology (2011) 216:1–11. doi:10.1016/j.imbio.2010.03.010
115. Elliott MR, Chekeni FB, Trampont PC, Lazarowski ER, Kadl A, Walk SF, et al. Nucleotides released by apoptotic cells act as a find-me signal to promote phagocytic clearance. Nature (2009) 461:282–6. doi:10.1038/nature08296
116. A-Gonzalez N, Bensinger SJ, Hong C, Beceiro S, Bradley MN, Zelcer N, et al. Apoptotic cells promote their own clearance and immune tolerance through activation of the nuclear receptor LXR. Immunity (2009) 31:245–58. doi:10.1016/j.immuni.2009.06.018
117. Chen Q, Chen J, Chen J, Lu XJ. Molecular and functional characterization of liver X receptor in ayu, Plecoglossus altivelis: regulator of inflammation and efferocytosis. Dev Comp Immunol (2016) 65:358–68. doi:10.1016/j.dci.2016.08.007
118. A-Gonzalez N, Hidalgo A. Nuclear receptors and clearance of apoptotic cells: stimulating the macrophage’s appetite. Front Immunol (2014) 5:211. doi:10.3389/fimmu.2014.00211
119. Pawar A, Botolin D, Mangelsdorf DJ, Jump DB. The role of liver X receptor-alpha in the fatty acid regulation of hepatic gene expression. J Biol Chem (2003) 278:40736–43. doi:10.1074/jbc.M307973200
120. Diao J, Michalak TI. Composition, antigenic properties and hepatocyte surface expression of the woodchuck asialoglycoprotein receptor. J Recept Signal Transduct Res (1996) 16:243–71. doi:10.3109/10799899609039951
121. Poralla T, Treichel U, Lohr H, Fleischer B. The asialoglycoprotein receptor as target structure in autoimmune liver diseases. Semin Liver Dis (1991) 11:215–22. doi:10.1055/s-2008-1040439
122. Treichel U, Poralla T, Hess G, Manns M, Meyer zum Büschenfelde KH. Autoantibodies to human asialoglycoprotein receptor in autoimmune-type chronic hepatitis. Hepatology (1990) 11:606–12. doi:10.1002/hep.1840110413
123. Kang JW, Lee SM. Resolvin D1 protects the liver from ischemia/reperfusion injury by enhancing M2 macrophage polarization and efferocytosis. Biochim Biophys Acta (2016) 1861:1025–35. doi:10.1016/j.bbalip.2016.06.002
124. Guo F, Ding Y, Caberoy N, Alvarado G, Wang F, Chen R, et al. ABCF1 extrinsically regulates retinal pigment epithelial cell phagocytosis. Mol Biol Cell (2015) 26:2311–20. doi:10.1091/mbc.E14-09-1343
125. Kojima Y, Volkmer JP, McKenna K, Civelek M, Lusis AJ, Miller CL, et al. CD47-blocking antibodies restore phagocytosis and prevent atherosclerosis. Nature (2016) 536:86–90. doi:10.1038/nature18935
126. Majeti R, Chao MP, Alizadeh AA, Pang WW, Jaiswal S, Gibbs KD Jr, et al. CD47 is an adverse prognostic factor and therapeutic antibody target on human acute myeloid leukemia stem cells. Cell (2009) 138:286–99. doi:10.1016/j.cell.2009.05.045
127. Gude DR, Alvarez SE, Paugh SW, Mitra P, Yu J, Griffiths R, et al. Apoptosis induces expression of sphingosine kinase 1 to release sphingosine-1-phosphate as a “come-and-get-me” signal. FASEB J (2008) 22:2629–38. doi:10.1096/fj.08-107169
128. Truman LA, Ford CA, Pasikowska M, Pound JD, Wilkinson SJ, Dumitriu IE, et al. CX3CL1/fractalkine is released from apoptotic lymphocytes to stimulate macrophage chemotaxis. Blood (2008) 112:5026–36. doi:10.1182/blood-2008-06-162404
129. Korns D, Frasch SC, Fernandez-Boyanapalli R, Henson PM, Bratton DL. Modulation of macrophage efferocytosis in inflammation. Front Immunol (2011) 2:57. doi:10.3389/fimmu.2011.00057
