- 1Faculty of Biosciences, Department of Biochemistry and Molecular Biology, Universitat Autònoma de Barcelona, Cerdanyola del Vallès, Spain
- 2Mycobacteria Research Laboratory, Department of Biological Sciences, Institute of Structural and Molecular Biology, Birkbeck University of London, London, United Kingdom
Tuberculosis (TB) continues to be a devastating infectious disease and remerges as a global health emergency due to an alarming rise of antimicrobial resistance to its treatment. Despite of the serious effort that has been applied to develop effective antitubercular chemotherapies, the potential of antimicrobial peptides (AMPs) remains underexploited. A large amount of literature is now accessible on the AMP mechanisms of action against a diversity of pathogens; nevertheless, research on their activity on mycobacteria is still scarce. In particular, there is an urgent need to integrate all available interdisciplinary strategies to eradicate extensively drug-resistant Mycobacterium tuberculosis strains. In this context, we should not underestimate our endogenous antimicrobial proteins and peptides as ancient players of the human host defense system. We are confident that novel antibiotics based on human AMPs displaying a rapid and multifaceted mechanism, with reduced toxicity, should significantly contribute to reverse the tide of antimycobacterial drug resistance. In this review, we have provided an up to date perspective of the current research on AMPs to be applied in the fight against TB. A better understanding on the mechanisms of action of human endogenous peptides should ensure the basis for the best guided design of novel antitubercular chemotherapeutics.
Introduction
Tuberculosis (TB) is currently one of the most devastating infectious diseases having caused around 1.8 million human deaths, with 10.4 million new cases reported in 2016 and approximately a third of the world’s population harboring its persistent form of the disease-causing pathogen, Mycobacterium tuberculosis (Mtb) (1). Statistical analysis of epidemiological data have been shown a steady increase of the disease incidences over the past decade and new drug-resistant forms of TB cases are currently more than 5% of the total. TB has represented a major challenge worldwide and is the first/top leading cause of death from a single infectious microorganism (1).
Although the TB causing pathogen was first identified at the end of the nineteenth century, effective drugs against Mtb were only introduced during the second half of the twentieth century XXs: streptomycin first, followed by isoniazid (INH), pyrazinamide (PZA), ethambutol (EMB), and rifampicin (RIF). Unfortunately, the misuse and overuse of antibiotics for human welfare and farming industry have facilitated the emergence of resistant strains (2–4). Multidrug-resistant TB strains (MDR-TB) do not respond to INH and RIF and extensively drug-resistant strains (XDR-TB) display an added resistance to any fluoroquinolone and at-least one of the three second-line injectable drugs, i.e., amikacin, kanamycin, or capreomycin. Although the development of the first combined anti-TB drug-therapy dramatically improved the disease prognosis outcome, the current alarming rise in multidrug resistance is jeopardizing our early attempt to control the disease (5, 6). Moreover, the current WHO approved treatments impair the patient life quality and have an enormous economic cost (2, 7). Mtb being an extremely successful intracellular pathogen, can remain within the host system by keeping the immune responses under control via a wide repertoire of escape pathways (8). To complicate matters further, latent tubercle bacilli infections have become a serious global threat because of the challenge in diagnosing them clinically and their regular conversion from dormancy to active infections in immunocompromised circumstances, due to HIV coinfection, immunosuppressive therapies (9, 10) or diabetes mellitus type 2 conditions (11). Although novel drug susceptibility testing methodologies, such as the GeneXpert® MTB/RIF (12, 13) and HT-SPOTi (14), are enabling the early detection of antibiotic-resistant strains, a complete comprehension of the host immune capability and the mode by which Mtb handles/endures/evades the host defenses will be needed to eradicate this infectious disease (15).
Despite the initial underestimation of the properties of antimicrobial peptides (AMPs) and the difficulties encountered in their attempt to reach the market, nowadays, it is widely accepted that AMPs are multifunctional molecules with key contributions in the mammalian host innate defense (2, 3, 16, 17). In addition, due to the evolution of drug resistance among Mtb strains and their rapid spread across the globe, the use of both natural and synthetic AMPs and their combination with conventional drugs (18, 19) are enabling the creation of a new generation of truly promising antibiotics (20–23). As Mtb can survive and replicate within macrophages, novel anti-TB agents should be able to target the intracellularly dwelling mycobacteria without causing any damage to the host. In this review, we will focus on AMPs, either exploited naturally by our immune system or artificially synthesized, as potential therapeutics to overcome and eradicate the pathogen infection. Special attention will be paid to the diverse mechanisms that can mediate the AMPs’ action against TB infection. Finally, we will discuss the advantages, limitations, and challenges of AMPs for its merchandising and clinical use.
A Unique and Pathogenic Bacteria
Although most mycobacteria (more than 150 species reported to date) are environmental, only a few species can infect both humans and livestock alike. Mtb is an obligate human pathogen with a low mutation rate (24) and no horizontal gene transfer (25). The TB-causing bacilli have coevolved with our civilization over millennia and its indefinite latency periods probably evolved as an adaptation to the sparse geographic distribution of early human settlements. However, our modern one-world globalization might be triggering a worryingly shorter latency in TB (4).
Tuberculosis is mainly an airborne respiratory disease that is conveyed through aerosolized particles. Once in contact with the lung tissues, Mtb can enter and dwell within the host macrophages and other phagocytic immune cells. Immediately after, the infection triggers a complex immune response, and as a result, the pathogens may manage to establish a long-term residence within the host (4, 12, 26). During the primary infection phase, the host defense response sequesters the bacilli in confined cages at the lung alveoli, known as granuloma (Figure 1). During this early period the infected alveolar macrophages, the favorite mycobacterial lodge, are actively releasing pro-inflammatory effectors and other signaling molecules to remove the resident pathogens (8, 27). Following, the tubercle bacilli manage to downregulate the host cell expression profile and enter into a dormant state (26, 28). Ultimately, granuloma will mature and endure a necrosis process. Dormancy responses will facilitate the pathogen’s long-term intra-host survival, and enable it to withstand the necrotic granuloma environment. Upon reactivation of dormant cells, the bacilli will start growing extracellularly and cover the lung cavities with a biofilm layer enriched with the most drug-resistant cells (29). The spread of reinfection is then mediated by coughing induced granuloma mechanical shear (12, 28).
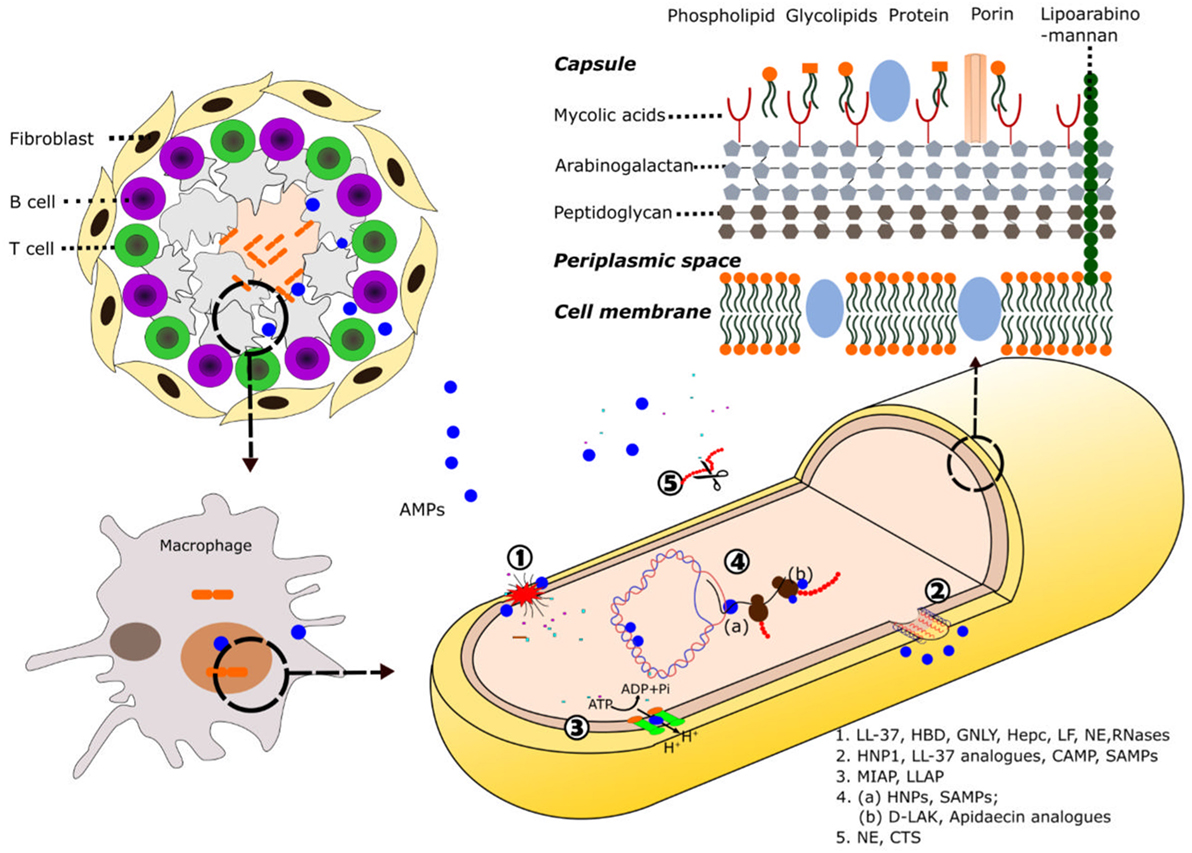
Figure 1. Schematic illustration of AMP mode of action against mycobacteria. Following induction of the immune response by mycobacteria, AMPs are directed toward the area of infection where they can be recruited into the granuloma. At the cellular level, the destruction of the pathogens takes place inside the macrophage phagolysosomes. Composition of the mycobacteria cell wall and the main described mechanisms of action of AMPs against mycobacteria are shown: (1) cell wall and plasmatic membrane disruption, (2) membrane pore formation, (3) inhibition of ATPase, (4) AMP intracellular targets: (a) nucleic acids binding, inhibition of replication, and transcription; (b) inhibition of translation, and (5) protein degradation. Selected AMPs for each activity are highlighted. See Tables 1 and 2 for a detailed description of each AMP mechanism of action. Abbreviation: LL-37, cathelicidin C-terminus; HBD, human β-defensin; GNLY, granulysin; Hepc, hepcidin; LF, lactoferrin; NE, neutrophil elastase; HNPs, human neutrophil proteins; CAMP, cationic antimicrobial peptides; SAMPs, synthetic antimicrobial peptides; MIAP, magainin-I derived antimicrobial peptide; LLAP, LL-37 derived antimicrobial peptide; d-LAK, d-enantiomeric antimicrobial peptides; CTS, cathepsins.
The Potential of Antimicrobial Peptides in the Anti-TB Chemotherapy: Unraveling Their Mechanisms of Action
Emergence of extensively antimicrobial resistance toward current anti-TB drugs has drawn back our attention toward alternative once neglected therapeutic strategies, including a resurge in AMPs research (2, 30). Expression of endogenous AMPs represents one of the most ancient host defense strategy of living organisms. Their multifunctional mode of action, natural origin, and effectiveness at low concentration have positioned them as prospective candidates in future antitubercular therapeutics market (3, 7, 31, 32). Notwithstanding, to ensure a successful therapy prior to drug design, we must deepen in the knowledge of the underlying mechanism of action of our own innate immunity players.
Despite a low level of amino acid sequence identity, AMPs adopt similar structural folds, indicating the existence of parallel mechanisms of antimicrobial action among distant living organisms (33). Among a significant variety of AMPs traits, we can outline the main common properties. We will review here the main known human AMPs secreted by innate immunity cells to counterbalance mycobacterial infections along with their mode of action.
