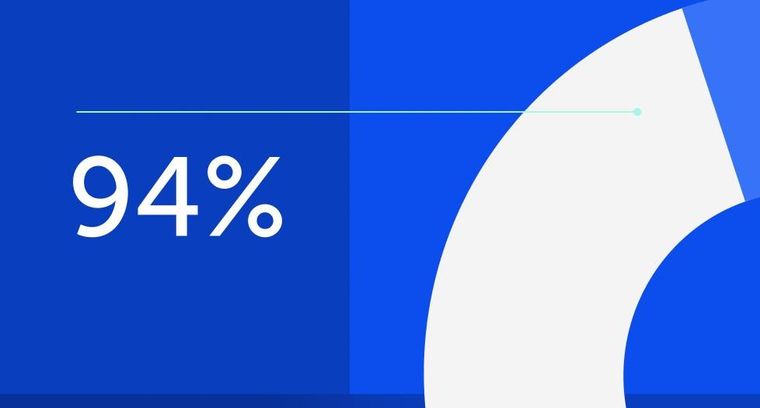
94% of researchers rate our articles as excellent or good
Learn more about the work of our research integrity team to safeguard the quality of each article we publish.
Find out more
REVIEW article
Front. Immunol., 05 October 2017
Sec. Vaccines and Molecular Therapeutics
Volume 8 - 2017 | https://doi.org/10.3389/fimmu.2017.01262
This article is part of the Research TopicReassessing Twenty Years of Vaccine Development Against TuberculosisView all 9 articles
Tuberculosis (TB), caused by Mycobacterium tuberculosis (Mtb), remains a leading cause of morbidity and mortality worldwide, despite the widespread use of the only licensed vaccine, Bacille Calmette Guerin (BCG). Eradication of TB will require a more effective vaccine, yet evaluation of new vaccine candidates is hampered by lack of defined correlates of protection. Animal and human studies of intracellular pathogens have extensively evaluated polyfunctional CD4+ T cells producing multiple pro-inflammatory cytokines (IFN-γ, TNF-α, and IL-2) as a possible correlate of protection from infection and disease. In this study, we review the published literature that evaluates whether or not BCG and/or novel TB vaccine candidates induce polyfunctional CD4+ T cells and if these T cell responses correlate with vaccine-mediated protection. Ample evidence suggests that BCG and several novel vaccine candidates evaluated in animal models and humans induce polyfunctional CD4+ T cells. However, while a number of studies utilizing the mouse TB model support that polyfunctional CD4+ T cells are associated with vaccine-induced protection, other studies in mouse and human infants demonstrate no correlation between these T cell responses and protection. We conclude that induction of polyfunctional CD4+ T cells is certainly not sufficient and may not even be necessary to mediate protection and suggest that other functional attributes, such as additional effector functions, T cell differentiation state, tissue homing potential, or long-term survival capacity of the T cell may be equally or more important to promote protection. Thus, a correlate of protection for TB vaccine development remains elusive. Future studies should address polyfunctional CD4+ T cells within the context of more comprehensive immunological signatures of protection that include other functions and phenotypes of T cells as well as the full spectrum of immune cells and mediators that participate in the immune response against Mtb.
Despite the widespread global use of the only licensed tuberculosis (TB) vaccine, Bacille Calmette Guerin (BCG), TB remains a significant cause of morbidity and mortality, with 10.4 million cases and 1.8 million deaths each year (1). The WHO End TB strategy cites that a new and more efficacious vaccine is one of the interventions required for worldwide elimination of TB by 2035 (2). Yet a new vaccine that improves upon the partial protection provided by BCG remains elusive (3). One of the significant challenges to rational TB vaccine development is lack of identified immune correlates of protection. The absence of bone fide correlates hampers both pre-clinical research as well as clinical trials (4). The Food and Drug Administration defines a correlate of protection as a laboratory parameter, which is associated with protection from the occurrence of clinical disease as shown after sufficient and controlled trials (5). Correlates of protection can be further divided into ones that are causally responsible for protection (mechanistic correlates of protection) or ones that are significantly correlated with, though not the cause of protection (non-mechanistic correlates of protection) (6). The search for correlates of protection in the TB vaccine field has generally focused on mechanistic correlates and in this regard, polyfunctional CD4+ T cells, defined by the simultaneous co-expression of multiple pro-inflammatory cytokines (e.g., IFN-γ, TNF-α, IL-2) on a single cell level, have garnered much attention (4). While both polyfunctional CD4+ and CD8+ T cells have been defined, the TB vaccine literature investigating polyfunctional T cells as a possible correlate of protection, has focused almost exclusively on CD4+ T cells. Therefore, we limited our review to articles that referred to induction of IFN-γ+TNF-α+IL-2+ polyfunctional CD4+ T cells in the context of TB vaccination and vaccine immunogenicity in pre-clinical and clinical studies and focused on reviewing the published evidence that polyfunctional CD4+ T cells represent a mechanistic correlate of protection for TB vaccines.
With advances in multi-parameter flow cytometry to include seven or more parameters, in combination with intracellular cytokine staining (ICS) techniques, it became possible to evaluate the production of multiple cytokines simultaneously on a single cell basis. Polyfunctional CD4+ T cells were defined as those cells producing two or more cytokines, and were first defined within the context of examination of vaccine-induced T cell responses (7). De Rosa et al. showed that vaccines to Hepatitis B virus, tetanus, and HIV induced antigen-specific T cell responses, which were functionally complex, differed from the antigen-specific T cell responses elicited by natural infection, and were underestimated when measured by IFN-γ production alone. Furthermore, Bansal et al. demonstrated with an HIV vaccine that vaccines can elicit a more diverse array of T cells than natural infection and that vaccine dose and route can alter the cytokine profile and polyfunctionality of the T cells elicited (8). Furthermore, by varying the administration of the same vaccine, these authors illustrated that polyfunctional T cells possess a dynamic range that could be evaluated for correlates of protection in vaccine models.
Darrah et al. provided the first definitive evidence that the magnitude of the vaccine-induced polyfunctional CD4+ T-cell response was highly correlated with protection from infection (9). Using a mouse model of Leishmania disease, various vaccines induced CD4+ T cells displaying distinct cytokine profiles and different degrees of protection against disease upon L. major challenge. In this study, frequencies of MML-specific polyfunctional CD4+ T cells co-expressing IFN-γ, TNF-α, and IL-2 were correlated closely with the various degrees of protection elicited by a panel of vaccines. By comparison, the total number of IFN-γ-producing CD4+ T cells, CD4+ T cells producing IL-4 or IL-13, or the T regulatory cell response did not correlate with vaccine-induced protection. This study was also the first to show that BCG elicits polyfunctional CD4+ T cells in both the murine TB model, in which BCG mediates a degree of control of bacterial replication after Mycobacterium tuberculosis (Mtb) challenge, and in humans (9), as discussed further below.
In humans, polyfunctional CD4+ T cells have been studied with reference to severity of disease due to some intracellular infections [reviewed in Ref. (10)]. For example, slower progression to AIDS with HIV-2 than HIV-1 infection (11) and control of HIV-1 without anti-retroviral medications (12) are associated with high frequency polyfunctional HIV Gag-specific CD4+ T cells. By comparison, studies of polyfunctional CD4+ T cells in relationship to host containment of Mtb infection are contradictory. On the one hand, stronger mycobacteria-specific polyfunctional CD4+ T cell responses are found in adults with sputum smears negative for acid fast bacilli (AFB) than those with AFB smear positive TB (13), and in adults with latent Mtb infection (LTBI) than in those with TB (14, 15). Moreover, successful TB treatment, which rapidly reduces the bacterial load, is associated with marked increases in proportions of polyfunctional CD4+ T cells (13). On the other hand, other studies demonstrate that polyfunctional CD4+ T cell responses positively correlate with increased bacillary load. For example, there are also studies that showed stronger mycobacteria-specific polyfunctional CD4+ T cell responses in adults with TB than those with LTBI (16, 17) and in adults with TB than in those in healthy household contacts of adults with TB (18). These contradictory results highlight an important limitation of such correlative studies, which is that it is not possible to discern whether or not polyfunctionality of CD4+ T cells plays a causal role in immune control of the pathogen, or simply reflects the underlying bacterial burden.
The mechanism(s) by which polyfunctional CD4+ T cells induced by vaccines or natural infection may be associated with protection from infection and/or disease have not been defined. It is certainly conceivable that cells expressing multiple effector functions may be more effective in controlling infection than those producing a single pro-inflammatory cytokine. For example, IFN-γ and TNF-α act synergistically to enhance the ability of macrophages to contain L. major infection (19, 20), which in turn is associated with enhanced control of disease by the combination of IFN-γ and TNF-α in the murine model (20). Similarly, IFN-γ and TNF-α synergistically inhibit Mtb replication within murine macrophage cell lines (21). As first defined in the murine Leishmania model, among vaccine-induced CD4+ T cells producing IFN-γ, TNF-α, and/or IL-2, cells producing all three cytokines (3+ cells) produce more cytokine on a per cell basis [as defined by mean fluorescence intensity (MFI)], than do those that produce two cytokines (2+ cells), which in turn produce more cytokine than cells producing a single cytokine (1+ cells) (9). Moreover, Darrah et al. defined integrated MFI (iMFI), a metric that combines the frequency of each cytokine-producing CD4+ T cell response with its associated MFI and showed that the iMFI for each of IFN-γ, TNF-α, or IL-2, also correlated with the degree of vaccine-induced protection. This analysis is consistent with the interpretation that it may be the high potency of multifunctional T cells to produce cytokines on a per cell basis that is associated with vaccine-induced protection. Finally, Seder et al. proposed a linear differentiation model for CD4+ T cells based upon their cytokine profile (22). In this model, naïve CD4+ T cells, upon activation with antigen, first acquire the capacity to secrete TNF-α or IL-2, followed by the capacity to produce both cytokines and finally, upon further differentiation, additionally produce IFN-γ as well. These early lineage T cells also express CCR7, consistent with central memory T cells (TCM cells). Subsequently, with continued antigenic stimulation these polyfunctional T cells lose CCR7 expression and capacity to secrete TNF-α or IL-2, and finally produce only IFN-γ in the highly differentiated, terminal effector stage. Thus, according to this model, protective potential of a polyfunctional CD4+ T cell producing IFN-γ, TNF-α, and IL-2 may be associated with its degree of differentiation and simultaneous capacity for memory and for effector function.