130. Szanto A, Balint BL, Nagy ZS, Barta E, Dezso B, Pap A, et al. STAT6 transcription factor is a facilitator of the nuclear receptor PPARgamma-regulated gene expression in macrophages and dendritic cells. Immunity (2010) 33:699–712. doi:10.1016/j.immuni.2010.11.009
131. Berry A, Balard P, Coste A, Olagnier D, Lagane C, Authier H, et al. IL-13 induces expression of CD36 in human monocytes through PPARgamma activation. Eur J Immunol (2007) 37:1642–52. doi:10.1002/eji.200636625
132. Xu W, Roos A, Schlagwein N, Woltman AM, Daha MR, van Kooten C. IL-10-producing macrophages preferentially clear early apoptotic cells. Blood (2006) 107:4930–7. doi:10.1182/blood-2005-10-4144
133. Freire-de-Lima CG, Xiao YQ, Gardai SJ, Bratton DL, Schiemann WP, Henson PM. Apoptotic cells, through transforming growth factor-beta, coordinately induce anti-inflammatory and suppress pro-inflammatory eicosanoid and NO synthesis in murine macrophages. J Biol Chem (2006) 281:38376–84. doi:10.1074/jbc.M605146200
134. McPhillips K, Janssen WJ, Ghosh M, Byrne A, Gardai S, Remigio L, et al. TNF-alpha inhibits macrophage clearance of apoptotic cells via cytosolic phospholipase A2 and oxidant-dependent mechanisms. J Immunol (2007) 178:8117–26. doi:10.4049/jimmunol.178.12.8117
135. Fernandez-Boyanapalli RF, Frasch SC, McPhillips K, Vandivier RW, Harry BL, Riches DW, et al. Impaired apoptotic cell clearance in CGD due to altered macrophage programming is reversed by phosphatidylserine-dependent production of IL-4. Blood (2009) 113:2047–55. doi:10.1182/blood-2008-05-160564
136. Ariel A, Serhan CN. New lives given by cell death: macrophage differentiation following their encounter with apoptotic leukocytes during the resolution of inflammation. Front Immunol (2012) 3:4. doi:10.3389/fimmu.2012.00004
137. Angsana J, Chen J, Liu L, Haller CA, Chaikof EL. Efferocytosis as a regulator of macrophage chemokine receptor expression and polarization. Eur J Immunol (2016) 46:1592–9. doi:10.1002/eji.201546262
138. Han CZ, Juncadella IJ, Kinchen JM, Buckley MW, Klibanov AL, Dryden K, et al. Macrophages redirect phagocytosis by non-professional phagocytes and influence inflammation. Nature (2016) 539:570–4. doi:10.1038/nature20141
139. Schlegel M, Kohler D, Korner A, Granja T, Straub A, Giera M, et al. The neuroimmune guidance cue netrin-1 controls resolution programs and promotes liver regeneration. Hepatology (2016) 63:1689–705. doi:10.1002/hep.28347
140. Yang M, Liu J, Piao C, Shao J, Du J. ICAM-1 suppresses tumor metastasis by inhibiting macrophage M2 polarization through blockade of efferocytosis. Cell Death Dis (2015) 6:e1780. doi:10.1038/cddis.2015.144
141. Schopf RE, Trompeter M, Bork K, Morsches B. Effects of ethanol and acetaldehyde on phagocytic functions. Arch Dermatol Res (1985) 277:131–7. doi:10.1007/BF00414111
142. Earnest DL, Abril ER, Jolley CS, Martinez F. Ethanol and diet-induced alterations in Kupffer cell function. Alcohol Alcohol (1993) 28:73–83.
143. Schwegler M, Wirsing AM, Dollinger AJ, Abendroth B, Putz F, Fietkau R, et al. Clearance of primary necrotic cells by non-professional phagocytes. Biol Cell (2015) 107(10):372–87. doi:10.1111/boc.201400090
144. Wood W, Turmaine M, Weber R, Camp V, Maki RA, McKercher SR, et al. Mesenchymal cells engulf and clear apoptotic footplate cells in macrophageless PU.1 null mouse embryos. Development (2000) 127:5245–52.
