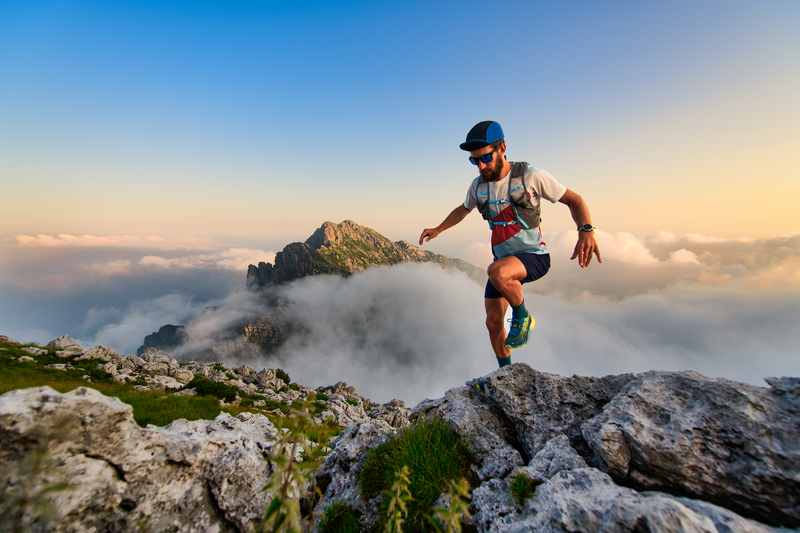
94% of researchers rate our articles as excellent or good
Learn more about the work of our research integrity team to safeguard the quality of each article we publish.
Find out more
REVIEW article
Front. Immunol. , 01 May 2017
Sec. Mucosal Immunity
Volume 8 - 2017 | https://doi.org/10.3389/fimmu.2017.00499
The lungs are vulnerable to attack by respiratory insults such as toxins, allergens, and pathogens, given their continuous exposure to the air we breathe. Our immune system has evolved to provide protection against an array of potential threats without causing collateral damage to the lung tissue. In order to swiftly detect invading pathogens, monocytes, macrophages, and dendritic cells (DCs)—together termed mononuclear phagocytes (MNPs)—line the respiratory tract with the key task of surveying the lung microenvironment in order to discriminate between harmless and harmful antigens and initiate immune responses when necessary. Each cell type excels at specific tasks: monocytes produce large amounts of cytokines, macrophages are highly phagocytic, whereas DCs excel at activating naïve T cells. Extensive studies in murine models have established a division of labor between the different populations of MNPs at steady state and during infection or inflammation. However, a translation of important findings in mice is only beginning to be explored in humans, given the challenge of working with rare cells in inaccessible human tissues. Important progress has been made in recent years on the phenotype and function of human lung MNPs. In addition to a substantial population of alveolar macrophages, three subsets of DCs have been identified in the human airways at steady state. More recently, monocyte-derived cells have also been described in healthy human lungs. Depending on the source of samples, such as lung tissue resections or bronchoalveolar lavage, the specific subsets of MNPs recovered may differ. This review provides an update on existing studies investigating human respiratory MNP populations during health and disease. Often, inflammatory MNPs are found to accumulate in the lungs of patients with pulmonary conditions. In respiratory infections or inflammatory diseases, this may contribute to disease severity, but in cancer patients this may improve clinical outcomes. By expanding on this knowledge, specific lung MNPs may be targeted or modulated in order to attain favorable responses that can improve preventive or treatment strategies against respiratory infections, lung cancer, or lung inflammatory diseases.
Respiratory diseases are among the leading causes of death worldwide, with lung infections, lung cancer, and chronic obstructive pulmonary disease (COPD) together accounting for several million deaths annually (1). The human respiratory tract can be broadly divided into the upper and lower airways. The upper airways consist of the nose, the pharynx, and the larynx. Organized like a tree, the lower airways begin with the trachea branching out into the bronchi, the bronchioles, and eventually the alveoli. A dense network of capillaries underlying the alveoli forms the basis of respiration with the critical exchange of oxygen and carbon dioxide that is necessary for life. The thin, permeable membrane of the alveolar epithelium is vulnerable to penetration of foreign particles and disruption upon inflammation. In humans, the total alveolar surface area is approximately 140 m2 (2). To ensure that such a vast area is well monitored, monocytes, macrophages, and dendritic cells (DCs) serve as sentinels at the interface between the external environment and our body. Together termed mononuclear phagocytes (MNPs), they are a heterogeneous population of antigen-presenting cells that have been well described in the lungs of mice and rats at steady state (3–8). Additionally, blood monocytes infiltrate the lungs upon inflammation (9). Their heterogeneity extends beyond morphological or phenotypic characteristics as different mouse MNPs exert different functionalities (10–12). MNPs play a central role in immune surveillance by being adept at capturing antigen to destroy them or to present them in order to activate the adaptive immune responses (13, 14). However, as shown in mice, each subset excels at different aspects of antigen uptake, processing, and presentation, with distinct capacities to migrate and polarize T cells, hence skewing immune responses differently (15–18). Importantly, investigations into MNPs are shifting toward tissue specificity, as immune cells residing in peripheral tissues have distinct traits compared to those in circulation, due to the cues given by the local microenvironment (19–21). The capacity of lung MNPs to regulate immunity has made them attractive targets for preventive or treatment strategies against respiratory infections, lung cancer, or lung inflammatory diseases (22–24). Before applying our knowledge of lung MNPs to the development of vaccine or therapeutic strategies for patients, a thorough characterization of human lung MNPs is required, to ensure a functional alignment of lung DCs from mice and men.
The term MNPs was first coined in the 1960s by van Furth, referring to both circulating monocytes and tissue macrophages (25), as opposed to polymorphonuclear phagocytes (granulocytes) (26), but their history dates further back. In the 1880s, the concept of phagocytosis (from ancient Greek, meaning “to devour”) was established by the Nobel Laureate Elie Metchnikoff, who described the ability of macrophages to engulf foreign entities as a defense mechanism (27, 28). Following labeling studies using radioactive thymidine, monocytes were defined as precursors of macrophages circulating in blood, as extensively studied by van Furth and others. In 1973, the Nobel Laureate Ralph Steinman discovered a novel type of “dendritic-shaped cell that can process and present antigen to activate naïve T cells” in the spleen of mice, calling them DCs in his seminal papers (29–32). DCs then joined monocytes and macrophages as another member of the MNP system.
For decades since their discovery, monocytes were thought to only exist in peripheral blood, differentiating into DCs and macrophages upon entry into tissues (33). This was supported by the ease in which monocytes can be skewed to behave like DCs or macrophages in vitro (34–37), depending on the culture conditions, and also in vivo during inflammation (38–41). However, more careful lineage studies in mice have identified hematopoietic precursors to DCs [called committed DC progenitors (CDPs)] that are distinct from monocytes (Figure 1A, left panel) (42–44). CDPs can further differentiate to plasmacytoid DCs (PDCs) or pre-classical DCs (cDCs) that can become cDC1 [CD141+ myeloid DCs (MDCs) in humans] or cDC2 (CD1c+ MDCs in humans). Maintenance of DC development is linked to their expression of Fms-like tyrosine kinase 3 (FLT3) and their ability to respond to FLT3 ligand (45, 46). In parallel, monocytes diverge at an earlier stage and are derived from a different progenitor (called common monocyte progenitor) (47), relying on the cytokine colony-stimulating factor 1 for their development (Figure 1A, middle panel). These observations in mice have also been confirmed in humans following the identification of DC precursors in circulation, cord blood, and bone marrow (Figure 1B) (48, 49). Another paradigm-shifting discovery is that tissue-resident macrophages are not exclusively derived from circulating monocytes, as has been the dogma following van Furth’s findings in the 1960s. Instead, mouse tissue-resident macrophages can develop from embryonic precursors such as yolk sac macrophages or fetal liver monocytes (5, 50–56) (Figure 1A, right panel). In short, there is mounting evidence to suggest that monocytes, DCs, and macrophages are not developmental progressions from one cell type to another, but instead originate from distinct precursors.
Figure 1. The embryonic and hematopoietic development of mononuclear phagocytes (MNPs) in mice and men. (A) In mice, monocytes, dendritic cells (DCs), and macrophages originate from distinct lineages. DCs (left panel) and monocytes (middle panel) originate from hematopoietic stem cell (HSC) precursors known as committed DC progenitor (CDP) and common monocyte progenitor (cMop), respectively. Some tissue macrophages have an embryonic origin, either from yolk sac macrophages or fetal liver monocytes (right panel). DCs express different transcription factors critical to their development, such as basic leucine zipper ATF-like 3 (BATF3) for cDC1s, interferon regulatory factor 4 (IRF4) for cDC2s, and E2-2 for plasmacytoid DCs (PDCs). MNPs are also differentially dependent on various growth factors such as Fms-related tyrosine kinase 3 ligand (FLT3L) for DCs (left panel), CSF1 and CSF2 for monocytes (middle panel), and colony-stimulating factor 1 (CSF1) and CSF2 and IL-34 for macrophages (right panel). (B) In humans, in vitro culture models have been used to recapitulate in vivo DC hematopoiesis employing progenitors from human cord blood and bone marrow; hGMP, human granulocyte–monocyte–DC progenitor; hMDP, human monocyte–DC progenitor; hCDP, human common DC progenitor; hprec-DC, human migratory precursor.