Mycobacterial Cell-Wall: A Complex Barrier Particularly Difficult to Overcome
The unusual high antimicrobial resistance in mycobacteria is primarily due to the unique complexity of its cell wall. The complex network of macromolecules such as peptidoglycan, arabinogalactan, and mycolic acids (MAgP complex), which are conglomerated by other proteins and polysaccharides, confirm the main mycobacterial cell-wall scaffold and constitute a highly difficult crossing-barrier for antimicrobial agents (34–37) (Figure 1). The unique covalently-linked MAgP complex of Mtb is a result of mycobacterial adaptation to secure the intracellular survival against continuous selective pressures exerted by the host immune system and other hostile environments. Furthermore, it has been found that the characteristics and composition of the cell wall can be modified during infection (38). The length and structure of the mycolic acids have been related to bacterial intracellular survival and are one of the favorite targets of successful antibiotics (12, 37). Unfortunately, the emergence of Mtb strains with acquired resistance to INH and EMB drugs that target the mycolic acids synthesis, demands novel strategies. Resistant strains have also emerged to PZA, a drug that targets the cell-envelope integrity (2). In this context, dermcidin, a human peptide secreted by sweat glands (39) has been predicted to inhibit the mycolyl transferase enzyme efficiently (40). Other re-emerged research lines target the cell-wall peptidoglycan metabolism (12). On the other hand, one of the main mechanisms by which the AMPs exert their effect is based on the ability to disrupt or permeate the cell membrane (Figure 1), either fully disrupting the lipid bilayer or by creating transient pores (41). Numerous AMPs have acquired amphipathic and cationic structures as short β-sheets and α-helices that allow them to establish interactions with bacterial membranes (42). The first step of AMPs interaction with the pathogen is generally mediated by their positive net charge and hydrophobicity. Unlike eukaryotic cells, in which the anionic lipids are predominantly in the inner leaflet of the lipid membrane providing a neutral cell surface, prokaryotic cells expose a negatively charged surface. Many AMPs can exert a direct killing mechanism against mycobacteria through cell membrane disruption. The binding between the mycobacterial anionic surface compounds and the cationic residues of the peptides promotes the membrane permeabilization (43). Contribution of the peptide cationicity has been corroborated in distinct AMPs by amino acid substitution. As an example, the replacement of lysines by arginines in lactoferrin (LF) variants enhanced their mycobactericidal effect (44). In addition, although the highly hydrophobic scaffold of the mycobacterial envelope offers resistance to AMPs action, the increase in the proportion of α-helical structure and peptide hydrophobicity has being engineered as an alternative strategy to enhance their mycobactericidal features (18). Moreover, some AMPs are directly targeting surface cell-wall proteins to interfere in the cell ion exchange and inhibit the mycobacterial growth. AMPs can interact with the mycobacterial membrane proteins such as ATPases and inhibit the cell pH homeostasis (45, 46). Interestingly, AMPs inducing the membrane permeation can be applied as adjuvants to conventional antibiotics (47).
Intracellular Targets
Although most of the known AMPs exert their action at the bacterial membrane level, there is a growing number of identified peptides endowed with other previously overlooked targets. Many AMPs have the ability to translocate across the membrane and novel methodologies are bringing the opportunity to identify the peptide interactions with intracellular components (48). As an example, human neutrophil peptides can effectively cross the lipid bilayer without causing significant membrane damage and bind to nucleic acids (49, 50). Selective mycobactericidal action has been achieved by synthetic antimicrobial peptides (SAMPs) that can be internalized by mycobacterial cells and bind to DNA, inhibiting replication, and transcription processes (51). Interestingly, the intracellular action can be achieved at very low peptide concentrations, reducing the potential toxicity to host cells.
Phagosome-Lysosomal Pathway and Autophagy Modulation
Mycobacterium tuberculosis has evolved to dwell within one of the most inhospitable cell types, the macrophage. The tubercle bacillus is able to interfere with the phagosomal maturation pathway, blocking the transfer of the phagocytosed compounds to lysosomes (52). At this stage, several mechanisms take place toward the elimination of the pathogen, among them: production of reactive oxygen and nitrogen species, vacuole acidification, lytic enzymes activation, and changes in ion fluxes (53). Mycobacterium is able to interfere not only in the recruitment of vesicular ATPase proton pump but also in the acquisition of markers for the endocytic pathway. The TB causing bacilli promote the fusion with early endosomal vesicles but arrest the fusion to the lysosomal compartment, thereby protecting its phagosomal niche from acidification and avoiding the action of lytic enzymes. Moreover, the pathogen inhibits the phosphatidylinositol kinase, reducing the phosphatidyl inositol triphosphate (PIP3) levels and impairing the phagosome maturation (54). The modulation of the phagocytic maturation seems to be carried out by components of the mycobacterial cell wall, such as the mannosylated lipoarabinomannan (7, 54). Altogether, mycobacteria ensure their survival within the host cell by intercepting the autophagic machinery at distinct levels (Figure 2) (8, 55). On their side, many AMPs promoting the phagolysosome formation also contribute to remove the pathogen intruder (56). Thereby, one of the strategy undertaken by the mycobacteria is the downregulation of AMP expression within the macrophage (57). Autophagy has other beneficial effects for the host, such as the restriction of inflammation (58). Indeed, one of main currently used anti-TB drug is rapamycin, an autophagy activator, and the search of novel autophagy inducers is a priority (3, 23, 59).
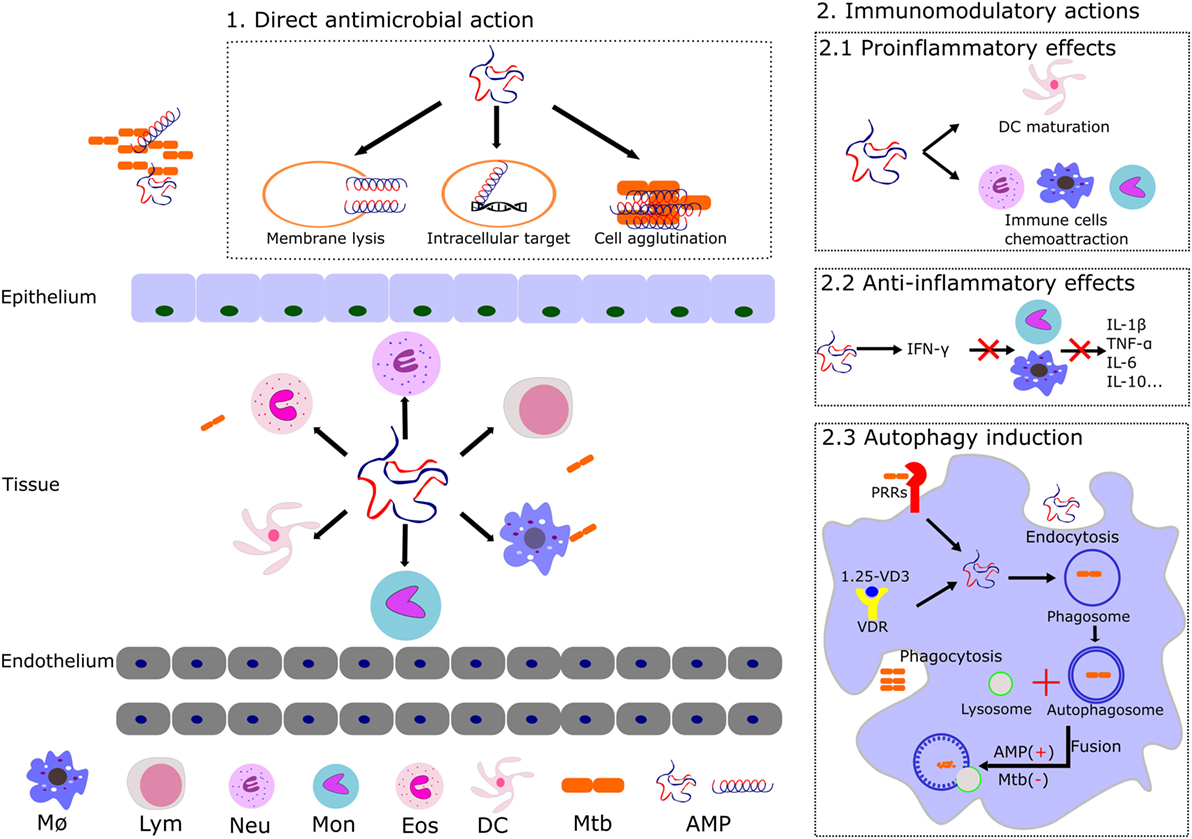
Figure 2. Illustration of the distinct reported mechanism of action of AMPs expressed by the host innate immune cells. The main AMP antimicrobial and immunomodulatory activities are shown: (1) AMPs can trigger the cell lysis, target intracellular key processes (described in Figure 1), and/or agglutinate the bacterial cells. (2) Main AMPs’ immunomodulatory actions that promote the mycobacterial clearance are illustrated. Induction of pro and anti-inflammatory activities contributes to the host defense by regulation of cytokines and chemokines expression and induction of innate cell maturation. AMPs can also intervene in the autophagosome and phagolysosome formation during autophagy. Abbreviations: MØ, macrophages; Lym, lymphocytes; Neu, neutrophils; Mon, monocytes; Eos, eosinophils; DC, dendritic cells; Mtb, Mycobacterium tuberculosis; AMP, antimicrobial peptides; VD3, vitamin D3; VDR, vitamin D receptor; PRRs, pattern recognition receptors.
Immunomodulatory Activities
Undoubtedly, immunotherapy is at the frontline of TB eradication programs. Following the bacteria engulfment by alveolar macrophages, the mycobacterial components are identified by several pattern recognition receptors resulting in the activation of signaling pathways and the subsequent leukocyte activation (27, 58). In this scenario, participation of endogenous AMPs during the host immune response (Figure 2) is key for a successful eradication of infection (28, 60). We can differentiate two main phases that would mediate the infection process, in the early acute step the AMPs can directly kill the Mtb bacilli, whereas in the secondary late step, the AMPs immunomodulatory action takes the leadership (26). Pro and anti-inflammatory effects can be induced by AMPs mediated by the release of a variety of cytokines (16, 23, 57). Interestingly, the same AMP can have a pro-inflammatory action at an early infection stage, while shifting to anti-inflammatory activity during late infection (3). Indeed, many immune factors play an essential role in the mediation of the infective process (8). For instance, the production of cytokines, which are important for the immune response, such as interferon gamma (IFNγ), are undermined by the mycobacterial infection (61).
Human Endogenous AMPs Involved in the Fight Against TB Infection
Following mycobacterial infection, a large assortment of antimicrobial peptides is released by our innate immune cells into the affected tissue (62). AMPs as key players of the non-specific immune response (2, 17) have attracted renewed attention as novel therapeutics and several comprehensive databases are now available open to the scientific community (2, 63–65). We describe, here, the main natural human AMPs involved in the fight against TB infection (Table 1).
Cathelicidins
Cathelicidins constitute a mammalian family of antimicrobial peptides mostly expressed in leukocytes and epithelial cells in response to different pathogens, contributing to their eradication (7, 37, 72). The human cationic antimicrobial peptide-18 (hCAP-18) is the unique known human member and the leading AMP in TB therapeutics (7, 131). hCAP-18 is essentially conformed by two regions, a highly conserved N-terminal sequence, called cathelin and the bactericidal C-terminal region known as LL-37, released by proteolysis (132, 133). LL-37 contributes to the recruitment of T-cells to the site of infection (66) and displays diverse immunomodulatory and antimicrobial activities (57, 73), undertaking a prominent role during mycobacterial infection (57, 69). In particular, a significant overexpression of LL-37 on neutrophils, epithelial cells, and alveolar macrophages has been observed during Mtb infection (67). The infection of mononuclear cells promotes the upregulation synthesis of LL-37 via the vitamin D induction pathway (134). Interestingly, vitamin D deficiency correlates with susceptibility to tuberculosis, while supplementation with vitamin D derivatives improves the efficiency to overcome TB (75). Phagosomal pathway is known to be a key defensive procedure to eradicate Mtb and recent studies point to vitamin D3 as an inducer of autophagy in human monocytes as well as an inhibitor of intracellular mycobacterial growth, via upregulation of autophagy-related gene expression (3, 76, 134). The LL-37 peptide thereby decreases, directly or indirectly, the rate of intracellular bacteria proliferation. Recently, transcriptome profiling confirmed the direct contribution of LL-37 at the lysosomal compartment (135). Jointly, all these experimental evidences highlight cathelicidin LL-37 not only as a forthright antimicrobial peptide but also as a prominent modulator of autophagy during mycobacterial infection (3, 77).
Defensins
Defensins were the first AMPs related to TB by pioneer researchers (49, 50, 81, 136). Defensins are a set of cationic and cysteine-rich peptides with immunomodulatory and microbicidal properties that constitute one of the major and most diverse group of AMPs in the mammalian pulmonary host defense system (3, 16, 137). They are classified according to their structure into alpha, beta, and theta. They show substantial variation in terms of amino acid sequences, and show a diversity of mechanism of action at membrane and intracellular levels. In addition, defensins can be induced and activated by proteolysis pathways to acquire their antibacterial activity (138). Interestingly, high-throughput gene expression of peripheral blood mononuclear cells profile analysis from patients with tuberculosis and Mtb-infected healthy donors revealed the existence of an overexpression of defensins levels in TB patients (139). The peptides were observed to bind to Mtb cells within the macrophage phagosome (140). The essential participation of defensins in the host fight against TB infection has also been corroborated in a murine model (23, 85).
Within the defensin family, we find a variety of cellular source types (Table 1) (82). Noteworthy, the β-defensin2 (HBD2) and the α-defensin (HAD) expression are inducible by mycobacteria wall components in epithelial cells and eosinophils, respectively (79, 81), and could have a preservative role in vivo against TB infection. Upregulation of HDB3 and 4 were also reported effective in Mtb MDR-infected mice (83).