The literature regarding the possible role of polyfunctional CD4+ T cells in mediating vaccine-induced protection for TB has primarily investigated those cells co-producing IFN-γ, TNF-α, and IL-2, hereafter, we will refer to as “polyfunctional CD4+ T cells.” There is substantial evidence derived from study of murine TB models and humans that all three of these cytokines are necessary for the control of Mtb infection.
Essential roles in host defense for IFN-γ, and for CD4+ T cells that produce IFN-γ, were initially defined in the murine TB model using IFN-γ-deficient mice (23, 24), CD4+ T cell deficient mice, and adoptive transfer of CD4+ T cells in the murine TB model [reviewed in Ref. (25)]. More recently, Green et al. showed that CD4+ T cells are important as a source of IFN-γ mediating protection from Mtb, by demonstrating that IFN-γ production from all other cellular sources than CD4+ T cells was insufficient in controlling chronic infection and maintaining survival and that CD4+ T cell derived IFN-γ promoted CD8+ T cell responses (26). Consistent with this, humans deficient in IL-12 receptor expression (27), IFN-γ receptor expression, or IFN-γ signaling (28) are more susceptible to mycobacterial disease. However, it is important to note that these deficiencies are not T cell-specific and thus the precise role of T cell-derived IFN-γ vs other sources of IFN-γ in humans remains unresolved. At least in the murine TB model, a mechanism by which IFN-γ may mediate its effector function is through activation of macrophages, which in turn inhibit Mtb growth via induction of iNOS and autophagy [reviewed in Ref. (29)].
TNF-α is also essential for TB host defense. Mice deficient in TNF receptor or TNF-α are highly susceptible to Mtb infection (30, 31). There are multiple cell types that produce TNF-α, including innate immune cells, epithelial cells, endothelial cells, and fibroblasts. Early control of Mtb infection in the mouse model, prior to the acquisition of adaptive immunity, is primarily mediated by TNF-α derived from macrophages (32). Conversely, mice deficient for TNF-α expression in T cells poorly control chronic infection (32). In humans, pharmacological blockade of TNF-α, used for treatment of rheumatologic disorders, increases susceptibility to mycobacterial disease (33, 34). As demonstrated in both the murine TB model and in humans, TNF-α promotes the formation of mature granulomas and like IFN-γ, also activates infected macrophages, which, at least in mice, contain infection via induction of iNOS and autophagy [reviewed in Ref. (29)].
IL-2 induces proliferation, promotes the survival of TCR-activated T cells [reviewed in Ref. (35)] and promotes the development of fully competent memory T cells during primary infection (36). Therefore, IL-2 is generally assumed to aid TB host defense through supporting the expansion and maintenance of the T cell response [reviewed in Ref. (35)]. IL-2 also has a role in T cell tolerance, through its role in maintaining CD4+CD25+ regulatory T cells. In support of this, IL-2 and IL-2R deficient mice develop early severe autoimmune disease [reviewed in Ref. (35)]. Consequently, these mice cannot be utilized to determine the role of IL-2 in TB host defense as has been done with IFN-γ and TNF-α. Nonetheless, in the murine TB model, induction of IL-2-producing CD4+ T cells is associated with vaccine-induced protection to Mtb and loss of IL-2-producing CD4+ T cells is associated with loss of protection [reviewed in Ref. (37)]. In humans, decreased proportions of polyfunctional CD4+ T cells in individuals with AFB smear positive TB compared to those with AFB smear negative TB, or in those with TB disease as compared to those with LTBI, was associated with decreases in proportions of total IL-2 producing T cells (13, 14).
BCG and novel TB vaccine candidates utilizing various antigens and vaccine platforms induce polyfunctional CD4+ T cells in murine, bovine, and non-human primate (NHP) TB models. These T cell populations possess attributes, which are critical for mediating vaccine-mediated immunity in that they can traffic to the lung and include memory T cell populations that persist in the vaccinated host.
As the only licensed TB vaccine and in light of its demonstrated record of partial efficacy, BCG is often used as a control vaccine in the mouse model of TB vaccination (Table 1). Several studies have shown that BCG induces polyfunctional mycobacteria-specific CD4+ T cells detected among lung (9, 38) and splenic (9, 39–44) lymphocyte populations (Table 1). Darrah et al. found that polyfunctional mycobacterial CD4+ T cells produced more cytokine on a per cell basis than do those producing two or one cytokines, similar to Leishmania-specific polyfunctional CD4+ T cells (9). In the murine TB model, these T cell responses constitute minor (38, 39, 42, 44), predominant (43), or major (9) subsets within the first 2–4 months after immunization. In addition, polyfunctional CD4+ T cells have been detected among CD44+ memory T cell populations as early as 3 weeks after vaccination (40) and persist as a major subset more than 40 weeks after vaccination (38). By contrast, though detecting polyfunctional CD4+ T cells as a predominant T cell population 2 and 8 months after BCG, Derrick et al. were unable to detect these cells 14 months after immunization, suggesting that this subset may not persist long-term (43). These differences in persistence of polyfunctional CD4+ T cells and wide phenotypic spectrum in cytokine-producing CD4+ T cells are not readily reconciled between studies. However, differences in the experimental protocols utilized, such as differences in BCG strain and/or delivery method, mouse strain and/or cell populations studied and the nature and dose of the Mtb challenge, may underlie the inconsistency in findings. Finally, BCG induces polyfunctional T cells in cattle (45) and rhesus macaque monkeys of Chinese origin (Macaca mulatta) (46). Maggioli et al. showed that BCG induced mycobacteria-specific polyfunctional CD4+ T cells as a major cytokine expressing subset in peripheral blood mononuclear cells (PBMC) obtained from calves 6 weeks after BCG and cultured with antigen for 2 weeks in vitro (45). These T cells expressed phenotypic markers consistent with memory T cells. Finally, White et al. showed that BCG delivered by the aerosol route induced PPD-specific polyfunctional CD4+ T cells as a major subset in both PBMC and bronchoalveolar lavage (BAL), fluid of Chinese rhesus macaque monkeys 8 weeks after BCG administration (46).
Similarly, recombinant BCG constructs (42, 44) induce polyfunctional CD4+ T cell responses in mice (Table 2). Yuan et al. compared BCG overexpressing Ag85B and HspX (rBCG:XB) to non-recombinant BCG and demonstrated that rBCG:XB elicited comparable PPD- and Ag85B-specific and stronger HspX-specific polyfunctional T cell responses 12 and 32 weeks after vaccination (44). Comparing a recombinant BCG expressing membrane-perforating listerolysin (with the rationale of promoting CD8+ T cell responses from cytosolic antigens) and deficient in urease C (ΔureC hly+, VPM1002), Tchilian et al. demonstrated low-frequency splenic PPD- and Ag85A-specific polyfunctional CD4+ T cells, comparable to those induced by BCG, 13 and 22 weeks after immunization (42). Maggioli et al. showed that vaccination with a cocktail of four distinct BCG deletion mutants (Δfdr8, ΔleuCD, Δpks16, ΔmmaA2, and ΔmetA) induced mycobacteria-specific polyfunctional CD4+ T cells in 2 weeks cultures of PBMC from bovine calves 6 weeks after vaccination. These responses were comparable to those induced by non-recombinant BCG (45).
Adenoviral vectors expressing Mtb proteins also elicit polyfunctional CD4+ T cells in murine and NHP models of TB (Table 2). Derrick et al. showed that a replication deficient adenoviral vector, Ad5, expressing ESAT-6 fused to the n-terminus of Ag85B (E6-85) induced BCG-specific polyfunctional CD4+ T cells, detected in spleen of mice 2 and 8 months after vaccination, but no longer detectable 14 months after vaccination (43). Forbes et al. used an Ad5 vector expressing Ag85A as a boost to BCG and compared intradermal (i.d.) to intranasal (i.n.) delivery of the adenovirus (39). Boosting i.d. but not i.n. induced Ag85A-specific polyfunctional CD4+ T cells, as a predominant subset in spleen 4 weeks after the adenoviral boost vaccination. By contrast, when lung T cells were examined, this adenoviral vector only induced Ag85A-specific polyfunctional CD4+ T cells when delivered i.n. but not i.d. Elevang et al. showed that an Ad5 vector expressing H4, a recombinant Ag85B/TB10.4 fusion protein (Ad-H4), induced Ag85A and TB10.4-specific polyfunctional CD4+ T cells, representing a predominant subset of CD44+ memory T cells in spleen 3 weeks following the vaccination and also when administered as a boost to recombinant H4 delivered in CAF01 (H4:CAF01) 1 week following this vaccination (40). Magalhaes et al. showed that an adenoviral vector, Ad35, expressing Ag85A, Ag85b, and TB10.4 (AERAS-402) delivered i.m. as a boost to either BCG or recombinant BCG expressing perfringolysin (AERAS-401), elicited transient Ag85A/B and TB10.4-specific polyfunctional CD4+ T cell responses in PBMC of rhesus macaque monkeys of Chinese origin (M. mulatta) 1 week after the first boost (47). Hokey et al. went on to show that AERAS-402 delivered via aerosol to Chinese rhesus macaques induced weakly detectable Ag85A/B-specific polyfunctional CD4+ T cells in PBMC 3 days after and in BAL 28 days after completion of immunization (48). Therefore, at least in the short-term, adenoviral vectors expressing Mtb proteins elicit polyfunctional CD4+ T cells when delivered with alone or as a boost to BCG in mice and NHPs.