145. Fond AM, Lee CS, Schulman IG, Kiss RS, Ravichandran KS. Apoptotic cells trigger a membrane-initiated pathway to increase ABCA1. J Clin Invest (2015) 125:2748–58. doi:10.1172/JCI80300
146. Juncadella IJ, Kadl A, Sharma AK, Shim YM, Hochreiter-Hufford A, Borish L, et al. Apoptotic cell clearance by bronchial epithelial cells critically influences airway inflammation. Nature (2013) 493:547–51. doi:10.1038/nature11714
147. Penberthy KK, Juncadella IJ, Ravichandran KS. Apoptosis and engulfment by bronchial epithelial cells. Implications for allergic airway inflammation. Ann Am Thorac Soc (2014) 11(Suppl 5):S259–62. doi:10.1513/AnnalsATS.201405-200AW
148. Burstyn-Cohen T, Lew ED, Traves PG, Burrola PG, Hash JC, Lemke G. Genetic dissection of TAM receptor-ligand interaction in retinal pigment epithelial cell phagocytosis. Neuron (2012) 76:1123–32. doi:10.1016/j.neuron.2012.10.015
149. Szatmari-Toth M, Kristof E, Vereb Z, Akhtar S, Facsko A, Fesus L, et al. Clearance of autophagy-associated dying retinal pigment epithelial cells – a possible source for inflammation in age-related macular degeneration. Cell Death Dis (2016) 7:e2367. doi:10.1038/cddis.2016.133
150. Petrovski G, Berenyi E, Moe MC, Vajas A, Fesus L, Berta A, et al. Clearance of dying ARPE-19 cells by professional and nonprofessional phagocytes in vitro—implications for age-related macular degeneration (AMD). Acta Ophthalmol (2011) 89:e30–4. doi:10.1111/j.1755-3768.2010.02047.x
151. Irschick EU, Sgonc R, Bock G, Wolf H, Fuchs D, Nussbaumer W, et al. Retinal pigment epithelial phagocytosis and metabolism differ from those of macrophages. Ophthalmic Res (2004) 36:200–10. doi:10.1159/000078778
152. Kaarniranta K, Sinha D, Blasiak J, Kauppinen A, Vereb Z, Salminen A, et al. Autophagy and heterophagy dysregulation leads to retinal pigment epithelium dysfunction and development of age-related macular degeneration. Autophagy (2013) 9:973–84. doi:10.4161/auto.24546
153. Kevany BM, Palczewski K. Phagocytosis of retinal rod and cone photoreceptors. Physiology (Bethesda) (2010) 25:8–15. doi:10.1152/physiol.00038.2009
154. Mitter SK, Song C, Qi X, Mao H, Rao H, Akin D, et al. Dysregulated autophagy in the RPE is associated with increased susceptibility to oxidative stress and AMD. Autophagy (2014) 10:1989–2005. doi:10.4161/auto.36184
155. Mesa KR, Rompolas P, Zito G, Myung P, Sun TY, Brown S, et al. Niche-induced cell death and epithelial phagocytosis regulate hair follicle stem cell pool. Nature (2015) 522:94–7. doi:10.1038/nature14306
156. Lee CS, Penberthy KK, Wheeler KM, Juncadella IJ, Vandenabeele P, Lysiak JJ, et al. Boosting apoptotic cell clearance by colonic epithelial cells attenuates inflammation in vivo. Immunity (2016) 44:807–20. doi:10.1016/j.immuni.2016.02.005
157. Hochreiter-Hufford AE, Arandjelovic S, Ravichandran KS. Using phosphatidylserine exposure on apoptotic cells to stimulate myoblast fusion. Methods Mol Biol (2015) 1313:141–8. doi:10.1007/978-1-4939-2703-6_10
