- Instituto de Medicina Molecular, Faculdade de Medicina da Universidade de Lisboa, Lisbon, Portugal
The trillions of microorganisms that reside in the gastrointestinal tract, essential for nutrient absorption, are kept under control by a single cell barrier and large amounts of immune cells. Intestinal epithelial cells (IECs) are critical in establishing an environment supporting microbial colonization and immunological tolerance. A large population of CD8+ T cells is in direct and constant contact with the IECs and the intraepithelial lymphocytes (IELs). Due to their location, at the interphase of the intestinal lumen and external environment and the host tissues, they seem ideally positioned to balance immune tolerance and protection to preserve the fragile intestinal barrier from invasion as well as immunopathology. IELs are a heterogeneous population, with a large innate-like contribution of unknown specificity, intercalated with antigen-specific tissue-resident memory T cells. In this review, we provide a comprehensive overview of IEL physiology and how they interact with the IECs and contribute to immune surveillance to preserve intestinal homeostasis and host-microbial relationships.
Introduction
The intestinal epithelia are a single cell layer of large surface. Together with a mucus layer, the epithelia form a dynamic physical barrier between the host and its environment. Estimates are up to 100 trillion microorganisms, including pathogens, have made the gastrointestinal tract their home (1), which makes the intestine the largest potential port for microbial invasion. However, a proportion of the microorganisms in the intestine can contribute to the hosts’ health and immunity. These commensal bacteria compete for resources with pathogenic microorganisms and provide metabolic capacity to digest food products by generating important compounds (e.g., vitamin K) or by assisting other microorganisms with supportive roles. The delicate nature of the single cell epithelial barrier, the essential function of the gastrointestinal tract to absorb nutrients and liquids, and the balance to maintain beneficial microbes, while offering protection against invasion and avoiding tissue damage, requires an effective and robust, yet tolerant, immune system.
The intestinal immune surveillance network is an integrated part of the organ, which enables it to swiftly pick up cues regarding its health status and contributes to tissue homeostasis as well as repair. Immune surveillance links rapid activation of innate immune cells to the more delayed recruitment of adaptive immune cells (2), ultimately resulting in immunological memory. Part of the innate system is the intestinal epithelial cells (IECs) themselves as well as classical innate immune cells. Mostly, macrophages, monocytes, and dendritic cells (DCs) migrate to the intestine from the bone marrow via blood (3). Following infection, interactions between antigen presenting cells and lymphocytes can take place in specialized structures, unique to the intestine, such as isolated lymphoid follicles and Peyer’s patches (4).
T-lymphocytes recognize foreign particles (antigens) by their surface expressed T cell receptor (TCR). With each T cell expressing a nearly unique TCR, collectively T cells can recognize nearly all foreign antigens. From the two major types of T cells found in blood and secondary lymphoid organs (SLO), CD4 expressing helper T (TH) cells are generated in the thymus as precursors without a defined function. They recognize antigens presented in major histocompatibility complexes class II (MHCII) after processing by antigen presenting cells. TH cells have an important orchestrating role, differentiating into effector cells with distinct supportive functions in type 1 (TH1), type 2 (TH2), and type 3 (TH17) immunity and high levels of flexibility (5, 6). Specialized regulatory T cells can curtail responses and form part of a carefully balanced immune system (7). CD8 expressing cytotoxic T cells similarly derive from the thymus as naive cells. They mainly recognize antigens resulting from the target cells’ transcriptional machinery and degradation of cytosolic proteins by the proteasome presented in MHCI, such as those resulting from viral infections as well as intracellular bacterial infections. Upon encountering their cognate antigen, CD8+ T cells differentiate into effector cells, classically thought to be part of type 1 immunity due to their high potential for interferon (IFN)γ production.
The maintenance of effector T cells is metabolically costly. Rapidly dividing cells require large amounts of energy for the production of cellular building blocks and secretion of effector molecules. These cells can potentially contribute to chronic inflammation and immunopathology. To avoid such possible danger and energy expense, the majority of effector cells undergo apoptosis after pathogen clearance, re-establishing homeostasis. Yet, some persist as memory cells, providing protection against re-infection. Memory CD8 T cells are a heterogeneous population, varying in phenotype, function, and localization (8) (Figure 1). This facilitates a swift and tailored response to a broad array of potential insults. In addition, the intestinal immune system has another important population of specialized CD8+ T-lymphocytes known as intraepithelial lymphocytes (IELs) (9). Intriguingly, IELs have characteristics of naive, effector, and memory cells require bidirectional cross-talk with IECs (10) (Figure 1), with one murine IEL estimated to be present for every 4–10 IECs (11, 12).
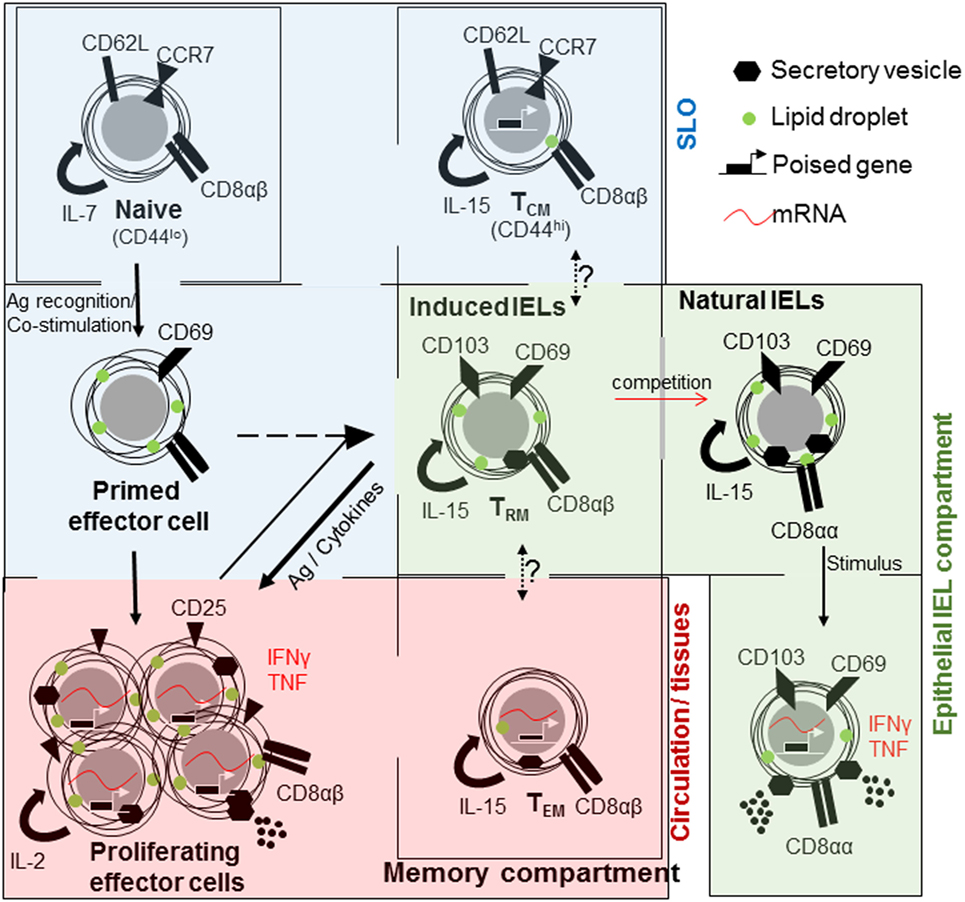
Figure 1. The relationships between CD8+ T cell populations in the small intestine. Naive CD8+ T cells (top left) are maintained in a quiescent state within their own compartment under homeostatic control. They mainly circulate through the secondary lymphoid organs (SLO). Upon encountering antigen, T cells are primed, acquire cellular building blocks such as lipids, and express CD69. Thereafter, they undergo rapid proliferation and express CD25 [high affinity interleukin (IL)-2 receptor], cytokines such as tumor necrosis factor (TNF) and interferon (IFN)γ and can release cytolytic factors, as effector T cells. Large proportions or effector T cells will die by apoptosis. Memory cells are derived from primed or effector T cells of which three subsets are distinguished; central memory T cell (TCM) that is present in the SLO, effector memory T cells (TEM) that are circulating and rapidly acquire effector functions and tissue-resident cells (TRM) in tissues, especially barrier sites, such as the skin and intestine. All memory cells rely on IL-15 for their maintenance. At barrier sites TRM cells compete with natural intraepithelial lymphocytes (IELs), both maintained in a semi-activated state expressing CD69 and CD103 and metabolically charged.
Aberrant immunity has severe consequences, especially in the intestine where a single epithelial cell layer forms the barrier between the host and a very high amount of microorganisms. Immunity against commensal bacteria can result in chronic inflammation, such as observed in inflammatory bowel diseases (IBDs). In this review, we focus on CD8 expressing T cells, particularly IELs, which, located in the very top layer of the intestinal barrier, are ideally positioned to monitor the intestinal microbiota. They may contribute to modulating immunity toward microbes as well as immunopathology, and are involved in tissue homeostasis and epithelial repair. We will discuss some of the properties of IELs and speculate on their role in the intestinal immune surveillance network.
Conventional CD8 T Cells
The initiation of an adaptive immune response requires several myeloid and lymphoid cell types. These cells need to be brought together and act in a strictly orchestrated manner in time and space to license immune cell activation (13). Critical interactions are those between antigen presenting cells, especially DCs and T cells (14). In order to become fully activated, naive T cells require signaling through TCR (signal 1) as well as costimulatory receptors (signal 2), such as CD28 and CD40. Additional cues (signal 3) provide inflammatory context and involve cytokines and chemokines (15, 16).
During the initiation phase, naive CD8+ T cells rapidly proliferate and differentiate into cytotoxic T-lymphocyte effector cells thereby gaining the ability to kill target cells by releasing perforin and granzymes, and secrete large amounts of cytokines, such as tumor necrosis factor (TNF) and IFNs (17) (Figure 1). The rapid proliferation during the expansion phase ensures that a limited number of precursor cells can counter infectious agents. Effector cells migrate to most tissues in the body to ensure the removal of all infected cells and pathogens (18). However, such a response cannot be sustained and proximally 95% of effector cells die in a contraction phase upon pathogen clearance (19).
A limited number of cells develop into memory cells, returning to a state of quiescence with slow cell turn over and effector molecule transcription. Despite this they are able to rapidly reactivate, proliferate, and express effector molecules upon reencounter with a similar pathogen (18, 20–23). How memory T cells develop remains incompletely understood. There are different signals influencing T cells upon and after encountering their cognate antigen that influence the size and quality of the T cell memory pool (8).
Three subtypes of memory T cells are recognized, they are; effector memory (TEM) T cells, central memory (TCM) T cells (18, 24, 25), and tissue-resident memory (TRM) cells expressing CD69 and CD103 (26–29) (Figure 1). Differences in cell localization, recall ability, and effector functions provide intersecting levels of protection against re-infection (30). Memory cells found circulating through blood, lymph, and SLO are referred to as TCM cells and express CD62L and CCR7, which enable entry in lymphoid organs and circulation (31–34). Those cells primarily found in non-lymphoid tissues are TEM cells (18, 22).
Although migration of T cells is a pillar of successful immune defense, experiments using defined tissue grafts from ganglia, skin, and intestine as well as the use of parabiosis have defined a residential population of memory T cells (27, 35–37). At epithelial barrier sites such as the skin, lungs, reproductive organs, and gastrointestinal tract, a unique memory population is found; TRM cells. These cells share characteristics with TEM cells, expressing CD44 and low levels of CD62L (Figure 1). They are found at the initial site of infection, providing very regional immune surveillance and protection against re-infection (35, 38, 39), and do not recirculate (40). The discovery of TRM cells and subsequent detailed analysis have resulted in a paradigm shift that most memory T cells are an integral part of non-lymphoid tissues (41). But, these cells did not settle on empty ground to fill a previously non-existent niche. TRM cells compete, successfully, with innate or innate-like lymphocytes, which are already present at the original site of infection (39).
Intraepithelial Lymphocytes
A variety of innate or innate-like lymphoid cell types reside in tissues, including natural killer (NK) cells, innate lymphoid cells, and T cells expressing the γδ TCR chains (γδ T cells), homodimers of CD8α or a semi-invariant TCRαβ such as NKT cells, and mucosal associated invariant T cells. The top layer of the epithelia, in murine and human intestine as well as murine skin, contains large populations of such innate-like T cells within the IEL population.
Intestinal IELs express the prototypical tissue-resident integrin CD103 (integrin αE), with which they interact with IECs (10), as well as C-Type lectin and early activation marker CD69, and the NK cell inhibitory receptor 2B4 (CD244) (42). Antibody staining for CD8α, CD69, and CD103 in lymphocytes sourced from the intestinal intraepithelial fraction provides a homogenous cell population (42). However, IELs can be divided into subsets based on their activation mechanism and on the antigens, which they may recognize. Induced or adaptive IELs are derived from conventional CD8αβ T cells, which recognize non-self antigens in the context of MHCI. They home to the intestinal barrier upon encountering their cognate antigen in the intestine as TRM cells (9, 43). Induced IELs accumulate with age (44), replacing natural IELs (39).