The matter is complicated by the plasticity of monocytes that can acquire different functional properties shared by macrophages and DCs, depending on the inflammatory environment (57, 58). Identification of cell types based purely on expression of surface markers or functional specialization presents a challenge as several different populations share the same receptors, and subsets can acquire or lose functional capacities during inflammation (59). Beyond semantics, the definition of cell populations is important for interpretation and translation of findings between different groups, especially when specific functional attributes are assigned to distinct populations. A shift toward complementing phenotypic identification with transcriptional profiling has allowed a better separation of DCs, monocytes, and macrophages, including a better alignment of cells across species and tissues.
There are several methods of sampling the human respiratory tract. The most common source of human lung tissue comes from surgical resections, due to lung tumor or other lung diseases (Figure 2A) (60). The surrounding, non-diseased parts of the lungs are used in studies as a representation of healthy tissue. These samples constitute parenchymal lung tissue. However, as the lungs are highly vascularized, the surgical tissues obtained also consist of intravascular cells from the circulation. Lungs from organ donors that are available but not used for transplantation are an alternative source of lung tissue for immunological research (61). Perfusion of whole lungs is possible to remove intravascular cells, in order to discriminate between cells residing in the lung tissue and those in the lung vasculature (62). Further, investigation of lung-associated draining lymph nodes is possible with whole lungs, enabling the assessment of migration to lymphoid tissues and interaction with adaptive immune cells—key features of DCs (62, 63). A dependence on surgical lung material or lung transplants presents a challenge to experimental research, as both types of samples are not readily available. Furthermore, migratory immune cells such as MNPs isolated from adjacent sites may already be affected by unpredictable direct or bystander effects due to the diseased tissue. Lung specimens can also be acquired by performing a bronchoscopy to obtain bronchial wash (Figure 2B), bronchoalveolar lavages (Figure 2C), bronchial biopsies (Figure 2D), or bronchial brushing (64–66). Unlike the parenchymal tissue obtained from surgical resections or organ transplants, specimens obtained by bronchoscopy reflect cells lining the airways and those embedded within mucosal surfaces. Sequential lavages allow separation of bronchial and alveolar samples, termed bronchial wash (Figure 2B) and bronchoalveolar lavage, respectively (64). One of the advantages of using cells from lavages is that the cells undergo minimal manipulation prior to phenotypic and functional analyses, unlike tissue samples that need to undergo mechanical processing or enzymatic digestion in order to obtain single cells. An alternative approach to understanding human immune cells in inaccessible tissues is by harnessing the humanized mouse models (67, 68). However, humanized mouse models have their own caveats: the lung architecture of mice is different from that of humans, as murine lungs show less airway branching and lack respiratory bronchioles (69). Importantly, cytokines and chemokines produced by mouse epithelial cells may not act on the human cognate receptors (70). Knock-in mice expressing human cytokines have been developed to address this limitation (67). Since immune cells are in close contact with the respiratory epithelium (71), compromised communication between epithelial cells and immune cells may influence immunological events in humanized mice. Finally, given the massive size of the respiratory tract, the composition of immune cells may not be equally distributed but instead vary depending on which portion of the lungs is being examined. Considering the anatomic compartmentalization within the lungs may be valuable when comparing findings between studies using different sources of respiratory material.
Figure 2. Identities of human mononuclear phagocytes (MNPs) differ in specific respiratory compartments sampled by different methods. Alveolar macrophages (AMs), interstitial macrophages (IM), tissue monocytes, monocyte-derived dendritic cells (mo-DCs), and three subsets of bona fide dendritic cells including cDC1, cDC2, and plasmacytoid DCs (PDCs) have been documented in different compartments of the human respiratory tract. (A) Cells within the lung parenchymal tissue can be assessed in whole lungs or surgical resections. Cellular components of the airways can be sampled by performing lavages including (B) a shallow bronchial wash or (C) a deeper bronchoalveolar lavage, whereas (D) cells within the respiratory mucosal tissue can be sampled by taking mucosal biopsies. Lung illustration modified from Servier Medical Art.
Cell surface markers continue to be a reliable source of information for classification of DCs, monocytes, and macrophages based on their phenotype, preferably supported by transcriptomic and functional analyses. Comparative studies have attempted to unify the MNP populations between mice and humans. The cell surface markers used to identify and sort out individual populations of MNPs based on flow cytometry are summarized in Table 1. A common flow cytometric gating strategy used by our group and several others to identify DCs in blood and tissue is by first gating on all hematopoietic cells (CD45+), excluding all lineage cells [monocytes, B cells, T cells, natural killer (NK) cells, and neutrophils] and then gating on cells expressing the MHC class II molecule, HLA-DR+ cells to identify DCs (72, 73). CD11c can be used to distinguish MDCs from PDCs. Aside from peripheral blood, these DC populations have also been identified in human bone marrow, skin, gut, lungs, liver, spleen, lymph nodes, and tonsils (62, 73–78). However, the precise phenotype of DCs in human tissue continues to be investigated and debated upon, with the most studied tissue in humans being the skin (79, 80). In light of different markers used to identify DCs in different human tissues, Guilliams et al. propose a framework to standardize the identification of DCs in human tissues at steady state and during inflammation (81). Here, we revisit early studies investigating human lung MNPs and review the most recent developments in the field, with special attention to cellular players in specific respiratory compartments.
Alveolar macrophages are the first MNP population to be described, given the relative ease of obtaining them by bronchoalveolar lavage and their abundance (up to 95% of cells lavaged in healthy subjects are AMs) (82). AMs play an important role in removing surfactants and other foreign materials, ensuring that the lungs remain free of debris (83). Impaired AM function in patients result in pulmonary alveolar proteinosis, a rare disorder that can be treated with granulocyte/macrophage colony-stimulating factor (84, 85). It was reported in the 1970s that human AMs have local proliferative capacity and therefore need not be replenished by monocytes from the bone marrow (86). Yet, AMs can also be replenished by monocytes from the bone marrow, as illustrated in patients receiving bone marrow transplants for hematologic disorders (87). The seemingly contradictory findings are now better understood based on recent studies in mice indicating that AMs can be derived from yolk sac macrophages, fetal liver, and adult monocytes given a vacant niche (56). In more recent studies involving human lung transplantations, almost 100% of AMs detected in BAL are donor derived, with a capacity to self-renew (88, 89). This finding supports the niche hypothesis, as transplanted lungs already occupied by the donor’s AMs need not be replaced by the recipient’s circulating monocytes (88, 89).
Pioneering studies on human MNPs in the lungs relied mostly on morphology visualized by microscopy and expression of single markers such as CD11c and HLA-DR by immunohistochemistry. By flow cytometry, human AMs can be identified based on their large size and high granularity (90, 91). Additional markers currently used for identification of AMs include CD206, CD163, and CD169, although there may be overlaps with other lung phagocytes (Table 1) (92–94). The superiority of human AMs at internalization of bacterial particles, compared to lung DCs or monocytes was recently highlighted by Patel et al. upon in vitro exposure of lung phagocytes to different bacteria such as Escherichia coli, Staphylococcus aureus, and Bacillus anthracis (95). However, unlike human lung DCs or monocytes, AMs do not upregulate CD83, CD86, or CCR7 upon exposure to bacteria (95). Despite their surface expression of HLA-DR, AMs are poor inducers of antigen-induced T cell proliferation and also poor stimulators of allogeneic mixed lymphocyte reactions (MLR) (96–98). Instead, AMs promote tolerance by suppressing lymphocyte activation via production of transforming growth factor β (TGF-β) and prostaglandins (99, 100). Although AMs are typically quiescent in order to minimize damage to the delicate alveoli, they can mount inflammatory responses when necessary (101). They release soluble mediators such as IL-8, a chemotactic factor important in the recruitment of neutrophils into the airways (102–104). Transcriptomic profiling of human AMs upon LPS stimulation has identified interferon-related genes that can fine-tune the early cytokine responses (105). The dynamic roles of AMs will be further discussed in this review during different disease conditions.