Human Neutrophil Peptides (HNPs)
Human neutrophil peptides are α-defensin type AMPs mainly secreted by neutrophils (50), although low levels of expression have also been detected in monocytes, eosinophils, and epithelial cells (79). HNP-1–4 expression is induced by TB infection (7, 37, 82). On the other hand, although macrophages express only small amounts of HNPs, high intracellular levels can be reached via neutrophil-phagocytosis. Interestingly, HNPs have been observed to colocalize with tuberculosis bacilli in early endosomes (84). Moreover, the administration of HNPs maximizes the antimicrobial capacity of macrophages against Mtb (50) and HNP1 was proven effective in a Mtb-infected mouse model (50). HNP-1 can permeabilize the Mtb cell membrane by forming transmembrane pore and then bind to intracellular DNA (7, 50). Interaction with nucleic acids could subsequently inhibit the main cell functions, as transcription and translation (48). On the other hand, combination studies using HNPs and β-defensins with conventional antitubercular drugs have shown a synergistic effect. Therefore, the AMP adjuvant role can reduce the required drug dose and also significantly diminish the bacterial load in vital organs (141). Overall, these findings together with recent experimental work with tuberculosis animal models entrench the therapeutic application in favor of the whole defensin family (7, 37, 83).
Hepcidin
Hepcidin (Hepc) is a short and highly cationic antimicrobial peptide that was originally detected in serum and urine (142). It adopts a hairpin loop that encompasses two short beta-strands. Hepcidin is predominantly synthesized in hepatocytes and is released from a precursor by proteolysis. Its expression is induced by infectious or inflammatory processes and plays a prominent role in the iron homeostasis, regulating uptake, and mobilization (92, 143). Specifically, hepcidin can downregulate the transmembrane transport of iron through its union with ferroportin, a transmembrane protein that exports iron to the extracellular space (93). The reduction in extracellular iron concentrations makes pathogen invasion conditions more hostile (91). Interestingly, during infection, hepcidin is released into the bloodstream and is considered to be responsible of the anemia associated with inflammation (94). Indeed, anemia is a common difficulty encountered in TB (144). Moreover, Lafuse and coworkers demonstrated that mycobacterial infection induced the emergence of high levels of hepcidin in macrophages phagosomes and confirmed the peptide inhibition of Mtb growth in vitro (87). Further research also reported the presence of hepcidin in other innate cell types such as dendritic cells. The peptide expression in non-phagocyte cells suggests an extracellular mycobactericidal activity mediated by iron reduction in both alveolar and interstitial spaces (88). Particularly, due to the hepcidin effect on iron levels, differences in the expression of the peptide could be related to different phenotypes of iron homeostasis in TB patients. A significant correlation was observed between serum hepcidin levels and the promoter polymorphism in TB patients and was suggested to be considered in the diagnosis and prognosis of tuberculosis (145).
Lactoferrin
Lactoferrin is another AMP related to iron homeostasis regulation. It is a multifunctional iron binding glycoprotein present in several tissues and most human body fluids. It has a molecular weight of 80 kDa and belongs to the transferrin family (99). LF and its natural N-terminal fragment released by proteolytic cleavage (Lactoferricin, LFcin) participate in host defense and have wide spectra antimicrobial effects (37, 44, 98). Noteworthy, LF is the only AMP given by systemic administration that is currently in clinical trials (146). Diverse studies have demonstrated the presence of LF in macrophages and blood cells and its activity against Mycobacterium. Moreover, LF immunomodulatory capacity can also contribute to the eradication of TB. Particularly, it has been observed that mice treated with LF manifest an increase in the proportion of IL-12/IL-10, which results in increased Th1 cells, with a protective role against Mtb (100, 101). The anti-inflammatory properties of human and mouse LF were also corroborated in another Mtb mouse infected model (102). In addition, other studies clearly demonstrated the immunomodulatory role of LF, improving BCG-vaccine efficacy when used as adjuvant (147, 148). Recently, it has been reported that LF expressed in azurophilic granules of neutrophils is capable of killing M. smegmatis (104).
Lipocalins
Lipocalins are a family of peptides involved in cellular traffic and inflammation which are also related to the iron homeostasis (149). Lipocalin2, also called neutrophil gelatinase associated lipocalin, is expressed in neutrophils and displays anti-TB activity (130).
Azurocidin
Azurocidin, a leukocyte polymorphonuclear (PMN) granule protein, is a cationic antimicrobial protein of 37 kDa, also called CAP37 or heparin-binding protein, due to its high affinity for heparin (103). Shortly after its discovery it was found that azurocidin, like other antimicrobial proteins, not only displayed an antimicrobial activity but was also capable of exerting a mediating role in the modulation of the host defense system (150). Azurocidin is stored in secretory granules and is released into the endothelial area by PMN cells, rapidly reaching the infected or inflammation area (151). Azurocidin, at the front line of infection, activates monocytes, macrophages, and epithelial cells (152). Moreover, azurocidin has a wide range antimicrobial activity, working efficiently at acidic pH, a condition promoted in mature phagolysosomes (153). Interestingly, it has recently been reported that azurophilic granule proteins are implicated in mycobacterial killing, facilitating the fusion of mycobacteria-containing phagosomes with lysosomes (104).
Elastases
Elastases are serine proteases secreted by neutrophils and macrophages involved in the fight against pulmonary infections (107). One of the best studied elastase is the neutrophil elastase (NE), also known as elastase2, a 29-kDa protein expressed during myeloid development and secreted by neutrophils during episodes of infection and inflammation (107, 108). NE was reported to confer a protective effect against M. bovis in mice pulmonary tract (109). Many studies emphasize NE multi-functionality; the protein can break the tight junctions to facilitate the migration of PMN cells to the inflammation/infection area and induce cell chemotaxis (108). The neutrophil granule protein can work within the macrophage phagosomes (154). Complementarily, NE is also reported to kill mycobacteria extracellularly in a rather peculiar way. Neutrophil granules can release their protein cargo together with chromatin, resulting in the formation of extracellular fibrillar structures that facilitate bacteria arrest. NE colocalizes with the neutrophil extracellular traps and can facilitate the degradation of virulence factors (110, 155). Interestingly, heavily infected macrophages can also explode and form extracellular traps, a process which is also regulated by elastases (106, 111).
Cathepsin (CTS)
Cathepsin is another serine protease involved in the host defense against TB infection that is mainly expressed in neutrophils and macrophages (130, 156). Procathepsins are converted to the mature enzyme in acidic conditions and are active within the lysosomal compartment (30). The Mtb bacilli can downregulate CTSs expression in macrophages to ensure its intracellular survival (120, 156). The antimicrobial protease is proposed to protect the host mostly by an immunoregulatory role rather than a direct bacteria killing activity, as observed in an infection mouse model (122). Recent work using the zebrafish/M. marinum model indicates the involvement of macrophage lysosomal CTSs to control the TB infection at the granuloma level (121, 157).
Granulysin (GNLY)
Granulysin is a small cationic human antimicrobial protein expressed by lymphocytes that is upregulated by HIV/TB coinfection (37, 158). GNLY can enter the macrophages and is able to disrupt the bacillus envelope (7).
Calgranulin
Calgranulin, also called calprotectin, is another AMP that is used as a TB infection marker in blood samples (30, 124, 127). Calgranulin is a calcium-binding protein that also interacts avidly with Zn2+ cations. Binding to Zn2+ activates the peptide antimicrobial activity. Recently, calgranulin overexpression has been associated to anti-TB activity at the macrophage intracellular level by promotion of the phagolysosomal fusion (30, 126).
Ubiquitin-Derived Peptides
Ubiquitin-derived peptides are ubiquitinated proteolytic peptides which can also be classified as AMPs (7, 37, 159). In particular, ubiquitin-conjugated peptides as products of the proteosome degradation activity accumulate in the lysosome and can inhibit Mtb growth within the autophagolysosome (129). Ubiquitin by itself is innocuous while ubiquitinated peptides, such as Ub2, can permeate the mycobacteria membrane (160).
Human Antimicrobial RNases
Human antimicrobial RNases are small secretory proteins (~15 kDa) belonging to the RNaseA superfamily. They are highly cationic and possess a wide range of biological properties, representing an excellent example of multitasking proteins (112, 161). The family comprises eight human members, expressed in diverse epithelial and blood cell types.
RNase3, also known as the eosinophil cationic protein (ECP), is mainly expressed during infection and inflammation in the secondary granules of eosinophils (162) and secondarily in neutrophils (163). Complementarily, the signal peptide of the ECP (ECPsp) was found to promote the migration of macrophages via pro-inflammatory molecules to sites of infection and inflammation (164). Interestingly, ECP is secreted, together with α-defensin, in response to M. bovis BCG infection (79). Although the recruitment of eosinophils in the respiratory tract during Mtb infection was first regarded as a mere response to inflammation (165), further work has shown that this cell type together with neutrophils can directly participate in the removal of the infection focus (166). Eosinophils are activated via TLR2 induction by the specific mycobacterial wall component, the lipomannan (79). Eosinophils, together with neutrophils, would then release the content of their granules into the granuloma macrophages (84, 159). To note, the eosinophil peroxidase, another eosinophil protein stored in the secondary granules, is also endowed with antimycobacterial activity (119). On the other hand, macrophages express upon bacterial infection two additional RNases, RNase6 and RNase7 (114). In addition, human RNase7, also called the skin derived RNase, is also secreted by keratinocytes and exerts a protective role against a variety of pathogens at the skin barrier (39, 115). Interestingly, RNase7, together with RNase3, can eradicate mycobacteria in vitro (117). Moreover, very recent results indicate that human RNases 3, 6, and 7 can also inhibit the growth of mycobacteria in a macrophage infection model (167). Considering that RNase6 and RNase 7 expression is induced in macrophages upon bacterial infection (114), one might hypothesize that these antimicrobial proteins can also play a physiological role against intracellular dwelling mycobacteria. Eventually, we cannot disregard a complementary contribution of the RNases reported immunomodulatory properties, such as the induction of pro-inflammatory cytokines and the dendritic cell chemoattraction (168, 169).
Synthetic Antimicrobial Peptides
In the race against TB, novel synthetic AMPs with potent mycobactericidal activities have been developed (2, 19, 22, 37, 170). AMP synthetic analogs are often considered to be the next generation of antibiotics and have attracted the attention of many companies aiming to develop new anti-TB therapies against drug-resistant strains (35). Following, we summarize the main SAMPs successfully designed (Table 2).
One of the favorite applied strategies for the design of potent AMPs is the engineering of stabilized amphipathic α-helix that are enriched with selected antimicrobial prone amino acids. Complementarily, peptide modifications are devised to endow them with enhanced resistance to proteolysis; thereby improving their in vivo stability and efficacy. The d-LAK peptides are a family of serial peptides consisting of 25 d-enantiomer amino acid residues in a primary sequence designed to adopt a left-handed α-helix conformation and containing eight lysine residues (175). The peptides were designed to enhance their antimicrobial activity and decrease their hemolytic effect (188), providing efficient antimycobacterial activity at non-toxic concentrations. Furthermore, d-LAK peptides can be administered as inhalable dry powder (176). Another synthetic α-helical peptide, the M(LLKK)2M, was proven successful against MDR strains when combined with RIF (187). On the other hand, a short synthetic cathelicidin variant (the HHC-10) is able to inhibit the growth of M. bovis BCG both in vitro and in a mouse model (178).
Interestingly, the N-terminus derived peptides of human antimicrobial RNases can reproduce the parental protein activity against several tested Mycobacterium species (117, 167). The RN(1-45) peptides encompass a highly cationic and amphipathic region that adopts an extended α-helix in a membrane-like environment (189). In addition, the RN3(1-45) and RN6(1-45) peptides include an aggregation prone sequence which promotes bacterial cell agglutination (117, 167, 190), a property that can facilitate the microbial clearance at the infectious focus (190).
Recently, particular interest has been drawn by a collection of short synthetic peptides with immunomodulatory activities, the innate defense regulators (IDRs). The peptides are effective at very low concentration and thereby can elude any toxicity to the host (181). They do not display a direct bactericidal activity but can promote the proper endogenous expression of antimicrobial agents by the host cells. Among others, the peptides enhance the release of chemokines and downregulate the inflammation pathway (181, 182). The IDR peptides, such as the IDR-1018 (Table 2), have been tested successfully in a MDR-TB infected mouse model by intra-tracheal administration (180). Likely, immunoregulatory peptides will take a leading role in the treatment of immunocompromised patients in a near future (16).