Natural or innate-like IELs also originate in the thymus where they acquire homing factors and identity upon selection on self-antigens and seed the intestine as a precursor population (45–51). They express CD8αα homodimers, in contrast to conventional CD8αβ T cells (52) (Figure 1). They express either the conventional αβ TCR or the non-conventional γδ TCR. In the small intestine around 60% of all IELs express TCRγδ, in marked contrast to SLO in which γδ T cell represent less than 1% (53). In humans, natural IELs predominantly express TCRVδ1 with a contribution from TCRVδ3, the majority of which express CD8 (54, 55). In contrast to murine cells, human γδ T cells may process and present antigen (56).
Contrary to T cells found in SLO and in line with TRM cells, IELs do not circulate through blood and lymph and are tissue-resident (57). IELs seem to respond to a broad range of inflammatory cues, but the precise identity of these signals remains unknown. They modulate epithelial cell homeostasis and local immune responses by targeting other immune cells, viruses, and bacteria (9, 58, 59). The majority of IELs hold cytoplasmic granules containing large amounts of granzymes, cytokines, and chemotactic factors (42, 43, 60–62). At the first sight, the cytotoxic properties of IELs suggest, they can cause damage to the epithelial barrier by powerfully attacking infected cells, particularly IECs (63). However, IELs are well adapted to the intestinal environment in order to survive and perform their functions in protecting the delicate epithelial layer. In recent years, several studies of IELs have revealed distinct characteristics regarding their maintenance, activation, and contribution to the host immune response to preserve a healthy epithelial barrier.
Maintaining IELs
Intraepithelial lymphocytes develop pre-birth, occupy the epithelia before microbial colonization, and play an important role in immune protection during early life (64). It remained debatable for a considerable time if natural IELs take up residence at the intestinal epithelia as precursor naive-like cells or as antigen-experienced memory-like CD8+ T cells poised for activation or reactivation. The later would suggest that a priming step may be required, post-thymic development in the SLO, before seeding in IEL compartment. Transcriptional analysis, comparing IELs harvested under non-inflammatory conditions with memory CD8+ T cells, revealed paradoxical findings of their activation status (42, 43). IELs constitutively express transcripts of genes associated with activated cytotoxic T cells [granzyme A, granzyme B, serglycin, Fas ligand (FasL), and CCL5]. Yet, at the same time, IELs highly express transcripts of genes involved in immune regulation. These include cytotoxic T-lymphocyte associated protein 4, Ly49E-G, the NK cell inhibitory receptor Ig superfamily-related gp49B, and programmed cell death 1 (42). Factors involved in microbe-toxicity, such as regenerating islet-derived protein 3 gamma (Reg3γ), are readily detected in IELs under steady state conditions (65). In addition, several transcripts have been translated, and proteins are present and stored in secretory vesicles, e.g., granzymes (66). IELs home are retained in a poised activation state in mice lacking most secondary organs (67, 68), suggesting priming in secondary organs for natural IELs is not essential and the IEL activation status may be maintained by factors in the local epithelial environment.
Natural IELs are present in axenic mice. However, reduced numbers (of induced IELs) and decreased cytotoxicity of IELs from germ-free mice indicate that signals from the microbiota or other environmental stimuli are required to maintain intestinal CD8+ T cells and their function (51, 69, 70). The ligand activated transcription factor, arylhydrocarbon receptor is critical for IEL maintenance (59, 71). Of interest, the provision of ligands can be achieved via food intake, especially green vegetables, and may also be obtained from the microbiota (59, 72). Curiously, 30–50% of IELs from conventional standard pathogen-free mice express the marker Thy1 (CD90), but those found in axenic mice do not. Colonization of germ-free mice results in the generation of Thy1-expressing IELs (69), but as yet no functional differences have been attributed to the expression of Thy1.
Intraepithelial lymphocyte maintenance and activation also critically relies on interactions between IECs and microorganisms. Myeloid differentiation primary response gene 88 (MyD88), the adapter protein used by many toll-like receptors (TLRs), interleukin (IL)-1R, and IL-18R activate the transcription factor nuclear factor-κB, is required for IEL maintenance via the production of IL-15 (65, 73) (Figure 2). IL-15 production signals via the type 1 transcription factor, Tbox expressed in T cells (Tbet) to maintain IEL precursors (51). TLR2 may be at least one of the pattern recognition receptors involved in IEL maintenance, via IL-15 induction, its absence resulting in marked reduction of intestinal IELs (74, 75). Although IL-15 may be induced by microorganisms, they may not be essential for its production as axenic mice have reportedly higher levels of Il15 transcripts, and no differences in numbers of natural IELs were observed (51). Nucleotide-binding oligomerization domain-containing protein (NOD)2, an intracellular sensor for microbial products has also been shown to be important for IEL maintenance (76). IELs in NOD2-deficient mice show reduced proliferation and increased levels of apoptosis. Once again, NOD2 signaling, via recognition of gut microbiota, results in IL-15 production (Figure 2). Of interest, NOD2 is able to tune the signaling of TLR2 dose dependently (77). Although these results have been achieved using whole body knock outs for MyD88, TLR2, or NOD2, and therefore, the exact role of IECs remains to be determined, they indicate that microorganisms may play an important role in IEL maintenance.
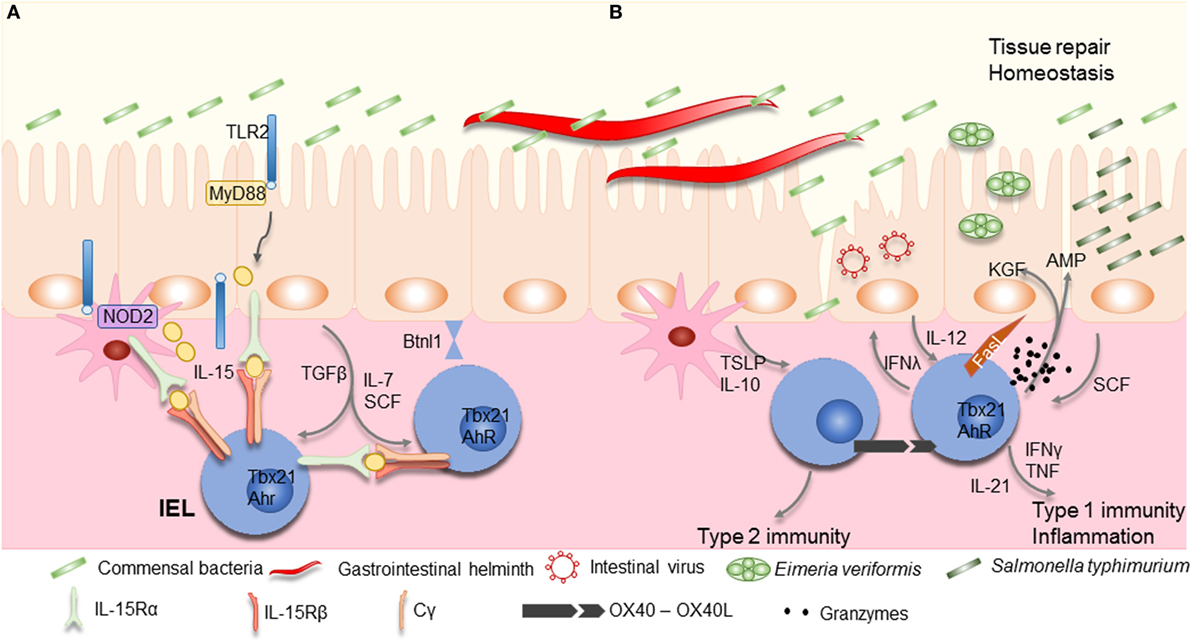
Figure 2. Maintenance and activation of intraepithelial lymphocytes (IELs). (A) Commensal bacteria can contribute to IEL maintenance. Signaling via TLR2 and myeloid differentiation primary response gene 88 (MyD88) increases interleukin (IL)-15 production, an important survival factor for IELs. Antigen presenting cells, such as dendritic cells (DCs) or macrophages, also produce IL-15 in a NOD2 dependent manner. IL-15 is bound to the IL-15Rα on the producing cells, and is presented in trans to the IEL, which carry the IL-15Rβ/Cγ chain receptor complex, and signals via the transcription factor Tbx21. IL-7 and stem cell factor (SCF) are additional examples for IEC derived cytokines important for IEL survival, while arylhydrocarbon receptor expression (AhR) and tissue-specific factors, such as butyrophilin-like 1 (Btnl1), play an additional role in maintaining IELs. (B) Infections cause disruption or damage to the epithelial barrier. Dependent on the type of insult, IEC and DCs produce cytokines like thymic stromal lymphopoietin (TSLP), IL-10, IL-12, or SCF, thereby directing the type of immune response. Additional stimulation may be derived from IEL–IEL cross-talk, such as via OX40–XO40L interactions. IELs produce pro-inflammatory cytokines such as interferons (IFNs) and tumor necrosis factor (TNF), and cytotoxic factors such as Fas ligand (FasL) and granzymes, as well as antimicrobial peptides (AMPs) to contain the infection and contribute to wound healing and restoration of homeostasis by secreting growth factors such as KGF. Aberrant IEL activation and potentiation by cytokines might be involved in the development of chronic inflammation and IBD.
IEL Activation Status
Due to the positioning of IELs just underneath the single epithelial layer and their potential involvement in modulating intestinal pathology, the activation status of IELs is intensively studied. Transcriptional data of IELs foretells puzzling semi-activation of IELs that could enable them to deal with a broad range of pathologies quickly, with reduced requirement for immediate energy absorption and new gene expression (78). Unlike conventional CD8+ T cells, IELs express high levels of Tnfsf6 transcripts during steady state (42, 43), but do not express the encoding FasL protein on their surface until additional activation takes place (61). Despite their poised state and effector-like or TEM cell characteristics, IELs do not contain transcripts for cytokines, which they secrete during conditions of inflammation (43). This suggests IELs require additional cues to initiate part of their effector function capacity.
Understanding the activation properties of IELs is essential to gain insight into mechanisms of local immunity and events associated with tolerance, chronic inflammation, and immunopathology. Intestinal mucosa resected from patients with IBD (79) or celiac disease (80) contains increased numbers of activated T cells, a hallmark of intestinal inflammatory disorders (81). Yet, in a chemically induced colitis model, dextran sulfate sodium, 2,4,6-trinitrobenzene sulfonic acid (TNBS) or T cell transfer colitis, and IELs were found to be protective (60, 76, 82–84). This raises questions regarding the role of IELs in the intestinal immune network, whether they can, at least in part, contribute to chronic inflammation and pathology, or if they have a more tolerogenic or regulatory role. Furthermore, although cytotoxicity and microbicidal activity are an important part of IEL activity, it is not clear if their potential to produce cytokines and chemokines can be tailored to the level or identity the microbial threat.
Transcriptional data also suggested that IELs are metabolically prepared for swift action. IELs, compared with memory CD8+ T cells, contain increased levels of mRNA for metabolic enzymes, especially those involved in the generation of fatty acids and cholesterol esters (42, 43). In line with the expression of CD69, IELs seem arrested in a semi-activated state. Yet, in stark contrast to effector cells, IELs survive for a considerable period of time. For example, murine skin IELs are generated only during embryogenesis, but can be found throughout adult life and into old age. The skin and intestinal epithelia are lipid-rich, but availability of other nutrients may be limited (85). This may explain why skin TRM cells appear to use mitochondrial β-oxidation of exogenous lipids, mediated by intracellular transport proteins, including fatty-acid-binding protein-4 and -5, supporting their longevity and protective function (78). Similarly, natural IELs highly express surface molecules involved in lipid uptake, such as apolipoprotein E and low-density lipoprotein receptor (42). The increased presence of receptors and enzymes involved in lipid metabolism in IELs compared with conventional T cells suggests that altered metabolic processes may be involved in maintaining their poised activation status. However, it remains to be determined if the increase in lipid metabolism sets IELs apart or if it reflects their semi-activated status, since recently activated conventional T cells utilize the same pathways (86). Lipids are also required for the differentiation of CD8+ memory T cells, the formation of which requires metabolic reprogramming characterized by enhanced mitochondrial fatty-acid oxidation (87). Although, cell-intrinsic lipolysis is implicated in memory cell formation, suggesting the acquisition of fatty acids from the external environment is not critical, these lipids may have been obtained during the initial priming stage. These data imply that the metabolism of IELs reflects, in part, that of recently activated T cells and that lipolysis may be inhibited in IELs to arrest them in a poised activation status, thereby preventing progression to a quiescent memory status.