Two additional viral vector systems have also been assessed in the mouse TB model (Table 2). A vesicular stomatitis virus (VSV) construct expressing Rv3615c, Mtb10.4, and Rv2660c elicited dual-cytokine-producing (IFN-γ+TNF-α+; TNF-α+IL-2+; and IFN-γ+IL-2+) but not triple-cytokine producing (IFN-γ+TNF-α+IL-2+) polyfunctional CD4+ T cell responses when delivered i.n. either alone or as a boost to BCG (49). By contrast, a modified vaccinia Ankara (MVA) expressing Ag85A (MVA85A), when delivered as a boost to BCG, elicited predominantly Ag85A-specific polyfunctional CD4+ T cells 3 and 12 weeks following MVA immunization (42). The capacity for MVA85A to elicit polyfunctional CD4+ T cell responses in humans has been more extensively evaluated and is discussed below.
Recombinant Mtb antigen subunit vaccines, typically comprising proteins formulated in adjuvant, have been extensively evaluated in the mouse TB model (Table 2). Several adjuvanted recombinant Mtb proteins or fusions of multiple Mtb proteins have been shown to elicit polyfunctional CD4+ T cells in mice, including recombinant Ag85B (50), and MT1721 (51) and fusion proteins comprised of ESAT-6 fused to the n-terminus of Ag85B (E6-85) (43), Ag85B/TB10.4 (H4) (40, 52), Ag85B/ESAT-6 (H1) (38, 41, 53), and gene products of Rv2608, Rv3619, Rv3620, and Rv1813 (ID93) (54). These recombinant protein vaccines have successfully utilized several different adjuvant formulations based upon immunostimulatory lipids (38, 40, 41, 43, 53, 54) or nucleotides (50–52) to elicit these T cell responses. In addition, adjuvanted recombinant protein vaccines induce polyfunctional CD4+ T cell responses when used alone (40, 41, 43, 50, 52–54) and when administered as a boost after BCG prime (38, 43) or a DNA vaccine prime (51). Moreover, one study examined antigen dose and found that a lower dose of H4:IC31 [recombinant H4 delivered in IC31 (cationic peptide and oligodeoxynucleotide), 0.5 μg] induced greater frequencies of polyfunctional CD4+ T cells than a higher dose (5 μg) (52). Finally, recombinant protein vaccines induce polyfunctional CD4+ T cells detected among CD44+ memory T cell populations (38, 40, 41) as early as 1 week following completed immunization (40) and persisted 56 weeks when recombinant H1 delivered in CAF01 (H1:CAF01) was used (41) and as long as 14 months when recombinant E6-85 delivered in DD/MPL (liposomal monophosphoryl lipid A, E6-85: DDA/MPL) was used to boost BCG (43). Therefore, polyfunctional CD4+ T cells are induced with several distinct adjuvanted recombinant Mtb protein vaccines, when used alone or as a boost to BCG or DNA vaccine, and are included among memory CD4+ T cell populations.
Recent studies in the mouse TB demonstrate the importance of tissue location for protective immune responses against M. tuberculosis and suggest that lung resident CD4+ T cells mediate control of Mtb infection better than CD4+ T cells that reside in the pulmonary vasculature but do not enter the parenchyma [reviewed in Ref. (37)]. Protective parenchymal CD4+ T cells express activation markers such as PD-1 and CD69, do not express the terminal differentiation marker KLRG1, and produce less IFN-γ than intravascular T cells. Therefore, the ability of TB vaccines to promote the accumulation of lung resident polyfunctional CD4+ T cells may be an additional important component of vaccine-induced protection by these cells.
Tuberculosis vaccine candidates can elicit polyfunctional CD4+ T cells in the lung (Table 3). Darrah et al. showed that BCG delivered i.m. induced PPD-specific polyfunctional CD4+ T cells, as a major subset, in the lung 4 months after vaccination (9). Lindenstrom et al. showed that BCG and BCG boosted with Ag85B-ESAT-6 fusion protein in CAF01 (H1:CAF01) induced PPD-specific polyfunctional CD4+ T cells, as a minor subset in lung 10 weeks after the completed immunization (38). By contrast, Forbes et al. showed that an Ag85A-expressing adenoviral vaccine-induced polyfunctional CD4+ T cells in the lung after i.n. but not i.d. administration of the vaccine (39). In NHP’s, polyfunctional CD4+ T cells are detected in BAL after aerosol delivery of BCG (46) or adenoviral vaccine, AERAS-402 (48).
Studies utilizing the mouse TB model have also investigated the effect of prior vaccine administration on the magnitude of the polyfunctional CD4+ T cell response in the lung following Mtb challenge (Table 3). Vaccination with recombinant ESAT-6 in CAF01 (rESAT-6:CAF01) (55) or Ag85B/ESAT-6/Rv2660c fusion protein in CAF01 (H56:CAF01) (56, 57), or VPM1002 (58), recombinant Ag85B/TB10.4 fusion protein in CAF01 (H4:CAF01), boosted with an Ad5 vector expressing recombinant H4 (Ad-H4) (40), or BCG boosted with Ag85B-ESAT-6 fusion protein in CAF01 (H1:CAF01) (38) all resulted in increased Mtb antigen-specific polyfunctional CD4+ T cell responses in lung relative to control mice 2 weeks (40, 55) up to 26 weeks (38) after Mtb challenge. In most of these studies both an increased polyfunctional CD4+ T cell response and vaccine-induced protection in the lung, as measured by decreased CFU in the lung relative to control mice, were observed at the same time point after infection. However, Desel et al. showed that 90 days after challenge, frequencies of polyfunctional T cells in the lung were comparable in VMP1002-immunized, BCG-immunized and control mice (58). Despite that, at this same time point, VMP1002-immunized mice controlled infection better than BCG-immunized mice and BCG-immunized mice controlled infection better than naïve mice. Also, Lindenstrom et al. showed that vaccination with BCG boosted with H1:CAF01 resulted in increased PPD-specific polyfunctional CD4+ T cell responses in lung relative to control mice and BCG-vaccinated mice, yet differences in lung CFU in BCG/H1:CAF01 vs BCG-vaccinated mice were not statistically significant (38). In another study, vaccination with recombinant Ag85B/ESAT-6/Rv2660c fusion protein (H56:CAF01) resulted in Ag85B-specific polyfunctional CD4+ T cells in the lungs, which were not detected in BCG-immunized or control mice, yet both BCG and H56-vaccinated mice demonstrated a similar reduction in lung CFU as compared to control mice at 6 and 12 weeks after challenge (56). In conclusion, in animal models, TB vaccines induce polyfunctional CD4+ T cells that are present in lung after immunization and in some, but not all studies, an increase in polyfunctional CD4+ T cells in the lung following Mtb challenge is temporally associated with vaccine-induced control of bacterial replication in the lung relative to control mice.
Whether or not vaccine-induced polyfunctional CD4+ T cells represent a correlate of protective immunity from Mtb infection has been addressed in several mouse studies investigating the correlation between the magnitude of the polyfunctional CD4+ T cell response present before Mtb challenge and vaccine-induced control of bacterial replication, the most common measure of vaccine protection against TB in the murine model (Table 2). The strongest correlative evidence for polyfunctional CD4+ T cells as a correlate of protective immunity comes from studies in which a correlation between control of bacterial replication and the magnitude of the CD4+ T cell response was established from experiments including multiple distinct vaccine candidates that elicit a range of protective responses. Conversely, the weakest correlative evidence comes from studies in which a single vaccine candidate both induces a polyfunctional CD4+ T cell response and demonstrates protection compared to unimmunized control mice. For example, BCG (9, 41), H1:CAF01 (38, 41), and ID93:GLA-SE (54), all induced mycobacteria-specific polyfunctional CD4+ T cell responses at the time of Mtb challenge, which were associated with protection as compared to naïve or control mice, who lack these T cell responses. Somewhat better correlative evidence comes from studies comparing two or more vaccine candidates to one another. Comparing rBCG:XB to BCG, Yuan et al. showed that rBCG:XB elicited stronger HspX-specific polyfunctional T cell responses than BCG, which was associated with greater protection than was observed in BCG-vaccinated mice (44). Ballester et al. showed that recombinant Ag85B delivered in CpG (Ag85B:CpG) with polypropylene sulfide nanoparticles (NP) delivered i.n. induced more polyfunctional Ag85B-specific CD4+ T cells in spleen pre-challenge than did Ag85B:CpG without NP and this correlated with better protection in the lung (50). Also, comparing priming alone with prime/boost strategies, Elevang et al. demonstrated that recombinant Ag85B/TB10.4 fusion protein in CAF01 (H4:CAF01), boosted with an Ad5 vector expressing recombinant H4 (Ad-H4) induced more Ag85A- and TB10.4-specific polyfunctional memory CD4+ T cells, pre-challenge than did either recombinant H4 or Ad-H4 alone, which in turn correlated with better protection in lung after challenge (40). In addition, comparing different dosages of recombinant antigen, Aagaard et al. showed that H4:IC31 induced stronger Ag85B- and TB10.4-specific polyfunctional CD4+ T cells in PBMC pre-challenge at a lower dose (0.5 μg) vs a higher dose (5.0 μg), and this correlated with better protection in the lung at the lower dose (52). Finally, the strongest degree of correlation was demonstrated using several different vaccine regimens inducing various levels of protection (43). Derrick et al. showed that both the frequency of polyfunctional CD4+ T cells and the iMFI for TNF-α and IFN-γ at the time of Mtb challenge correlated with vaccine-induced protection in the lung and spleen over a 14-month period. For example, the magnitude of BCG-specific CD4+ polyfunctional T cell responses at the time of challenge 8 months after immunization with BCG or BCG mixed with recombinant E6-85, were greater than those induced by a DNA construct expressing recombinant E6-85 and correlated with greater protection afforded by either of these vaccine BCG regimens than the DNA construct. Moreover, using Pearson correlation analysis of iMFI values at all the challenge time points, these investigators showed that IFN-γ iMFI values were highly correlated with protection in the lung and spleen. Yet, of note, these investigators only reported measurements of the polyfunctional CD4+ T cell response, while other immune parameters that may also be mediators of protection, such as antibody responses and CD8+ T cell responses, were not evaluated for correlation with control of bacterial replication.