AMPs to Combat Antimicrobial Resistance in TB: A Time for Hope
In recent years, thousands of antimicrobial peptides have been identified from natural sources, mostly classified as key players of the non-specific host defense response (30, 33, 191). On the other hand, despite the existence of a wide range of successful antibiotics since their entry into the worldwide trade, nowadays there is an increasing demand of novel drugs to tackle multidrug-resistance mycobacteria strains (2, 20, 192). The antimicrobial proteins and peptides (AMPPs), given their direct bacilli killing mechanism and immunomodulatory properties provide an attractive pharmacological potential against mycobacterial infections (see Table 3 for a summary of main AMP-based therapies). However, despite their appealing properties, AMPs are still facing major challenges to join the pharmaceutical industry (30–32). The main advantages and disadvantages associated with AMPs are listed in Table 4. Although the high cost of synthesis is one of the main drawbacks that the manufacturing of peptides faces, some companies are already managing commercial-scale peptide production platforms. For example, recombinant AMPs can be prepared in fungi and plants at high yield and low cost (2). Another drawback of AMPs therapy is their susceptibility to proteolytic cleavage, in particular when delivered by systemic administration (2, 31). In addition, the antimicrobial activity of some peptides appears to be decimated in physiological saline and serum conditions (32, 193). Novel design strategies are focusing on the production of cheaper and reduced-size analogs (2, 194) with improved selectivity toward prokaryotic targets and broaden therapeutic indexes (195). To improve the peptide bioavailability and stability in vivo several strategies have been developed such as incorporation of non-natural amino acids, backbone mimetics, conjugation with fatty acids, N and C- terminus modifications (196). The peptide performance can also be improved by intra-tracheal administration (184). In addition, encapsulation within biodegradable particles or liposomes improves the distribution of the drug toward the site of action (31, 196). Fortunately, macrophage nature by itself should promote the engulfment of such nanovehicles (19) and extensive research has been applied to define the parameters that determine the nanoparticles uptake by the phagocytic cells and intracellular traffic (2, 197). Very recently, a novel delivery system has been achieved by a LL-37 analog embedded within a hyaluronic nanogel. The self-assembled polymer stabilizes the peptide inside its hydrophobic core, allows a higher dose cargo and promotes the macrophage uptake, with increased antimicrobial efficiency and reduced toxicity to host cells. Besides, AMPs are also prone to aggregate and show occasionally poor solubility (198, 199). Luckily, there are currently different strategies and predictive software available to prevent aggregation and improve physicochemical properties (42, 200, 201). Complementarily, cleavage protection can be enhanced through secondary structure stabilization (37, 202). Alternatively, AMPs, as effector players of the host immune system (203) can also be upregulated by immunostimulation therapies (23, 204–206), overcoming the drawbacks inherent to the peptide administration via. Furthermore, recent studies on mycobacterial infection reveal how some species such as Mtb are capable of inhibiting the expression and release of endogenous AMPs (30, 77). Thus, the administration of supplementary AMPs, the use of gene therapy (136) or an immunomodulatory hormonal induction would be necessary to achieve an effective dose (207). This approach would be mostly recommended for immunocompromised patients (60, 204, 205). However, researchers should not disregard the unpredictable long-term consequences of exposing the bacteria to an overdose of AMPs. Mycobacteria pathogens exposed to either externally administered or endogenous overexpressed AMPs might develop novel resistance strategies to face back this new affront (2, 146, 208). In fact, the co-evolution of the pathogens with natural AMPs has already induced some bacterial resistance mechanisms (209–212). First, bacteria can alter their cell envelope composition to reduce their affinity toward cationic peptides (82, 209, 212, 213). Pathogenic mycobacteria can also ensure their intracellular survival by the control of the macrophage efflux pump (210, 211). Other observed strategies are the release of extracellular proteases (212, 214, 215) or the downregulation of host AMPs (74). Therefore, resistance to AMPs should be anticipated and might be overcome by innovative peptide variants (216), host-directed therapies or the use of combined synergies (47, 215). Eventually, recent collaborative initiatives have been launched to join efforts in the fight against mycobacterial resistance (TBNET, FightTB, TB-Platform, and TB-PACTS) (2), opening a window of hope.
Concluding Remarks
Peptide-based therapy to treat infectious diseases is recently experiencing resurgence. AMPs, as mere components of the immune system, promote the direct killing of mycobacteria and often have immunomodulatory effects. Their non-specific pleiotropic mechanisms of action and unique immunomodulatory properties over conventional antibiotics have awakened the pharmaceutical market interest. Moreover, the efficacy of BCG vaccine is highly variable and the alarming increase of extensively drug-resistant strains of Mtb is a major global health emergency to address. In this context and considering the limitations in the current antituberculosis drug treatment, AMPs represent an immediate alternative approach in tackling antimicrobial resistance. Scientific evidences provide a solid basis to ensure that the future development of peptide-based therapy will continue to address the unsolved drawbacks that the pharmaceutical industry is currently facing. Novel research methodologies and integrated interdisciplinary strategies should provide the opportunity to boast current antimicrobial peptide research efforts in the fight against tuberculosis.
Author Contributions
JA-T, LL, DP, SB, and EB contributed to the original draft and edited versions. JA-T and LL prepared the graphical material and JA-T, DP, LL, and EB prepared the tables. SB and EB wrote, edited, and revised the final manuscript version. All authors approved the final manuscript version.
Conflict of Interest Statement
No potential conflicts of interest were disclosed. The authors declare that the research was conducted in the absence of any commercial or financial relationships that could be construed as a potential conflict of interest.
Funding
Research work was supported by the Ministerio de Economía y Competitividad (SAF2015-66007P) co-financed by FEDER funds. LL is a recipient of a CSC predoctoral fellowship. JA-T was a recipient of a predoctoral fellowship (PIF, UAB). SB is a Cipla distinguished Fellow in Pharmaceutical Sciences.
References
2. Silva JP, Appelberg R, Gama FM. Antimicrobial peptides as novel anti-tuberculosis therapeutics. Biotechnol Adv (2016) 34:924–40. doi:10.1016/j.biotechadv.2016.05.007
3. Rodriguez Plaza JG. Prospective tuberculosis treatment: peptides, immunity and autophagy. J Mol Genet Med (2014) 8:128. doi:10.4172/1747-0862.1000128
4. Eldholm V, Balloux F. Antimicrobial resistance in Mycobacterium tuberculosis: the odd one out. Trends Microbiol (2016) 24:637–48. doi:10.1016/j.tim.2016.03.007
5. Zager EM, McNerney R. Multidrug-resistant tuberculosis. BMC Infect Dis (2008) 8:10. doi:10.1186/1471-2334-8-10
6. Dye C. Doomsday postponed? Preventing and reversing epidemics of drug-resistant tuberculosis. Nat Rev Microbiol (2009) 7:81–7. doi:10.1038/nrmicro2048
7. Khusro A, Aarti C, Agastian P. Anti-tubercular peptides: a quest of future therapeutic weapon to combat tuberculosis. Asian Pac J Trop Med (2016) 9:1023–34. doi:10.1016/j.apjtm.2016.09.005
8. Gupta A, Kaul A, Tsolaki AG, Kishore U, Bhakta S. Mycobacterium tuberculosis: immune evasion, latency and reactivation. Immunobiology (2012) 217:363–74. doi:10.1016/j.imbio.2011.07.008
9. Keane J, Gershon S, Wise RP, Mirabile-Levens E, Kasznica J, Schwieterman WD, et al. Tuberculosis associated with infliximab, a tumor necrosis factor alpha-neutralizing agent. N Engl J Med (2001) 345:1098–104. doi:10.1056/NEJMoa011110
10. Yasui K. Immunity against Mycobacterium tuberculosis and the risk of biologic anti-TNF-α reagents. Pediatr Rheumatol Online J (2014) 12:45. doi:10.1186/1546-0096-12-45
11. Montoya-Rosales A, Castro-Garcia P, Torres-Juarez F, Enciso-Moreno JA, Rivas-Santiago B. Glucose levels affect LL-37 expression in monocyte-derived macrophages altering the Mycobacterium tuberculosis intracellular growth control. Microb Pathog (2016) 97:148–53. doi:10.1016/j.micpath.2016.06.002
12. Maitra A, Kamil TK, Shaik M, Danquah CA, Chrzastek A, Bhakta S. Early diagnosis and effective treatment regimens are the keys to tackle antimicrobial resistance in tuberculosis (TB): a report from Euroscicon’s international TB Summit 2016. Virulence (2016) 8(6):1005–24. doi:10.1080/21505594.2016.1256536
13. Catanzaro A, Rodwell TC, Catanzaro DG, Garfein RS, Jackson RL, Seifert M, et al. Performance comparison of three rapid tests for the diagnosis of drug-resistant tuberculosis. PLoS One (2015) 10:1–14. doi:10.1371/journal.pone.0136861
14. Danquah CA, Maitra A, Gibbons S, Faull J, Bhakta S. HT-SPOTi: a rapid drug susceptibility test (DST) to evaluate antibiotic resistance profiles and novel chemicals for anti-infective drug discovery. Curr Protoc Microbiol (2016) 40:1–12. doi:10.1002/9780471729259.mc1708s40
15. Dheda K, Barry CE, Maartens G. Tuberculosis. Lancet (2016) 387(10024):1211–26. doi:10.1016/S0140-6736(15)00151-8
16. Hancock REW, Haney EF, Gill EE. The immunology of host defence peptides: beyond antimicrobial activity. Nat Rev Immunol (2016) 16:321–34. doi:10.1038/nri.2016.29
17. Bastos P, Trindade F, da Costa J, Ferreira R, Vitorino R. Human antimicrobial peptides in bodily fluids: current knowledge and therapeutic perspectives in the postantibiotic era. Med Res Rev (2017). doi:10.1002/med.21435
18. Khara JS, Lim FK, Wang Y, Ke X-Y, Voo ZX, Yang YY, et al. Designing α-helical peptides with enhanced synergism and selectivity against Mycobacterium smegmatis: discerning the role of hydrophobicity and helicity. Acta Biomater (2015) 28:99–108. doi:10.1016/j.actbio.2015.09.015
19. Mohanty S, Jena P, Mehta R, Pati R, Banerjee B, Patil S, et al. Cationic antimicrobial peptides and biogenic silver nanoparticles kill mycobacteria without eliciting DNA damage and cytotoxicity in mouse macrophages. Antimicrob Agents Chemother (2013) 57:3688–98. doi:10.1128/AAC.02475-12
20. Zumla A, George A, Sharma V, Herbert RHN, Oxley A, Oliver M. The WHO 2014 global tuberculosis report – further to go. Lancet Glob Heal (2015) 3:e10–2. doi:10.1016/S2214-109X(14)70361-4