Role for the TCR in IEL Activation
The understanding of the role of the TCR on IEL development, differentiation, homing, and activation has long been hampered by the absence of a known selective or activating ligand. Recent work has identified tissue-specific ligands expressed in the thymus, driving the development and homing of either murine skin IELs or intestinal TCRγδ IELs, not peptide-MHC or lipid-CD1 complexes, but the butyrophilin-like molecules skint-1 and butyrophilin-like 1 (Btnl1), respectively (88–90). The role of the TCRαβ expressed on natural CD8αα IELs remains unknown (91). The collective data from these studies strongly suggest that the TCRγδ is required for both thymic selection and imprinting of IEL identity as well as their maintenance in specific tissue niches. However, butyrophilins, part of the immunoglobulin superfamily [for detailed review see Rhodes et al. (92)], are, unlike MHC or CD1 molecules, not known to present ligands. Thus, it remains unclear if IELs are stimulated via their TCR or if they sense other cues, such as inflammatory, tissue damage or cell stress factors provided by IECs or accessory cells (93), or are under the influence of metabolic alterations as a result of cell damage or bacterial growth in the microenvironment which can enhance TH cell subset differentiation (94).
Agonist-driven positive selection of IELs in the thymus suggests that mature IELs at the epithelial barriers could subsequently be activated by specific TCR ligands. IEL TCR activation may be achieved by cell surface receptors, such as non-classical MHC molecules (95, 96). The parameters required for an agonist to activate IELs upon conditions of inflammation or tissue damage exclude constitutively expressed surface molecules, such as skint-1 and Btnl1, unless a gradient reaching a critical activation threshold can be achieved. If an agonist able to activate IELs exists, it does not preclude direct IEL activation by microbial products such as observed for γδ T cells in both man and mouse (97–99). Such direct activation is commonly observed in conventional CD8+ T cells that have been pre-selected in the thymus and successfully primed and expanded in the periphery. Reactivation of memory CD8+ T cells is readily achieved by cytokines and TLR ligands, resulting in secretion of IFNγ from polyclonal T cells that bridge innate and adaptive immunity (100). Similarly, peripheral γδ T cells can be directly activated by TLR ligands and combinations of cytokines (99, 101).
Administration of anti-CD3ε antibodies, which directly stimulate the TCR signaling complex thereby bypassing TCR-specific ligation, has often been used as a proxy to stimulate IELs in mice. However, its systemic activity, due to indiscriminate total T cell activation in all tissues, results in “cytokine release syndrome,” increasing serum levels of IL-2, TNF, and IFNγ, and leading to intestinal phenotypes, such as diarrhea (102, 103). The small intestines from mice treated with anti-CD3 show increased epithelial ion transport, altered spontaneous muscle activity, and reduced IEC viability (104). The effect of anti-CD3 is rapid, with DNA fragmentation observed after 30 min in the areas most enriched with IELs, followed within hours by IEC shedding into the lumen (105). Similar effects on IEC viability were observed upon administration of anti-TCRγδ antibodies, but not with those stimulating TCRαβ (106). The effect on IEC shedding, however, was fully dependent on TNF receptor signaling and may not necessarily depend on IEL activation since conventional T cells can also secrete large amounts of TNF.
Following anti-CD3 stimulation, poised IELs acquire aspects of fully activated effector T cells with higher expression of CD44, Ly-6C, OX40, FasL, and CD25 and reduced expression of CD45RB protein, accompanied by expression of cytotoxic mediators as well as cytokine transcripts (61, 107). Effects of anti-CD3 on IEC viability appear to correlate well with the cytotoxic capacity of IELs, especially since release of granzyme B is observed upon anti-CD3 stimulation (62). However, DNA fragmentation is independent of the pore forming protein perforin (62). This suggests that IECs are non-specifically targeted by their proximity to activated T cells or by their susceptibility to soluble mediators. The accumulative data postulate that in vivo activation of IELs can at least in part be achieved via TCR ligation. IEL activity can have a major impact on intestinal physiology, altering electrolyte balance and IEC viability. However, their potential to damage IECs markedly contrast with the requirement to maintain an intact single cell intestinal barrier to efficiently protect the host and questions if TCR stimulation accurately recapitulates the physiological role of IELs. IEC–IEL bidirectional interactions are instrumental to maintain IELs, but it remains unknown if IECs directly contribute to IEL activation and, if they do, what the identities of the activating cues are?
IEL Activation by Microbes
Commensal bacteria can invade tissues when opportunity arises. Such opportunities occur upon initial microbial invasion of new-borns before species-specific adaptive immunity has fully developed or when the host is immune compromised (108). Since, activating IELs may not require antigen processing or rely on presentation by MHC-like molecules, it remains possible that IELs recognize molecular patterns generated by bacterial non-peptide antigens or conserved unprocessed protein antigens produced by bacteria or released by epithelial cells upon damage or cell stress (109).
Invasion of pathogens or tissue damage could create the conditions for commensal microorganisms to invade the intestinal tissues. Innate immunity relies on the detection of highly conserved pathogen-associated molecular patterns (110). Receptors involved in the detection of invasion will respond to the microbial components present in both pathogen and commensal microorganisms. But it has become clear that not all microorganisms evoke a similar response. Indicators of viability, such as the presence of prokaryotic mRNA invoke a much stronger immune response (111). The balance of immunity and tolerance at the epithelial interphase is also illustrated by the production of IgA, the predominant antibody isotype critical at mucosal sites (112). IgA mainly coats pathogenic bacteria, which can confer colitis in axenic mice (113). IgA is a poor activator of the immune system; in line with the idea that strong immunity at mucosal sites is best avoided. IELs have been implicated in coordinating IgA response. TCRδ-deficient mice, harboring reduced IEL numbers, show reduced IgA levels in serum, saliva, and fecal samples. TCRδ-deficient mice also produce much lower levels of IgA antibodies upon oral immunization (114).
Role for IECs in IEL Activation; Co-stimulation
A requirement for co-stimuli or TH cell help is linked with the need for clonal expansion and differentiation, creating a significant delay in adaptive immunity. Immune surveillance by tissue-resident lymphocytes requires a swift response without prior cell expansion or differentiation, observed for γδ T cells and memory T cells, such as TRM cells (27, 115). This is in line with the hypothesis that the IEL response primarily serves to contain a potential threat, not necessarily resulting in microbe eradication or the establishment of immunological memory, thereby limiting microbial or toxin dissemination and keeping the single cell barrier intact by avoiding intestinal pathology.
Homing of IELs to the small intestine seems consistent with oligoclonal activation by commonly encountered antigens. The poised activation status of IELs could ensure their rapid activation without the need for an array of instructive signals. IELs seem not to depend on signal 2, required for conventional T cell activation and protective immunity (116). CD28 as well as additional co-receptors, such as CD2 and CD5, appear reduced or absent from IELs (43, 117–119). Furthermore, expression of MHC molecules or the costimulatory B7 proteins on at least keratinocytes is not required for activation of skin IELs (97). However, since the triggering of IELs could contribute to immunopathology, their activation is likely controlled on several levels, such as by signals derived from inflammation or tissue damage. The absence of a requirement for classic costimulatory signals for IEL activation suggests that close interactions with IECs play a prominent role.
OX40 (CD134, TNFRSF4) is expressed by activated T cells controlling cell expansion (120), including IELs (121). Its expression correlates well with T cell activity observed in patients with IBD, active celiac disease, Crohn’s disease (CD), and ulcerative colitis (UC) (122, 123). In vitro activation of IELs with anti-CD3ε antibodies results in the expression of both OX40 and its ligand (OX40L) (121). Of note, OX40L is not expressed upon activation of conventional T cells. Additional ligation of OX40 seems to boost IEL activity and reduce the secretion of IL-10. This suggests that accumulation of IELs at sites of inflammation may alter their potential and that such co-stimulation may not necessarily depend on OX40L expression by IECs or myeloid cells (Figure 2).
Skin and intestinal IELs express the junctional adhesion-like molecule-1 (JAML-1), which provides co-stimulation upon ligation with the coxsackie-adenovirus receptor (CAR) (124, 125). JAML signaling results in cytokine production from skin IELs and may provide additional context for full IEL activation, presumably as response to infection or tissue damage. However, the latter requires its expression to be regulated upon insult or microbial invasion, which remains to be determined. Furthermore, its ligation by neutrophil-derived soluble JAML compromises intestinal barrier integrity and reduces wound repair through decreased IEC proliferation (126). Thus, the role of JAML—CAR in barrier defense remains to be clarified.
Role for IECs in IEL Activation; Cytokines
Intestinal IELs can express receptors for TNF, leukemia inhibitory factor, thymic stromal lymphopoietin (TSLP), stem cell factor (SCF; c-Kit ligand), transforming growth factor (TGF)β, IL-12, IL-15, and IL-21 (43, 127). TGFβ, most likely derived from IECs upon microbial stimulation, is required to maintain natural CD8αα IELs and to induce CD103 expression. The absence of TGFβ or its receptor results in markedly reduced numbers of IELs, while over expression increased the IEL population (50). How TGFβ influences IEL activity remains unknown.
Interleukin-15 plays a central role in maintenance of natural IELs and emphasizes the close interactions between IECs and IELs (128–131). IL-15 is presented in trans to IELs by epithelial cells, in the thymus, skin, and the intestine, which express both the IL-15Rα and IL-15 (132). IL-15R signaling induces the expression of anti-apoptotic molecules, Bcl-2 and Bcl-xL by IELs (133). The production of IL-15 is regulated, at least in part, by contact with microbial components. MyD88- and TLR2-derived signals are required for IEL maintenance via the induction of IL-15 production (65, 73, 75). TRM cells are similarly dependent on IL-15-mediated signals (134), whereby high levels of IL-15 can TCR-independently trigger CD8+ T cells to become cytotoxic (135, 136). Upon IEC damage, IL-15 production increases (65), as observed during celiac disease, and correlates strongly with IEL activity (133, 137). IL-15 stimulation of IELs results in increased IFNγ and TNF production, granzyme-dependent cytotoxicity, NK receptor expression, and increased survival (138). Of note, the increase in IL-15 production in conjunction with additional cues, such as retinoic acid can stimulate DCs, thereby inducing the secretion of pro-inflammatory factors and indirectly activate IELs. IL-15 induces the secretion of IL-21 by IELs, observed in celiac disease, which may be part of a self-sustaining feed-forward loop as observed in Th17 cells, enhancing IEL activation and cytotoxicity (139, 140).
Another important cytokine involved in T cell homeostasis is IL-7 (141). It is secreted by non-hematopoietic cells, especially thymic and IECs, with enhanced expression observed upon tissue damage (142–144). IEL development requires IL-7R signaling in the thymus, but local IL-7 expression by IECs can restore the γδIEL subset, not other γδ T cell subsets, suggesting extrathymic development or maturation of γδIELs may take place in the intestinal compartment (51, 142, 145). The use of acute IL-7 reporter mice indicates that production of IFNγ by T cells, such as IELs, can modulate the level of IL-7 and IL-15 produced by IECs, thereby regulating IEC homeostasis, absorptive function as well as the composition of the microbiota (144, 146). Vice versa, IEC derived IL-7 can regulate IEL survival and proliferation, particularly induced CD8αβ IELs (147). Overexpression of IL-7 results in lymphoid expansion and colitis (148).
Intestinal epithelial cells show a basal production of TSLP that is important for host protection during helminth infections (149). Similar to IL-15, TSLP receptor stimulation of CD8+ T cells enhances expression of Bcl-2 (150), and may play a role in IEL survival. TSLP seems to enhance type 2-mediated immunity (Figure 2). Upon TSLP encounter, IECs and DCs produce IL-10 and reduce IL-12 production, thereby reducing type 1 immunity (151). IELs are known to be able to secrete IFNγ and loss of intestinal integrity results in IEC-produced IL-12 (152), the prototypical driver of type 1 immunity. In the absence of TSLP, mice are more susceptible to colitis and have increased levels of IFNγ producing cells. Salmonella typhimurium infection increases the expression of SCF produced by IECs (153). Its receptor, c-Kit, is expressed by IELs (127). The absence of SCF results in marked reduction of IEL numbers in mice, while its presence seems to play a role in IEL activation (154). Whether SCF and TLSP act as instructive cues initiating divergent IEL activation profiles remains to be investigated.
Containing Invasive Microbes
An ascending bacterial load exists from duodenum to jejunum and ileum, accumulating in very high numbers in the cecum and colon. Of note, IEL numbers are descending from duodenum to ileum, with few found in the colon (4). The causality of this striking inverted relationship remains unknown. IELs can produce antimicrobial factors and tissue repair factors in response to bacteria that penetrate the intestinal epithelium (60). IELs play an important role in the regulation and differentiation of epithelial cells at the base of the crypts (58, 155). IELs thereby help to preserve the integrity of damaged epithelial surface by providing the localized delivery of an epithelial cell growth factor (60). The mucosal protection afforded by IELs is of critical importance particularly during the first hours after bacterial exposure (156), in line with the hypothesis that IELs function to contain microbes upon invasion and initiate barrier repair thereby reducing immunopathology. With respect to pathogenic infections, in the majority studied, IELs offer protection against a wide variety of intestinal species, including Eimeria vermiformis (64, 157, 158), Toxoplasma (159, 160), Encephalitozoon cuniculi (161), Norovirus (107), and Salmonella (159, 160). Interestingly, infections with at least the pathogens Salmonella and Toxoplasma have indicated that IELs migrate to the site of infection and into the lateral intra-intestinal space (160), possibly initiating IEL–IEL co-stimulation (121). Collective release of preformed antimicrobial peptides (65, 156, 162) could directly contribute to microorganism containment and clearance (163).