By contrast, two other studies show either equivocal evidence for or evidence against a correlation between pre-challenge polyfunctional CD4+ T cells and vaccine-induced protection. For example, boosting BCG with an Ad5 vector expressing Ag85A, induced Ag85A-specific polyfunctional CD4+ T cells pre-challenge in the lung only when delivered i.n. but not i.d. and this correlated with improved protection in the lung in i.n. vaccinated mice (39). However, i.d. rather than i.n. delivery induced polyfunctional CD4+ T cells in the spleen, such that only lung, not splenic polyfunctional CD4+ T cells correlated with the degree of vaccine-induced protection. In another study, both BCG and recombinant BCG (VPM1002) required boosting with MVA85A to elicit PPD- and Ag85A-specific polyfunctional CD4+ T cells, with BCG boosted with MVA85A eliciting greater T cell responses than VPM1002 boosted with MVA85A (42). Yet VPM1002 provided better protection in lung compared to BCG and the MVA85A boost, which induced high levels of polyfunctional CD4+ T cells, did not augment protection obtained with either BCG or VPM1002. Differences in these two studies as compared to numerous studies that support a correlation between polyfunctional CD4+ T cells are difficult to resolve, but may simply reflect differences in the vaccination protocol, or how polyfunctional T cells or protection was measured. In addition, two studies showed a stronger correlation between dual IL-2 and TNF-α producing CD4+ T cells and vaccine-induced protection than with triple IL-2, TNF-α, and IFN-γ-producing CD4+ T cells (38, 41). Finally, Yuan et al. showed a stronger correlation between the magnitude of dual TNF-α and IFN-γ-producing CD4+ T cells and vaccine-induced protection than with triple IL-2, TNF-α, and IFN-γ-producing CD4+ T cells (44). In summary, studies of the role of polyfunctional CD4+ T cells in mediating vaccine-induced protection in the mouse TB vaccine model provide evidence that these T cell responses represent at best an imperfect correlate of protection. In addition, these data can neither confirm nor refute CD4+ polyfunctional T cells as a mechanistic correlate of protection—i.e., a causal relationship between the vaccine-induced polyfunctional CD4+ T cell responses and control of bacterial replication following challenge is uncertain and cannot be ruled in or ruled out based upon these studies.
Bacille Calmette Guerin and novel TB vaccine candidates utilizing various antigens and vaccine platforms induce polyfunctional CD4+ T cells in infants, older children and adults. These T cell populations include memory T cell populations that persist for months to years after vaccination.
Several studies have demonstrated that BCG induces CD4+ polyfunctional T cells in infants (Table 4). BCG induces mycobacteria-specific polyfunctional CD4+ T cells, in healthy infants from South Africa (59–62), Uganda (63), Australia (64, 65), and the UK (66). These T cell responses represented the predominant subset among cytokine-producing T cells (59, 60, 66) peaked between 6 and 10 weeks of age and persisted for at least 1 year (60, 66). Comparison to unimmunized infants demonstrated that the polyfunctional T cell response was elicited by BCG and not by exposure to environmental mycobacteria (60, 66). Studies of Australian infants (65), South African infants (60), and Ugandan infants (63) compared BCG immunization at birth to delayed immunization at 6–10 weeks. These studies in general demonstrated no differences in polyfunctional CD4+ T cell responses during the first few weeks following immunization. However, South African infants who received delayed BCG had higher polyfunctional CD4+ T cell responses at 1 year of age than did those receiving BCG at birth (60). Also, Ritz et al. showed that BCG (Denmark, SSI) and BCG (Japan, Tokyo-172) induced higher polyfunctional CD4+ T cell responses than did BCG (Russian, SL-222), which correlated with more severe local reactions to the vaccine (64). BCG at birth also induced polyfunctional CD4+ T cell responses in HIV-infected infants, though at decreased numbers relative to HIV-exposed/uninfected infants and HIV-unexposed infants (61). Finally, Loxton et al. compared the immunogenicity of BCG to recombinant BCG, VPM1002, in healthy South African newborns and demonstrated comparable frequencies of polyfunctional CD4+ T cell responses 6 weeks, 18 weeks and 6 months after vaccination (67). Thus, ample evidence demonstrates that BCG induces polyfunctional CD4+ T cells in human infants.
Fewer studies have evaluated polyfunctional CD4+ T cells in BCG-immunized adults (Table 5). Two small studies of adults with a remote history of BCG immunization demonstrated mycobacteria-specific polyfunctional CD4+ T cell responses in these individuals, which represented the predominant subset of cytokine-producing cells (9, 71) and displayed a memory phenotype (71). In a small study of BCG naïve, Mtb-uninfected adults immunized with BCG, BCG-specific polyfunctional CD4+ T cell responses were variably detected and when present, peaked 8 weeks following vaccination, demonstrated an effector phenotype and correlated with local inflammation at the vaccination site (72). These responses waned by 1 year after vaccination. Consistent with these results, no polyfunctional CD4+ T cell responses were observed in British adolescents 1 year after BCG vaccination (73). Finally, Spertini et al. compared the immunogenicity of BCG with MTBVAC, a live attenuated strain of Mtb, in a randomized double-blind Phase I trial of BCG naïve, Mtb-uninfected Swiss adults (74). Both BCG and MTBVAC induced MTBVAC- and BCG-specific polyfunctional CD4+ T cell responses that persisted up to 210 days after vaccination. Therefore, although the evidence is less abundant than for infants, live mycobacterial vaccines also induce polyfunctional CD4+ T cell responses in adults.
Several recombinant antigen TB vaccines have been studied in adults for their capacity to induce polyfunctional CD4+ T cell responses (Table 5). Recombinant Ag85B/TB10.4 fusion protein formulated with IC31 adjuvant (H4:IC31), induced H4-specific polyfunctional CD4+ T cells in previously BCG-vaccinated, Mtb-uninfected South African adults (75). While all doses induced these T cell responses, lower doses (5 or 15 μg) induced higher magnitudes of CD4+ T cells than higher doses (50 or 150 μg), which could be detected up to 182 days after vaccination. Recombinant Ag85B/ESAT-6/Rv2660c fusion protein formulated with IC31 (H56:IC31) also induced polyfunctional CD4+ T cells in both previously BCG-immunized, Mtb-uninfected and Mtb-infected South African adults (76). Frequencies of these polyfunctional CD4+ T cells were higher in Mtb-infected than uninfected individuals and comprised the predominant proportion of the cytokine-producing cells 70 days after vaccination. These cells persisted, expressing markers of central memory cells, up to 210 days after immunization. As in the H4:IC31 study, a lower dose of H56:IC31 resulted in higher proportions of polyfunctional CD4+ T cells while in recipients of a high 50 μg H56 dose, CD4+ T cells expressing only IFN-γ predominated. Recombinant Ag85B/ESAT-6 formulated in IC31 (H1:IC31) induced H1-specific polyfunctional CD4+ T cells in Mtb-uninfected and infected South African adolescents which persisted at least 70 days in Mtb-infected and 224 days in Mtb-uninfected individuals (77), and in HIV-infected Tanzanian adults, which peaked at 70 days and persisted for 182 days after vaccination (78). Finally, a recombinant Mtb32A/Mtb39A fusion protein formulated in AS02A (Mtb72F:AS02A) induced polyfunctional CD4+ T cells in Mtb-infected and previously BCG-immunized Swiss adults (79), a recombinant Mtb32A/Mtb39A modified to increase stability formulated in ASO1B (M72:ASO1B) or in AS02A (M72:AS02A) induced polyfunctional CD4+ T cells in BCG naïve, Mtb-uninfected Belgian adults (80), and M72 formulated in AS01E (M72: AS01E) induced polyfunctional CD4+ T cells in Mtb-infected and uninfected South African adults and adolescents (81, 82), HIV-infected and uninfected Indian adults (83), and HIV-infected Swiss adults on anti-retroviral therapy (84). In the latter study, polyfunctional CD4+ T cell populations also co-expressed CD40L and persisted from 7 months to 3 years after immunization. Thus, adjuvanted recombinant protein TB vaccine candidates are good inducers of CD4+ polyfunctional T cell responses in humans.
Adenoviral TB vaccine candidates have been studied in a few trials of infants and adults (Tables 4 and 5). In a Phase I double-blinded randomized placebo-controlled trial of BCG-immunized, Mtb and HIV-uninfected South African adults, an Ad35 expressing Ag85A, Ag85B, and TB10.4 (AERAS-402), induced polyfunctional CD4+ T cells that constituted the predominant CD4+ T cell subset 28 days after immunization, but this subset was not detectable 182 days after immunization (85). AERAS-402 also induced polyfunctional CD4+ T cells in BCG-immunized, HIV-infected South African adults, which peaked 42 days after the first vaccination (86). By contrast, in a double-blinded randomized trial of healthy BCG-immunized HIV-uninfected infants performed in South Africa, Kenya, and Mozambique, AERAS-402 induced detectable but low level CD4+ polyfunctional T cell responses, at frequencies lower than had been observed in BCG-vaccinated adults in the afore mentioned study (68). Finally, an Ad5 expressing Ag85A vaccine candidate induced polyfunctional CD4+ T cell responses in both BCG naïve and previously BCG-vaccinated, Mtb and HIV-uninfected Canadian adults (87). Polyfunctional CD4+ T cells comprised the predominant subset of cytokine-producing T cells and peaked at 2–4 weeks after vaccination. Therefore, adenoviral TB vaccines induce polyfunctional CD4+ T cell responses in humans that do not persist as long as those induced by protein subunit TB vaccines.