158. Tso GH, Law HK, Tu W, Chan GC, Lau YL. Phagocytosis of apoptotic cells modulates mesenchymal stem cells osteogenic differentiation to enhance IL-17 and RANKL expression on CD4+ T cells. Stem Cells (2010) 28:939–54. doi:10.1002/stem.406
159. Seol D, McCabe DJ, Choe H, Zheng H, Yu Y, Jang K, et al. Chondrogenic progenitor cells respond to cartilage injury. Arthritis Rheum (2012) 64:3626–37. doi:10.1002/art.34613
160. Jiao K, Zhang J, Zhang M, Wei Y, Wu Y, Qiu ZY, et al. The identification of CD163 expressing phagocytic chondrocytes in joint cartilage and its novel scavenger role in cartilage degradation. PLoS One (2013) 8:e53312. doi:10.1371/journal.pone.0053312
161. Lu Z, Elliott MR, Chen Y, Walsh JT, Klibanov AL, Ravichandran KS, et al. Phagocytic activity of neuronal progenitors regulates adult neurogenesis. Nat Cell Biol (2011) 13:1076–83. doi:10.1038/ncb2299
162. Crispe IN. Hepatic T cells and liver tolerance. Nat Rev Immunol (2003) 3:51–62. doi:10.1038/nri981
163. Humphreys EH, Williams KT, Adams DH, Afford SC. Primary and malignant cholangiocytes undergo CD40 mediated Fas dependent apoptosis, but are insensitive to direct activation with exogenous Fas ligand. PLoS One (2010) 5:e14037. doi:10.1371/journal.pone.0014037
164. Bhogal RH, Weston CJ, Curbishley SM, Bhatt AN, Adams DH, Afford SC. Variable responses of small and large human hepatocytes to hypoxia and hypoxia/reoxygenation (H-R). FEBS Lett (2011) 585:935–41. doi:10.1016/j.febslet.2011.02.030
165. Bhogal RH, Weston CJ, Curbishley SM, Adams DH, Afford SC. Autophagy: a cyto-protective mechanism which prevents primary human hepatocyte apoptosis during oxidative stress. Autophagy (2012) 8:545–58. doi:10.4161/auto.19012
166. Faubion WA, Gores GJ. Death receptors in liver biology and pathobiology. Hepatology (1999) 29:1–4. doi:10.1002/hep.510290101
167. Afford SC, Hubscher S, Strain AJ, Adams DH, Neuberger JM. Apoptosis in the human liver during allograft rejection and end-stage liver disease. J Pathol (1995) 176:373–80. doi:10.1002/path.1711760408
168. Afford SC, Randhawa S, Eliopoulos AG, Hubscher SG, Young LS, Adams DH. CD40 activation induces apoptosis in cultured human hepatocytes via induction of cell surface fas ligand expression and amplifies fas-mediated hepatocyte death during allograft rejection. J Exp Med (1999) 189:441–6. doi:10.1084/jem.189.2.441
169. Bhogal RH, Curbishley SM, Weston CJ, Adams DH, Afford SC. Reactive oxygen species mediate human hepatocyte injury during hypoxia/reoxygenation. Liver Transpl (2010) 16:1303–13. doi:10.1002/lt.22157
170. Bhogal RH, Weston CJ, Curbishley SM, Adams DH, Afford SC. Activation of CD40 with platelet derived CD154 promotes reactive oxygen species dependent death of human hepatocytes during hypoxia and reoxygenation. PLoS One (2012) 7:e30867. doi:10.1371/journal.pone.0030867
171. Kiener PA, Davis PM, Starling GC, Mehlin C, Klebanoff SJ, Ledbetter JA, et al. Differential induction of apoptosis by Fas-Fas ligand interactions in human monocytes and macrophages. J Exp Med (1997) 185:1511–6. doi:10.1084/jem.185.8.1511