Upon intestinal infectious challenges, protection and pathology are a result of the interplay between the microbes, the IELs, conventional T cells, and other immune cells. During Eimeria infection, IELs’ production of IFNγ and TNF is instrumental in protective immunity, and expression of junctional molecules to preserve epithelial barrier integrity (158). However, elevated IFNγ and TNF levels in the intestinal mucosa also contribute to the pro-inflammatory cascade involved in barrier disruption and pathology (164). γδIELs are able to reduce pathology and their absence exaggerates mucosal injury upon Eimeria vermiformis infection (157). The absence of αβ T cells results in reduced capacity to clear the parasite, in part compensated by the adoptive transfer of CD4+ T cells. In the absence of γδIELs, Salmonella or Toxoplasma infection result in increased microbe transmigration due to reduced epithelial barrier integrity. This increased transmigration leads to increased immunity mediated by conventional T cells (159). This indicates that IELs are not ultimately responsible for microbial clearance, but can modulate the initial response and recruitment of immune cells in order to moderate the risk of immunopathology.
Intraepithelial lymphocytes can contribute to viral immunity. Viral control depends largely on conventional T cells but, at least, αβIELs take part in viral clearance in the mucosa (165–167). Intestinal viral challenge, such as the non-enveloped RNA virus norovirus (MNV) present in many laboratory animal facilities, results in infection of IEC and myeloid cells (168). The infection can be controlled by IFNs, particularly IFNλ (169). Of note, the IFNλ (IFN type III) response seems to be operating particularly at epithelia barriers. This indicates that barrier immunity is kept local, to avoid systemic responses in which type I IFNs play a dominant role (170, 171). IELs, upon stimulation with plate-bound anti-CD3, can transcribe IFN genes, type I, II, and III, and the supernatant of in vitro activated IELs reduces viral infection (107, 172). In vivo anti-CD3 administration as well as culture supernatant from activated IELs results in IFN type I/III receptor-dependent expression of IFN responsive genes in intestinal IECs. Administration of anti-CD3 antibodies before intestinal viral challenge with murine norovirus can reduce viral load (107). However, due to the polyclonal stimulation of all T-lymphocytes, it remains unclear what contribution IELs provide and which properties may be uniquely attributed to them. It remains unknown if IELs are stimulated upon intestinal viral invasion and if so, how such invasion would enable the activation of IELs.
Besides microbicidal and cytotoxic activity, IELs produce cytokines and chemokines. Some chemokine transcripts are already present in IELs under steady state conditions, such as CCL5 and XCL1, but not those encoding for cytokines, such as IFNγ and TNF (43). This suggests that the recruitment of additional immune cells and release of these powerful cytokines and other chemoattractants such as CXCL1, CXCL2, and CXCL9 (65), might be delayed compared with cytotoxicity. The clearance of pathogens and the instigation of immunological memory involve careful orchestration of various cellular components. At epithelial sites, pathogen containment by innate-like T-lymphocytes may precede recruitment of myeloid cells and subsequent involvement of the adoptive arm of the immune response.
IELs in IBDs
The initiation of an immune response is not taken lightly, especially at the intestinal barrier. When immune activation does take place, pathogen clearance, pathology, and the need to maintain tissue integrity and its repair are offset. The consequences of microbial invasion or aberrant immunity can be severe (81). IBD can affect any part of the intestine and present with extra-intestinal manifestations. Despite advances in IBD understanding, the cause(s) and mechanism(s) remain unknown and disease incidence is increasing with changes in the environment and life style likely making a contribution (173). Disease occurrence and severity are further compounded by diet, dehydration, and antibiotic use (174) as well as age (175).
There are indications that IBD results from alterations in innate immunity resulting in excessive adaptive immune activation (176, 177). IBD seems to result from immune-genetic predispositions and environmental factors, especially a dysregulated response to microorganisms (178). The presence of micro-infections or patches of affected intestinal tissue, associated with bacterial presence, next to seemingly unaffected tissue suggest localized immune activation or inefficient immune resolution (179). Several immunologic and histopathologic features of IBD, such as the presence of activated T cells secreting IFNγ and IL-17 and immunopathology (180), can be explained as a defect in mucosal immune regulation and as a consequence of persistent mucosal T cell activation (181). Several studies have shown that IL-12 production is increased in inflammatory lesions of patients with CD (182). In line with activated T cells, UC patients have an increased level of IL-7 and IL- 15, which may perpetrate additional T cell activation (183, 184).
There is limited data for a role of IELs in IBD, either disease enhancing or reducing. Accumulation of γδIELs in inflamed areas of IBD patients have been reported, and associated with increased levels of IL-15 and TSLP (133, 151). Although identifying lamina propria migrated γδ T cells and γδIELs is difficult, these cells constitutively produce IFNγ in patients suffering from either CD or UC (185). It remains to be determined if reduced numbers of IELs contribute to increased susceptibility to inflammation or are a consequence of ongoing inflammation, such as due to an increase in αβ effector T cells or activation-related cell death. Murine studies have demonstrated potential protective roles for γδIELs in intestinal inflammation as well as IBD models (59, 82, 186), but others have suggested that IEL expansion and activation can exacerbate the progression of colitis (187, 188). Lesions in IBD are mainly found in areas of reduced IEL density and highest load of bacteria, the colon, and ileum (178). The numbers of IELs correlate inversely with disease severity, and IEL numbers are restored to levels observed in healthy controls upon treatment with anti-TNF (189). This indicates vulnerability at those sites with altered immune surveillance and intense bacteria–IEC interactions, in line with the presence of adherent-invasive Escherichia coli at IBD lesions (179).
Loss-of-function mutations of NOD2 are strongly associated with CD (190–193). This correlates with the loss of IELs seen in mice deficient for NOD2 (76), but detailed insights are lacking and NOD2 plays an important role in other cell populations such as DCs, able to influence type 1 and type 3 immunity (194). Reduced proportions of γδIELs at the intestinal mucosa of CD patients suggested a protective role for these cells (189, 195, 196). IELs show increased activity at inflammatory sites in IBD patients, secreting IFNγ and TNF (189). Furthermore, IELs may enhance the production of IFNγ in the human colon (197). Nevertheless, murine IELs have been shown to be able to reduce the production of IFNγ production by conventional CD4 T cells, indicating their capacity to reduce type 1 immunity (198). In line with IELs forming an important part of the first line of defense is the increased susceptibility to infection of CD patients, which have reduced IEL numbers, with the intracellular parasite microsporidia (199). Unfortunately, the data on a role for IELs in preventing or reducing susceptibility to IBD remain inconclusive. The majority of IELs are found in the small intestine, a site not easily accessible, and IELs as a population of human T-lymphocytes are not well defined with respect to their identity and location. Often γδ T cells, such as those found in the circulation, are used as a proxy for IELs. However, murine studies and data on human TCRδ-chain usage have shown IELs to be very different from γδ T cells found in other tissues [(55, 59) #1587].
Conclusion
Intraepithelial lymphocytes are an integral part of the epithelial barrier. They do not exist as isolated cells monitoring the front line, but have close bidirectional interactions with IECs and possibly other immune cells. This close interaction enables the reception and provision of signals at very close range to maintain epithelial integrity. This interaction may be crucial in initiating local repair and containing low level of microbial invasion. Localized cues of potentially low dose would enable the activation of poised IELs without necessarily alerting the adaptive immune system. The single cell epithelial barrier is under constant threat of assault. Containing such threats locally with minimal immune activation is of great benefit to limit immunopathology and maintain optimal nutrient uptake.
How IELs, displaying many characteristics of effector T cells, are maintained in a poised state remains poorly understood. Differences identified in metabolic pathways may reflect their partial activation status or indicate differential metabolic wiring of IELs. The cues that enable full IEL activation remain ill-defined and the activity of IELs, the existence of different modes of action, are unknown. With technological advantages, such as multicolor flow cytometry and microscopy new players at the mucosal sites have been identified. This may now help us to unravel the complex multiplayer immune surveillance network of the mucosal immune response with real potential to discover novel targets for therapies to alleviate or even cure the different forms of IBD and celiac disease.
Author Contributions
All authors wrote the review and contributed to the figures.
Conflict of Interest Statement
The authors declare that the research was conducted in the absence of any commercial or financial relationships that could be construed as a potential conflict of interest.
Acknowledgments
Members of the Veldhoen laboratory are supported by European Union H2020 ERA project (N°667824—EXCELLtoINNOV), publication costs were provided by LISBOA-01-0145-FEDER-007391, projeto cofinanciado pelo FEDER através POR Lisboa 2020—Programa Operacional Regional de Lisboa, do PORTUGAL 2020, e pela Fundação para a Ciência e a Tecnologia.
References
1. Cieza RJ, Cao AT, Cong Y, Torres AG. Immunomodulation for gastrointestinal infections. Expert Rev Anti Infect Ther (2012) 10(3):391–400. doi:10.1586/eri.11.176
2. Medzhitov R, Janeway C Jr. Innate immunity. N Engl J Med (2000) 343(5):338–44. doi:10.1056/NEJM200008033430506
3. Guilliams M, Ginhoux F, Jakubzick C, Naik SH, Onai N, Schraml BU, et al. Dendritic cells, monocytes and macrophages: a unified nomenclature based on ontogeny. Nat Rev Immunol (2014) 14(8):571–8. doi:10.1038/nri3712
4. Mowat AM, Agace WW. Regional specialization within the intestinal immune system. Nat Rev Immunol (2014) 14(10):667–85. doi:10.1038/nri3738
5. Veldhoen M. The role of T helper subsets in autoimmunity and allergy. Curr Opin Immunol (2009) 21(6):606–11. doi:10.1016/j.coi.2009.07.009
6. Brucklacher-Waldert V, Carr EJ, Linterman MA, Veldhoen M. Cellular plasticity of CD4+ T cells in the intestine. Front Immunol (2014) 5:488. doi:10.3389/fimmu.2014.00488
7. Ohnmacht C, Park JH, Cording S, Wing JB, Atarashi K, Obata Y, et al. Mucosal immunology. The microbiota regulates type 2 immunity through RORgammat(+) T cells. Science (2015) 349(6251):989–93. doi:10.1126/science.aac4263
8. Laidlaw BJ, Craft JE, Kaech SM. The multifaceted role of CD4(+) T cells in CD8(+) T cell memory. Nat Rev Immunol (2016) 16(2):102–11. doi:10.1038/nri.2015.10
9. Cheroutre H, Lambolez F, Mucida D. The light and dark sides of intestinal intraepithelial lymphocytes. Nat Rev Immunol (2011) 11(7):445–56. doi:10.1038/nri3007
10. Cepek KL, Shaw SK, Parker CM, Russell GJ, Morrow JS, Rimm DL, et al. Adhesion between epithelial cells and T lymphocytes mediated by E-cadherin and the alpha E beta 7 integrin. Nature (1994) 372(6502):190–3. doi:10.1038/372190a0
11. Beagley KW, Fujihashi K, Lagoo AS, Lagoo-Deenadaylan S, Black CA, Murray AM, et al. Differences in intraepithelial lymphocyte T cell subsets isolated from murine small versus large intestine. J Immunol (1995) 154(11):5611–9.