21. Olmo ED, Molina-Salinas GM, Bini EI, González-Hernández S, Bustos LA, Escarcena R, et al. Efficacious in vitro and in vivo effects of dihydrosphingosine-ethambutol analogues against susceptible and multi-drug-resistant Mycobacterium tuberculosis. Arch Med Res (2016) 47:262–70. doi:10.1016/j.arcmed.2016.07.004
22. Khara JS, Priestman M, Uhía I, Hamilton MS, Krishnan N, Wang Y, et al. Unnatural amino acid analogues of membrane-active helical peptides with anti-mycobacterial activity and improved stability. J Antimicrob Chemother (2016) 71:2181–91. doi:10.1093/jac/dkw107
23. Rivas-Santiago CE, Hernández-Pando R, Rivas-Santiago B. Immunotherapy for pulmonary TB: antimicrobial peptides and their inducers. Immunotherapy (2013) 5:1117–26. doi:10.2217/imt.13.111
24. Mcgrath M, Gey van Pittius NC, Van Helden PD, Warren RM, Warner DF. Mutation rate and the emergence of drug resistance in Mycobacterium tuberculosis. J Antimicrob Chemother (2014) 69:292–302. doi:10.1093/jac/dkt364
25. Gröschel MI, Sayes F, Simeone R, Majlessi L, Brosch R. ESX secretion systems: mycobacterial evolution to counter host immunity. Nat Rev Microbiol (2016) 14:677–91. doi:10.1038/nrmicro.2016.131
26. Volpe E, Cappelli G, Grassi M, Martino A, Serafino A, Colizzi V, et al. Gene expression profiling of human macrophages at late time of infection with Mycobacterium tuberculosis. Immunology (2006) 118:449–60. doi:10.1111/j.1365-2567.2006.02378.x
27. Portugal B, Motta FN, Correa AF, Nolasco DO, de Almeida H, Magalhães KG, et al. Mycobacterium tuberculosis prolyl oligopeptidase induces in vitro secretion of proinflammatory cytokines by peritoneal macrophages. Front Microbiol (2017) 08:155. doi:10.3389/fmicb.2017.00155
28. Wallis RS, Hafner R. Advancing host-directed therapy for tuberculosis. Nat Rev Immunol (2015) 15:255–63. doi:10.1038/nri3813
29. Ojha AK, Baughn AD, Sambandan D, Hsu T, Trivelli X, Guerardel Y, et al. Growth of Mycobacterium tuberculosis biofilms containing free mycolic acids and harbouring drug-tolerant bacteria. Mol Microbiol (2008) 69:164–74. doi:10.1111/j.1365-2958.2008.06274.x
30. Padhi A, Sengupta M, Sengupta S, Roehm KH, Sonawane A. Antimicrobial peptides and proteins in mycobacterial therapy: current status and future prospects. Tuberculosis (Edinb) (2014) 94:363–73. doi:10.1016/j.tube.2014.03.011
31. Mahlapuu M, Håkansson J, Ringstad L, Björn C. Antimicrobial peptides: an emerging category of therapeutic agents. Front Cell Infect Microbiol (2016) 6:194. doi:10.3389/fcimb.2016.00194
32. Kosikowska P, Lesner A. Antimicrobial peptides (AMPs) as drug candidates: a patent review (2003–2015). Expert Opin Ther Pat (2016) 26:689–702. doi:10.1080/13543776.2016.1176149
33. Diamond G, Beckloff N, Weinberg A, Kisich KO. The roles of antimicrobial peptides in innate host defense. Curr Pharm Des (2009) 15:2377–92. doi:10.2174/138161209788682325
34. Mishra AK, Driessen NN, Appelmelk BJ, Besra GS. Lipoarabinomannan and related glycoconjugates: structure, biogenesis and role in Mycobacterium tuberculosis physiology and host-pathogen interaction. FEMS Microbiol Rev (2011) 35:1126–57. doi:10.1111/j.1574-6976.2011.00276.x
35. Abedinzadeh M, Gaeini M, Sardari S. Natural antimicrobial peptides against Mycobacterium tuberculosis. J Antimicrob Chemother (2015) 70:1285–9. doi:10.1093/jac/dku570
36. Bansal-Mutalik R, Nikaido H. Mycobacterial outer membrane is a lipid bilayer and the inner membrane is unusually rich in diacyl phosphatidylinositol dimannosides. Proc Natl Acad Sci U S A (2014) 111:4958–63. doi:10.1073/pnas.1403078111
37. Gutsmann T. Interaction between antimicrobial peptides and mycobacteria. Biochim Biophys Acta (2016) 1858:1034–43. doi:10.1016/j.bbamem.2016.01.031
38. Bhamidi S, Shi L, Chatterjee D, Belisle JT, Crick DC, McNeil MR. A bioanalytical method to determine the cell wall composition of Mycobacterium tuberculosis grown in vivo. Anal Biochem (2012) 421:240–9. doi:10.1016/j.ab.2011.10.046
39. Niyonsaba F, Kiatsurayanon C, Chieosilapatham P, Ogawa H. Friends or foes? Host defense (antimicrobial) peptides and proteins in human skin diseases. Exp Dermatol (2017) 26(11):989–98. doi:10.1111/exd.13314
40. Banerjee DI, Gohil TP. Interaction of antimicrobial peptide with mycolyl transferase in Mycobacterium tuberculosis. Int J Mycobacteriol (2016) 5:83–8. doi:10.1016/j.ijmyco.2015.07.002
41. Wimley WC. Describing the mechanism of antimicrobial peptide action with the interfacial activity model. ACS Chem Biol (2010) 5:905–17. doi:10.1021/cb1001558
42. Torrent M, Andreu D, Nogués VM, Boix E. Connecting peptide physicochemical and antimicrobial properties by a rational prediction model. PLoS One (2011) 6:e16968. doi:10.1371/journal.pone.0016968
43. Méndez-Samperio P. The human cathelicidin hCAP18/LL-37: a multifunctional peptide involved in mycobacterial infections. Peptides (2010) 31:1791–8. doi:10.1016/j.peptides.2010.06.016
44. Silva T, Magalhães B, Maia S, Gomes P, Nazmi K, Bolscher JGM, et al. Killing of Mycobacterium avium by lactoferricin peptides: improved activity of arginine- and d-amino-acid-containing molecules. Antimicrob Agents Chemother (2014) 58:3461–7. doi:10.1128/AAC.02728-13
45. Rao M, Streur TL, Aldwell FE, Cook GM. Intracellular pH regulation by Mycobacterium smegmatis and Mycobacterium bovis BCG. Microbiology (2001) 147:1017–24. doi:10.1099/00221287-147-4-1017
46. Santos P, Gordillo A, Osses L, Salazar LM, Soto CY. Effect of antimicrobial peptides on ATPase activity and proton pumping in plasma membrane vesicles obtained from mycobacteria. Peptides (2012) 36:121–8. doi:10.1016/j.peptides.2012.04.018
47. Wright GD. Antibiotic adjuvants: rescuing antibiotics from resistance. Trends Microbiol (2016) 24:862–71. doi:10.1016/j.tim.2016.06.009
48. Le C-F, Fang C-M, Sekaran SD. Beyond membrane-lytic: intracellular targeting mechanisms by antimicrobial peptides. Antimicrob Agents Chemother (2017) 61:e02340–16. doi:10.1128/AAC.02340-16
49. Sharma S, Verma I, Khuller GK. Antibacterial activity of human neutrophil peptide-1 against Mycobacterium tuberculosis H37Rv: in vitro and ex vivo study. Eur Respir J (2000) 16:112–7. doi:10.1034/j.1399-3003.2000.16a20.x
50. Sharma S, Verma I, Khuller GK. Therapeutic potential of human neutrophil peptide 1 against experimental tuberculosis. Antimicrob Agents Chemother (2001) 45:639–40. doi:10.1128/AAC.45.2.639-640.2001
51. Sharma A, Pohane AA, Bansal S, Bajaj A, Jain V, Srivastava A. Cell penetrating synthetic antimicrobial peptides (SAMPs) exhibiting potent and selective killing of Mycobacterium by targeting its DNA. Chemistry (2015) 21:3540–5. doi:10.1002/chem.201404650
52. Goldberg MF, Saini NK, Porcelli SA. Evasion of innate and adaptive immunity by Mycobacterium tuberculosis. Microbiol Spectr (2014) 2:1–24. doi:10.1128/microbiolspec.MGM2-0005-2013
53. Soldati T, Neyrolles O. Mycobacteria and the intraphagosomal environment: take it with a pinch of salt(s)! Traffic (2012) 13:1042–52. doi:10.1111/j.1600-0854.2012.01358.x
54. Vergne I, Fratti RA, Hill PJ, Chua J, Belisle J, Deretic V. Mycobacterium tuberculosis phagosome maturation arrest: mycobacterial phosphatidylinositol analog phosphatidylinositol mannoside stimulates early endosomal fusion. Mol Biol Cell (2004) 15:751–60. doi:10.1091/mbc.E03-05-0307
55. Cemma M, Brumell JH. Interactions of pathogenic bacteria with autophagy systems. Curr Biol (2012) 22:R540–5. doi:10.1016/j.cub.2012.06.001
56. Muciño G, Castro-Obregón S, Hernandez-Pando R, Del Rio G. Autophagy as a target for therapeutic uses of multifunctional peptides. IUBMB Life (2016) 68:259–67. doi:10.1002/iub.1483
57. Torres-Juarez F, Cardenas-Vargas A, Montoya-Rosales A, González-Curiel I, Garcia-Hernandez MH, Enciso-Moreno JA, et al. LL-37 immunomodulatory activity during Mycobacterium tuberculosis infection in macrophages. Infect Immun (2015) 83:4495–503. doi:10.1128/IAI.00936-15
58. Yu X, Li C, Hong W, Pan W, Xie J. Autophagy during Mycobacterium tuberculosis infection and implications for future tuberculosis medications. Cell Signal (2013) 25:1272–8. doi:10.1016/j.cellsig.2013.02.011
59. Stanley SA, Barczak AK, Silvis MR, Luo SS, Sogi K, Vokes M, et al. Identification of host-targeted small molecules that restrict intracellular Mycobacterium tuberculosis growth. PLoS Pathog (2014) 10:e1003946. doi:10.1371/journal.ppat.1003946
60. Castañeda-Delgado JE, Frausto-Lujan I, González-Curiel I, Montoya-Rosales A, Serrano CJ, Torres-Juarez F, et al. Differences in cytokine production during aging and its relationship with antimicrobial peptides production. Immunol Investig (2017) 46:48–58. doi:10.1080/08820139.2016.1212873
61. Kim B-H, Shenoy AR, Kumar P, Das R, Tiwari S, MacMicking JD. A family of IFN-γ-inducible 65-kD GTPases protects against bacterial infection. Science (2011) 332:717–21. doi:10.1126/science.1201711
62. Shin D-M, Jo E-K. Antimicrobial peptides in innate immunity against mycobacteria. Immune Netw (2011) 11:245. doi:10.4110/in.2011.11.5.245
63. Wang G, Li X, Wang Z. APD3: the antimicrobial peptide database as a tool for research and education. Nucleic Acids Res (2016) 44:D1087–93. doi:10.1093/nar/gkv1278
64. Zhao X, Wu H, Lu H, Li G, Huang Q. LAMP: a database linking antimicrobial peptides. PLoS One (2013) 8:6–11. doi:10.1371/journal.pone.0066557
65. Aguilera-Mendoza L, Marrero-Ponce Y, Tellez-Ibarra R, Llorente-Quesada MT, Salgado J, Barigye SJ, et al. Overlap and diversity in antimicrobial peptide databases: compiling a non-redundant set of sequences. Bioinformatics (2015) 31:2553–9. doi:10.1093/bioinformatics/btv180
66. De Y, Chen Q, Schmidt AP, Anderson GM, Wang JM, Wooters J, et al. LL-37, the neutrophil granule- and epithelial cell-derived cathelicidin, utilizes formyl peptide receptor-like 1 (FPRL1) as a receptor to chemoattract human peripheral blood neutrophils, monocytes, and T cells. J Exp Med (2000) 192:1069–74. doi:10.1084/jem.192.7.1069
67. Rivas-Santiago B, Hernandez-Pando R, Carranza C, Juarez E, Contreras JL, Aguilar-Leon D, et al. Expression of cathelicidin LL-37 during Mycobacterium tuberculosis infection in human alveolar macrophages, monocytes, neutrophils, and epithelial cells. Infect Immun (2008) 76:935–41. doi:10.1128/IAI.01218-07
68. Di Nardo A, Vitiello A, Gallo RL. Cutting edge: mast cell antimicrobial activity is mediated by expression of cathelicidin antimicrobial peptide. J Immunol (2003) 170:2274–8. doi:10.4049/jimmunol.170.5.2274
69. Sonawane A, Santos JC, Mishra BB, Jena P, Progida C, Sorensen OE, et al. Cathelicidin is involved in the intracellular killing of mycobacteria in macrophages. Cell Microbiol (2011) 13:1601–17. doi:10.1111/j.1462-5822.2011.01644.x
70. Sigurdardottir SL, Thorleifsdottir RH, Guzman AM, Gudmundsson GH, Valdimarsson H, Johnston A. The anti-microbial peptide LL-37 modulates immune responses in the palatine tonsils where it is exclusively expressed by neutrophils and a subset of dendritic cells. Clin Immunol (2012) 142:139–49. doi:10.1016/j.clim.2011.09.013
71. Büchau AS, Morizane S, Trowbridge J, Schauber J, Kotol P, Bui JD, et al. The host defense peptide cathelicidin is required for NK cell-mediated suppression of tumor growth. J Immunol (2009) 184:369–78. doi:10.4049/jimmunol.0902110