Dendritic cells have been described in the nasal mucosa (106–108), the epithelium, and submucosa of conducting airways (6, 7, 109, 110), the lung parenchyma (7), and also alveolar surfaces (91). The first reference to human lung DCs was in 1986, when Sertl et al. identified HLA-DR+ cells in preparations of human airway epithelium, lung parenchyma, and visceral pleura by light and electron microscopy (7). HLA-DR+ DCs with extending processes are interspersed between columnar epithelial cells in the large airways; in samples obtained from the lower trachea and mainstem bronchi. Lung mononuclear cells isolated from whole lungs are able to stimulate T cells in an allogeneic MLR more efficiently than blood monocytes (111). Removal of FcR+ cells improved the efficiency of stimulating allogeneic T cells, suggesting that the lung mononuclear cells consist of both DCs and monocytes/monocyte-derived cells (111). However, compared to blood monocytes, lung DCs are less potent at producing pro-inflammatory cytokines such as interleukin 1 (IL-1) and tumor necrosis factor (TNF) in response to LPS (112). In bronchoalveolar lavage, mononuclear cells can be distinguished from the majority population of AMs by flow cytometry based on their lack of autofluorescence (73, 90, 91, 113). Immunohistochemical staining in situ suggests a local interaction between lung DCs and T cells, as they form small clusters in the subepithelial tissue of the bronchus (91). In vitro, the non-autofluorescent mononuclear cells excel at stimulating T cell proliferation, compared to the highly autofluorescent AMs (90, 114, 115). Several differences in the expression of cell surface receptors allow a distinction between lung DCs and blood DCs: a portion of DCs residing in the bronchial epithelium express CD1a (110), and mannose receptor (CD206), as illustrated by their capacity to take-up soluble antigens (114). Due to the limited use of phenotypic markers for identification of lung MNPs, lung DCs referred to in earlier studies may be a mixed population of bona fide DCs and monocyte-derived cells. Furthermore, the isolation methods used are often extensive, involving enzymatic digestion followed by Ficoll separation and plastic adherence (114), which may lead to the induction of phenotypical and functional differences.
Following the identification of specific blood DC antigen (BDCA) markers (116), similar subpopulations of human DCs have been detected in the nasal mucosa, lung parenchyma, vascular walls as well as bronchial and alveolar surfaces; PDCs expressing BDCA-2 and BDCA-4 or MDCs expressing either BDCA-1 (CD1c+ MDCs or cDC2) or BDCA-3 (CD141+ MDCs or cDC1) (62, 68, 73–75, 93, 117–121) (Figure 2). Additionally, DCs expressing CD1a can be detected in both the epithelium and submucosa of the airways (117, 118, 122). More detailed phenotypic analyses revealed that a subpopulation of CD1c+ MDCs express CD1a, mannose receptor (CD206) and langerin (CD207) in human lungs, unlike blood CD1c+ MDCs (62, 73, 74, 123). Similar to their blood counterparts, lung CD141+ MDCs also express CLEC9A, whereas lung PDCs express CD123 (68, 73, 74). Many of the markers mentioned above are unique to humans. However, CADM1 and CD172a, commonly expressed in different tissues of mice and men, can be used to identify cDC1 (CD141+ MDCs) and cDC2 (CD1c+ MDCs), respectively, thus allowing comparisons of DC subsets across species and tissues (65). Additionally, identification of DC subsets via transcription factors such as IRF8 and IRF4, required for the development or function of cDC1 and cDC2, respectively (124), has been successfully used in identifying human lung DCs too (63, 81). This standardization will be valuable in streamlining investigations of the same cell from a specific lineage that may express distinct markers under different conditions.
Importantly, there are functional differences between the different subsets of lung DCs. First, they express different repertoires of toll-like receptors, enabling them to respond to different microbial products. CD1c+ MDCs and CD141+ MDCs express TLR1, TLR2, TLR3, TLR4, TLR6, and TLR8 at the mRNA level, whereas PDCs express TLR7 and TLR9 (125). This is consistent with the TLR repertoire of the corresponding subsets in blood (126). Upon stimulation of TLR4 with LPS and TLR3 with poly(I:C), both lung CD141+ MDCs and CD1c+ MDCs induce TNF, IL-1β, and IL-6, whereas stimulation of TLR7/8 induces pro-inflammatory cytokines on lung PDCs (73, 125). Second, they have different T cell stimulatory capacities. Altogether, lung DCs are superior at stimulating allogeneic MLR compared to the highly autofluorescent AMs or lung CD14+ monocytes (62, 117, 118). Among the lung DC subsets, CD1c+ MDCs are best at activating T cells, followed by CD141+ MDCs with a more intermediate capacity, and PDCs being the poorest inducers of T cell proliferation in an allogeneic MLR, when compared side-by-side (125). Further, CD4+ T cells cocultured with lung MDCs upregulate the activation marker CD25 compared to unstimulated T cells and can also differentiate into memory T cells by upregulating expression of CD45RO (119). Human lung CD1c+ MDCs are potent at inducing a Th17 phenotype upon Aspergillus fumigatus challenge by producing IL-23p19, compared to CD141+ MDCs or CD14+ cells in the lungs (75). Further, human lung CD1c+ MDCs induce CD103 expression on CD8 T cells, a marker of tissue residency, via expression of TGF-β, unlike CD141+ MDCs (68). Following exposure to live-attenuated influenza virus (LAIV) in vitro, human lung CD141+ MDCs can induce both Th1 (IFN-γ) and Th2 (IL-4) responses (127). Interestingly, lung CD141+ MDCs are more efficient than lung CD1c+ MDCs at inducing Th2 responses, due to increased expression of OX40 ligand upon exposure to LAIV (127). However, most studies investigating T cell activating capacity of human lung DCs have been conducted in vitro. Assessment of lung-associated lymph nodes suggests that only CD1c+ MDCs (cDC2) accumulate in follicular zones of lung-draining lymph nodes (62, 63).
In human peripheral blood, monocytes can be subdivided into three populations based on their expression of CD14 and CD16: classical monocytes (CD14+CD16−), intermediate monocytes (CD14+CD16+), and non-classical monocytes (CD14dimCD16+). By comparison, less is known about monocytes in the lungs, as they were long thought to differentiate into DCs or macrophages upon arrival into peripheral tissues (9, 25). Only recently have tissue monocytes been demonstrated in peripheral tissues including lungs of mice, deriving from Ly6Chi classical monocytes (5, 12). In human lungs, Schlitzer et al. and Haniffa et al. refer to CD14+ cells as CD14+ DCs (74, 75), whereas Yu et al. could identify a large population of CD14+ cells that express HLA-DR and CD11c but had not characterized them further as they were not the focus of their study (68). Applying a similar flow cytometric gating strategy as used for blood monocytes, we and others have identified three populations of monocytes in lung tissue and bronchoalveolar lavage at varying proportions (62, 73, 93). In the airways, cells corresponding to intermediate monocytes are the most frequent monocyte subset (73). All monocyte populations from the airways responded by producing TNF upon stimulation of TLR3, TLR4, and TLR7/8 ex vivo (73). Lung CD14+ cells could also stimulate both CD4 and CD8 T cells in MLRs, but not as potently as CD1c+ MDCs (62). Desch et al. further subdivided CD14+ cells into tissue monocytes and monocyte-derived DCs based on expression of CD1c (62). We could confirm that a subpopulation of monocytes upregulated CD1c in the airways and in bronchial tissue, without upregulating typical macrophage markers (73). In contrast, CD14+ cells in the human dermis are referred to as monocyte-derived macrophages, as their gene expression program strongly overlaps with human monocytes and macrophages, but not with human DCs (128). The characterization of interstitial macrophages (IM) in human lung parenchymal tissue has been limited (129). Based on studies in rodents, IM originate from bone marrow-derived monocytes and unlike AMs, have a short half-life (130, 131). As a consequence, they may share common phenotypic markers with monocytes. In rhesus macaques, IM share many cell surface markers as blood CD14+ monocytes using an extensive flow cytometric panel, except for CCR2, a tissue-homing chemokine receptor that is not expressed by IM, presumably downregulated by their monocytic precursors upon entering tissue (130). Yu et al. propose the usage of sialoadhesin (CD169) to distinguish between AMs (CD169+) and IM (CD169−) (93, 94). Taken together, our detailed knowledge on human MNPs during steady state has greatly improved in recent years. Nevertheless, many questions remain, including the extent to which functional qualities of these subsets are cell-intrinsic or influenced by the environment. Investigation of human lung MNPs during respiratory infection or inflammation offers an insight into how these cells behave during dysregulated situations.
At steady state, monocytes, macrophages, and DCs are important in maintaining homeostasis and ensuring tolerance toward harmless antigens arriving in the lungs. In the event of infection or inflammation, how lung MNPs behave in a perturbed environment may clarify the individual roles the different subsets play in initiating immunity or contributing to pathogenicity. Respiratory diseases encompass several pathological conditions affecting the lungs. Together, lung diseases remain among the leading causes of death and disability (1). Focusing on diseases of the lower respiratory tract, respiratory diseases can be further subdivided into bronchial (e.g., acute bronchitis, COPD, and asthma) or interstitial [e.g., sarcoidosis and idiopathic pulmonary fibrosis (IPF)] diseases (132–134). Bronchial diseases are often obstructive leading to blocked airways, whereas interstitial diseases are restrictive leading to decreased lung volume. Infections with viruses or bacteria can affect both bronchial and interstitial compartments. Lung MNPs are also implicated in lung cancers, divided into small-cell lung carcinoma or non-small-cell lung carcinoma. Here, we discuss the existing literature on the involvement of human lung MNPs in the lung diseases that have been best studied (Table 2). However, care should be taken in prescribing function to specific populations in pathological conditions, as the distinction between monocytes, DCs, and individual subsets within them are limited in earlier studies.