12. Mysorekar IU, Lorenz RG, Gordon JI. A gnotobiotic transgenic mouse model for studying interactions between small intestinal enterocytes and intraepithelial lymphocytes. J Biol Chem (2002) 277(40):37811–9. doi:10.1074/jbc.M205300200
13. Qi H, Kastenmuller W, Germain RN. Spatiotemporal basis of innate and adaptive immunity in secondary lymphoid tissue. Annu Rev Cell Dev Biol (2014) 30:141–67. doi:10.1146/annurev-cellbio-100913-013254
14. Jung S, Unutmaz D, Wong P, Sano G, De los Santos K, Sparwasser T, et al. In vivo depletion of CD11c(+) dendritic cells abrogates priming of CD8(+) T cells by exogenous cell-associated antigens. Immunity (2002) 17(2):211–20. doi:10.1016/S1074-7613(02)00365-5
15. Mescher MF, Curtsinger JM, Agarwal P, Casey KA, Gerner M, Hammerbeck CD, et al. Signals required for programming effector and memory development by CD8+ T cells. Immunol Rev (2006) 211:81–92. doi:10.1111/j.0105-2896.2006.00382.x
16. Chen L, Flies DB. Molecular mechanisms of T cell co-stimulation and co-inhibition. Nat Rev Immunol (2013) 13(4):227–42. doi:10.1038/nri3405
17. Knickelbein JE, Khanna KM, Yee MB, Baty CJ, Kinchington PR, Hendricks RL. Noncytotoxic lytic granule-mediated CD8+ T cell inhibition of HSV-1 reactivation from neuronal latency. Science (2008) 322(5899):268–71. doi:10.1126/science.1164164
18. Masopust D, Vezys V, Marzo AL, Lefrancois L. Preferential localization of effector memory cells in nonlymphoid tissue. Science (2001) 291(5512):2413–7. doi:10.1126/science.1058867
19. Hildeman DA, Zhu Y, Mitchell TC, Bouillet P, Strasser A, Kappler J, et al. Activated T cell death in vivo mediated by proapoptotic bcl-2 family member bim. Immunity (2002) 16(6):759–67. doi:10.1016/S1074-7613(02)00322-9
20. Tanchot C, Guillaume S, Delon J, Bourgeois C, Franzke A, Sarukhan A, et al. Modifications of CD8+ T cell function during in vivo memory or tolerance induction. Immunity (1998) 8(5):581–90. doi:10.1016/S1074-7613(00)80563-4
21. Veiga-Fernandes H, Walter U, Bourgeois C, McLean A, Rocha B. Response of naive and memory CD8+ T cells to antigen stimulation in vivo. Nat Immunol (2000) 1(1):47–53. doi:10.1038/76907
22. Reinhardt RL, Khoruts A, Merica R, Zell T, Jenkins MK. Visualizing the generation of memory CD4 T cells in the whole body. Nature (2001) 410(6824):101–5. doi:10.1038/35065111
23. Whitmire JK, Eam B, Whitton JL. Tentative T cells: memory cells are quick to respond, but slow to divide. PLoS Pathog (2008) 4(4):e1000041. doi:10.1371/journal.ppat.1000041
24. Hamann D, Baars PA, Rep MH, Hooibrink B, Kerkhof-Garde SR, Klein MR, et al. Phenotypic and functional separation of memory and effector human CD8+ T cells. J Exp Med (1997) 186(9):1407–18. doi:10.1084/jem.186.9.1407
25. Sallusto F, Lenig D, Forster R, Lipp M, Lanzavecchia A. Two subsets of memory T lymphocytes with distinct homing potentials and effector functions. Nature (1999) 401(6754):708–12. doi:10.1038/44385
26. Schon MP, Arya A, Murphy EA, Adams CM, Strauch UG, Agace WW, et al. Mucosal T lymphocyte numbers are selectively reduced in integrin alpha E (CD103)-deficient mice. J Immunol (1999) 162(11):6641–9.
27. Gebhardt T, Wakim LM, Eidsmo L, Reading PC, Heath WR, Carbone FR. Memory T cells in nonlymphoid tissue that provide enhanced local immunity during infection with herpes simplex virus. Nat Immunol (2009) 10(5):524–30. doi:10.1038/ni.1718
28. Casey KA, Fraser KA, Schenkel JM, Moran A, Abt MC, Beura LK, et al. Antigen-independent differentiation and maintenance of effector-like resident memory T cells in tissues. J Immunol (2012) 188(10):4866–75. doi:10.4049/jimmunol.1200402
29. Mackay LK, Rahimpour A, Ma JZ, Collins N, Stock AT, Hafon ML, et al. The developmental pathway for CD103(+)CD8+ tissue-resident memory T cells of skin. Nat Immunol (2013) 14(12):1294–301. doi:10.1038/ni.2744
30. Stary G, Olive A, Radovic-Moreno AF, Gondek D, Alvarez D, Basto PA, et al. VACCINES. A mucosal vaccine against Chlamydia trachomatis generates two waves of protective memory T cells. Science (2015) 348(6241):aaa8205. doi:10.1126/science.aaa8205
31. Springer TA. Traffic signals for lymphocyte recirculation and leukocyte emigration: the multistep paradigm. Cell (1994) 76(2):301–14. doi:10.1016/0092-8674(94)90337-9
32. Forster R, Schubel A, Breitfeld D, Kremmer E, Renner-Muller I, Wolf E, et al. CCR7 coordinates the primary immune response by establishing functional microenvironments in secondary lymphoid organs. Cell (1999) 99(1):23–33. doi:10.1016/S0092-8674(00)80059-8
33. Bromley SK, Thomas SY, Luster AD. Chemokine receptor CCR7 guides T cell exit from peripheral tissues and entry into afferent lymphatics. Nat Immunol (2005) 6(9):895–901. doi:10.1038/ni1240
34. Debes GF, Arnold CN, Young AJ, Krautwald S, Lipp M, Hay JB, et al. Chemokine receptor CCR7 required for T lymphocyte exit from peripheral tissues. Nat Immunol (2005) 6(9):889–94. doi:10.1038/ni1238
35. Masopust D, Choo D, Vezys V, Wherry EJ, Duraiswamy J, Akondy R, et al. Dynamic T cell migration program provides resident memory within intestinal epithelium. J Exp Med (2010) 207(3):553–64. doi:10.1084/jem.20090858
36. Jiang X, Clark RA, Liu L, Wagers AJ, Fuhlbrigge RC, Kupper TS. Skin infection generates non-migratory memory CD8+ T(RM) cells providing global skin immunity. Nature (2012) 483(7388):227–31. doi:10.1038/nature10851
37. Schenkel JM, Fraser KA, Vezys V, Masopust D. Sensing and alarm function of resident memory CD8(+) T cells. Nat Immunol (2013) 14(5):509–13. doi:10.1038/ni.2568
38. Wakim LM, Waithman J, van Rooijen N, Heath WR, Carbone FR. Dendritic cell-induced memory T cell activation in nonlymphoid tissues. Science (2008) 319(5860):198–202. doi:10.1126/science.1151869
39. Zaid A, Mackay LK, Rahimpour A, Braun A, Veldhoen M, Carbone FR, et al. Persistence of skin-resident memory T cells within an epidermal niche. Proc Natl Acad Sci U S A (2014) 111(14):5307–12. doi:10.1073/pnas.1322292111
40. Masopust D, Schenkel JM. The integration of T cell migration, differentiation and function. Nat Rev Immunol (2013) 13(5):309–20. doi:10.1038/nri3442
41. Steinert EM, Schenkel JM, Fraser KA, Beura LK, Manlove LS, Igyarto BZ, et al. Quantifying memory CD8 T cells reveals regionalization of immunosurveillance. Cell (2015) 161(4):737–49. doi:10.1016/j.cell.2015.03.031
42. Fahrer AM, Konigshofer Y, Kerr EM, Ghandour G, Mack DH, Davis MM, et al. Attributes of gammadelta intraepithelial lymphocytes as suggested by their transcriptional profile. Proc Natl Acad Sci U S A (2001) 98(18):10261–6. doi:10.1073/pnas.171320798
43. Shires J, Theodoridis E, Hayday AC. Biological insights into TCRgammadelta+ and TCRalphabeta+ intraepithelial lymphocytes provided by serial analysis of gene expression (SAGE). Immunity (2001) 15(3):419–34. doi:10.1016/S1074-7613(01)00192-3
44. Suzuki H, Jeong K II, Doi K. Age-related changes in the regional variations in the number and subsets of intraepithelial lymphocytes in mouse small intestine. Dev Comp Immunol (2002) 26(6):589–95. doi:10.1016/S0145-305X(02)00004-6
45. Rocha B, Vassalli P, Guy-Grand D. Thymic and extrathymic origins of gut intraepithelial lymphocyte populations in mice. J Exp Med (1994) 180(2):681–6. doi:10.1084/jem.180.2.681
46. Leishman AJ, Gapin L, Capone M, Palmer E, MacDonald HR, Kronenberg M, et al. Precursors of functional MHC class I- or class II-restricted CD8alphaalpha(+) T cells are positively selected in the thymus by agonist self-peptides. Immunity (2002) 16(3):355–64. doi:10.1016/S1074-7613(02)00284-4
47. Eberl G, Littman DR. Thymic origin of intestinal alphabeta T cells revealed by fate mapping of RORgammat+ cells. Science (2004) 305(5681):248–51. doi:10.1126/science.1096472
48. Yamagata T, Mathis D, Benoist C. Self-reactivity in thymic double-positive cells commits cells to a CD8 alpha alpha lineage with characteristics of innate immune cells. Nat Immunol (2004) 5(6):597–605. doi:10.1038/ni1070
49. Gangadharan D, Lambolez F, Attinger A, Wang-Zhu Y, Sullivan BA, Cheroutre H. Identification of pre- and postselection TCRalphabeta+ intraepithelial lymphocyte precursors in the thymus. Immunity (2006) 25(4):631–41. doi:10.1016/j.immuni.2006.08.018
50. Konkel JE, Maruyama T, Carpenter AC, Xiong Y, Zamarron BF, Hall BE, et al. Control of the development of CD8alphaalpha+ intestinal intraepithelial lymphocytes by TGF-beta. Nat Immunol (2011) 12(4):312–9. doi:10.1038/ni.1997
51. Klose CS, Blatz K, d’Hargues Y, Hernandez PP, Kofoed-Nielsen M, Ripka JF, et al. The transcription factor T-bet is induced by IL-15 and thymic agonist selection and controls CD8alphaalpha(+) intraepithelial lymphocyte development. Immunity (2014) 41(2):230–43. doi:10.1016/j.immuni.2014.06.018
52. Leishman AJ, Naidenko OV, Attinger A, Koning F, Lena CJ, Xiong Y, et al. T cell responses modulated through interaction between CD8alphaalpha and the nonclassical MHC class I molecule, TL. Science (2001) 294(5548):1936–9. doi:10.1126/science.1063564
53. Goodman T, Lefrancois L. Expression of the gamma-delta T-cell receptor on intestinal CD8+ intraepithelial lymphocytes. Nature (1988) 333(6176):855–8. doi:10.1038/333855a0
54. Deusch K, Luling F, Reich K, Classen M, Wagner H, Pfeffer K. A major fraction of human intraepithelial lymphocytes simultaneously expresses the gamma/delta T cell receptor, the CD8 accessory molecule and preferentially uses the V delta 1 gene segment. Eur J Immunol (1991) 21(4):1053–9. doi:10.1002/eji.1830210429
55. Dunne MR, Elliott L, Hussey S, Mahmud N, Kelly J, Doherty DG, et al. Persistent changes in circulating and intestinal gammadelta T cell subsets, invariant natural killer T cells and mucosal-associated invariant T cells in children and adults with coeliac disease. PLoS One (2013) 8(10):e76008. doi:10.1371/journal.pone.0076008
56. Brandes M, Willimann K, Bioley G, Levy N, Eberl M, Luo M, et al. Cross-presenting human gammadelta T cells induce robust CD8+ alphabeta T cell responses. Proc Natl Acad Sci U S A (2009) 106(7):2307–12. doi:10.1073/pnas.0810059106
57. Chennupati V, Worbs T, Liu X, Malinarich FH, Schmitz S, Haas JD, et al. Intra- and intercompartmental movement of gammadelta T cells: intestinal intraepithelial and peripheral gammadelta T cells represent exclusive nonoverlapping populations with distinct migration characteristics. J Immunol (2010) 185(9):5160–8. doi:10.4049/jimmunol.1001652
58. Komano H, Fujiura Y, Kawaguchi M, Matsumoto S, Hashimoto Y, Obana S, et al. Homeostatic regulation of intestinal epithelia by intraepithelial gamma delta T cells. Proc Natl Acad Sci U S A (1995) 92(13):6147–51. doi:10.1073/pnas.92.13.6147