172. Canbay A, Feldstein AE, Higuchi H, Werneburg N, Grambihler A, Bronk SF, et al. Kupffer cell engulfment of apoptotic bodies stimulates death ligand and cytokine expression. Hepatology (2003) 38:1188–98. doi:10.1053/jhep.2003.50472
173. Wei Y, Wang D, Topczewski F, Pagliassotti MJ. Saturated fatty acids induce endoplasmic reticulum stress and apoptosis independently of ceramide in liver cells. Am J Physiol Endocrinol Metab (2006) 291:E275–81. doi:10.1152/ajpendo.00644.2005
174. Wang D, Wei Y, Pagliassotti MJ. Saturated fatty acids promote endoplasmic reticulum stress and liver injury in rats with hepatic steatosis. Endocrinology (2006) 147:943–51. doi:10.1210/en.2005-0570
175. McVicker BL, Casey CA. Ethanol-impaired hepatic protein trafficking: concepts from the asialoglycoprotein receptor system. Clin Biochem (1999) 32:557–61. doi:10.1016/S0009-9120(99)00055-7
176. McVicker BL, Casey CA. Effects of ethanol on receptor-mediated endocytosis in the liver. Alcohol (1999) 19:255–60. doi:10.1016/S0741-8329(99)00043-9
177. Lee SM, Casey CA, McVicker BL. Impact of asialoglycoprotein receptor deficiency on the development of liver injury. World J Gastroenterol (2009) 15:1194–200. doi:10.3748/wjg.15.1194
178. Casey CA, Lee SM, Aziz-Seible R, McVicker BL. Impaired receptor-mediated endocytosis: its role in alcohol-induced apoptosis. J Gastroenterol Hepatol (2008) 23(Suppl 1):S46–9. doi:10.1111/j.1440-1746.2007.05275.x
179. Huang H, Nace GW, McDonald KA, Tai S, Klune JR, Rosborough BR, et al. Hepatocyte-specific high-mobility group box 1 deletion worsens the injury in liver ischemia/reperfusion: a role for intracellular high-mobility group box 1 in cellular protection. Hepatology (2014) 59:1984–97. doi:10.1002/hep.26976
180. Huebener P, Pradere JP, Hernandez C, Gwak GY, Caviglia JM, Mu X, et al. The HMGB1/RAGE axis triggers neutrophil-mediated injury amplification following necrosis. J Clin Invest (2015) 125:539–50. doi:10.1172/JCI76887
181. Dalton SR, Wiegert RL, Baldwin CR, Kassel KM, Casey CA. Impaired receptor-mediated endocytosis by the asialoglycoprotein receptor in ethanol-fed mice: implications for studying the role of this receptor in alcoholic apoptosis. Biochem Pharmacol (2003) 65:535–43. doi:10.1016/S0006-2952(02)01555-1
182. Dini L, Pagliara P, Carla EC. Phagocytosis of apoptotic cells by liver: a morphological study. Microsc Res Tech (2002) 57:530–40. doi:10.1002/jemt.10107
183. Rosin A, Doljanski L. Erythrocytes in the cytoplsm and nuclei of liver cells. Br J Exp Pathol (1944) 25:111–5.
184. Alfrey EJ, Most D, Wang X, Lee LK, Holm B, Krieger NR, et al. Interferon-gamma and interleukin-10 messenger RNA are up-regulated after orthotopic liver transplantation in tolerant rats: evidence for cytokine-mediated immune dysregulation. Surgery (1995) 118:399–404; discussion 404–5. doi:10.1016/S0039-6060(05)80351-4
185. Rowell DL, Eckmann L, Dwinell MB, Carpenter SP, Raucy JL, Yang SK, et al. Human hepatocytes express an array of proinflammatory cytokines after agonist stimulation or bacterial invasion. Am J Physiol (1997) 273:G322–32.