Table 2. Dysregulations in frequencies or functions of lung mononuclear phagocytes in human disease.a
A striking observation in many studies is that cigarette smoking can alter both frequency and function of lung MNPs (135–140). Cigarette smokers have substantially increased numbers of AMs in the lungs, with identical capacity to phagocytose bacteria compared to AMs of non-smokers (141, 142). AMs of smokers have altered metabolic and enzymatic activities (138, 140, 143). Production of cytokines such as IL-1 is also reduced in AMs of smokers (139). Hence, it is important to compare observations in patients with not only age- and sex-matched healthy controls but also with similar smoking status. Other inhaled particles including air pollutants, such as diesel exhaust, however, typically cannot be controlled in study subjects. These inhaled particles have been shown to impair the ability of AMs to phagocytose, described in detail in the following review (144). As such, comparisons of patient groups with endemic healthy controls exposed to similar particulate matter in the air would account for the contribution of environmental factors.
Lung MNPs play a central role in COPD, a disease characterized by aberrant inflammatory responses to cigarette smoke and other inhaled particles (145–147). Persistent inflammation occurs in the lungs of COPD patients (148). A key player is the pro-inflammatory cytokine TNF, potentially produced by lung MNPs, which is increased in sputum and serum of COPD patients (149). However, anti-TNF treatment with infliximab alone was not effective on COPD patients (150). The timing of treatment may be an important factor, as AMs in exacerbation-prone COPD patients exhibit exhaustion: upon bacterial challenge in vitro, poorer cytokine responses are observed compared to non-exacerbation-prone COPD patients (151). Langerin+ DCs expressing CCR6 accumulate in the airways of COPD patients, increasing with disease severity and higher levels of the chemoattractant CCL20 (the ligand for CCR6) (152, 153). Maturation markers such as CD40, CD80, CD83, and CD86 are also upregulated on lung DC subsets in COPD patients, correlating with disease severity (153–155). Conflicting with this observation, others report that in their cohort of COPD patients, DCs are more immature than DCs isolated from smokers without COPD (156, 157). Tsoumakidou et al. support the hypothesis that lung DCs in COPD patients are tolerogenic by reporting that lung CD1c+ MDCs produce more IL-10 and induce regulatory T cells, unlike CD1c+ MDCs from smokers without COPD (158). Differences in source of lung tissue and sample preparation may contribute to the conflicting data, underlining the importance of harmonized protocols to allow for comparisons across different study cohorts.
Asthma is characterized by bronchial hyperresponsiveness and influx of inflammatory cells, and the role of lung MNPs in asthma has been widely studied (159–161). Lambrecht et al. pioneered the field more than 15 years ago by illustrating how DCs induce Th2 responses to inhaled antigens in mice (162, 163). Indeed, studies in humans indicate that DCs, especially CD1c+ MDCs, accumulate in sputum and bronchial mucosa of asthmatic patients upon allergen challenge (164–169). The increase in DCs in the lungs can be controlled by inhaled corticosteroids, currently the preferred treatment for asthma management (164). In pediatric patients with steroid-treated asthma, their airway DCs expressed lower levels of the co-stimulatory molecule CD86 (167). Supporting data in mouse models suggesting that DCs are responsible for maintaining Th2 responses to inhaled allergens (170), Greer et al. report an increase in CD1c+ MDCs in the epithelium of patients with high expression of Th2 genes in the airways, but not in those with low Th2 genes (169). In addition to Th2-driven asthma, Th17 cells and cytokines have been described as driving more severe disease with neutrophilic inflammation (171). Although human MNPs have not been investigated in contributing to Th17-mediated asthma, existing studies suggest that CD1c+ MDCs can control mucosal IL-17 responses (75). AMs are also implicated in airway remodeling (172), a central feature of asthma. Further, AMs from asthmatic patients appear to overexpress CCL17, the ligand for CCR4 that is upregulated on T cells homing to the lungs (17, 173). This may contribute to airway inflammation experienced by asthmatics.
Pulmonary sarcoidosis is characterized by the formation of granulomas in the lungs: T cells accumulate in the lungs surrounding an unknown antigen that has been phagocytosed by AMs or DCs (174). AMs from sarcoidosis patients produce a variety of cytokines including TNF, IL-2, and IL-6 (175). Massive TNF production by AMs is indicative of increased disease progression (176). Pulmonary fibrosis, observed in both IPF and end stage sarcoidosis, is hypothesized to be a consequence of aberrant wound healing; abnormally excessive production of fibrous connective tissue results in fibrosis (177). Rennard et al. illustrated in the 1980s that AMs from patients with IPF and pulmonary sarcoidosis produce 10 times more fibronectin, a chemoattractant for human lung fibroblasts, than AMs from healthy controls (178). Prasse et al. further identified CCL18, a signature cytokine produced by anti-inflammatory macrophages, as the key component perpetuating pulmonary fibrosis by stimulating collagen production by fibroblasts (179).
Similar to COPD and asthma, DCs accumulate in the lungs of patients with IPF and sarcoidosis, in particular CD1c+ MDCs (169, 180–185). In IPF patients, DCs aggregate together with infiltrating lymphocytes, forming an organized lymphoid structure, close to fibroblasts that produce chemokines for recruitment of immune cells (186). In an in vitro study, Freynet et al. illustrate that lung fibroblasts from IPF patients may modulate DC function by downregulating their capacity to stimulate T cells in an MLR (187). However, as DCs derived from the Mutz-3 myeloid cell line were used in this study, it remains to be assessed if primary lung DCs of IPF patients would respond in a similar manner. In sarcoidosis, the precise role of DCs is still unclear: there is evidence suggesting that lung DCs initiate the inflammatory T cell response (188), whereas others report that DCs in sarcoidosis patients are anergic and less immunostimulatory (182, 189). This may be a consequence of studying patient samples at different times of disease progression and further studies are needed to better dissect this. A more detailed review of MNP involvement in granuloma formation has been discussed by Broos et al. recently (190).
Despite extensive studies in mice on the role of MNPs in detecting, controlling, and clearing infection in the lungs, investigation of human lung MNPs during respiratory infection has been limited. Most existing studies exploring the role of human MNPs and respiratory pathogens have used blood-derived MNPs exposed to specific pathogens in vitro. Most patients with respiratory infections do not typically undergo surgery or bronchoscopy, except for patients with tuberculosis. Bronchoscopies are occasionally performed on patients infected with Mycobacterium tuberculosis (M.tb) as a diagnostic strategy. Pathogenesis of tuberculosis involves a complex interplay between the bacterium and the host immune response, reviewed in greater detail by Sasindran and Torrelles (191). A key player, AMs can serve as a reservoir for the bacterium (191). When exposed to M.tb in vitro, AMs produce the pro-inflammatory cytokine TNF that correlates with the ability of AMs to support bacterial replication (192). An accumulation of immature DCs has also been reported in the airways of tuberculosis patients (193).
Another respiratory infection that is a global public health concern is influenza. In influenza-infected patients and also in participants of human challenge studies with influenza virus, cytokine levels during infection have been reported to increase both in the lungs and in circulation (194–196). As a potential contributor to pro-inflammatory cytokines, both monocytes and DCs have been reported to accumulate in the nasal mucosa of patients infected with influenza virus, at higher levels than in patients with respiratory syncytial virus (RSV) infection (197–200). However, in vitro infection of AMs with a highly pathogenic strain of influenza A virus does not cause excessive TNF production (201). In contrast, in vitro infection of AMs with RSV leads to production of TNF, IL-6, and IL-8 (202). In summary, our increased appreciation of how MNPs behave differently depending on their anatomical location suggests that earlier observations using blood MNPs may merit revisiting to more accurately understand the role of human lung MNPs, present at the site of infection, during infection with respiratory pathogens.
In lung cancer, various innate and adaptive immune cells are present in the cancer microenvironment (often referred to as the immune contexture), including MNPs (203, 204). The identification of cancer antigens has accelerated the development of antigen-specific immunotherapy targeting specific DCs to enhance the effector immune response, including cytotoxic T lymphocytes (CTLs), NK cells, and macrophages, ultimately responsible for destruction of tumor cells (23). In a study involving 74 patients with non-small-cell lung cancer, DCs are reported in lymphoid structures close to the tumor in pathologic lung biopsies (205). The density of mature DCs in these tumor sites correlate with improved clinical outcome (205). Similarly, another study involving 458 patients found that increased numbers of mature DCs in tumor-associated tertiary lymphoid structures correlated with an infiltration of T cells carrying an effector memory phenotype (206). In both studies, DCs were identified as cells expressing DC-LAMP (CD208) (205, 206). A more detailed phenotypic analysis of these cells would help in identifying and attributing functional qualities based on our existing knowledge from studies of human lung MNPs. In other studies, expression of programmed death-ligand 1 (PD-L1) on DCs found at tumor sites indicates poor prognosis, as lung DCs with high PD-L1 expression can retain their immature status and thus limit the activation of immunity (23, 207, 208). Hence, drugs such as monoclonal antibodies that target PD-L1 on DCs or the receptor PD-1 on T cells may boost immune responses by removing the inhibitory mechanism of DCs on T cells (209).