59. Li Y, Innocentin S, Withers DR, Roberts NA, Gallagher AR, Grigorieva EF, et al. Exogenous stimuli maintain intraepithelial lymphocytes via aryl hydrocarbon receptor activation. Cell (2011) 147(3):629–40. doi:10.1016/j.cell.2011.09.025
60. Chen Y, Chou K, Fuchs E, Havran WL, Boismenu R. Protection of the intestinal mucosa by intraepithelial gamma delta T cells. Proc Natl Acad Sci U S A (2002) 99(22):14338–43. doi:10.1073/pnas.212290499
61. Wang HC, Zhou Q, Dragoo J, Klein JR. Most murine CD8+ intestinal intraepithelial lymphocytes are partially but not fully activated T cells. J Immunol (2002) 169(9):4717–22. doi:10.4049/jimmunol.169.9.4717
62. Ogata M, Ota Y, Matsutani T, Nanno M, Suzuki R, Itoh T. Granzyme B-dependent and perforin-independent DNA fragmentation in intestinal epithelial cells induced by anti-CD3 mAb-activated intra-epithelial lymphocytes. Cell Tissue Res (2013) 352(2):287–300. doi:10.1007/s00441-012-1549-7
63. Yang H, Antony PA, Wildhaber BE, Teitelbaum DH. Intestinal intraepithelial lymphocyte gamma delta-T cell-derived keratinocyte growth factor modulates epithelial growth in the mouse. J Immunol (2004) 172(7):4151–8. doi:10.4049/jimmunol.172.7.4151
64. Smith AL, Hayday AC. An alphabeta T-cell-independent immunoprotective response towards gut coccidia is supported by gammadelta cells. Immunology (2000) 101(3):325–32. doi:10.1046/j.1365-2567.2000.00122.x
65. Ismail AS, Behrendt CL, Hooper LV. Reciprocal interactions between commensal bacteria and gamma delta intraepithelial lymphocytes during mucosal injury. J Immunol (2009) 182(5):3047–54. doi:10.4049/jimmunol.0802705
66. Cai SF, Fehniger TA, Cao X, Mayer JC, Brune JD, French AR, et al. Differential expression of granzyme B and C in murine cytotoxic lymphocytes. J Immunol (2009) 182(10):6287–97. doi:10.4049/jimmunol.0804333
67. Lambolez F, Arcangeli ML, Joret AM, Pasqualetto V, Cordier C, Di Santo JP, et al. The thymus exports long-lived fully committed T cell precursors that can colonize primary lymphoid organs. Nat Immunol (2006) 7(1):76–82. doi:10.1038/ni1293
68. Staton TL, Habtezion A, Winslow MM, Sato T, Love PE, Butcher EC. CD8+ recent thymic emigrants home to and efficiently repopulate the small intestine epithelium. Nat Immunol (2006) 7(5):482–8. doi:10.1038/ni1319
69. Lefrancois L, Goodman T. In vivo modulation of cytolytic activity and Thy-1 expression in TCR-gamma delta+ intraepithelial lymphocytes. Science (1989) 243(4899):1716–8. doi:10.1126/science.2564701
70. Kawaguchi M, Nanno M, Umesaki Y, Matsumoto S, Okada Y, Cai Z, et al. Cytolytic activity of intestinal intraepithelial lymphocytes in germ-free mice is strain dependent and determined by T cells expressing gamma delta T-cell antigen receptors. Proc Natl Acad Sci U S A (1993) 90(18):8591–4. doi:10.1073/pnas.90.18.8591
71. Stange J, Veldhoen M. The aryl hydrocarbon receptor in innate T cell immunity. Semin Immunopathol (2013) 35(6):645–55. doi:10.1007/s00281-013-0389-1
72. Moura-Alves P, Fae K, Houthuys E, Dorhoi A, Kreuchwig A, Furkert J, et al. AhR sensing of bacterial pigments regulates antibacterial defence. Nature (2014) 512(7515):387–92. doi:10.1038/nature13684
73. Yu Q, Tang C, Xun S, Yajima T, Takeda K, Yoshikai Y. MyD88-dependent signaling for IL-15 production plays an important role in maintenance of CD8 alpha alpha TCR alpha beta and TCR gamma delta intestinal intraepithelial lymphocytes. J Immunol (2006) 176(10):6180–5. doi:10.4049/jimmunol.176.10.6180
74. Rakoff-Nahoum S, Paglino J, Eslami-Varzaneh F, Edberg S, Medzhitov R. Recognition of commensal microflora by toll-like receptors is required for intestinal homeostasis. Cell (2004) 118(2):229–41. doi:10.1016/j.cell.2004.07.002
75. Qiu Y, Pu A, Zheng H, Liu M, Chen W, Wang W, et al. TLR2-dependent signaling for IL-15 production is essential for the homeostasis of intestinal intraepithelial lymphocytes. Mediators Inflamm (2016) 2016:4281865. doi:10.1155/2016/4281865
76. Jiang W, Wang X, Zeng B, Liu L, Tardivel A, Wei H, et al. Recognition of gut microbiota by NOD2 is essential for the homeostasis of intestinal intraepithelial lymphocytes. J Exp Med (2013) 210(11):2465–76. doi:10.1084/jem.20122490
77. Borm ME, van Bodegraven AA, Mulder CJ, Kraal G, Bouma G. The effect of NOD2 activation on TLR2-mediated cytokine responses is dependent on activation dose and NOD2 genotype. Genes Immun (2008) 9(3):274–8. doi:10.1038/gene.2008.9
78. Pan Y, Tian T, Park CO, Lofftus SY, Mei S, Liu X, et al. Survival of tissue-resident memory T cells requires exogenous lipid uptake and metabolism. Nature (2017) 543(7644):252–6. doi:10.1038/nature21379
79. Burgio VL, Fais S, Boirivant M, Perrone A, Pallone F. Peripheral monocyte and naive T-cell recruitment and activation in Crohn’s disease. Gastroenterology (1995) 109(4):1029–38. doi:10.1016/0016-5085(95)90560-X
80. Maiuri L, Picarelli A, Boirivant M, Coletta S, Mazzilli MC, De Vincenzi M, et al. Definition of the initial immunologic modifications upon in vitro gliadin challenge in the small intestine of celiac patients. Gastroenterology (1996) 110(5):1368–78. doi:10.1053/gast.1996.v110.pm8613040
81. Fonseca-Camarillo G, Yamamoto-Furusho JK. Immunoregulatory pathways involved in inflammatory bowel disease. Inflamm Bowel Dis (2015) 21(9):2188–93. doi:10.1097/MIB.0000000000000477
82. Hoffmann JC, Peters K, Henschke S, Herrmann B, Pfister K, Westermann J, et al. Role of T lymphocytes in rat 2,4,6-trinitrobenzene sulphonic acid (TNBS) induced colitis: increased mortality after gammadelta T cell depletion and no effect of alphabeta T cell depletion. Gut (2001) 48(4):489–95. doi:10.1136/gut.48.4.489
83. Poussier P, Ning T, Banerjee D, Julius M. A unique subset of self-specific intraintestinal T cells maintains gut integrity. J Exp Med (2002) 195(11):1491–7. doi:10.1084/jem.20011793
84. Tsuchiya T, Fukuda S, Hamada H, Nakamura A, Kohama Y, Ishikawa H, et al. Role of gamma delta T cells in the inflammatory response of experimental colitis mice. J Immunol (2003) 171(10):5507–13. doi:10.4049/jimmunol.171.10.5507
85. Zhang Y, Li Q, Rao E, Sun Y, Grossmann ME, Morris RJ, et al. Epidermal fatty acid binding protein promotes skin inflammation induced by high-fat diet. Immunity (2015) 42(5):953–64. doi:10.1016/j.immuni.2015.04.016
86. van der Windt GJ, Everts B, Chang CH, Curtis JD, Freitas TC, Amiel E, et al. Mitochondrial respiratory capacity is a critical regulator of CD8+ T cell memory development. Immunity (2012) 36(1):68–78. doi:10.1016/j.immuni.2011.12.007
87. O’Sullivan D, van der Windt GJ, Huang SC, Curtis JD, Chang CH, Buck MD, et al. Memory CD8(+) T cells use cell-intrinsic lipolysis to support the metabolic programming necessary for development. Immunity (2014) 41(1):75–88. doi:10.1016/j.immuni.2014.06.005
88. Barbee SD, Woodward MJ, Turchinovich G, Mention JJ, Lewis JM, Boyden LM, et al. Skint-1 is a highly specific, unique selecting component for epidermal T cells. Proc Natl Acad Sci U S A (2011) 108(8):3330–5. doi:10.1073/pnas.1010890108
89. Turchinovich G, Hayday AC. Skint-1 identifies a common molecular mechanism for the development of interferon-gamma-secreting versus interleukin-17-secreting gammadelta T cells. Immunity (2011) 35(1):59–68. doi:10.1016/j.immuni.2011.04.018
90. Di Marco Barros R, Roberts NA, Dart RJ, Vantourout P, Jandke A, Nussbaumer O, et al. Epithelia use butyrophilin-like molecules to shape organ-specific gammadelta T cell compartments. Cell (2016) 167(1):203–218e217. doi:10.1016/j.cell.2016.08.030
91. Park SH, Guy-Grand D, Lemonnier FA, Wang CR, Bendelac A, Jabri B. Selection and expansion of CD8alpha/alpha(1) T cell receptor alpha/beta(1) intestinal intraepithelial lymphocytes in the absence of both classical major histocompatibility complex class I and nonclassical CD1 molecules. J Exp Med (1999) 190(6):885–90. doi:10.1084/jem.190.6.885
92. Rhodes DA, Reith W, Trowsdale J. Regulation of immunity by butyrophilins. Annu Rev Immunol (2016) 34:151–72. doi:10.1146/annurev-immunol-041015-055435
93. Hayday AC. Gammadelta T cells and the lymphoid stress-surveillance response. Immunity (2009) 31(2):184–96. doi:10.1016/j.immuni.2009.08.006
94. Brucklacher-Waldert V, Ferreira C, Stebegg M, Fesneau F, Innocentin S, Marie JC, et al. Cellular stress in context of an inflammatory environment supports TGFβ independent T helper-17 differentiation. Cell Rep (2017) 19(11). doi:10.1016/j.celrep.2017.05.052
95. Adams EJ, Chien YH, Garcia KC. Structure of a gammadelta T cell receptor in complex with the nonclassical MHC T22. Science (2005) 308(5719):227–31. doi:10.1126/science.1106885
96. Adams EJ, Strop P, Shin S, Chien YH, Garcia KC. An autonomous CDR3delta is sufficient for recognition of the nonclassical MHC class I molecules T10 and T22 by gammadelta T cells. Nat Immunol (2008) 9(7):777–84. doi:10.1038/ni.1620
97. Hayday AC. [gamma][delta] cells: a right time and a right place for a conserved third way of protection. Annu Rev Immunol (2000) 18:975–1026. doi:10.1146/annurev.immunol.18.1.975
98. Scotet E, Martinez LO, Grant E, Barbaras R, Jeno P, Guiraud M, et al. Tumor recognition following Vgamma9Vdelta2 T cell receptor interactions with a surface F1-ATPase-related structure and apolipoprotein A-I. Immunity (2005) 22(1):71–80. doi:10.1016/j.immuni.2004.11.012
99. Martin B, Hirota K, Cua DJ, Stockinger B, Veldhoen M. Interleukin-17-producing gammadelta T cells selectively expand in response to pathogen products and environmental signals. Immunity (2009) 31(2):321–30. doi:10.1016/j.immuni.2009.06.020
100. Leggat JA, Gibbons DL, Haque SF, Smith AL, Wells JW, Choy K, et al. Innate responsiveness of CD8 memory T-cell populations nonspecifically inhibits allergic sensitization. J Allergy Clin Immunol (2008) 122(5):e1014. doi:10.1016/j.jaci.2008.08.011
101. Sutton CE, Lalor SJ, Sweeney CM, Brereton CF, Lavelle EC, Mills KH. Interleukin-1 and IL-23 induce innate IL-17 production from gammadelta T cells, amplifying Th17 responses and autoimmunity. Immunity (2009) 31(2):331–41. doi:10.1016/j.immuni.2009.08.001
102. Ferran C, Sheehan K, Dy M, Schreiber R, Merite S, Landais P, et al. Cytokine-related syndrome following injection of anti-CD3 monoclonal antibody: further evidence for transient in vivo T cell activation. Eur J Immunol (1990) 20(3):509–15. doi:10.1002/eji.1830200308
103. Finck BK, Yung CM, Carteron NL, Wofsy D. The role of T-cell subsets in the response to anti-CD3 monoclonal antibodies. Clin Immunol Immunopathol (1992) 65(3):234–41. doi:10.1016/0090-1229(92)90152-E
104. Radojevic N, McKay DM, Merger M, Vallance BA, Collins SM, Croitoru K. Characterization of enteric functional changes evoked by in vivo anti-CD3 T cell activation. Am J Physiol (1999) 276(3 Pt 2):R715–23.