186. Stonans I, Stonane E, Russwurm S, Deigner HP, Bohm KJ, Wiederhold M, et al. HepG2 human hepatoma cells express multiple cytokine genes. Cytokine (1999) 11:151–6. doi:10.1006/cyto.1998.0366
187. Dalton SR, Lee SM, King RN, Nanji AA, Kharbanda KK, Casey CA, et al. Carbon tetrachloride-induced liver damage in asialoglycoprotein receptor-deficient mice. Biochem Pharmacol (2009) 77:1283–90. doi:10.1016/j.bcp.2008.12.023
188. Munoz LE, Berens C, Lauber K, Gaipl US, Herrmann M. Apoptotic cell clearance and its role in the origin and resolution of chronic inflammation. Front Immunol (2015) 6:139. doi:10.3389/fimmu.2015.00139
189. Jaiswal S, Jamieson CH, Pang WW, Park CY, Chao MP, Majeti R, et al. CD47 is upregulated on circulating hematopoietic stem cells and leukemia cells to avoid phagocytosis. Cell (2009) 138:271–85. doi:10.1016/j.cell.2009.05.046
190. Chao MP, Alizadeh AA, Tang C, Myklebust JH, Varghese B, Gill S, et al. Anti-CD47 antibody synergizes with rituximab to promote phagocytosis and eradicate non-Hodgkin lymphoma. Cell (2010) 142:699–713. doi:10.1016/j.cell.2010.07.044
191. Bosurgi L, Bernink JH, Delgado Cuevas V, Gagliani N, Joannas L, Schmid ET, et al. Paradoxical role of the proto-oncogene Axl and Mer receptor tyrosine kinases in colon cancer. Proc Natl Acad Sci U S A (2013) 110:13091–6. doi:10.1073/pnas.1302507110
192. Akitake-Kawano R, Seno H, Nakatsuji M, Kimura Y, Nakanishi Y, Yoshioka T, et al. Inhibitory role of Gas6 in intestinal tumorigenesis. Carcinogenesis (2013) 34:1567–74. doi:10.1093/carcin/bgt069
193. Karikoski M, Marttila-Ichihara F, Elima K, Rantakari P, Hollmen M, Kelkka T, et al. Clever-1/stabilin-1 controls cancer growth and metastasis. Clin Cancer Res (2014) 20:6452–64. doi:10.1158/1078-0432.CCR-14-1236
194. Weinger JG, Brosnan CF, Loudig O, Goldberg MF, Macian F, Arnett HA, et al. Loss of the receptor tyrosine kinase Axl leads to enhanced inflammation in the CNS and delayed removal of myelin debris during experimental autoimmune encephalomyelitis. J Neuroinflammation (2011) 8:49. doi:10.1186/1742-2094-8-49
195. Czaja AJ. Targeting apoptosis in autoimmune hepatitis. Dig Dis Sci (2014) 59:2890–904. doi:10.1007/s10620-014-3284-2
196. Popov Y, Sverdlov DY, Bhaskar KR, Sharma AK, Millonig G, Patsenker E, et al. Macrophage-mediated phagocytosis of apoptotic cholangiocytes contributes to reversal of experimental biliary fibrosis. Am J Physiol Gastrointest Liver Physiol (2010) 298:G323–34. doi:10.1152/ajpgi.00394.2009
197. Allina J, Hu B, Sullivan DM, Fiel MI, Thung SN, Bronk SF, et al. T cell targeting and phagocytosis of apoptotic biliary epithelial cells in primary biliary cirrhosis. J Autoimmun (2006) 27:232–41. doi:10.1016/j.jaut.2006.11.004
198. Garabuczi E, Sarang Z, Szondy Z. Glucocorticoids enhance prolonged clearance of apoptotic cells by upregulating liver X receptor, peroxisome proliferator-activated receptor-delta and UCP2. Biochim Biophys Acta (2015) 1853:573–82. doi:10.1016/j.bbamcr.2014.12.014
199. Sasaki M, Kakuda Y, Miyakoshi M, Sato Y, Nakanuma Y. Infiltration of inflammatory cells expressing mitochondrial proteins around bile ducts and in biliary epithelial layer may be involved in the pathogenesis in primary biliary cirrhosis. J Clin Pathol (2014) 67:470–6. doi:10.1136/jclinpath-2013-201917
200. Lleo A, Selmi C, Invernizzi P, Podda M, Coppel RL, Mackay IR, et al. Apotopes and the biliary specificity of primary biliary cirrhosis. Hepatology (2009) 49:871–9. doi:10.1002/hep.22736
201. Webb GJ, Hirschfield GM. Primary biliary cholangitis in 2016: high-definition PBC: biology, models and therapeutic advances. Nat Rev Gastroenterol Hepatol (2017) 14:76–8. doi:10.1038/nrgastro.2016.201