72. Zanetti M. The role of cathelicidins in the innate host defenses of mammals. Curr Issues Mol Biol (2005) 7:179–96.
73. Kai-Larsen Y, Agerberth B. The role of the multifunctional peptide LL-37 in host defense. Front Biosci (2008) 13:3760–7. doi:10.2741/2964
74. Gupta S, Winglee K, Gallo R, Bishai WR. Bacterial subversion of cAMP signalling inhibits cathelicidin expression, which is required for innate resistance to Mycobacterium tuberculosis. J Pathol (2017) 242:52–61. doi:10.1002/path.4878
75. Campbell GR, Spector SA. Autophagy induction by vitamin D inhibits both Mycobacterium tuberculosis and human immunodeficiency virus type 1. Autophagy (2012) 8:1523–5. doi:10.4161/auto.21154
76. Yuk J-M, Shin D-M, Lee H-M, Yang C-S, Jin HS, Kim K-K, et al. Vitamin D3 induces autophagy in human monocytes/macrophages via cathelicidin. Cell Host Microbe (2009) 6:231–43. doi:10.1016/j.chom.2009.08.004
77. Rekha RS, Rao Muvva SSVJ, Wan M, Raqib R, Bergman P, Brighenti S, et al. Phenylbutyrate induces LL-37-dependent autophagy and intracellular killing of Mycobacterium tuberculosis in human macrophages. Autophagy (2015) 11:1688–99. doi:10.1080/15548627.2015.1075110
78. Bradfute SB, Castillo EF, Arko-Mensah J, Chauhan S, Jiang S, Mandell M, et al. Autophagy as an immune effector against tuberculosis. Curr Opin Microbiol (2013) 16:355–65. doi:10.1016/j.mib.2013.05.003
79. Driss V, Legrand F, Hermann E, Loiseau S, Guerardel Y, Kremer L, et al. TLR2-dependent eosinophil interactions with mycobacteria: role of α-defensins. Blood (2009) 113:3235–44. doi:10.1182/blood-2008-07-166595
80. Duits LA, Ravensbergen B, Rademaker M, Hiemstra PS, Nibbering PH. Expression of beta-defensin 1 and 2 mRNA by human monocytes, macrophages and dendritic cells. Immunology (2002) 106:517–25. doi:10.1046/j.1365-2567.2002.01430.x
81. Rivas-Santiago B, Schwander SK, Sarabia C, Diamond G, Klein-Patel ME, Hernandez-Pando R, et al. Human {beta}-defensin 2 is expressed and associated with Mycobacterium tuberculosis during infection of human alveolar epithelial cells. Infect Immun (2005) 73:4505–11. doi:10.1128/IAI.73.8.4505-4511.2005
82. Dong H, Lv Y, Zhao D, Barrow P, Zhou X. Defensins: the case for their use against mycobacterial infections. J Immunol Res (2016) 2016:7515687. doi:10.1155/2016/7515687
83. Rivas-Santiago CE, Rivas-Santiago B, León DA, Castañeda-Delgado J, Hernández Pando R. Induction of β-defensins by l-isoleucine as novel immunotherapy in experimental murine tuberculosis. Clin Exp Immunol (2011) 164:80–9. doi:10.1111/j.1365-2249.2010.04313.x
84. Tan BH, Meinken C, Bastian M, Bruns H, Legaspi A, Ochoa MT, et al. Macrophages acquire neutrophil granules for antimicrobial activity against intracellular pathogens. J Immunol (2006) 177:1864–71. doi:10.4049/jimmunol.177.3.1864
85. Biragyn A, Ruffini PA, Leifer CA, Klyushnenkova E, Shakhov A, Chertov O, et al. Toll-like receptor 4-dependent activation of dendritic cells by beta-defensin 2. Science (2002) 298:1025–9. doi:10.1126/science.1075565
86. Kanamori Y, Murakami M, Matsui T, Funaba M. Hepcidin expression in liver cells: evaluation of mRNA levels and transcriptional regulation. Gene (2014) 546:50–5. doi:10.1016/j.gene.2014.05.040
87. Sow FB, Florence WC, Satoskar AR, Schlesinger LS, Zwilling BS, Lafuse WP. Expression and localization of hepcidin in macrophages: a role in host defense against tuberculosis. J Leukoc Biol (2007) 82:934–45. doi:10.1189/jlb.0407216
88. Sow FB, Nandakumar S, Velu V, Kellar KL, Schlesinger LS, Amara RR, et al. Mycobacterium tuberculosis components stimulate production of the antimicrobial peptide hepcidin. Tuberculosis (Edinb) (2011) 91:314–21. doi:10.1016/j.tube.2011.03.003
89. Sow FB, Sable SB, Plikaytis BB, Lafuse WP, Shinnick TM. Role of hepcidin in the innate immune response to Mycobacterium tuberculosis. FASEB J (2008) 22:556. doi:10.1096/fj.1530-6860
90. Pinto JP, Dias V, Zoller H, Porto G, Carmo H, Carvalho F, et al. Hepcidin messenger RNA expression in human lymphocytes. Immunology (2010) 130:217–30. doi:10.1111/j.1365-2567.2009.03226.x
91. Sow FB, Alvarez GR, Gross RP, Satoskar AR, Schlesinger LS, Zwilling BS, et al. Role of STAT1, NF- B, and C/EBP in the macrophage transcriptional regulation of hepcidin by mycobacterial infection and IFN. J Leukoc Biol (2009) 86:1247–58. doi:10.1189/jlb.1208719
92. Yamaji S. Inhibition of iron transport across human intestinal epithelial cells by hepcidin. Blood (2004) 104:2178–80. doi:10.1182/blood-2004-03-0829
93. Nemeth E, Ganz T. The role of hepcidin in iron metabolism. Acta Haematol (2009) 122:78–86. doi:10.1159/000243791
94. Ganz T. Hepcidin, a key regulator of iron metabolism and mediator of anemia of inflammation. Blood (2003) 102:783–8. doi:10.1182/blood-2003-03-0672
95. Close MJ, Howlett AR, Roskelley CD, Desprez PY, Bailey N, Rowning B, et al. Lactoferrin expression in mammary epithelial cells is mediated by changes in cell shape and actin cytoskeleton. J Cell Sci (1997) 110:2861–71.
96. Teng CT. Lactoferrin gene expression and regulation: an overview. Biochem Cell Biol (2002) 80:7–16. doi:10.1139/o01-215
97. Oseas R, Yang HH, Baehner RL, Boxer LA. Lactoferrin: a promoter of polymorphonuclear leukocyte adhesiveness. Blood (1981) 57:939–45.
98. Gifford JL, Hunter HN, Vogel HJ. Lactoferricin: a lactoferrin-derived peptide with antimicrobial, antiviral, antitumor and immunological properties. Cell Mol Life Sci (2005) 62:2588–98. doi:10.1007/s00018-005-5373-z
99. Arnold RR, Russell JE, Champion WJ, Brewer M, Gauthier JJ. Bactericidal activity of human lactoferrin: differentiation from the stasis of iron deprivation. Infect Immun (1982) 35:792–9.
100. Welsh KJ, Hwang S-A, Boyd S, Kruzel ML, Hunter RL, Actor JK. Influence of oral lactoferrin on Mycobacterium tuberculosis induced immunopathology. Tuberculosis (2011) 91:S105–13. doi:10.1016/j.tube.2011.10.019
101. Hwang S-A, Wilk KM, Bangale YA, Kruzel ML, Actor JK. Lactoferrin modulation of IL-12 and IL-10 response from activated murine leukocytes. Med Microbiol Immunol (2007) 196:171–80. doi:10.1007/s00430-007-0041-6
102. Hwang S-A, Kruzel ML, Actor JK. Oral recombinant human or mouse lactoferrin reduces Mycobacterium tuberculosis TDM induced granulomatous lung pathology. Biochem Cell Biol (2017) 95:148–54. doi:10.1139/bcb-2016-0061
103. Pereira HA, Spitznagel JK, Winton EF, Shafer WM, Martin LE, Guzman GS, et al. The ontogeny of a 57-Kd cationic antimicrobial protein of human polymorphonuclear leukocytes: localization to a novel granule population. Blood (1990) 76:825–34.
104. Jena P, Mohanty S, Mohanty T, Kallert S, Morgelin M, Lindstrøm T, et al. Azurophil granule proteins constitute the major mycobactericidal proteins in human neutrophils and enhance the killing of mycobacteria in macrophages. PLoS One (2012) 7:e50345. doi:10.1371/journal.pone.0050345
105. Fouret P, du Bois RM, Bernaudin JF, Takahashi H, Ferrans VJ, Crystal RG. Expression of the neutrophil elastase gene during human bone marrow cell differentiation. J Exp Med (1989) 169:833–45. doi:10.1084/jem.169.3.833
106. Wong KW, Jacobs WR. Mycobacterium tuberculosis exploits human interferon γ to stimulate macrophage extracellular trap formation and necrosis. J Infect Dis (2013) 208:109–19. doi:10.1093/infdis/jit097
107. Bieth J. Elastases: catalytic and biological properties. In: Mecham R, editor. Regul Matrix Accumulation. New York: Academic Press (1986). p. 217–320.
108. Pham CTN. Neutrophil serine proteases: specific regulators of inflammation. Nat Rev Immunol (2006) 6:541–50. doi:10.1038/nri1841
109. Steinwede K, Maus R, Bohling J, Voedisch S, Braun A, Ochs M, et al. Cathepsin G and neutrophil elastase contribute to lung-protective immunity against mycobacterial infections in mice. J Immunol (2012) 188:4476–87. doi:10.4049/jimmunol.1103346
110. Brinkmann V, Reichard U, Goosmann C, Fauler B, Uhlemann Y, Weiss DS, et al. Neutrophil extracellular traps kill bacteria. Science (2004) 303:1532–5. doi:10.1126/science.1092385
111. Je S, Quan H, Yoon Y, Na Y, Kim BJ, Seok SH. Mycobacterium massiliense induces macrophage extracellular traps with facilitating bacterial growth. PLoS One (2016) 11:e0155685. doi:10.1371/journal.pone.0155685
112. Boix E, Nogués MV. Mammalian antimicrobial proteins and peptides: overview on the RNase A superfamily members involved in innate host defence. Mol Biosyst (2007) 3:317–35. doi:10.1039/b617527a
113. Boix E, Salazar VA, Torrent M, Pulido D, Nogués MV, Moussaoui M. Structural determinants of the eosinophil cationic protein antimicrobial activity. Biol Chem (2012) 393:801–15. doi:10.1515/hsz-2012-0160
114. Becknell B, Eichler TE, Beceiro S, Li B, Easterling RS, Carpenter AR, et al. Ribonucleases 6 and 7 have antimicrobial function in the human and murine urinary tract. Kidney Int (2015) 87:151–61. doi:10.1038/ki.2014.268
115. Harder J, Schroder J-M. RNase 7, a novel innate immune defense antimicrobial protein of healthy human skin. J Biol Chem (2002) 277:46779–84. doi:10.1074/jbc.M207587200
116. Zhang J, Dyer KD, Rosenberg HF. Human RNase 7: a new cationic ribonuclease of the RNase A superfamily. Nucleic Acids Res (2003) 31:602–7. doi:10.1093/nar/gkg157
117. Pulido D, Torrent M, Andreu D, Nogues MV, Boix E. Two human host defense ribonucleases against mycobacteria, the eosinophil cationic protein (RNase 3) and RNase 7. Antimicrob Agents Chemother (2013) 57:3797–805. doi:10.1128/AAC.00428-13
118. Gruart V, Truong M-J, Plumas J, Zandecki M, Kusnierz J-P, Prin L, et al. Decreased expression of eosinophil peroxidase and major basic protein messenger RNAs during eosinophil maturation. Blood (1992) 79(10):2592–7.