Our understanding of human lung MNPs has improved significantly in the past decade, with combined efforts to describe human counterparts to well-described populations in mice. However, differences in tissue sampling, processing protocols, and phenotypic gating strategies may hamper the ability to directly compare findings between research groups. A more collaborative approach, such as an expansion of the Immunological Genome project (210) focusing on human MNPs, could resolve inconsistencies and also provide broader understanding of the transcriptomic profiles of each population. More attention can also be given to monocytes and monocyte-derived cells in the lungs, given their plasticity and wide range of functionalities. An important body of research exists to support the view that lung MNPs are involved in various lung diseases, as illustrated by their overabundance in the lungs. Production of pro-inflammatory cytokines by MNPs contributes to an amplification of the inflammatory response in the lungs, central to the pathology of many lung diseases. Further efforts should focus on mechanisms leading to the aberrant accumulation of inflammatory MNPs in the lungs in order to aid the development of suitable therapeutic strategies. During respiratory infections, a relevant question to address is whether MNPs encountering pathogens, especially those lining the airways, can translocate and migrate to draining lymph nodes in order to participate in the activation and expansion of pathogen-specific T cells. Although tracking of individual cells would be technically difficult to perform in humans, this knowledge may influence the effectiveness of live vaccines that are delivered intranasally. Further, cancer immunotherapies may be enhanced by targeting specific populations of lung MNPs that can activate cancer antigen-specific CTLs and ensure that they home back to the lungs and remain in the tissue. Finally, the contribution of the microbiome has not been considered by most studies, despite indications that even in healthy humans, a diverse community of microbes exists in our lungs (211). A broader and deeper understanding of the complex cellular and molecular mechanisms dictating tissue trafficking and immune activity of human lung MNPs can be pivotal in our fight against respiratory diseases.
FB performed literature review and designed figures and tables. FB and AS-S organized and wrote the manuscript. FB, GR, AB, and AS-S edited the manuscript.
The authors declare that the research was conducted in the absence of any commercial or financial relationships that could be construed as a potential conflict of interest.
This work was supported by grants to AS-S from the following sources: the Swedish Research Council, the Swedish Foundation for Strategic Research, and the Swedish Heart-Lung Foundation.
1. World Health Organization. The Top 10 Causes of Death – Fact Sheet No. 310 2014. (2016) Available from: http://www.who.int/mediacentre/factsheets/fs310/en/
2. Gehr P, Bachofen M, Weibel ER. The normal human lung: ultrastructure and morphometric estimation of diffusion capacity. Respir Physiol (1978) 32(2):121–40. doi: 10.1016/0034-5687(78)90104-4
3. Green GM, Kass EH. The role of the alveolar macrophage in the clearance of bacteria from the lung. J Exp Med (1964) 119:167–76. doi:10.1084/jem.119.1.167
4. Holt PG, Schon-Hegrad MA, Oliver J. MHC class II antigen-bearing dendritic cells in pulmonary tissues of the rat. Regulation of antigen presentation activity by endogenous macrophage populations. J Exp Med (1988) 167(2):262–74. doi:10.1084/jem.167.2.262
5. Jakubzick C, Gautier EL, Gibbings SL, Sojka DK, Schlitzer A, Johnson TE, et al. Minimal differentiation of classical monocytes as they survey steady-state tissues and transport antigen to lymph nodes. Immunity (2013) 39(3):599–610. doi:10.1016/j.immuni.2013.08.007
6. Schon-Hegrad MA, Oliver J, McMenamin PG, Holt PG. Studies on the density, distribution, and surface phenotype of intraepithelial class II major histocompatibility complex antigen (Ia)-bearing dendritic cells (DC) in the conducting airways. J Exp Med (1991) 173(6):1345–56. doi:10.1084/jem.173.6.1345
7. Sertl K, Takemura T, Tschachler E, Ferrans VJ, Kaliner MA, Shevach EM. Dendritic cells with antigen-presenting capability reside in airway epithelium, lung parenchyma, and visceral pleura. J Exp Med (1986) 163(2):436–51. doi:10.1084/jem.163.2.436
8. Vermaelen K, Pauwels R. Pulmonary dendritic cells. Am J Respir Crit Care Med (2005) 172(5):530–51. doi:10.1164/rccm.200410-1384SO
9. Shi C, Pamer EG. Monocyte recruitment during infection and inflammation. Nat Rev Immunol (2011) 11(11):762–74. doi:10.1038/nri3070
10. GeurtsvanKessel CH, Lambrecht BN. Division of labor between dendritic cell subsets of the lung. Mucosal Immunol (2008) 1(6):442–50. doi:10.1038/mi.2008.39
11. Plantinga M, Hammad H, Lambrecht BN. Origin and functional specializations of DC subsets in the lung. Eur J Immunol (2010) 40(8):2112–8. doi:10.1002/eji.201040562
12. Tamoutounour S, Guilliams M, Montanana Sanchis F, Liu H, Terhorst D, Malosse C, et al. Origins and functional specialization of macrophages and of conventional and monocyte-derived dendritic cells in mouse skin. Immunity (2013) 39(5):925–38. doi:10.1016/j.immuni.2013.10.004
13. Banchereau J, Steinman RM. Dendritic cells and the control of immunity. Nature (1998) 392(6673):245–52. doi:10.1038/32588
14. Blank F, von Garnier C, Obregon C, Rothen-Rutishauser B, Gehr P, Nicod L. Role of dendritic cells in the lung: in vitro models, animal models and human studies. Expert Rev Respir Med (2008) 2(2):215–33. doi:10.1586/17476348.2.2.215
15. Vermaelen KY, Carro-Muino I, Lambrecht BN, Pauwels RA. Specific migratory dendritic cells rapidly transport antigen from the airways to the thoracic lymph nodes. J Exp Med (2001) 193(1):51–60. doi:10.1084/jem.193.1.51
16. Helft J, Manicassamy B, Guermonprez P, Hashimoto D, Silvin A, Agudo J, et al. Cross-presenting CD103+ dendritic cells are protected from influenza virus infection. J Clin Invest (2012) 122(11):4037–47. doi:10.1172/JCI60659
17. Mikhak Z, Strassner JP, Luster AD. Lung dendritic cells imprint T cell lung homing and promote lung immunity through the chemokine receptor CCR4. J Exp Med (2013) 210(9):1855–69. doi:10.1084/jem.20130091
18. Lambrecht BN. Allergen uptake and presentation by dendritic cells. Curr Opin Allergy Clin Immunol (2001) 1(1):51–9. doi:10.1097/00130832-200102000-00010
20. Davies LC, Jenkins SJ, Allen JE, Taylor PR. Tissue-resident macrophages. Nat Immunol (2013) 14(10):986–95. doi:10.1038/ni.2705
21. Heath WR, Carbone FR. The skin-resident and migratory immune system in steady state and memory: innate lymphocytes, dendritic cells and T cells. Nat Immunol (2013) 14(10):978–85. doi:10.1038/ni.2680
22. Yewdell JW. Designing CD8+ T cell vaccines: it’s not rocket science (yet). Curr Opin Immunol (2010) 22(3):402–10. doi:10.1016/j.coi.2010.04.002
23. Palucka K, Banchereau J. Cancer immunotherapy via dendritic cells. Nat Rev Cancer (2012) 12(4):265–77. doi:10.1038/nrc3258
24. Figdor CG, de Vries IJ, Lesterhuis WJ, Melief CJ. Dendritic cell immunotherapy: mapping the way. Nat Med (2004) 10(5):475–80. doi:10.1038/nm1039
25. van Furth R, Cohn ZA. The origin and kinetics of mononuclear phagocytes. J Exp Med (1968) 128(3):415–35. doi:10.1084/jem.128.3.415
26. van Furth R, Cohn ZA, Hirsch JG, Humphrey JH, Spector WG, Langevoort HL. The mononuclear phagocyte system: a new classification of macrophages, monocytes, and their precursor cells. Bull World Health Organ (1972) 46(6):845–52.
27. Yona S, Gordon S. From the reticuloendothelial to mononuclear phagocyte system – the unaccounted years. Front Immunol (2015) 6:328. doi:10.3389/fimmu.2015.00328
28. Metchnikoff E, Binnie FG. Immunity in Infective Diseases. Cambridge: University Press (1905). xvi, 591 p.
29. Steinman RM, Cohn ZA. Identification of a novel cell type in peripheral lymphoid organs of mice. I. Morphology, quantitation, tissue distribution. J Exp Med (1973) 137(5):1142–62. doi:10.1084/jem.137.5.1142
30. Steinman RM, Kaplan G, Witmer MD, Cohn ZA. Identification of a novel cell type in peripheral lymphoid organs of mice. V. Purification of spleen dendritic cells, new surface markers, and maintenance in vitro. J Exp Med (1979) 149(1):1–16. doi:10.1084/jem.149.1.1
31. Steinman RM, Cohn ZA. Identification of a novel cell type in peripheral lymphoid organs of mice. II. Functional properties in vitro. J Exp Med (1974) 139(2):380–97. doi:10.1084/jem.139.6.1431
32. Steinman RM, Adams JC, Cohn ZA. Identification of a novel cell type in peripheral lymphoid organs of mice. IV. Identification and distribution in mouse spleen. J Exp Med (1975) 141(4):804–20.