202. Karlsen TH, Folseraas T, Thorburn D, Vesterhus M. Primary sclerosing cholangitis – a comprehensive review. J Hepatol (2017) 67(6):1298–323. doi:10.1016/j.jhep.2017.07.022
203. McVicker BL, Thiele GM, Tuma DJ, Casey CA. Hepatocyte-mediated cytotoxicity and host defense mechanisms in the alcohol-injured liver. Hepatol Int (2014) 8(Suppl 2):432–8. doi:10.1007/s12072-013-9511-7
204. Casey CA, McVicker BL, Donohue TM Jr, McFarland MA, Wiegert RL, Nanji AA. Liver asialoglycoprotein receptor levels correlate with severity of alcoholic liver damage in rats. J Appl Physiol (2004) 96:76–80. doi:10.1152/japplphysiol.00375.2003
205. Lopategi A, Lopez-Vicario C, Alcaraz-Quiles J, Garcia-Alonso V, Rius B, Titos E, et al. Role of bioactive lipid mediators in obese adipose tissue inflammation and endocrine dysfunction. Mol Cell Endocrinol (2016) 419:44–59. doi:10.1016/j.mce.2015.09.033
206. Lopez-Vicario C, Rius B, Alcaraz-Quiles J, Garcia-Alonso V, Lopategi A, Titos E, et al. Pro-resolving mediators produced from EPA and DHA: overview of the pathways involved and their mechanisms in metabolic syndrome and related liver diseases. Eur J Pharmacol (2016) 785:133–43. doi:10.1016/j.ejphar.2015.03.092
207. Titos E, Rius B, Lopez-Vicario C, Alcaraz-Quiles J, Garcia-Alonso V, Lopategi A, et al. Signaling and immunoresolving actions of resolvin D1 in inflamed human visceral adipose tissue. J Immunol (2016) 197:3360–70. doi:10.4049/jimmunol.1502522
208. Serban KA, Petrusca DN, Mikosz A, Poirier C, Lockett AD, Saint L, et al. Alpha-1 antitrypsin supplementation improves alveolar macrophages efferocytosis and phagocytosis following cigarette smoke exposure. PLoS One (2017) 12:e0176073. doi:10.1371/journal.pone.0176073
209. Wang X, Bu HF, Zhong W, Asai A, Zhou Z, Tan XD. MFG-E8 and HMGB1 are involved in the mechanism underlying alcohol-induced impairment of macrophage efferocytosis. Mol Med (2013) 19:170–82. doi:10.2119/molmed.2012.00260
210. Ji H, Liu Y, Zhang Y, Shen XD, Gao F, Busuttil RW, et al. T-cell immunoglobulin and mucin domain 4 (TIM-4) signaling in innate immune-mediated liver ischemia-reperfusion injury. Hepatology (2014) 60:2052–64. doi:10.1002/hep.27334
Keywords: efferocytosis, phagocytosis, liver, hepatocytes, regeneration, apoptosis, necrosis, cell death
Citation: Davies SP, Reynolds GM and Stamataki Z (2018) Clearance of Apoptotic Cells by Tissue Epithelia: A Putative Role for Hepatocytes in Liver Efferocytosis. Front. Immunol. 9:44. doi: 10.3389/fimmu.2018.00044
Received: 16 October 2017; Accepted: 08 January 2018;
Published: 25 January 2018
Edited by:
Amiram Ariel, University of Haifa, IsraelReviewed by:
Christina Janko, Universitätsklinikum Erlangen, GermanyCopyright: © 2018 Davies, Reynolds and Stamataki. This is an open-access article distributed under the terms of the Creative Commons Attribution License (CC BY). The use, distribution or reproduction in other forums is permitted, provided the original author(s) or licensor are credited and that the original publication in this journal is cited, in accordance with accepted academic practice. No use, distribution or reproduction is permitted which does not comply with these terms.
*Correspondence: Zania Stamataki, ei5zdGFtYXRha2lAYmhhbS5hYy51aw==
Disclaimer: All claims expressed in this article are solely those of the authors and do not necessarily represent those of their affiliated organizations, or those of the publisher, the editors and the reviewers. Any product that may be evaluated in this article or claim that may be made by its manufacturer is not guaranteed or endorsed by the publisher.
Research integrity at Frontiers
Learn more about the work of our research integrity team to safeguard the quality of each article we publish.