The capacity for MVA expressing A85A (MVA85A) to elicit polyfunctional CD4+ T cells, as a boost to previous BCG vaccination, has been extensively evaluated in several studies of infants, children, adolescents, and adults (Tables 4 and 5). MVA85A induced polyfunctional CD4+ T cell responses in previously BCG-vaccinated Mtb- and HIV-uninfected South African adults (88), Mtb- and HIV-uninfected British adults (89, 90), Mtb-infected HIV-uninfected South African adults (91), Mtb-infected HIV-uninfected British adults (92), and Mtb- and HIV-uninfected infants (3, 69), young children (69) and adolescents (69). In these studies, polyfunctional CD4+ T cells represented the predominant subset of cytokine-producing T cells and persisted at least up to 168 days after vaccination. Moreover, in a follow-up study of the South Africa cohorts (69, 88, 91), Tameris et al. demonstrated that MVA85A induced polyfunctional CD4+ T cell responses persisted 3–5 years after immunization (70). Of note, these T cell responses were of lower magnitude in infants (3) than observed previously in adults (89). Thus, MVA85A is a potent inducer of polyfunctional CD4+ T cells responses, which persist for 3–5 years after vaccination.
Understandably little is known about the correlation of polyfunctional CD4+ T cells and risk of TB, although two infant vaccine trials have evaluated the relationship of vaccine-induced polyfunctional CD4+ T cells and subsequent development of TB. Kagina et al. performed a subanalysis of a large trial evaluating the immunogenicity of BCG (Japanese) delivered i.d. vs s.c. in South African infants (62). Infants who developed TB over the 2-year follow-up period were compared to healthy, Mtb exposed, and healthy Mtb unexposed infants. There was no correlation between the magnitude of polyfunctional CD4+ T cells and subsequent development of TB during 2 years of follow-up. More recently, Tameris et al. conducted a double-blind Phase IIb trial in South African infants evaluating the immunogenicity and efficacy of MVA85A as a boost after BCG prime at birth, compared with a placebo (3). Although MVA85A-vaccinated infants developed polyfunctional Ag85A-specific CD4+ T cell responses, albeit at lower magnitudes than typically observed in adults, the MVA85A boost provided no additional efficacy against development of TB over BCG alone. By contrast, the majority of mouse TB studies evaluating polyfunctional CD4+ T cells and vaccine-induced protection have noted a correlative relationship. This discrepancy may reflect differences between the mouse TB vaccine model and vaccine-induced protection from human disease, publication bias, or, in the case of the MVA85A study, possibly induction of inadequate levels of polyfunctional CD4+ T cells required for protection. Regarding the latter, Fletcher et al. found that immune activation at the time of MVA85A or placebo vaccination was associated with risk of developing TB, which in turn may be associated with poor BCG vaccine take, i.e., lower frequencies of BCG-specific IFN-γ-expressing cells (93). These data suggest that additional factors over and above the functionality of T cells play a role in protective immunity.
Discrepancies between some mouse TB vaccine studies and others, and between mouse and human TB vaccine studies, could reflect that a correlate of protection may be specific for each particular vaccine platform or may vary for different TB antigens. In this regard, standardization of vaccine study protocols and harmonization between animal and human studies could improve the ability to discern correlates of protection. In addition, discrepant results among animal model and human studies of polyfunctional CD4+ T cells, in general, could reflect that frequencies of polyfunctional CD4+ T cells correlate with a more accurate, yet-to-be determined correlate of protection. In this regard, mouse studies suggest that total IL-2-producing CD4+ T cells may be the best correlate of protection against Mtb infection (38, 41) and in humans, IL-2-producing CD4+ T cells are associated with successful containment of Mtb infection in persons with LTBI (13, 14). Conversely, loss of IL-2 expression by IFN-γ and/or TNF-expressing Th1 cells is likely indicative of greater T cell differentiation, typically observed in scenarios of high bacterial loads, such as TB disease (13, 14), or following delivery of high doses of vaccine antigens (76). This is consistent with the linear differentiation model of CD4+ T cells derived primarily from acute and chronic viral infections (22). In this model, IL-2-production reflects central memory T cells in early stages of differentiation, with capacity for long-lived memory and proliferation. Loss of IL-2 expression thus reflects more advanced differentiation of CD4+ T cells, such as effector memory or terminal effector cells. This is further supported by a recent study of CD4+ T cell responses to Ag85B and ESAT-6 in mice and humans, which showed that Ag85B-specific T cells were significantly less differentiated than ESAT-6-specific T cells, which correlated with lack of persistent Ag85B antigen expression and persistent ESAT-6 expression by the bacterium during chronic infection in the mouse model (94). Distinct attributes observed between these T cell subsets were higher proportions of polyfunctional and lower proportions of IL-2-expressing ESAT-6-specific T cells, when compared with Ag85B-specific T cells.
BCG, as well as novel TB vaccine candidates, induce polyfunctional CD4+ T cells in both animal models and humans. These cells possess important functional attributes that may potentially play a role in vaccine-mediated protection including long-lived memory function, persistence in the vaccinated host, and at least in animal models, ability to traffic to and persist in the lung. In the mouse model, the magnitude of vaccine-induced polyfunctional CD4+ T cells often correlates with vaccine-induced protection, making polyfunctional T cells a good candidate for a mechanistic correlate of protection. However, definitive evidence that it is in fact the co-expression of IFN-γ, TNF-α, and IL-2 by these T cells, rather than another functional or phenotypic attribute, that mediates host defense against Mtb remains lacking and would require sophisticated knock-down or adoptive transfer experiments. Some mouse studies, and of note, two human infant studies do not support polyfunctional CD4+ T cells as correlate of protection. Moreover, because these studies focus mainly and often exclusively on defining polyfunctional CD4+ T cells, it is certainly possible that a stronger immunologic correlate was present and not measured. We conclude that induction of polyfunctional T cells is certainly not sufficient and may not even be necessary to mediate protective immunity against Mtb and speculate that the production of multiple pro-inflammatory cytokines by T cells may reflect properties of T cells that may not necessarily be required for protection. Other functional attributes, such as additional effector functions, the differentiation state, tissue homing potential, long-term survival capacity of the T cell, or their ability to recognize the Mtb-infected cell may be equally or more important to promote protection. It is also possible that the induction of polyfunctional CD4+ T cells may be dependent upon the particular antigen or adjuvant utilized. Thus, a correlate of protection for TB vaccine development remains elusive. We propose that studies of protective immunity against Mtb should reach well beyond the measurement of IFNγ, TNF, and IL-2 by investigating other functions, phenotypes and correlates of immunity. Further, in light of the lack of direct causal association between T cell polyfunctionality and protective immunity, care should be taken not to bias studies in favor of the hypothesis that polyfunctional cells are indeed the mediators of protection. The definition of correlates of protection may benefit from standardization of animal TB vaccine studies and harmonization of these protocols with human trials. Finally, future studies should address the full spectrum of CD4+ T cell flavors and colors within the context of more complete immunological signatures of protection, including for example, additional phenotypic and functional attributes of CD4+ T cells, such as IL-17 production, and other major cells, such as classically restricted CD8+ T cells, donor unrestricted T cells, and NK cells, as well as B cells.
DAL performed the literature search and designed the tables. DAL and TS outlined and wrote the manuscript. DAL, DML, and TS discussed and edited the manuscript.
The authors declare that the research was conducted in the absence of any commercial or financial relationships that could be construed as a potential conflict of interest.
This work was supported by funding from the Bill and Melinda Gates Foundation to DAL and DML.
1. World Health Organization. Global Tuberculosis Report 2016. Geneva, Switzerland: World Health Organization (2016).
2. World Health Organization. The End TB Strategy: Global Strategy and Targets for Tuberculosis Prevention, Care and Control after 2015. Geneva, Switzerland: World Health Organization (2015).
3. Tameris MD, Hatherill M, Landry BS, Scriba TJ, Snowden MA, Lockhart S, et al. Safety and efficacy of MVA85A, a new tuberculosis vaccine, in infants previously vaccinated with BCG: a randomised, placebo-controlled phase 2b trial. Lancet (2013) 381(9871):1021–8. doi:10.1016/S0140-6736(13)60177-4
4. Bhatt K, Verma S, Ellner JJ, Salgame P. Quest for correlates of protection against tuberculosis. Clin Vaccine Immunol (2015) 22(3):258–66. doi:10.1128/CVI.00721-14
5. Food and Drug Administration. Guidance for Industry: For the Evaluation of Combination Vaccines for Preventable Diseases: Production, Testing and Clinical Studies. Silver Springs: US Department of Health and Human Services, Food and Drug Administration, Center for Biologics Evaluation and Research (1997).