105. Yaguchi K, Kayaba S, Soga H, Yamagishi M, Tamura A, Kasahara S, et al. DNA fragmentation and detachment of enterocytes induced by anti-CD3 mAb-activated intraepithelial lymphocytes. Cell Tissue Res (2004) 315(1):71–84. doi:10.1007/s00441-003-0795-0
106. Ogata M, Itoh T. Gamma/delta intraepithelial lymphocytes in the mouse small intestine. Anat Sci Int (2016) 91(4):301–12. doi:10.1007/s12565-016-0341-2
107. Swamy M, Abeler-Dorner L, Chettle J, Mahlakoiv T, Goubau D, Chakravarty P, et al. Intestinal intraepithelial lymphocyte activation promotes innate antiviral resistance. Nat Commun (2015) 6:7090. doi:10.1038/ncomms8090
108. Macpherson AJ, Uhr T. Induction of protective IgA by intestinal dendritic cells carrying commensal bacteria. Science (2004) 303(5664):1662–5. doi:10.1126/science.1091334
109. Tanaka Y, Morita CT, Tanaka Y, Nieves E, Brenner MB, Bloom BR. Natural and synthetic non-peptide antigens recognized by human gamma delta T cells. Nature (1995) 375(6527):155–8. doi:10.1038/375155a0
110. Medzhitov R, Janeway CA Jr. How does the immune system distinguish self from nonself? Semin Immunol (2000) 12(3):185–8; discussion 257–344. doi:10.1006/smim.2000.0230
111. Sander LE, Davis MJ, Boekschoten MV, Amsen D, Dascher CC, Ryffel B, et al. Detection of prokaryotic mRNA signifies microbial viability and promotes immunity. Nature (2011) 474(7351):385–9. doi:10.1038/nature10072
112. Slack E, Balmer ML, Fritz JH, Hapfelmeier S. Functional flexibility of intestinal IgA – broadening the fine line. Front Immunol (2012) 3:100. doi:10.3389/fimmu.2012.00100
113. Palm NW, de Zoete MR, Cullen TW, Barry NA, Stefanowski J, Hao L, et al. Immunoglobulin A coating identifies colitogenic bacteria in inflammatory bowel disease. Cell (2014) 158(5):1000–10. doi:10.1016/j.cell.2014.08.006
114. Fujihashi K, McGhee JR, Kweon MN, Cooper MD, Tonegawa S, Takahashi I, et al. gamma/delta T cell-deficient mice have impaired mucosal immunoglobulin A responses. J Exp Med (1996) 183(4):1929–35. doi:10.1084/jem.183.4.1929
115. Wang L, Kamath A, Das H, Li L, Bukowski JF. Antibacterial effect of human V gamma 2V delta 2 T cells in vivo. J Clin Invest (2001) 108(9):1349–57. doi:10.1172/JCI13584
116. Linterman MA, Denton AE, Divekar DP, Zvetkova I, Kane L, Ferreira C, et al. CD28 expression is required after T cell priming for helper T cell responses and protective immunity to infection. Elife (2014) 3. doi:10.7554/eLife.03180
117. Lefrancois L. Phenotypic complexity of intraepithelial lymphocytes of the small intestine. J Immunol (1991) 147(6):1746–51.
118. Van Houten N, Mixter PF, Wolfe J, Budd RC. CD2 expression on murine intestinal intraepithelial lymphocytes is bimodal and defines proliferative capacity. Int Immunol (1993) 5(6):665–72. doi:10.1093/intimm/5.6.665
119. Ohishi K, Varnum-Finney B, Bernstein ID. Delta-1 enhances marrow and thymus repopulating ability of human CD34(+)CD38(-) cord blood cells. J Clin Invest (2002) 110(8):1165–74. doi:10.1172/JCI16167
120. Marriott CL, Mackley EC, Ferreira C, Veldhoen M, Yagita H, Withers DR. OX40 controls effector CD4+ T-cell expansion, not follicular T helper cell generation in acute Listeria infection. Eur J Immunol (2014) 44(8):2437–47. doi:10.1002/eji.201344211
121. Wang HC, Klein JR. Multiple levels of activation of murine CD8(+) intraepithelial lymphocytes defined by OX40 (CD134) expression: effects on cell-mediated cytotoxicity, IFN-gamma, and IL-10 regulation. J Immunol (2001) 167(12):6717–23. doi:10.4049/jimmunol.167.12.6717
122. Souza HS, Elia CC, Spencer J, MacDonald TT. Expression of lymphocyte-endothelial receptor-ligand pairs, alpha4beta7/MAdCAM-1 and OX40/OX40 ligand in the colon and jejunum of patients with inflammatory bowel disease. Gut (1999) 45(6):856–63. doi:10.1136/gut.45.6.856
123. Stuber E, Buschenfeld A, Luttges J, Von Freier A, Arendt T, Folsch UR. The expression of OX40 in immunologically mediated diseases of the gastrointestinal tract (celiac disease, Crohn’s disease, ulcerative colitis). Eur J Clin Invest (2000) 30(7):594–9. doi:10.1046/j.1365-2362.2000.00658.x
124. Witherden DA, Verdino P, Rieder SE, Garijo O, Mills RE, Teyton L, et al. The junctional adhesion molecule JAML is a costimulatory receptor for epithelial gammadelta T cell activation. Science (2010) 329(5996):1205–10. doi:10.1126/science.1192698
125. Verdino P, Witherden DA, Ferguson MS, Corper AL, Schiefner A, Havran WL, et al. Molecular insights into gammadelta T cell costimulation by an anti-JAML antibody. Structure (2011) 19(1):80–9. doi:10.1016/j.str.2010.10.007
126. Weber DA, Sumagin R, McCall IC, Leoni G, Neumann PA, Andargachew R, et al. Neutrophil-derived JAML inhibits repair of intestinal epithelial injury during acute inflammation. Mucosal Immunol (2014) 7(5):1221–32. doi:10.1038/mi.2014.12
127. Puddington L, Olson S, Lefrancois L. Interactions between stem cell factor and c-Kit are required for intestinal immune system homeostasis. Immunity (1994) 1(9):733–9. doi:10.1016/S1074-7613(94)80015-4
128. Lodolce JP, Boone DL, Chai S, Swain RE, Dassopoulos T, Trettin S, et al. IL-15 receptor maintains lymphoid homeostasis by supporting lymphocyte homing and proliferation. Immunity (1998) 9(5):669–76. doi:10.1016/S1074-7613(00)80664-0
129. Kennedy MK, Glaccum M, Brown SN, Butz EA, Viney JL, Embers M, et al. Reversible defects in natural killer and memory CD8 T cell lineages in interleukin 15-deficient mice. J Exp Med (2000) 191(5):771–80. doi:10.1084/jem.191.5.771
130. Schluns KS, Nowak EC, Cabrera-Hernandez A, Puddington L, Lefrancois L, Aguila HL. Distinct cell types control lymphoid subset development by means of IL-15 and IL-15 receptor alpha expression. Proc Natl Acad Sci U S A (2004) 101(15):5616–21. doi:10.1073/pnas.0307442101
131. Ma LJ, Acero LF, Zal T, Schluns KS. Trans-presentation of IL-15 by intestinal epithelial cells drives development of CD8alphaalpha IELs. J Immunol (2009) 183(2):1044–54. doi:10.4049/jimmunol.0900420
132. Dubois S, Mariner J, Waldmann TA, Tagaya Y. IL-15ralpha recycles and presents IL-15 in trans to neighboring cells. Immunity (2002) 17(5):537–47. doi:10.1016/S1074-7613(02)00429-6
133. Malamut G, El Machhour R, Montcuquet N, Martin-Lanneree S, Dusanter-Fourt I, Verkarre V, et al. IL-15 triggers an antiapoptotic pathway in human intraepithelial lymphocytes that is a potential new target in celiac disease-associated inflammation and lymphomagenesis. J Clin Invest (2010) 120(6):2131–43. doi:10.1172/JCI41344
134. Mackay LK, Wynne-Jones E, Freestone D, Pellicci DG, Mielke LA, Newman DM, et al. T-box transcription factors combine with the cytokines TGF-beta and IL-15 to control tissue-resident memory T cell fate. Immunity (2015) 43(6):1101–11. doi:10.1016/j.immuni.2015.11.008
135. Meresse B, Chen Z, Ciszewski C, Tretiakova M, Bhagat G, Krausz TN, et al. Coordinated induction by IL-15 of a TCR-independent NKG2D signaling pathway converts CTL into lymphokine-activated killer cells in celiac disease. Immunity (2004) 21(3):357–66. doi:10.1016/j.immuni.2004.06.020
136. Itsumi M, Yoshikai Y, Yamada H. IL-15 is critical for the maintenance and innate functions of self-specific CD8(+) T cells. Eur J Immunol (2009) 39(7):1784–93. doi:10.1002/eji.200839106
137. DePaolo RW, Abadie V, Tang F, Fehlner-Peach H, Hall JA, Wang W, et al. Co-adjuvant effects of retinoic acid and IL-15 induce inflammatory immunity to dietary antigens. Nature (2011) 471(7337):220–4. doi:10.1038/nature09849
138. Jabri B, Sollid LM. Tissue-mediated control of immunopathology in coeliac disease. Nat Rev Immunol (2009) 9(12):858–70. doi:10.1038/nri2670
139. Strengell M, Matikainen S, Siren J, Lehtonen A, Foster D, Julkunen I, et al. IL-21 in synergy with IL-15 or IL-18 enhances IFN-gamma production in human NK and T cells. J Immunol (2003) 170(11):5464–9. doi:10.4049/jimmunol.170.11.5464
140. Sarra M, Cupi ML, Monteleone I, Franze E, Ronchetti G, Di Sabatino A, et al. IL-15 positively regulates IL-21 production in celiac disease mucosa. Mucosal Immunol (2013) 6(2):244–55. doi:10.1038/mi.2012.65
141. Jameson SC. Maintaining the norm: T-cell homeostasis. Nat Rev Immunol (2002) 2(8):547–56. doi:10.1038/nri853
142. Laky K, Lefrancois L, Lingenheld EG, Ishikawa H, Lewis JM, Olson S, et al. Enterocyte expression of interleukin 7 induces development of gammadelta T cells and Peyer’s patches. J Exp Med (2000) 191(9):1569–80. doi:10.1084/jem.191.9.1569
143. Hara T, Shitara S, Imai K, Miyachi H, Kitano S, Yao H, et al. Identification of IL-7-producing cells in primary and secondary lymphoid organs using IL-7-GFP knock-in mice. J Immunol (2012) 189(4):1577–84. doi:10.4049/jimmunol.1200586
144. Shalapour S, Deiser K, Kuhl AA, Glauben R, Krug SM, Fischer A, et al. Interleukin-7 links T lymphocyte and intestinal epithelial cell homeostasis. PLoS One (2012) 7(2):e31939. doi:10.1371/journal.pone.0031939
145. Moore TA, von Freeden-Jeffry U, Murray R, Zlotnik A. Inhibition of gamma delta T cell development and early thymocyte maturation in IL-7-/- mice. J Immunol (1996) 157(6):2366–73.
146. Colpitts SL, Stoklasek TA, Plumlee CR, Obar JJ, Guo C, Lefrancois L. Cutting edge: the role of IFN-alpha receptor and MyD88 signaling in induction of IL-15 expression in vivo. J Immunol (2012) 188(6):2483–7. doi:10.4049/jimmunol.1103609
147. Yang H, Spencer AU, Teitelbaum DH. Interleukin-7 administration alters intestinal intraepithelial lymphocyte phenotype and function in vivo. Cytokine (2005) 31(6):419–28. doi:10.1016/j.cyto.2005.06.014
148. Watanabe M, Ueno Y, Yajima T, Okamoto S, Hayashi T, Yamazaki M, et al. Interleukin 7 transgenic mice develop chronic colitis with decreased interleukin 7 protein accumulation in the colonic mucosa. J Exp Med (1998) 187(3):389–402. doi:10.1084/jem.187.3.389
149. Taylor BC, Zaph C, Troy AE, Du Y, Guild KJ, Comeau MR, et al. TSLP regulates intestinal immunity and inflammation in mouse models of helminth infection and colitis. J Exp Med (2009) 206(3):655–67. doi:10.1084/jem.20081499
150. Rochman Y, Leonard WJ. The role of thymic stromal lymphopoietin in CD8+ T cell homeostasis. J Immunol (2008) 181(11):7699–705. doi:10.4049/jimmunol.181.11.7699
151. Rimoldi M, Chieppa M, Salucci V, Avogadri F, Sonzogni A, Sampietro GM, et al. Intestinal immune homeostasis is regulated by the crosstalk between epithelial cells and dendritic cells. Nat Immunol (2005) 6(5):507–14. doi:10.1038/ni1192
152. Watanabe T, Kitani A, Murray PJ, Wakatsuki Y, Fuss IJ, Strober W. Nucleotide binding oligomerization domain 2 deficiency leads to dysregulated TLR2 signaling and induction of antigen-specific colitis. Immunity (2006) 25(3):473–85. doi:10.1016/j.immuni.2006.06.018
153. Klimpel GR, Langley KE, Wypych J, Abrams JS, Chopra AK, Niesel DW. A role for stem cell factor (SCF): c-Kit interaction(s) in the intestinal tract response to Salmonella typhimurium infection. J Exp Med (1996) 184(1):271–6. doi:10.1084/jem.184.1.271
154. Wang T, Langley KE, Gourley WK, Klimpel GR. Stem cell factor (SCF) can regulate the activation and expansion of murine intraepithelial lymphocytes. Cytokine (2000) 12(3):272–80. doi:10.1006/cyto.1999.0551
155. Hayday A, Tigelaar R. Immunoregulation in the tissues by gammadelta T cells. Nat Rev Immunol (2003) 3(3):233–42. doi:10.1038/nri1030
156. Ismail AS, Severson KM, Vaishnava S, Behrendt CL, Yu X, Benjamin JL, et al. Gammadelta intraepithelial lymphocytes are essential mediators of host-microbial homeostasis at the intestinal mucosal surface. Proc Natl Acad Sci U S A (2011) 108(21):8743–8. doi:10.1073/pnas.1019574108
157. Roberts SJ, Smith AL, West AB, Wen L, Findly RC, Owen MJ, et al. T-cell alpha beta + and gamma delta + deficient mice display abnormal but distinct phenotypes toward a natural, widespread infection of the intestinal epithelium. Proc Natl Acad Sci U S A (1996) 93(21):11774–9. doi:10.1073/pnas.93.21.11774
158. Inagaki-Ohara K, Dewi FN, Hisaeda H, Smith AL, Jimi F, Miyahira M, et al. Intestinal intraepithelial lymphocytes sustain the epithelial barrier function against Eimeria vermiformis infection. Infect Immun (2006) 74(9):5292–301. doi:10.1128/IAI.02024-05
159. Dalton JE, Cruickshank SM, Egan CE, Mears R, Newton DJ, Andrew EM, et al. Intraepithelial gammadelta+ lymphocytes maintain the integrity of intestinal epithelial tight junctions in response to infection. Gastroenterology (2006) 131(3):818–29. doi:10.1053/j.gastro.2006.06.003
160. Edelblum KL, Singh G, Odenwald MA, Lingaraju A, El Bissati K, McLeod R, et al. Gammadelta intraepithelial lymphocyte migration limits transepithelial pathogen invasion and systemic disease in mice. Gastroenterology (2015) 148(7):1417–26. doi:10.1053/j.gastro.2015.02.053
161. Moretto M, Durell B, Schwartzman JD, Khan IA. Gamma delta T cell-deficient mice have a down-regulated CD8+ T cell immune response against Encephalitozoon cuniculi infection. J Immunol (2001) 166(12):7389–97. doi:10.4049/jimmunol.166.12.7389
162. Chien YH, Meyer C, Bonneville M. Gammadelta T cells: first line of defense and beyond. Annu Rev Immunol (2014) 32:121–55. doi:10.1146/annurev-immunol-032713-120216
163. Ganz T. Defensins: antimicrobial peptides of innate immunity. Nat Rev Immunol (2003) 3(9):710–20. doi:10.1038/nri1180
164. Mankertz J, Tavalali S, Schmitz H, Mankertz A, Riecken EO, Fromm M, et al. Expression from the human occludin promoter is affected by tumor necrosis factor alpha and interferon gamma. J Cell Sci (2000) 113(Pt 11):2085–90.