33. Cohn ZA, Benson B. The differentiation of mononuclear phagocytes. Morphology, cytochemistry, and biochemistry. J Exp Med (1965) 121:153–70. doi:10.1084/jem.121.1.153
34. Palucka KA, Taquet N, Sanchez-Chapuis F, Gluckman JC. Dendritic cells as the terminal stage of monocyte differentiation. J Immunol (1998) 160(9):4587–95.
35. Sallusto F, Lanzavecchia A. Efficient presentation of soluble antigen by cultured human dendritic cells is maintained by granulocyte/macrophage colony-stimulating factor plus interleukin 4 and downregulated by tumor necrosis factor alpha. J Exp Med (1994) 179(4):1109–18. doi:10.1084/jem.179.4.1109
36. Inaba K, Inaba M, Romani N, Aya H, Deguchi M, Ikehara S, et al. Generation of large numbers of dendritic cells from mouse bone marrow cultures supplemented with granulocyte/macrophage colony-stimulating factor. J Exp Med (1992) 176(6):1693–702. doi:10.1084/jem.176.6.1693
37. Caux C, Vanbervliet B, Massacrier C, Dezutter-Dambuyant C, de Saint-Vis B, Jacquet C, et al. CD34+ hematopoietic progenitors from human cord blood differentiate along two independent dendritic cell pathways in response to GM-CSF+TNF alpha. J Exp Med (1996) 184(2):695–706. doi:10.1084/jem.184.2.695
38. Randolph GJ, Inaba K, Robbiani DF, Steinman RM, Muller WA. Differentiation of phagocytic monocytes into lymph node dendritic cells in vivo. Immunity (1999) 11(6):753–61. doi:10.1016/S1074-7613(00)80149-1
39. Geissmann F, Jung S, Littman DR. Blood monocytes consist of two principal subsets with distinct migratory properties. Immunity (2003) 19(1):71–82. doi:10.1016/S1074-7613(03)00174-2
40. Serbina NV, Salazar-Mather TP, Biron CA, Kuziel WA, Pamer EG. TNF/iNOS-producing dendritic cells mediate innate immune defense against bacterial infection. Immunity (2003) 19(1):59–70. doi:10.1016/S1074-7613(03)00171-7
41. Varol C, Landsman L, Fogg DK, Greenshtein L, Gildor B, Margalit R, et al. Monocytes give rise to mucosal, but not splenic, conventional dendritic cells. J Exp Med (2007) 204(1):171–80. doi:10.1084/jem.20061011
42. Naik SH, Sathe P, Park HY, Metcalf D, Proietto AI, Dakic A, et al. Development of plasmacytoid and conventional dendritic cell subtypes from single precursor cells derived in vitro and in vivo. Nat Immunol (2007) 8(11):1217–26. doi:10.1038/ni1522
43. Onai N, Obata-Onai A, Schmid MA, Ohteki T, Jarrossay D, Manz MG. Identification of clonogenic common Flt3+M-CSFR+ plasmacytoid and conventional dendritic cell progenitors in mouse bone marrow. Nat Immunol (2007) 8(11):1207–16. doi:10.1038/ni1518
44. Merad M, Sathe P, Helft J, Miller J, Mortha A. The dendritic cell lineage: ontogeny and function of dendritic cells and their subsets in the steady state and the inflamed setting. Annu Rev Immunol (2013) 31:563–604. doi:10.1146/annurev-immunol-020711-074950
45. D’Amico A, Wu L. The early progenitors of mouse dendritic cells and plasmacytoid predendritic cells are within the bone marrow hemopoietic precursors expressing Flt3. J Exp Med (2003) 198(2):293–303. doi:10.1084/jem.20030107
46. Karsunky H, Merad M, Cozzio A, Weissman IL, Manz MG. Flt3 ligand regulates dendritic cell development from Flt3+ lymphoid and myeloid-committed progenitors to Flt3+ dendritic cells in vivo. J Exp Med (2003) 198(2):305–13. doi:10.1084/jem.20030323
47. Hettinger J, Richards DM, Hansson J, Barra MM, Joschko AC, Krijgsveld J, et al. Origin of monocytes and macrophages in a committed progenitor. Nat Immunol (2013) 14(8):821–30. doi:10.1038/ni.2638
48. Breton G, Lee J, Zhou YJ, Schreiber JJ, Keler T, Puhr S, et al. Circulating precursors of human CD1c+ and CD141+ dendritic cells. J Exp Med (2015) 212(3):401–13. doi:10.1084/jem.20141441
49. Lee J, Breton G, Oliveira TY, Zhou YJ, Aljoufi A, Puhr S, et al. Restricted dendritic cell and monocyte progenitors in human cord blood and bone marrow. J Exp Med (2015) 212(3):385–99. doi:10.1084/jem.20141442
50. Ginhoux F, Greter M, Leboeuf M, Nandi S, See P, Gokhan S, et al. Fate mapping analysis reveals that adult microglia derive from primitive macrophages. Science (2010) 330(6005):841–5. doi:10.1126/science.1194637
51. Schulz C, Gomez Perdiguero E, Chorro L, Szabo-Rogers H, Cagnard N, Kierdorf K, et al. A lineage of myeloid cells independent of Myb and hematopoietic stem cells. Science (2012) 336(6077):86–90. doi:10.1126/science.1219179
52. Guilliams M, De Kleer I, Henri S, Post S, Vanhoutte L, De Prijck S, et al. Alveolar macrophages develop from fetal monocytes that differentiate into long-lived cells in the first week of life via GM-CSF. J Exp Med (2013) 210(10):1977–92. doi:10.1084/jem.20131199
53. Hashimoto D, Chow A, Noizat C, Teo P, Beasley MB, Leboeuf M, et al. Tissue-resident macrophages self-maintain locally throughout adult life with minimal contribution from circulating monocytes. Immunity (2013) 38(4):792–804. doi:10.1016/j.immuni.2013.04.004
54. Yona S, Kim KW, Wolf Y, Mildner A, Varol D, Breker M, et al. Fate mapping reveals origins and dynamics of monocytes and tissue macrophages under homeostasis. Immunity (2013) 38(1):79–91. doi:10.1016/j.immuni.2012.12.001
55. Gomez Perdiguero E, Klapproth K, Schulz C, Busch K, Azzoni E, Crozet L, et al. Tissue-resident macrophages originate from yolk-sac-derived erythro-myeloid progenitors. Nature (2015) 518(7540):547–51. doi:10.1038/nature13989
56. van de Laar L, Saelens W, De Prijck S, Martens L, Scott CL, Van Isterdael G, et al. Yolk sac macrophages, fetal liver, and adult monocytes can colonize an empty niche and develop into functional tissue-resident macrophages. Immunity (2016) 44(4):755–68. doi:10.1016/j.immuni.2016.02.017
57. Guilliams M, van de Laar L. A Hitchhiker’s guide to myeloid cell subsets: practical implementation of a novel mononuclear phagocyte classification system. Front Immunol (2015) 6:406. doi:10.3389/fimmu.2015.00406
58. Guilliams M, Ginhoux F, Jakubzick C, Naik SH, Onai N, Schraml BU, et al. Dendritic cells, monocytes and macrophages: a unified nomenclature based on ontogeny. Nat Rev Immunol (2014) 14(8):571–8. doi:10.1038/nri3712
59. Boltjes A, van Wijk F. Human dendritic cell functional specialization in steady-state and inflammation. Front Immunol (2014) 5:131. doi:10.3389/fimmu.2014.00131
60. Fernandez FG, Kosinski AS, Burfeind W, Park B, DeCamp MM, Seder C, et al. The society of thoracic surgeons lung cancer resection risk model: higher quality data and superior outcomes. Ann Thorac Surg (2016) 102(2):370–7. doi:10.1016/j.athoracsur.2016.02.098
61. Ojo AO, Heinrichs D, Emond JC, McGowan JJ, Guidinger MK, Delmonico FL, et al. Organ donation and utilization in the USA. Am J Transplant (2004) 4(Suppl 9):27–37. doi:10.1111/j.1600-6135.2004.00396.x
62. Desch AN, Gibbings SL, Goyal R, Kolde R, Bednarek J, Bruno T, et al. Flow cytometric analysis of mononuclear phagocytes in nondiseased human lung and lung-draining lymph nodes. Am J Respir Crit Care Med (2016) 193(6):614–26. doi:10.1164/rccm.201507-1376OC
63. Granot T, Senda T, Carpenter DJ, Matsuoka N, Weiner J, Gordon CL, et al. Dendritic cells display subset and tissue-specific maturation dynamics over human life. Immunity (2017) 46(3):504–15. doi:10.1016/j.immuni.2017.02.019
64. Rennard SI, Ghafouri M, Thompson AB, Linder J, Vaughan W, Jones K, et al. Fractional processing of sequential bronchoalveolar lavage to separate bronchial and alveolar samples. Am Rev Respir Dis (1990) 141(1):208–17. doi:10.1164/ajrccm/141.1.208
65. Zavala DC. Diagnostic fiberoptic bronchoscopy: techniques and results of biopsy in 600 patients. Chest (1975) 68(1):12–9. doi:10.1378/chest.68.1.12