6. Plotkin SA, Gilbert PB. Nomenclature for immune correlates of protection after vaccination. Clin Infect Dis (2012) 54(11):1615–7. doi:10.1093/cid/cis238
7. De Rosa SC, Lu FX, Yu J, Perfetto SP, Falloon J, Moser S, et al. Vaccination in humans generates broad T cell cytokine responses. J Immunol (2004) 173(9):5372–80. doi:10.4049/jimmunol.173.9.5372
8. Bansal A, Jackson B, West K, Wang S, Lu S, Kennedy JS, et al. Multifunctional T-cell characteristics induced by a polyvalent DNA prime/protein boost human immunodeficiency virus type 1 vaccine regimen given to healthy adults are dependent on the route and dose of administration. J Virol (2008) 82(13):6458–69. doi:10.1128/JVI.00068-08
9. Darrah PA, Patel DT, De Luca PM, Lindsay RW, Davey DF, Flynn BJ, et al. Multifunctional TH1 cells define a correlate of vaccine-mediated protection against Leishmania major. Nat Med (2007) 13(7):843–50. doi:10.1038/nm1592
10. Caccamo N, Dieli F. Are polyfunctional cells protective in M. tuberculosis infection? In: Cardona PJ, editor. Understanding Tuberculosis—Analyzing the Origin of Mycobacterium tuberculosis Pathogenicity. InTech (2012).
11. Duvall MG, Precopio ML, Ambrozak DA, Jaye A, McMichael AJ, Whittle HC, et al. Polyfunctional T cell responses are a hallmark of HIV-2 infection. Eur J Immunol (2008) 38(2):350–63. doi:10.1002/eji.200737768
12. Kannanganat S, Kapogiannis BG, Ibegbu C, Chennareddi L, Goepfert P, Robinson HL, et al. Human immunodeficiency virus type 1 controllers but not noncontrollers maintain CD4 T cells coexpressing three cytokines. J Virol (2007) 81(21):12071–6. doi:10.1128/JVI.01261-07
13. Day CL, Abrahams DA, Lerumo L, Janse van Rensburg E, Stone L, O’Rie T, et al. Functional capacity of Mycobacterium tuberculosis-specific T cell responses in humans is associated with mycobacterial load. J Immunol (2011) 187(5):2222–32. doi:10.4049/jimmunol.1101122
14. Harari A, Rozot V, Bellutti Enders F, Perreau M, Stalder JM, Nicod LP, et al. Dominant TNF-alpha+ Mycobacterium tuberculosis-specific CD4+ T cell responses discriminate between latent infection and active disease. Nat Med (2011) 17(3):372–6. doi:10.1038/nm.2299
15. Hutchinson P, Barkham TM, Tang W, Kemeny DM, Chee CB, Wang YT. Measurement of phenotype and absolute number of circulating heparin-binding hemagglutinin, ESAT-6 and CFP-10, and purified protein derivative antigen-specific CD4 T cells can discriminate active from latent tuberculosis infection. Clin Vaccine Immunol (2015) 22(2):200–12. doi:10.1128/CVI.00607-14
16. Caccamo N, Guggino G, Joosten SA, Gelsomino G, Di Carlo P, Titone L, et al. Multifunctional CD4+ T cells correlate with active Mycobacterium tuberculosis infection. Eur J Immunol (2010) 40(8):2211–20. doi:10.1002/eji.201040455
17. Qiu Z, Zhang M, Zhu Y, Zheng F, Lu P, Liu H, et al. Multifunctional CD4 T cell responses in patients with active tuberculosis. Sci Rep (2012) 2:216. doi:10.1038/srep00216
18. Sutherland JS, Adetifa IM, Hill PC, Adegbola RA, Ota MO. Pattern and diversity of cytokine production differentiates between Mycobacterium tuberculosis infection and disease. Eur J Immunol (2009) 39(3):723–9. doi:10.1002/eji.200838693
19. Bogdan C, Moll H, Solbach W, Rollinghoff M. Tumor necrosis factor-alpha in combination with interferon-gamma, but not with interleukin 4 activates murine macrophages for elimination of Leishmania major amastigotes. Eur J Immunol (1990) 20(5):1131–5. doi:10.1002/eji.1830200528
20. Liew FY, Li Y, Millott S. Tumor necrosis factor-alpha synergizes with IFN-gamma in mediating killing of Leishmania major through the induction of nitric oxide. J Immunol (1990) 145(12):4306–10.
21. Chan J, Xing Y, Magliozzo RS, Bloom BR. Killing of virulent Mycobacterium tuberculosis by reactive nitrogen intermediates produced by activated murine macrophages. J Exp Med (1992) 175(4):1111–22. doi:10.1084/jem.175.4.1111
22. Seder RA, Darrah PA, Roederer M. T-cell quality in memory and protection: implications for vaccine design. Nat Rev Immunol (2008) 8(4):247–58. doi:10.1038/nri2274
23. Cooper AM, Dalton DK, Stewart TA, Griffin JP, Russell DG, Orme IM. Disseminated tuberculosis in interferon gamma gene-disrupted mice. J Exp Med (1993) 178(6):2243–7. doi:10.1084/jem.178.6.2243
24. Flynn JL, Chan J, Triebold KJ, Dalton DK, Stewart TA, Bloom BR. An essential role for interferon gamma in resistance to Mycobacterium tuberculosis infection. J Exp Med (1993) 178(6):2249–54. doi:10.1084/jem.178.6.2249
25. Winslow GM, Cooper A, Reiley W, Chatterjee M, Woodland DL. Early T-cell responses in tuberculosis immunity. Immunol Rev (2008) 225:284–99. doi:10.1111/j.1600-065X.2008.00693.x
26. Green AM, Difazio R, Flynn JL. IFN-gamma from CD4 T cells is essential for host survival and enhances CD8 T cell function during Mycobacterium tuberculosis infection. J Immunol (2013) 190(1):270–7. doi:10.4049/jimmunol.1200061
27. de Jong R, Altare F, Haagen IA, Elferink DG, Boer T, van Breda Vriesman PJ, et al. Severe mycobacterial and Salmonella infections in interleukin-12 receptor-deficient patients. Science (1998) 280(5368):1435–8. doi:10.1126/science.280.5368.1435
28. Haverkamp MH, van Dissel JT, Holland SM. Human host genetic factors in nontuberculous mycobacterial infection: lessons from single gene disorders affecting innate and adaptive immunity and lessons from molecular defects in interferon-gamma-dependent signaling. Microbes Infect (2006) 8(4):1157–66. doi:10.1016/j.micinf.2005.10.029
29. Lewinsohn DA, Gold MC, Lewinsohn DM. Views of immunology: effector T cells. Immunol Rev (2011) 240(1):25–39. doi:10.1111/j.1600-065X.2010.00997.x
30. Flynn JL, Goldstein MM, Chan J, Triebold KJ, Pfeffer K, Lowenstein CJ, et al. Tumor necrosis factor-alpha is required in the protective immune response against Mycobacterium tuberculosis in mice. Immunity (1995) 2(6):561–72. doi:10.1016/1074-7613(95)90001-2
31. Bean AG, Roach DR, Briscoe H, France MP, Korner H, Sedgwick JD, et al. Structural deficiencies in granuloma formation in TNF gene-targeted mice underlie the heightened susceptibility to aerosol Mycobacterium tuberculosis infection, which is not compensated for by lymphotoxin. J Immunol (1999) 162(6):3504–11.