119. Borelli V, Vita F, Shankar S, Soranzo MR, Banfi E, Scialino G, et al. Human eosinophil peroxidase induces surface alteration, killing, and lysis of Mycobacterium tuberculosis. Infect Immun (2003) 71:605–13. doi:10.1128/IAI.71.2.605-613.2003
120. Rivera-Marrero CA, Stewart J, Shafer WM, Roman J. The down-regulation of cathepsin G in THP-1 monocytes after infection with Mycobacterium tuberculosis is associated with increased intracellular survival of bacilli. Infect Immun (2004) 72:5712–21. doi:10.1128/IAI.72.10.5712-5721.2004
121. Berg RD, Levitte S, O’Sullivan MP, O’Leary SM, Cambier CJ, Cameron J, et al. Lysosomal disorders drive susceptibility to tuberculosis by compromising macrophage migration. Cell (2016) 165:139–52. doi:10.1016/j.cell.2016.02.034
122. Walter K, Steinwede K, Aly S, Reinheckel T, Bohling J, Maus UA, et al. Cathepsin G in experimental tuberculosis: relevance for antibacterial protection and potential for immunotherapy. J Immunol (2015) 195:3325–33. doi:10.4049/jimmunol.1501012
123. Stenger S, Hanson DA, Teitelbaum R, Dewan P, Niazi KR, Froelich CJ, et al. An antimicrobial activity of cytolytic T cells mediated by granulysin. Science (1998) 282:121–5. doi:10.1126/science.282.5386.121
124. Ross KF, Herzberg MC. Calprotectin expression by gingival epithelial cells. Infect Immun (2001) 69:3248–54. doi:10.1128/IAI.69.5.3248-3254.2001
125. Bagheri V. S100A12: friend or foe in pulmonary tuberculosis? Cytokine (2017) 92:80–2. doi:10.1016/j.cyto.2017.01.009
126. Dhiman R, Venkatasubramanian S, Paidipally P, Barnes PF, Tvinnereim A, Vankayalapati R. Interleukin 22 inhibits intracellular growth of Mycobacterium tuberculosis by enhancing calgranulin a expression. J Infect Dis (2014) 209:578–87. doi:10.1093/infdis/jit495
127. Mork G, Schjerven H, Mangschau L, Soyland E, Brandtzaeg P. Proinflammatory cytokines upregulate expression of calprotectin (L1 protein, MRP-8/MRP-14) in cultured human keratinocytes. Br J Dermatol (2003) 149:484–91. doi:10.1046/j.1365-2133.2003.05536.x
128. Lusitani D, Malawista SE, Montgomery RR. Calprotectin, an abundant cytosolic protein from human polymorphonuclear leukocytes, inhibits the growth of Borrelia burgdorferi. Infect Immun (2003) 71:4711–6. doi:10.1128/IAI.71.8.4711-4716.2003
129. Alonso S, Pethe K, Russell DG, Purdy GE. Lysosomal killing of Mycobacterium mediated by ubiquitin-derived peptides is enhanced by autophagy. Proc Natl Acad Sci U S A (2007) 104:6031–6. doi:10.1073/pnas.0700036104
130. Martineau AR, Newton SM, Wilkinson KA, Kampmann B, Hall BM, Nawroly N, et al. Neutrophil-mediated innate immune resistance to mycobacteria. J Clin Invest (2007) 117:1988–94. doi:10.1172/JCI31097
131. Bandurska K, Berdowska A, Barczyńska-Felusiak R, Krupa P. Unique features of human cathelicidin LL-37. Biofactors (2015) 41:289–300. doi:10.1002/biof.1225
132. Zanetti M, Gennaro R, Romeo D. Cathelicidins: a novel protein family with a common proregion and a variable C-terminal antimicrobial domain. FEBS Lett (1995) 374:1–5. doi:10.1016/0014-5793(95)01050-O
133. Sørensen OE, Follin P, Johnsen AH, Calafat J, Tjabringa GS, Hiemstra PS, et al. Human cathelicidin, hCAP-18, is processed to the antimicrobial peptide LL-37 by extracellular cleavage with proteinase 3. Blood (2001) 97:3951–9. doi:10.1182/blood.V97.12.3951
134. Liu PT, Stenger S, Tang DH, Modlin RL. Cutting edge: vitamin D-mediated human antimicrobial activity against Mycobacterium tuberculosis is dependent on the induction of cathelicidin. J Immunol (2007) 179:2060–3. doi:10.4049/jimmunol.179.4.2060
135. Lin W, Florez de Sessions P, Teoh GHK, Mohamed ANN, Zhu YO, Koh VHQ, et al. Transcriptional profiling of Mycobacterium tuberculosis exposed to in vitro lysosomal stress. Infect Immun (2016) 84:2505–23. doi:10.1128/IAI.00072-16
136. Kisich KO, Heifets L, Higgins M, Diamond G. Antimycobacterial agent based on mRNA encoding human β-defensin 2 enables primary macrophages to restrict growth of Mycobacterium tuberculosis. Infect Immun (2001) 69:2692–9. doi:10.1128/IAI.69.4.2692-2699.2001
137. Ganz T. Defensins: antimicrobial peptides of innate immunity. Nat Rev Immunol (2003) 3:710–20. doi:10.1038/nri1180
138. Faurschou M, Kamp S, Cowland JB, Udby L, Johnsen AH, Calafat J, et al. Prodefensins are matrix proteins of specific granules in human neutrophils. J Leukoc Biol (2005) 78:785–93. doi:10.1189/jlb.1104688
139. Jacobsen M, Repsilber D, Gutschmidt A, Neher A, Feldmann K, Mollenkopf HJ, et al. Candidate biomarkers for discrimination between infection and disease caused by Mycobacterium tuberculosis. J Mol Med (2007) 85:613–21. doi:10.1007/s00109-007-0157-6
140. Kisich KO, Higgins M, Diamond G, Heifets L. Tumor necrosis factor alpha stimulates killing of Mycobacterium tuberculosis by human neutrophils. Infect Immun (2002) 70:4591–9. doi:10.1128/IAI.70.8.4591
141. Kalita A, Verma I, Khuller GK. Role of human neutrophil peptide-1 as a possible adjunct to antituberculosis chemotherapy. J Infect Dis (2004) 190:1476–80. doi:10.1086/424463
142. Park CH, Valore EV, Waring AJ, Ganz T. Hepcidin, a urinary antimicrobial peptide synthesized in the liver. J Biol Chem (2001) 276:7806–10. doi:10.1074/jbc.M008922200
143. Michels K, Nemeth E, Ganz T, Mehrad B. Hepcidin and host defense against infectious diseases. PLoS Pathog (2015) 11:e1004998. doi:10.1371/journal.ppat.1004998
144. Morris CD, Bird AR, Nell H. The haematological and biochemical changes in severe pulmonary tuberculosis. Q J Med (1989) 73:1151–9.
145. Javaheri-Kermani M, Farazmandfar T, Ajami A, Yazdani Y. Impact of hepcidin antimicrobial peptide on iron overload in tuberculosis patients. Scand J Infect Dis (2014) 46:693–6. doi:10.3109/00365548.2014.929736
146. Andersson DI, Hughes D, Kubicek-Sutherland JZ. Mechanisms and consequences of bacterial resistance to antimicrobial peptides. Drug Resist Updat (2016) 26:43–57. doi:10.1016/j.drup.2016.04.002
147. Hwang S-A, Kruzel ML, Actor JK. CHO expressed recombinant human lactoferrin as an adjuvant for BCG. Int J Immunopathol Pharmacol (2015) 28:452–68. doi:10.1177/0394632015599832
148. Thom RE, Elmore MJ, Williams A, Andrews SC, Drobniewski F, Marsh PD, et al. The expression of ferritin, lactoferrin, transferrin receptor and solute carrier family 11A1 in the host response to BCG-vaccination and Mycobacterium tuberculosis challenge. Vaccine (2012) 30:3159–68. doi:10.1016/j.vaccine.2012.03.008
149. Jensen-Jarolim E, Pacios LF, Bianchini R, Hofstetter G, Roth-Walter F. Structural similarities of human and mammalian lipocalins, and their function in innate immunity and allergy. Allergy (2016) 71:286–94. doi:10.1111/all.12797
150. Soehnlein O, Zernecke A, Eriksson EE, Rothfuchs AG, Pham CT, Herwald H, et al. Neutrophil secretion products pave the way for inflammatory monocytes. Blood (2008) 112:1461–71. doi:10.1182/blood-2008-02-139634
151. Tapper H, Karlsson A, Mörgelin M, Flodgaard H, Herwald H. Secretion of heparin-binding protein from human neutrophils is determined by its localization in azurophilic granules and secretory vesicles. Blood (2002) 99:1785–93. doi:10.1182/blood.V99.5.1785
152. Soehnlein O, Kai-Larsen Y, Frithiof R, Sorensen OE, Kenne E, Scharffetter-Kochanek K, et al. Neutrophil primary granule proteins HBP and HNP1-3 boost bacterial phagocytosis by human and murine macrophages. J Clin Invest (2008) 118:3491–502. doi:10.1172/JCI35740
153. Shafer WM, Martin LE, Spitznagel JK. Late intraphagosomal hydrogen ion concentration favors the in vitro antimicrobial capacity of a 37-kilodalton cationic granule protein of human neutrophil granulocytes. Infect Immun (1986) 53:651–5.
154. Ribeiro-Gomes FL, Moniz-de-Souza MCA, Alexandre-Moreira MS, Dias WB, Lopes MF, Nunes MP, et al. Neutrophils activate macrophages for intracellular killing of Leishmania major through recruitment of TLR4 by neutrophil elastase. J Immunol (2007) 179:3988–94. doi:10.4049/jimmunol.179.6.3988
155. Belaaouaj A, McCarthy R, Baumann M, Gao Z, Ley TJ, Abraham SN, et al. Mice lacking neutrophil elastase reveal impaired host defense against gram negative bacterial sepsis. Nat Med (1998) 4:615–8. doi:10.1038/nm0598-615
156. Pires D, Marques J, Pombo JP, Carmo N, Bettencourt P, Neyrolles O, et al. Role of cathepsins in Mycobacterium tuberculosis survival in human macrophages. Sci Rep (2016) 6:32247. doi:10.1038/srep32247
157. Meijer AH. Protection and pathology in TB: learning from the zebrafish model. Semin Immunopathol (2016) 38:261–73. doi:10.1007/s00281-015-0522-4
158. Pitabut N, Sakurada S, Tanaka T, Ridruechai C, Tanuma J, Aoki T, et al. Potential function of granulysin, other related effector molecules and lymphocyte subsets in patients with TB and HIV/TB coinfection. Int J Med Sci (2013) 10:1003–14. doi:10.7150/ijms.6437
159. Saiga H, Shimada Y, Takeda K. Innate immune effectors in mycobacterial infection. Clin Dev Immunol (2011) 2011:347594. doi:10.1155/2011/347594
160. Foss MH, Powers KM, Purdy GE. Structural and functional characterization of mycobactericidal ubiquitin-derived peptides in model and bacterial membranes. Biochemistry (2012) 51:9922–9. doi:10.1021/bi301426j
161. Koczera P, Martin L, Marx G, Schuerholz T. The ribonuclease a superfamily in humans: canonical RNases as the buttress of innate immunity. Int J Mol Sci (2016) 17:E1278. doi:10.3390/ijms17081278
162. Bystrom J, Amin K, Bishop-Bailey D. Analysing the eosinophil cationic protein – a clue to the function of the eosinophil granulocyte. Respir Res (2011) 12:10. doi:10.1186/1465-9921-12-10
163. Monteseirín J, Vega A, Chacón P, Camacho MJ, El Bekay R, Asturias JA, et al. Neutrophils as a novel source of eosinophil cationic protein in IgE-mediated processes. J Immunol (2007) 179:2634–41. doi:10.4049/jimmunol.179.4.2634
164. Liu Y-S, Tsai P-W, Wang Y, Fan T, Hsieh C-H, Chang M, et al. Chemoattraction of macrophages by secretory molecules derived from cells expressing the signal peptide of eosinophil cationic protein. BMC Syst Biol (2012) 6:105. doi:10.1186/1752-0509-6-105
165. Vijayan VK, Reetha AM, Jawahar MS, Sankaran K, Prabhakar R. Pulmonary eosinophilia in pulmonary tuberculosis. Chest (1992) 101:1708–9. doi:10.1378/chest.101.6.1708
166. Kita H. Eosinophils: multifaceted biological properties and roles in health and disease. Immunol Rev (2011) 242:161–77. doi:10.1111/j.1600-065X.2011.01026.x
167. Arranz-Trullén J, Lu L, Pulido D, Bhakta S, Boix E. Unveiling the mode of action of human antimicrobial RNases against Mycobacterium tuberculosis using a surrogate macrophage infected model. TB Summit 2016. London (2016).