66. Wang KP, Mehta AC, Turner JF. Flexible Bronchoscopy. 2nd ed. Malden, MA: Blackwell Pub (2004). xi, 287 p.
67. Willinger T, Rongvaux A, Takizawa H, Yancopoulos GD, Valenzuela DM, Murphy AJ, et al. Human IL-3/GM-CSF knock-in mice support human alveolar macrophage development and human immune responses in the lung. Proc Natl Acad Sci U S A (2011) 108(6):2390–5. doi:10.1073/pnas.1019682108
68. Yu CI, Becker C, Wang Y, Marches F, Helft J, Leboeuf M, et al. Human CD1c+ dendritic cells drive the differentiation of CD103+ CD8+ mucosal effector T cells via the cytokine TGF-beta. Immunity (2013) 38(4):818–30. doi:10.1016/j.immuni.2013.03.004
69. Pinkerton KE, Gehr P, Castañeda A, Crapo JD. Architecture and cellular composition of the air-blood tissue barrier. In: Parent RA, editor. Comparative Biology of the Normal Lung. 2nd ed. Elsevier Inc. (2015). p. 105–17.
70. Manz MG. Human-hemato-lymphoid-system mice: opportunities and challenges. Immunity (2007) 26(5):537–41. doi:10.1016/j.immuni.2007.05.001
71. Whitsett JA, Alenghat T. Respiratory epithelial cells orchestrate pulmonary innate immunity. Nat Immunol (2015) 16(1):27–35. doi:10.1038/ni.3045
72. McGovern N, Schlitzer A, Janela B, Ginhoux F. Protocols for the identification and isolation of antigen-presenting cells in human and mouse tissues. Methods Mol Biol (2016) 1423:169–80. doi:10.1007/978-1-4939-3606-9_12
73. Baharom F, Thomas S, Rankin G, Lepzien R, Pourazar J, Behndig AF, et al. Dendritic cells and monocytes with distinct inflammatory responses reside in lung mucosa of healthy humans. J Immunol (2016) 196(11):4498–509. doi:10.4049/jimmunol.1600071
74. Haniffa M, Shin A, Bigley V, McGovern N, Teo P, See P, et al. Human tissues contain CD141hi cross-presenting dendritic cells with functional homology to mouse CD103+ nonlymphoid dendritic cells. Immunity (2012) 37(1):60–73. doi:10.1016/j.immuni.2012.04.012
75. Schlitzer A, McGovern N, Teo P, Zelante T, Atarashi K, Low D, et al. IRF4 transcription factor-dependent CD11b+ dendritic cells in human and mouse control mucosal IL-17 cytokine responses. Immunity (2013) 38(5):970–83. doi:10.1016/j.immuni.2013.04.011
76. McIlroy D, Troadec C, Grassi F, Samri A, Barrou B, Autran B, et al. Investigation of human spleen dendritic cell phenotype and distribution reveals evidence of in vivo activation in a subset of organ donors. Blood (2001) 97(11):3470–7. doi:10.1182/blood.V97.11.3470
77. Nizzoli G, Krietsch J, Weick A, Steinfelder S, Facciotti F, Gruarin P, et al. Human CD1c+ dendritic cells secrete high levels of IL-12 and potently prime cytotoxic T-cell responses. Blood (2013) 122(6):932–42. doi:10.1182/blood-2013-04-495424
78. Segura E, Valladeau-Guilemond J, Donnadieu MH, Sastre-Garau X, Soumelis V, Amigorena S. Characterization of resident and migratory dendritic cells in human lymph nodes. J Exp Med (2012) 209(4):653–60. doi:10.1084/jem.20111457
79. Haniffa M, Bigley V, Collin M. Human mononuclear phagocyte system reunited. Semin Cell Dev Biol (2015) 41:59–69. doi:10.1016/j.semcdb.2015.05.004
80. Haniffa M, Gunawan M, Jardine L. Human skin dendritic cells in health and disease. J Dermatol Sci (2015) 77(2):85–92. doi:10.1016/j.jdermsci.2014.08.012
81. Guilliams M, Dutertre C, Scott CL, McGovern N, Sichien D, Chakarov S, et al. Unsupervised high-dimensional analysis aligns dendritic cells across tissues and species. Cell (2016) 45(3):669–84. doi:10.1016/j.immuni.2016.08.015
82. Hunninghake GW, Gadek JE, Szapiel SV, Strumpf IJ, Kawanami O, Ferrans VJ, et al. The human alveolar macrophage. In: Lecuit T, editor. Methods in Cell Biology. (Vol. 21), New York: Academic Press (1980). p. 95–112.
83. Green GM. The J. Burns Amberson Lecture – in defense of the lung. Am Rev Respir Dis (1970) 102(5):691–703. doi:10.1164/arrd.1970.102.5.691
84. Schoch OD, Schanz U, Koller M, Nakata K, Seymour JF, Russi EW, et al. BAL findings in a patient with pulmonary alveolar proteinosis successfully treated with GM-CSF. Thorax (2002) 57(3):277–80. doi:10.1136/thorax.57.3.277
85. Greenhill SR, Kotton DN. Pulmonary alveolar proteinosis: a bench-to-bedside story of granulocyte-macrophage colony-stimulating factor dysfunction. Chest (2009) 136(2):571–7. doi:10.1378/chest.08-2943
86. Golde DW, Byers LA, Finley TN. Proliferative capacity of human alveolar macrophage. Nature (1974) 247(5440):373–5. doi:10.1038/247373a0
87. Thomas ED, Ramberg RE, Sale GE, Sparkes RS, Golde DW. Direct evidence for a bone marrow origin of the alveolar macrophage in man. Science (1976) 192(4243):1016–8. doi:10.1126/science.775638
88. Eguiluz-Gracia I, Schultz HH, Sikkeland LI, Danilova E, Holm AM, Pronk CJ, et al. Long-term persistence of human donor alveolar macrophages in lung transplant recipients. Thorax (2016) 71(11):1006–11. doi:10.1136/thoraxjnl-2016-208292
89. Nayak DK, Zhou F, Xu M, Huang J, Tsuji M, Hachem R, et al. Long-term persistence of donor alveolar macrophages in human lung transplant recipients that influences donor-specific immune responses. Am J Transplant (2016) 16(8):2300–11. doi:10.1111/ajt.13819
90. Nicod LP, Lipscomb MF, Toews GB, Weissler JC. Separation of potent and poorly functional human lung accessory cells based on autofluorescence. J Leukoc Biol (1989) 45(5):458–65.
91. van Haarst JM, de Wit HJ, Drexhage HA, Hoogsteden HC. Distribution and immunophenotype of mononuclear phagocytes and dendritic cells in the human lung. Am J Respir Cell Mol Biol (1994) 10(5):487–92. doi:10.1165/ajrcmb.10.5.8179911
92. Ballinger MN, Christman JW. Pulmonary macrophages: overlooked and underappreciated. Am J Respir Cell Mol Biol (2016) 54(1):1–2. doi:10.1165/rcmb.2015-0270ED
93. Yu YA, Hotten DF, Malakhau Y, Volker E, Ghio AJ, Noble PW, et al. Flow cytometric analysis of myeloid cells in human blood, bronchoalveolar lavage, and lung tissues. Am J Respir Cell Mol Biol (2016) 54(1):13–24. doi:10.1165/rcmb.2015-0146OC
94. Bharat A, Bhorade SM, Morales-Nebreda L, McQuattie-Pimentel AC, Soberanes S, Ridge K, et al. Flow cytometry reveals similarities between lung macrophages in humans and mice. Am J Respir Cell Mol Biol (2016) 54(1):147–9. doi:10.1165/rcmb.2015-0147LE
95. Patel VI, Booth JL, Duggan ES, Cate S, White VL, Hutchings D, et al. Transcriptional classification and functional characterization of human airway macrophage and dendritic cell subsets. J Immunol (2017) 198(3):1183–201. doi:10.4049/jimmunol.1600777
96. Toews GB, Vial WC, Dunn MM, Guzzetta P, Nunez G, Stastny P, et al. The accessory cell function of human alveolar macrophages in specific T cell proliferation. J Immunol (1984) 132(1):181–6.
97. Lipscomb MF, Lyons CR, Nunez G, Ball EJ, Stastny P, Vial W, et al. Human alveolar macrophages: HLA-DR-positive macrophages that are poor stimulators of a primary mixed leukocyte reaction. J Immunol (1986) 136(2):497–504.