32. Allie N, Grivennikov SI, Keeton R, Hsu NJ, Bourigault ML, Court N, et al. Prominent role for T cell-derived tumour necrosis factor for sustained control of Mycobacterium tuberculosis infection. Sci Rep (2013) 3:1809. doi:10.1038/srep01809
33. Keane J, Gershon S, Wise RP, Mirabile-Levens E, Kasznica J, Schwieterman WD, et al. Tuberculosis associated with infliximab, a tumor necrosis factor alpha-neutralizing agent. N Engl J Med (2001) 345(15):1098–104. doi:10.1056/NEJMoa011110
34. Harris J, Keane J. How tumour necrosis factor blockers interfere with tuberculosis immunity. Clin Exp Immunol (2010) 161(1):1–9. doi:10.1111/j.1365-2249.2010.04146.x
35. Waldmann TA, Dubois S, Tagaya Y. Contrasting roles of IL-2 and IL-15 in the life and death of lymphocytes: implications for immunotherapy. Immunity (2001) 14(2):105–10. doi:10.1016/S1074-7613(09)00091-0
36. Williams MA, Tyznik AJ, Bevan MJ. Interleukin-2 signals during priming are required for secondary expansion of CD8+ memory T cells. Nature (2006) 441(7095):890–3. doi:10.1038/nature04790
37. Sakai S, Mayer-Barber KD, Barber DL. Defining features of protective CD4 T cell responses to Mycobacterium tuberculosis. Curr Opin Immunol (2014) 29:137–42. doi:10.1016/j.coi.2014.06.003
38. Lindenstrom T, Knudsen NP, Agger EM, Andersen P. Control of chronic Mycobacterium tuberculosis infection by CD4 KLRG1- IL-2-secreting central memory cells. J Immunol (2013) 190(12):6311–9. doi:10.4049/jimmunol.1300248
39. Forbes EK, Sander C, Ronan EO, McShane H, Hill AV, Beverley PC, et al. Multifunctional, high-level cytokine-producing Th1 cells in the lung, but not spleen, correlate with protection against Mycobacterium tuberculosis aerosol challenge in mice. J Immunol (2008) 181(7):4955–64. doi:10.4049/jimmunol.181.7.4955
40. Elvang T, Christensen JP, Billeskov R, Thi Kim Thanh Hoang T, Holst P, Thomsen AR, et al. CD4 and CD8 T cell responses to the M. tuberculosis Ag85B-TB10.4 promoted by adjuvanted subunit, adenovector or heterologous prime boost vaccination. PLoS One (2009) 4(4):e5139. doi:10.1371/journal.pone.0005139
41. Lindenstrom T, Agger EM, Korsholm KS, Darrah PA, Aagaard C, Seder RA, et al. Tuberculosis subunit vaccination provides long-term protective immunity characterized by multifunctional CD4 memory T cells. J Immunol (2009) 182(12):8047–55. doi:10.4049/jimmunol.0801592
42. Tchilian EZ, Desel C, Forbes EK, Bandermann S, Sander CR, Hill AV, et al. Immunogenicity and protective efficacy of prime-boost regimens with recombinant (delta)ureC hly+ Mycobacterium bovis BCG and modified vaccinia virus ankara expressing M. tuberculosis antigen 85A against murine tuberculosis. Infect Immun (2009) 77(2):622–31. doi:10.1128/IAI.00685-08
43. Derrick SC, Yabe IM, Yang A, Morris SL. Vaccine-induced anti-tuberculosis protective immunity in mice correlates with the magnitude and quality of multifunctional CD4 T cells. Vaccine (2011) 29(16):2902–9. doi:10.1016/j.vaccine.2011.02.010
44. Yuan X, Teng X, Jing Y, Ma J, Tian M, Yu Q, et al. A live attenuated BCG vaccine overexpressing multistage antigens Ag85B and HspX provides superior protection against Mycobacterium tuberculosis infection. Appl Microbiol Biotechnol (2015) 99(24):10587–95. doi:10.1007/s00253-015-6962-x
45. Maggioli MF, Palmer MV, Thacker TC, Vordermeier HM, McGill JL, Whelan AO, et al. Increased TNF-alpha/IFN-gamma/IL-2 and decreased TNF-alpha/IFN-gamma production by central memory T cells are associated with protective responses against bovine tuberculosis following BCG vaccination. Front Immunol (2016) 7:421. doi:10.3389/fimmu.2016.00421
46. White AD, Sarfas C, West K, Sibley LS, Wareham AS, Clark S, et al. Evaluation of the immunogenicity of Mycobacterium bovis BCG delivered by aerosol to the lungs of macaques. Clin Vaccine Immunol (2015) 22(9):992–1003. doi:10.1128/CVI.00289-15
47. Magalhaes I, Sizemore DR, Ahmed RK, Mueller S, Wehlin L, Scanga C, et al. rBCG induces strong antigen-specific T cell responses in rhesus macaques in a prime-boost setting with an adenovirus 35 tuberculosis vaccine vector. PLoS One (2008) 3(11):e3790. doi:10.1371/journal.pone.0003790
48. Hokey DA, Wachholder R, Darrah PA, Bolton DL, Barouch DH, Hill K, et al. A nonhuman primate toxicology and immunogenicity study evaluating aerosol delivery of AERAS-402/Ad35 vaccine: evidence for transient T cell responses in peripheral blood and robust sustained responses in the lungs. Hum Vaccin Immunother (2014) 10(8):2199–210. doi:10.4161/hv.29108
49. Zhang M, Dong C, Xiong S. Heterologous boosting with recombinant VSV-846 in BCG-primed mice confers improved protection against Mycobacterium infection. Hum Vaccin Immunother (2017) 13(4):816–22. doi:10.1080/21645515.2016.1261229
50. Ballester M, Nembrini C, Dhar N, de Titta A, de Piano C, Pasquier M, et al. Nanoparticle conjugation and pulmonary delivery enhance the protective efficacy of Ag85B and CpG against tuberculosis. Vaccine (2011) 29(40):6959–66. doi:10.1016/j.vaccine.2011.07.039
51. Cayabyab MJ, Kashino SS, Campos-Neto A. Robust immune response elicited by a novel and unique Mycobacterium tuberculosis protein using an optimized DNA/protein heterologous prime/boost protocol. Immunology (2012) 135(3):216–25. doi:10.1111/j.1365-2567.2011.03525.x
52. Aagaard C, Hoang TT, Izzo A, Billeskov R, Troudt J, Arnett K, et al. Protection and polyfunctional T cells induced by Ag85B-TB10.4/IC31 against Mycobacterium tuberculosis is highly dependent on the antigen dose. PLoS One (2009) 4(6):e5930. doi:10.1371/journal.pone.0005930
53. Andersen CA, Rosenkrands I, Olsen AW, Nordly P, Christensen D, Lang R, et al. Novel generation mycobacterial adjuvant based on liposome-encapsulated monomycoloyl glycerol from Mycobacterium bovis bacillus Calmette-Guerin. J Immunol (2009) 183(4):2294–302. doi:10.4049/jimmunol.0804091
54. Bertholet S, Ireton GC, Ordway DJ, Windish HP, Pine SO, Kahn M, et al. A defined tuberculosis vaccine candidate boosts BCG and protects against multidrug-resistant Mycobacterium tuberculosis. Sci Transl Med (2010) 2(53):53ra74. doi:10.1126/scitranslmed.3001094
55. Aagaard CS, Hoang TT, Vingsbo-Lundberg C, Dietrich J, Andersen P. Quality and vaccine efficacy of CD4+ T cell responses directed to dominant and subdominant epitopes in ESAT-6 from Mycobacterium tuberculosis. J Immunol (2009) 183(4):2659–68. doi:10.4049/jimmunol.0900947
56. Aagaard C, Hoang T, Dietrich J, Cardona PJ, Izzo A, Dolganov G, et al. A multistage tuberculosis vaccine that confers efficient protection before and after exposure. Nat Med (2011) 17(2):189–94. doi:10.1038/nm.2285
57. Woodworth JS, Cohen SB, Moguche AO, Plumlee CR, Agger EM, Urdahl KB, et al. Subunit vaccine H56/CAF01 induces a population of circulating CD4 T cells that traffic into the Mycobacterium tuberculosis-infected lung. Mucosal Immunol (2017) 10(2):555–64. doi:10.1038/mi.2016.70
58. Desel C, Dorhoi A, Bandermann S, Grode L, Eisele B, Kaufmann SH. Recombinant BCG DeltaureC hly+ induces superior protection over parental BCG by stimulating a balanced combination of type 1 and type 17 cytokine responses. J Infect Dis (2011) 204(10):1573–84. doi:10.1093/infdis/jir592
59. Soares AP, Scriba TJ, Joseph S, Harbacheuski R, Murray RA, Gelderbloem SJ, et al. Bacillus Calmette-Guerin vaccination of human newborns induces T cells with complex cytokine and phenotypic profiles. J Immunol (2008) 180(5):3569–77. doi:10.4049/jimmunol.180.5.3569
60. Kagina BM, Abel B, Bowmaker M, Scriba TJ, Gelderbloem S, Smit E, et al. Delaying BCG vaccination from birth to 10 weeks of age may result in an enhanced memory CD4 T cell response. Vaccine (2009) 27(40):5488–95. doi:10.1016/j.vaccine.2009.06.103
61. Mansoor N, Scriba TJ, de Kock M, Tameris M, Abel B, Keyser A, et al. HIV-1 infection in infants severely impairs the immune response induced by Bacille Calmette-Guerin vaccine. J Infect Dis (2009) 199(7):982–90. doi:10.1086/597304
62. Kagina BM, Abel B, Scriba TJ, Hughes EJ, Keyser A, Soares A, et al. Specific T cell frequency and cytokine expression profile do not correlate with protection against tuberculosis after bacillus Calmette-Guerin vaccination of newborns. Am J Respir Crit Care Med (2010) 182(8):1073–9. doi:10.1164/rccm.201003-0334OC
63. Lutwama F, Kagina BM, Wajja A, Waiswa F, Mansoor N, Kirimunda S, et al. Distinct T-cell responses when BCG vaccination is delayed from birth to 6 weeks of age in Ugandan infants. J Infect Dis (2014) 209(6):887–97. doi:10.1093/infdis/jit570
64. Ritz N, Dutta B, Donath S, Casalaz D, Connell TG, Tebruegge M, et al. The influence of Bacille Calmette-Guerin vaccine strain on the immune response against tuberculosis: a randomized trial. Am J Respir Crit Care Med (2012) 185(2):213–22. doi:10.1164/rccm.201104-0714OC
65. Ritz N, Casalaz D, Donath S, Tebruegge M, Dutta B, Connell TG, et al. Comparable CD4 and CD8 T cell responses and cytokine release after at-birth and delayed BCG immunisation in infants born in Australia. Vaccine (2016) 34(35):4132–9. doi:10.1016/j.vaccine.2016.06.077
66. Smith SG, Zelmer A, Blitz R, Fletcher HA, Dockrell HM. Polyfunctional CD4 T-cells correlate with in vitro mycobacterial growth inhibition following Mycobacterium bovis BCG-vaccination of infants. Vaccine (2016) 34(44):5298–305. doi:10.1016/j.vaccine.2016.09.002