165. Dharakul T, Rott L, Greenberg HB. Recovery from chronic rotavirus infection in mice with severe combined immunodeficiency: virus clearance mediated by adoptive transfer of immune CD8+ T lymphocytes. J Virol (1990) 64(9):4375–82.
166. Sydora BC, Jamieson BD, Ahmed R, Kronenberg M. Intestinal intraepithelial lymphocytes respond to systemic lymphocytic choriomeningitis virus infection. Cell Immunol (1996) 167(2):161–9. doi:10.1006/cimm.1996.0023
167. Muller S, Buhler-Jungo M, Mueller C. Intestinal intraepithelial lymphocytes exert potent protective cytotoxic activity during an acute virus infection. J Immunol (2000) 164(4):1986–94. doi:10.4049/jimmunol.164.4.1986
168. Karst SM, Wobus CE, Lay M, Davidson J, Virgin HW IV. STAT1-dependent innate immunity to a Norwalk-like virus. Science (2003) 299(5612):1575–8. doi:10.1126/science.1077905
169. Nice TJ, Baldridge MT, McCune BT, Norman JM, Lazear HM, Artyomov M, et al. Interferon-lambda cures persistent murine norovirus infection in the absence of adaptive immunity. Science (2015) 347(6219):269–73. doi:10.1126/science.1258100
170. Hernandez PP, Mahlakoiv T, Yang I, Schwierzeck V, Nguyen N, Guendel F, et al. Interferon-lambda and interleukin 22 act synergistically for the induction of interferon-stimulated genes and control of rotavirus infection. Nat Immunol (2015) 16(7):698–707. doi:10.1038/ni.3180
171. Mahlakoiv T, Hernandez P, Gronke K, Diefenbach A, Staeheli P. Leukocyte-derived IFN-alpha/beta and epithelial IFN-lambda constitute a compartmentalized mucosal defense system that restricts enteric virus infections. PLoS Pathog (2015) 11(4):e1004782. doi:10.1371/journal.ppat.1004782
172. Kuss SK, Best GT, Etheredge CA, Pruijssers AJ, Frierson JM, Hooper LV, et al. Intestinal microbiota promote enteric virus replication and systemic pathogenesis. Science (2011) 334(6053):249–52. doi:10.1126/science.1211057
173. von Hertzen L, Beutler B, Bienenstock J, Blaser M, Cani PD, Eriksson J, et al. Helsinki alert of biodiversity and health. Ann Med (2015) 47(3):218–25. doi:10.3109/07853890.2015.1010226
174. Attia S, Versloot CJ, Voskuijl W, van Vliet SJ, Di Giovanni V, Zhang L, et al. Mortality in children with complicated severe acute malnutrition is related to intestinal and systemic inflammation: an observational cohort study. Am J Clin Nutr (2016) 104(5):1441–9. doi:10.3945/ajcn.116.130518
175. Koga T, McGhee JR, Kato H, Kato R, Kiyono H, Fujihashi K. Evidence for early aging in the mucosal immune system. J Immunol (2000) 165(9):5352–9. doi:10.4049/jimmunol.165.9.5352
176. Glocker E, Grimbacher B. Inflammatory bowel disease: is it a primary immunodeficiency? Cell Mol Life Sci (2012) 69(1):41–8. doi:10.1007/s00018-011-0837-9
177. Vinh DC, Behr MA. Crohn’s as an immune deficiency: from apparent paradox to evolving paradigm. Expert Rev Clin Immunol (2013) 9(1):17–30. doi:10.1586/eci.12.87
178. de Souza HS, Fiocchi C. Immunopathogenesis of IBD: current state of the art. Nat Rev Gastroenterol Hepatol (2016) 13(1):13–27. doi:10.1038/nrgastro.2015.186
179. Ryan P, Kelly RG, Lee G, Collins JK, O’Sullivan GC, O’Connell J, et al. Bacterial DNA within granulomas of patients with Crohn’s disease – detection by laser capture microdissection and PCR. Am J Gastroenterol (2004) 99(8):1539–43. doi:10.1111/j.1572-0241.2004.40103.x
180. Veldhoen M. Interleukin 17 is a chief orchestrator of immunity. Nat Immunol (2017) 18(6):612–21. doi:10.1038/ni.3742
181. MacDonald TT, Spencer J. Evidence that activated mucosal T cells play a role in the pathogenesis of enteropathy in human small intestine. J Exp Med (1988) 167(4):1341–9. doi:10.1084/jem.167.4.1341
182. Peluso I, Pallone F, Monteleone G. Interleukin-12 and Th1 immune response in Crohn’s disease: pathogenetic relevance and therapeutic implication. World J Gastroenterol (2006) 12(35):5606–10. doi:10.3748/wjg.v12.i35.5606
183. Watanabe M, Ueno Y, Yajima T, Iwao Y, Tsuchiya M, Ishikawa H, et al. Interleukin 7 is produced by human intestinal epithelial cells and regulates the proliferation of intestinal mucosal lymphocytes. J Clin Invest (1995) 95(6):2945–53. doi:10.1172/JCI118002
184. Liu Z, Geboes K, Colpaert S, D’Haens GR, Rutgeerts P, Ceuppens JL. IL-15 is highly expressed in inflammatory bowel disease and regulates local T cell-dependent cytokine production. J Immunol (2000) 164(7):3608–15. doi:10.4049/jimmunol.164.7.3608
185. McVay LD, Li B, Biancaniello R, Creighton MA, Bachwich D, Lichtenstein G, et al. Changes in human mucosal gamma delta T cell repertoire and function associated with the disease process in inflammatory bowel disease. Mol Med (1997) 3(3):183–203.
186. Inagaki-Ohara K, Chinen T, Matsuzaki G, Sasaki A, Sakamoto Y, Hiromatsu K, et al. Mucosal T cells bearing TCRgammadelta play a protective role in intestinal inflammation. J Immunol (2004) 173(2):1390–8. doi:10.4049/jimmunol.173.2.1390
187. Kohyama M, Nanno M, Kawaguchi-Miyashita M, Shimada S, Watanabe M, Hibi T, et al. Cytolytic and IFN-gamma-producing activities of gamma delta T cells in the mouse intestinal epithelium are T cell receptor-beta-chain dependent. Proc Natl Acad Sci U S A (1999) 96(13):7451–5. doi:10.1073/pnas.96.13.7451
188. Kawaguchi-Miyashita M, Shimada S, Kurosu H, Kato-Nagaoka N, Matsuoka Y, Ohwaki M, et al. An accessory role of TCRgammadelta (+) cells in the exacerbation of inflammatory bowel disease in TCRalpha mutant mice. Eur J Immunol (2001) 31(4):980–8. doi:10.1002/1521-4141(200104)31:4<980::AID-IMMU980>3.0.CO;2-U
189. Kadivar M, Petersson J, Svensson L, Marsal J. CD8alphabeta+ gammadelta T Cells: a novel T cell subset with a potential role in inflammatory bowel disease. J Immunol (2016) 197(12):4584–92. doi:10.4049/jimmunol.1601146
190. Hugot JP, Chamaillard M, Zouali H, Lesage S, Cezard JP, Belaiche J, et al. Association of NOD2 leucine-rich repeat variants with susceptibility to Crohn’s disease. Nature (2001) 411(6837):599–603. doi:10.1038/35079107
191. Ogura Y, Bonen DK, Inohara N, Nicolae DL, Chen FF, Ramos R, et al. A frameshift mutation in NOD2 associated with susceptibility to Crohn’s disease. Nature (2001) 411(6837):603–6. doi:10.1038/35079114
192. Girardin SE, Hugot JP, Sansonetti PJ. Lessons from Nod2 studies: towards a link between Crohn’s disease and bacterial sensing. Trends Immunol (2003) 24(12):652–8. doi:10.1016/j.it.2003.10.007
193. Liu JZ, van Sommeren S, Huang H, Ng SC, Alberts R, Takahashi A, et al. Association analyses identify 38 susceptibility loci for inflammatory bowel disease and highlight shared genetic risk across populations. Nat Genet (2015) 47(9):979–86. doi:10.1038/ng.3359
194. Watanabe T, Kitani A, Murray PJ, Strober W. NOD2 is a negative regulator of toll-like receptor 2-mediated T helper type 1 responses. Nat Immunol (2004) 5(8):800–8. doi:10.1038/ni1092
195. Fukushima K, Masuda T, Ohtani H, Sasaki I, Funayama Y, Matsuno S, et al. Immunohistochemical characterization, distribution, and ultrastructure of lymphocytes bearing T-cell receptor gamma/delta in inflammatory bowel disease. Gastroenterology (1991) 101(3):670–8. doi:10.1016/0016-5085(91)90524-O
196. Bucht A, Soderstrom K, Esin S, Grunewald J, Hagelberg S, Magnusson I, et al. Analysis of gamma delta V region usage in normal and diseased human intestinal biopsies and peripheral blood by polymerase chain reaction (PCR) and flow cytometry. Clin Exp Immunol (1995) 99(1):57–64. doi:10.1111/j.1365-2249.1995.tb03472.x
197. McCarthy NE, Bashir Z, Vossenkamper A, Hedin CR, Giles EM, Bhattacharjee S, et al. Proinflammatory Vdelta2+ T cells populate the human intestinal mucosa and enhance IFN-gamma production by colonic alphabeta T cells. J Immunol (2013) 191(5):2752–63. doi:10.4049/jimmunol.1202959
198. Kuhl AA, Pawlowski NN, Grollich K, Loddenkemper C, Zeitz M, Hoffmann JC. Aggravation of intestinal inflammation by depletion/deficiency of gammadelta T cells in different types of IBD animal models. J Leukoc Biol (2007) 81(1):168–75. doi:10.1189/jlb.1105696
Keywords: mucosal immunology, intraepithelial lymphocytes, inflammatory bowel disease, CD8+ T-lymphocytes, epithelial cells
Citation: Konjar Š, Ferreira C, Blankenhaus B and Veldhoen M (2017) Intestinal Barrier Interactions with Specialized CD8 T Cells. Front. Immunol. 8:1281. doi: 10.3389/fimmu.2017.01281
Received: 29 May 2017; Accepted: 25 September 2017;
Published: 11 October 2017
Edited by:
Christoph Becker, University of Erlangen-Nuremberg, GermanyReviewed by:
Gianluca Matteoli, KU Leuven, BelgiumRita Carsetti, Bambino Gesù Ospedale Pediatrico (IRCCS), Italy
Copyright: © 2017 Konjar, Ferreira, Blankenhaus and Veldhoen. This is an open-access article distributed under the terms of the Creative Commons Attribution License (CC BY). The use, distribution or reproduction in other forums is permitted, provided the original author(s) or licensor are credited and that the original publication in this journal is cited, in accordance with accepted academic practice. No use, distribution or reproduction is permitted which does not comply with these terms.
*Correspondence: Marc Veldhoen, bWFyYy52ZWxkaG9lbkBtZWRpY2luYS51bGlzYm9hLnB0