168. Gupta SK, Haigh BJ, Griffin FJ, Wheeler TT. The mammalian secreted RNases: mechanisms of action in host defence. Innate Immun (2012) 19:86–97. doi:10.1177/1753425912446955
169. Yang D, Chen Q, Su SB, Zhang P, Kurosaka K, Caspi RR, et al. Eosinophil-derived neurotoxin acts as an alarmin to activate the TLR2-MyD88 signal pathway in dendritic cells and enhances Th2 immune responses. J Exp Med (2008) 205:79–90. doi:10.1084/jem.20062027
170. Jiang Z, Higgins MP, Whitehurst J, Kisich KO, Voskuil MI, Hodges RS. Anti-tuberculosis activity of α-helical antimicrobial peptides: de novo designed L- and D-enantiomers versus L- and D-LL-37. Protein Pept Lett (2011) 18:241–52. doi:10.2174/092986611794578288
171. Kapoor R, Eimerman PR, Hardy JW, Cirillo JD, Contag CH, Barron AE. Efficacy of antimicrobial peptoids against Mycobacterium tuberculosis. Antimicrob Agents Chemother (2011) 55:3058–62. doi:10.1128/AAC.01667-10
173. Ramón-García S, Mikut R, Ng C, Ruden S, Volkmer R, Reischl M, et al. Targeting Mycobacterium tuberculosis and other microbial pathogens using improved synthetic antibacterial peptides. Antimicrob Agents Chemother (2013) 57:2295–303. doi:10.1128/AAC.00175-13
174. Rivas-Santiago B, Rivas Santiago CE, Castañeda-Delgado JE, León-Contreras JC, Hancock REW, Hernandez-Pando R. Activity of LL-37, CRAMP and antimicrobial peptide-derived compounds E2, E6 and CP26 against Mycobacterium tuberculosis. Int J Antimicrob Agents (2013) 41:143–8. doi:10.1016/j.ijantimicag.2012.09.015
175. Lan Y, Lam JT, Siu GKH, Yam WC, Mason AJ, Lam JKW. Cationic amphipathic D-enantiomeric antimicrobial peptides with in vitro and ex vivo activity against drug-resistant Mycobacterium tuberculosis. Tuberculosis (2014) 94:678–89. doi:10.1016/j.tube.2014.08.001
176. Kwok PCL, Grabarek A, Chow MYT, Lan Y, Li JCW, Casettari L, et al. Inhalable spray-dried formulation of D-LAK antimicrobial peptides targeting tuberculosis. Int J Pharm (2015) 491:367–74. doi:10.1016/j.ijpharm.2015.07.001
177. Spindler EC, Hale JDF, Giddings TH, Hancock REW, Gill RT. Deciphering the mode of action of the synthetic antimicrobial peptide bac8c. Antimicrob Agents Chemother (2011) 55:1706–16. doi:10.1128/AAC.01053-10
178. Llamas-González YY, Pedroza-Roldán C, Cortés-Serna MB, Márquez-Aguirre AL, Gálvez-Gastélum FJ, Flores-Valdez MA. The synthetic cathelicidin HHC-10 inhibits Mycobacterium bovis BCG in vitro and in C57BL/6 mice. Microb Drug Resist (2013) 19:124–9. doi:10.1089/mdr.2012.0149
179. Cherkasov A, Hilpert K, Jenssen H, Fjell CD, Waldbrook M, Mullaly SC, et al. Use of artificial intelligence in the design of small peptide antibiotics effective against a broad spectrum of highly antibiotic-resistant superbugs. ACS Chem Biol (2009) 4:65–74. doi:10.1021/cb800240j
180. Mansour SC, de la Fuente-Nunez C, Hancock REW. Peptide IDR-1018: modulating the immune system and targeting bacterial biofilms to treat antibiotic-resistant bacterial infections. J Pept Sci (2015) 21:323–9. doi:10.1002/psc.2708
181. Rivas-Santiago B, Castañeda-Delgado JE, Rivas Santiago CE, Waldbrook M, González-Curiel I, León-Contreras JC, et al. Ability of innate defence regulator peptides IDR-1002, IDR-HH2 and IDR-1018 to protect against Mycobacterium tuberculosis infections in animal models. PLoS One (2013) 8:e59119. doi:10.1371/journal.pone.0059119
182. Huante-Mendoza A, Silva-García O, Oviedo-Boyso J, Hancock REW, Baizabal-Aguirre VM. Peptide IDR-1002 inhibits NF-κB nuclear translocation by inhibition of IκBα degradation and activates p38/ERK1/2–MSK1-dependent CREB phosphorylation in macrophages stimulated with lipopolysaccharide. Front Immunol (2016) 7:533. doi:10.3389/fimmu.2016.00533
183. Chingaté S, Delgado G, Salazar LM, Soto CY. The ATPase activity of the mycobacterial plasma membrane is inhibited by the LL37-analogous peptide LLAP. Peptides (2015) 71:222–8. doi:10.1016/j.peptides.2015.07.021
184. Silva JP, Gonçalves C, Costa C, Sousa J, Silva-Gomes R, Castro AG, et al. Delivery of LLKKK18 loaded into self-assembling hyaluronic acid nanogel for tuberculosis treatment. J Control Release (2016) 235:112–24. doi:10.1016/j.jconrel.2016.05.064
185. Ghobrial O, Derendorf H, Hillman JD. Pharmacokinetic and pharmacodynamic evaluation of the lantibiotic MU1140. J Pharm Sci (2010) 99:2521–8. doi:10.1002/jps.22015
186. Rodríguez A, Villegas E, Montoya-Rosales A, Rivas-Santiago B, Corzo G. Characterization of antibacterial and hemolytic activity of synthetic pandinin 2 variants and their inhibition against Mycobacterium tuberculosis. PLoS One (2014) 9:e101742. doi:10.1371/journal.pone.0101742
187. Khara JS, Wang Y, Ke X-Y, Liu S, Newton SM, Langford PR, et al. Anti-mycobacterial activities of synthetic cationic α-helical peptides and their synergism with rifampicin. Biomaterials (2014) 35:2032–8. doi:10.1016/j.biomaterials.2013.11.035
188. Vermeer LS, Lan Y, Abbate V, Ruh E, Bui TT, Wilkinson LJ, et al. Conformational flexibility determines selectivity and antibacterial, antiplasmodial, and anticancer potency of cationic α-helical peptides. J Biol Chem (2012) 287:34120–33. doi:10.1074/jbc.M112.359067
189. Torrent M, Pulido D, Valle J, Nogués MV, Andreu D, Boix E. Ribonucleases as a host-defence family: evidence of evolutionarily conserved antimicrobial activity at the N-terminus. Biochem J (2013) 456:99–108. doi:10.1042/BJ20130123
190. Torrent M, Pulido D, Nogués MV, Boix E. Exploring new biological functions of amyloids: bacteria cell agglutination mediated by host protein aggregation. PLoS Pathog (2012) 8:e1003005. doi:10.1371/journal.ppat.1003005
191. Hilchie AL, Wuerth K, Hancock REW. Immune modulation by multifaceted cationic host defense (antimicrobial) peptides. Nat Chem Biol (2013) 9:761–8. doi:10.1038/nchembio.1393
192. Fosgerau K, Hoffmann T. Peptide therapeutics: current status and future directions. Drug Discov Today (2014) 20:122–8. doi:10.1016/j.drudis.2014.10.003
193. Goldman MJ, Anderson GM, Stolzenberg ED, Kari UP, Zasloff M, Wilson JM. Human beta-defensin-1 is a salt-sensitive antibiotic in lung that is inactivated in cystic fibrosis. Cell (1997) 88:553–60. doi:10.1016/S0092-8674(00)81895-4
194. Hilpert K, Volkmer-Engert R, Walter T, Hancock REW. High-throughput generation of small antibacterial peptides with improved activity. Nat Biotechnol (2005) 23:1008–12. doi:10.1038/nbt1113
195. Chen Y, Mant CT, Farmer SW, Hancock REW, Vasil ML, Hodges RS. Rational design of alpha-helical antimicrobial peptides with enhanced activities and specificity/therapeutic index. J Biol Chem (2005) 280:12316–29. doi:10.1074/jbc.M413406200
196. Nordström R, Malmsten M. Delivery systems for antimicrobial peptides. Adv Colloid Interface Sci (2017) 242:17–34. doi:10.1016/j.cis.2017.01.005
197. Zhang J, Zhang X, Liu G, Chang D, Liang X, Zhu X, et al. Intracellular trafficking network of protein nanocapsules: endocytosis, exocytosis and autophagy. Theranostics (2016) 6:2099–113. doi:10.7150/thno.16587
198. Hamley I. Peptide fibrillization. Angw Chem Int Ed Eng (2007) 46:8128–47. doi:10.1002/anie.200700861
199. Torrent M, Valle J, Nogués MV, Boix E, Andreu D. The generation of antimicrobial peptide activity: a trade-off between charge and aggregation? Angw Chem Int Ed Eng (2011) 50:10686–9. doi:10.1002/anie.201103589
200. Mikut R, Ruden S, Reischl M, Breitling F, Volkmer R, Hilpert K. Improving short antimicrobial peptides despite elusive rules for activity. Biochim Biophys Acta (2016) 1858:1024–33. doi:10.1016/j.bbamem.2015.12.013
201. Haney EF, Mansour SC, Hilchie AL, de la Fuente-Núñez C, Hancock RE. High throughput screening methods for assessing antibiofilm and immunomodulatory activities of synthetic peptides. Peptides (2015) 71:276–85. doi:10.1016/j.peptides.2015.03.015
202. Sim S, Kim Y, Kim T, Lim S, Lee M. Directional assembly of α-helical peptides induced by cyclization. J Am Chem Soc (2012) 134:20270–2. doi:10.1021/ja3098756
203. Bowdish DME, Davidson DJ, Lau YE, Lee K, Scott MG, Hancock REW. Impact of LL-37 on anti-infective immunity. J Leukoc Biol (2005) 77:451–9. doi:10.1189/jlb.0704380
204. Zumla A, Rao M, Wallis RS, Kaufmann SHE, Rustomjee R, Mwaba P, et al. Host-directed therapies for infectious diseases: current status, recent progress, and future prospects. Lancet Infect Dis (2016) 16:e47–63. doi:10.1016/S1473-3099(16)00078-5
205. Ottosson H, Nylén F, Sarker P, Miraglia E, Bergman P, Gudmundsson GH, et al. Highly potent inducers of endogenous antimicrobial peptides for host directed therapy of infections. Sci Rep (2016) 6:36692. doi:10.1038/srep36692
206. Rivas-Santiago B, Rivas-Santiago C, Sada E, Hernandez-Pando R. Prophylactic potential of defensins and L-isoleucine in tuberculosis household contacts: an experimental model. Immunotherapy (2015) 7:207–13. doi:10.2217/imt.14.119
207. Liu PT, Stenger S, Li H, Wenzel L, Tan BH, Krutzik SR, et al. Toll-like receptor triggering of a vitamin D-mediated human antimicrobial response. Science (2006) 311:1770–3. doi:10.1126/science.1123933
208. Lofton H, Pränting M, Thulin E, Andersson DI. Mechanisms and fitness costs of resistance to antimicrobial peptides LL-37, CNY100HL and wheat germ histones. PLoS One (2013) 8:e68875. doi:10.1371/journal.pone.0068875
209. Kraus D, Peschel A. Molecular mechanisms of bacterial resistance to antimicrobial peptides. Curr Top Microbiol Immunol (2006) 306:231–50.
210. Adams KN, Takaki K, Connolly LE, Wiedenhoft H, Winglee K, Humbert O, et al. Drug tolerance in replicating mycobacteria mediated by a macrophage-induced efflux mechanism. Cell (2011) 145:39–53. doi:10.1016/j.cell.2011.02.022
211. Szumowski JD, Adams KN, Edelstein PH, Ramakrishnan L. Antimicrobial efflux pumps and Mycobacterium tuberculosis drug tolerance: evolutionary considerations. Curr Top Microbiol Immunol (2013) 374:81–108. doi:10.1007/82_2012_300
212. Rios AC, Moutinho CG, Pinto FC, Del Fiol FS, Jozala A, Chaud MV, et al. Alternatives to overcoming bacterial resistances: state-of-the-art. Microbiol Res (2016) 191:51–80. doi:10.1016/j.micres.2016.04.008
213. Gao LY, Laval F, Lawson EH, Groger RK, Woodruff A, Morisaki JH, et al. Requirement for kasB in Mycobacterium mycolic acid biosynthesis, cell wall impermeability and intracellular survival: implications for therapy. Mol Microbiol (2003) 49:1547–63. doi:10.1046/j.1365-2958.2003.03667.x
214. Gryllos I, Tran-Winkler HJ, Cheng M-F, Chung H, Bolcome R, Lu W, et al. Induction of group A Streptococcus virulence by a human antimicrobial peptide. Proc Natl Acad Sci U S A (2008) 105:16755–60. doi:10.1073/pnas.0803815105
215. Brown ED, Wright GD. Antibacterial drug discovery in the resistance era. Nature (2016) 529:336–43. doi:10.1038/nature17042
216. Bauer ME, Shafer WM. On the in vivo significance of bacterial resistance to antimicrobial peptides. Biochim Biophys Acta (2015) 1848:3101–11. doi:10.1016/j.bbamem.2015.02.012
217. Fattorini L, Gennaro R, Zanetti M, Tan D, Brunori L, Giannoni F, et al. In vitro activity of protegrin-1 and beta-defensin-1, alone and in combination with isoniazid, against Mycobacterium tuberculosis. Peptides (2004) 25:1075–7. doi:10.1016/j.peptides.2004.04.003
218. Nylen F, Bergman P, Gudmundsson GH, Agerberth B. Assays for identifying inducers of the antimicrobial peptide LL-37. Methods Mol Biol (2017) 1548:271–81. doi:10.1007/978-1-4939-6737-7_19
219. Ramos-Espinosa O, Hernández-Bazán S, Francisco-Cruz A, Mata-Espinosa D, Barrios-Payán J, Marquina-Castillo B, et al. Gene therapy based in antimicrobial peptides and proinflammatory cytokine prevents reactivation of experimental latent tuberculosis. Pathog Dis (2016) 74:ftw075. doi:10.1093/femspd/ftw075
Keywords: antimicrobial peptides, innate immunity, tuberculosis, infectious diseases, mycobacteria, antimicrobial resistance, host defense
Citation: Arranz-Trullén J, Lu L, Pulido D, Bhakta S and Boix E (2017) Host Antimicrobial Peptides: The Promise of New Treatment Strategies against Tuberculosis. Front. Immunol. 8:1499. doi: 10.3389/fimmu.2017.01499
Received: 26 April 2017; Accepted: 24 October 2017;
Published: 07 November 2017
Edited by:
Uday Kishore, Brunel University London, United KingdomReviewed by:
Suraj Sable, Centers for Disease Control and Prevention, United StatesKushagra Bansal, Harvard Medical School, United States
Copyright: © 2017 Arranz-Trullén, Lu, Pulido, Bhakta and Boix. This is an open-access article distributed under the terms of the Creative Commons Attribution License (CC BY). The use, distribution or reproduction in other forums is permitted, provided the original author(s) or licensor are credited and that the original publication in this journal is cited, in accordance with accepted academic practice. No use, distribution or reproduction is permitted which does not comply with these terms.
*Correspondence: Ester Boix, RXN0ZXIuQm9peCYjeDAwMDQwO3VhYi5jYXQ=;
Sanjib Bhakta, cy5iaGFrdGEmI3gwMDA0MDtiYmsuYWMudWs=
†Present address: David Pulido, Nuffield Department of Medicine, University of Oxford, Oxford, United Kingdom
‡These authors have contributed equally to this work.