67. Loxton AG, Knaul JK, Grode L, Gutschmidt A, Meller C, Eisele B, et al. Safety and immunogenicity of the recombinant Mycobacterium bovis BCG vaccine VPM1002 in HIV-unexposed newborn infants in South Africa. Clin Vaccine Immunol (2017) 24(2):e439–416. doi:10.1128/CVI.00439-16
68. Tameris M, Hokey DA, Nduba V, Sacarlal J, Laher F, Kiringa G, et al. A double-blind, randomised, placebo-controlled, dose-finding trial of the novel tuberculosis vaccine AERAS-402, an adenovirus-vectored fusion protein, in healthy, BCG-vaccinated infants. Vaccine (2015) 33(25):2944–54. doi:10.1016/j.vaccine.2015.03.070
69. Scriba TJ, Tameris M, Mansoor N, Smit E, van der Merwe L, Mauff K, et al. Dose-finding study of the novel tuberculosis vaccine, MVA85A, in healthy BCG-vaccinated infants. J Infect Dis (2011) 203(12):1832–43. doi:10.1093/infdis/jir195
70. Tameris M, Geldenhuys H, Luabeya AK, Smit E, Hughes JE, Vermaak S, et al. The candidate TB vaccine, MVA85A, induces highly durable Th1 responses. PLoS One (2014) 9(2):e87340. doi:10.1371/journal.pone.0087340
71. Adekambi T, Ibegbu CC, Kalokhe AS, Yu T, Ray SM, Rengarajan J. Distinct effector memory CD4+ T cell signatures in latent Mycobacterium tuberculosis infection, BCG vaccination and clinically resolved tuberculosis. PLoS One (2012) 7(4):e36046. doi:10.1371/journal.pone.0036046
72. Boer MC, Prins C, van Meijgaarden KE, van Dissel JT, Ottenhoff TH, Joosten SA. Mycobacterium bovis BCG vaccination induces divergent proinflammatory or regulatory T cell responses in adults. Clin Vaccine Immunol (2015) 22(7):778–88. doi:10.1128/CVI.00162-15
73. Smith SG, Lalor MK, Gorak-Stolinska P, Blitz R, Beveridge NE, Worth A, et al. Mycobacterium tuberculosis PPD-induced immune biomarkers measurable in vitro following BCG vaccination of UK adolescents by multiplex bead array and intracellular cytokine staining. BMC Immunol (2010) 11:35. doi:10.1186/1471-2172-11-35
74. Spertini F, Audran R, Chakour R, Karoui O, Steiner-Monard V, Thierry AC, et al. Safety of human immunisation with a live-attenuated Mycobacterium tuberculosis vaccine: a randomised, double-blind, controlled phase I trial. Lancet Respir Med (2015) 3(12):953–62. doi:10.1016/S2213-2600(15)00435-X
75. Geldenhuys H, Mearns H, Miles DJ, Tameris M, Hokey D, Shi Z, et al. The tuberculosis vaccine H4:IC31 is safe and induces a persistent polyfunctional CD4 T cell response in South African adults: a randomized controlled trial. Vaccine (2015) 33(30):3592–9. doi:10.1016/j.vaccine.2015.05.036
76. Luabeya AK, Kagina BM, Tameris MD, Geldenhuys H, Hoff ST, Shi Z, et al. First-in-human trial of the post-exposure tuberculosis vaccine H56:IC31 in Mycobacterium tuberculosis infected and non-infected healthy adults. Vaccine (2015) 33(33):4130–40. doi:10.1016/j.vaccine.2015.06.051
77. Mearns H, Geldenhuys HD, Kagina BM, Musvosvi M, Little F, Ratangee F, et al. H1:IC31 vaccination is safe and induces long-lived TNF-alpha+IL-2+CD4 T cell responses in M. tuberculosis infected and uninfected adolescents: a randomized trial. Vaccine (2017) 35(1):132–41. doi:10.1016/j.vaccine.2016.11.023
78. Reither K, Katsoulis L, Beattie T, Gardiner N, Lenz N, Said K, et al. Safety and immunogenicity of H1/IC31(R), an adjuvanted TB subunit vaccine, in HIV-infected adults with CD4+ lymphocyte counts greater than 350 cells/mm3: a phase II, multi-centre, double-blind, randomized, placebo-controlled trial. PLoS One (2014) 9(12):e114602. doi:10.1371/journal.pone.0114602
79. Spertini F, Audran R, Lurati F, Ofori-Anyinam O, Zysset F, Vandepapeliere P, et al. The candidate tuberculosis vaccine Mtb72F/AS02 in PPD positive adults: a randomized controlled phase I/II study. Tuberculosis (Edinb) (2013) 93(2):179–88. doi:10.1016/j.tube.2012.10.011
80. Leroux-Roels I, Forgus S, De Boever F, Clement F, Demoitie MA, Mettens P, et al. Improved CD4+ T cell responses to Mycobacterium tuberculosis in PPD-negative adults by M72/AS01 as compared to the M72/AS02 and Mtb72F/AS02 tuberculosis candidate vaccine formulations: a randomized trial. Vaccine (2013) 31(17):2196–206. doi:10.1016/j.vaccine.2012.05.035
81. Day CL, Tameris M, Mansoor N, van Rooyen M, de Kock M, Geldenhuys H, et al. Induction and regulation of T-cell immunity by the novel tuberculosis vaccine M72/AS01 in South African adults. Am J Respir Crit Care Med (2013) 188(4):492–502. doi:10.1164/rccm.201208-1385OC
82. Penn-Nicholson A, Geldenhuys H, Burny W, van der Most R, Day CL, Jongert E, et al. Safety and immunogenicity of candidate vaccine M72/AS01E in adolescents in a TB endemic setting. Vaccine (2015) 33(32):4025–34. doi:10.1016/j.vaccine.2015.05.088
83. Kumarasamy N, Poongulali S, Bollaerts A, Moris P, Beulah FE, Ayuk LN, et al. A randomized, controlled safety, and immunogenicity trial of the M72/AS01 candidate tuberculosis vaccine in HIV-positive Indian adults. Medicine (Baltimore) (2016) 95(3):e2459. doi:10.1097/MD.0000000000002459
84. Thacher EG, Cavassini M, Audran R, Thierry AC, Bollaerts A, Cohen J, et al. Safety and immunogenicity of the M72/AS01 candidate tuberculosis vaccine in HIV-infected adults on combination antiretroviral therapy: a phase I/II, randomized trial. AIDS (2014) 28(12):1769–81. doi:10.1097/QAD.0000000000000343
85. Abel B, Tameris M, Mansoor N, Gelderbloem S, Hughes J, Abrahams D, et al. The novel tuberculosis vaccine, AERAS-402, induces robust and polyfunctional CD4+ and CD8+ T cells in adults. Am J Respir Crit Care Med (2010) 181(12):1407–17. doi:10.1164/rccm.200910-1484OC
86. Churchyard GJ, Snowden MA, Hokey D, Dheenadhayalan V, McClain JB, Douoguih M, et al. The safety and immunogenicity of an adenovirus type 35-vectored TB vaccine in HIV-infected, BCG-vaccinated adults with CD4+ T cell counts >350 cells/mm(3). Vaccine (2015) 33(15):1890–6. doi:10.1016/j.vaccine.2015.02.004
87. Smaill F, Jeyanathan M, Smieja M, Medina MF, Thanthrige-Don N, Zganiacz A, et al. A human type 5 adenovirus-based tuberculosis vaccine induces robust T cell responses in humans despite preexisting anti-adenovirus immunity. Sci Transl Med (2013) 5(205):205ra134. doi:10.1126/scitranslmed.3006843
88. Hawkridge T, Scriba TJ, Gelderbloem S, Smit E, Tameris M, Moyo S, et al. Safety and immunogenicity of a new tuberculosis vaccine, MVA85A, in healthy adults in South Africa. J Infect Dis (2008) 198(4):544–52. doi:10.1086/590185
89. Meyer J, Harris SA, Satti I, Poulton ID, Poyntz HC, Tanner R, et al. Comparing the safety and immunogenicity of a candidate TB vaccine MVA85A administered by intramuscular and intradermal delivery. Vaccine (2013) 31(7):1026–33. doi:10.1016/j.vaccine.2012.12.042
90. Beveridge NE, Price DA, Casazza JP, Pathan AA, Sander CR, Asher TE, et al. Immunisation with BCG and recombinant MVA85A induces long-lasting, polyfunctional Mycobacterium tuberculosis-specific CD4+ memory T lymphocyte populations. Eur J Immunol (2007) 37(11):3089–100. doi:10.1002/eji.200737504
91. Scriba TJ, Tameris M, Smit E, van der Merwe L, Hughes EJ, Kadira B, et al. A phase IIa trial of the new tuberculosis vaccine, MVA85A, in HIV- and/or Mycobacterium tuberculosis-infected adults. Am J Respir Crit Care Med (2012) 185(7):769–78. doi:10.1164/rccm.201108-1548OC
92. Sander CR, Pathan AA, Beveridge NE, Poulton I, Minassian A, Alder N, et al. Safety and immunogenicity of a new tuberculosis vaccine, MVA85A, in Mycobacterium tuberculosis-infected individuals. Am J Respir Crit Care Med (2009) 179(8):724–33. doi:10.1164/rccm.200809-1486OC
93. Fletcher HA, Snowden MA, Landry B, Rida W, Satti I, Harris SA, et al. T-cell activation is an immune correlate of risk in BCG vaccinated infants. Nat Commun (2016) 7:11290. doi:10.1038/ncomms11290
Keywords: T-cell immunity, CD4+ T cells, vaccine-induced immunity, tuberculosis, vaccine, protective immunity, BCG, polyfunctional T cells
Citation: Lewinsohn DA, Lewinsohn DM and Scriba TJ (2017) Polyfunctional CD4+ T Cells As Targets for Tuberculosis Vaccination. Front. Immunol. 8:1262. doi: 10.3389/fimmu.2017.01262
Received: 14 July 2017; Accepted: 21 September 2017;
Published: 05 October 2017
Edited by:
Ulrich Emil Schaible, Forschungszentrum Borstel (LG), GermanyReviewed by:
Juraj Ivanyi, King’s College London, United KingdomCopyright: © 2017 Lewinsohn, Lewinsohn and Scriba. This is an open-access article distributed under the terms of the Creative Commons Attribution License (CC BY). The use, distribution or reproduction in other forums is permitted, provided the original author(s) or licensor are credited and that the original publication in this journal is cited, in accordance with accepted academic practice. No use, distribution or reproduction is permitted which does not comply with these terms.
*Correspondence: Deborah A. Lewinsohn, bGV3aW5zZGVAb2hzdS5lZHU=
Disclaimer: All claims expressed in this article are solely those of the authors and do not necessarily represent those of their affiliated organizations, or those of the publisher, the editors and the reviewers. Any product that may be evaluated in this article or claim that may be made by its manufacturer is not guaranteed or endorsed by the publisher.
Research integrity at Frontiers
Learn more about the work of our research integrity team to safeguard the quality of each article we publish.