98. Weissler JC, Lyons CR, Lipscomb MF, Toews GB. Human pulmonary macrophages. Functional comparison of cells obtained from whole lung and by bronchoalveolar lavage. Am Rev Respir Dis (1986) 133(3):473–7. doi:10.1164/arrd.1986.133.3.473
99. Roth MD, Golub SH. Human pulmonary macrophages utilize prostaglandins and transforming growth factor beta 1 to suppress lymphocyte activation. J Leukoc Biol (1993) 53(4):366–71.
100. Blumenthal RL, Campbell DE, Hwang P, DeKruyff RH, Frankel LR, Umetsu DT. Human alveolar macrophages induce functional inactivation in antigen-specific CD4 T cells. J Allergy Clin Immunol (2001) 107(2):258–64. doi:10.1067/mai.2001.112845
101. Lambrecht BN. Alveolar macrophage in the driver’s seat. Immunity (2006) 24(4):366–8. doi:10.1016/j.immuni.2006.03.008
102. Hunninghake GW, Gadek JE, Fales HM, Crystal RG. Human alveolar macrophage-derived chemotactic factor for neutrophils. Stimuli and partial characterization. J Clin Invest (1980) 66(3):473–83. doi:10.1172/JCI109878
103. Kunkel SL, Standiford T, Kasahara K, Strieter RM. Interleukin-8 (IL-8): the major neutrophil chemotactic factor in the lung. Exp Lung Res (1991) 17(1):17–23. doi:10.3109/01902149109063278
104. Strieter RM, Chensue SW, Basha MA, Standiford TJ, Lynch JP, Baggiolini M, et al. Human alveolar macrophage gene expression of interleukin-8 by tumor necrosis factor-alpha, lipopolysaccharide, and interleukin-1 beta. Am J Respir Cell Mol Biol (1990) 2(4):321–6. doi:10.1165/ajrcmb/2.4.321
105. Pinilla-Vera M, Xiong Z, Zhao Y, Zhao J, Donahoe MP, Barge S, et al. Full spectrum of LPS activation in alveolar macrophages of healthy volunteers by whole transcriptomic profiling. PLoS One (2016) 11(7):e0159329. doi:10.1371/journal.pone.0159329
106. Fokkens WJ, Vroom TM, Rijntjes E, Mulder PG. CD-1 (T6), HLA-DR-expressing cells, presumably Langerhans cells, in nasal mucosa. Allergy (1989) 44(3):167–72. doi:10.1111/j.1398-9995.1989.tb02257.x
107. Graeme-Cook F, Bhan AK, Harris NL. Immunohistochemical characterization of intraepithelial and subepithelial mononuclear cells of the upper airways. Am J Pathol (1993) 143(5):1416–22.
108. Nelson DJ, McMenamin C, McWilliam AS, Brenan M, Holt PG. Development of the airway intraepithelial dendritic cell network in the rat from class II major histocompatibility (Ia)-negative precursors: differential regulation of Ia expression at different levels of the respiratory tract. J Exp Med (1994) 179(1):203–12. doi:10.1084/jem.179.1.203
109. Holt PG, Schon-Hegrad MA, Phillips MJ, McMenamin PG. Ia-positive dendritic cells form a tightly meshed network within the human airway epithelium. Clin Exp Allergy (1989) 19(6):597–601. doi:10.1111/j.1365-2222.1989.tb02752.x
110. Tazi A, Bouchonnet F, Grandsaigne M, Boumsell L, Hance AJ, Soler P. Evidence that granulocyte macrophage-colony-stimulating factor regulates the distribution and differentiated state of dendritic cells/Langerhans cells in human lung and lung cancers. J Clin Invest (1993) 91(2):566–76. doi:10.1172/JCI116236
111. Nicod LP, Lipscomb MF, Weissler JC, Lyons CR, Albertson J, Toews GB. Mononuclear cells in human lung parenchyma. Characterization of a potent accessory cell not obtained by bronchoalveolar lavage. Am Rev Respir Dis (1987) 136(4):818–23. doi:10.1164/ajrccm/136.4.818
112. Nicod LP, Galve-de Rochemonteix B, Dayer JM. Dissociation between allogeneic T cell stimulation and interleukin-1 or tumor necrosis factor production by human lung dendritic cells. Am J Respir Cell Mol Biol (1990) 2(6):515–22. doi:10.1165/ajrcmb/2.6.515
113. Streck RJ, Jezewski HM, Rodriguez MI, Hurley EL, Rich GA, Braun KM, et al. A method for isolating human lung macrophages and observations of fluorescent phagocytes from the lungs of habitual cigarette smokers. J Immunol Methods (1994) 174(1–2):67–82. doi:10.1016/0022-1759(94)90011-6
114. Cochand L, Isler P, Songeon F, Nicod LP. Human lung dendritic cells have an immature phenotype with efficient mannose receptors. Am J Respir Cell Mol Biol (1999) 21(5):547–54. doi:10.1165/ajrcmb.21.5.3785
115. van Haarst JM, Hoogsteden HC, de Wit HJ, Verhoeven GT, Havenith CE, Drexhage HA. Dendritic cells and their precursors isolated from human bronchoalveolar lavage: immunocytologic and functional properties. Am J Respir Cell Mol Biol (1994) 11(3):344–50. doi:10.1165/ajrcmb.11.3.8086170
116. Dzionek A, Fuchs A, Schmidt P, Cremer S, Zysk M, Miltenyi S, et al. BDCA-2, BDCA-3, and BDCA-4: three markers for distinct subsets of dendritic cells in human peripheral blood. J Immunol (2000) 165(11):6037–46. doi:10.4049/jimmunol.165.11.6037
117. Demedts IK, Brusselle GG, Vermaelen KY, Pauwels RA. Identification and characterization of human pulmonary dendritic cells. Am J Respir Cell Mol Biol (2005) 32(3):177–84. doi:10.1165/rcmb.2004-0279OC
118. Masten BJ, Olson GK, Tarleton CA, Rund C, Schuyler M, Mehran R, et al. Characterization of myeloid and plasmacytoid dendritic cells in human lung. J Immunol (2006) 177(11):7784–93. doi:10.4049/jimmunol.177.11.7784
119. Ten Berge B, Muskens F, Kleinjan A, Hammad H, Hoogsteden HC, Lambrecht BN, et al. A novel method for isolating dendritic cells from human bronchoalveolar lavage fluid. J Immunol Methods (2009) 351(1–2):13–23. doi:10.1016/j.jim.2009.09.009
120. Tsoumakidou M, Tzanakis N, Papadaki HA, Koutala H, Siafakas NM. Isolation of myeloid and plasmacytoid dendritic cells from human bronchoalveolar lavage fluid. Immunol Cell Biol (2006) 84(3):267–73. doi:10.1111/j.1440-1711.2006.01428.x
121. Lee H, Ruane D, Law K, Ho Y, Garg A, Rahman A, et al. Phenotype and function of nasal dendritic cells. Mucosal Immunol (2015) 8(5):1083–98. doi:10.1038/mi.2014.135
122. Baharom F, Rankin G, Scholz S, Pourazar J, Ahlm C, Blomberg A, et al. Human lung dendritic cells: spatial distribution and phenotypic identification in endobronchial biopsies using immunohistochemistry and flow cytometry. J Vis Exp (2017) (119). doi:10.3791/55222
123. Bigley V, McGovern N, Milne P, Dickinson R, Pagan S, Cookson S, et al. Langerin-expressing dendritic cells in human tissues are related to CD1c+ dendritic cells and distinct from Langerhans cells and CD141high XCR1+ dendritic cells. J Leukoc Biol (2015) 97(4):627–34. doi:10.1189/jlb.1HI0714-351R
124. Breton G, Zheng S, Valieris R, Tojal da Silva I, Satija R, Nussenzweig MC. Human dendritic cells (DCs) are derived from distinct circulating precursors that are precommitted to become CD1c+ or CD141+ DCs. J Exp Med (2016) 213(13):2861–70. doi:10.1084/jem.20161135
125. Demedts IK, Bracke KR, Maes T, Joos GF, Brusselle GG. Different roles for human lung dendritic cell subsets in pulmonary immune defense mechanisms. Am J Respir Cell Mol Biol (2006) 35(3):387–93. doi:10.1165/rcmb.2005-0382OC
126. Harman AN, Bye CR, Nasr N, Sandgren KJ, Kim M, Mercier SK, et al. Identification of lineage relationships and novel markers of blood and skin human dendritic cells. J Immunol (2013) 190(1):66–79. doi:10.4049/jimmunol.1200779
127. Yu CI, Becker C, Metang P, Marches F, Wang Y, Toshiyuki H, et al. Human CD141+ dendritic cells induce CD4+ T cells to produce type 2 cytokines. J Immunol (2014) 193(9):4335–43. doi:10.4049/jimmunol.1401159
128. McGovern N, Schlitzer A, Gunawan M, Jardine L, Shin A, Poyner E, et al. Human dermal CD14(+) cells are a transient population of monocyte-derived macrophages. Immunity (2014) 41(3):465–77. doi:10.1016/j.immuni.2014.08.006
129. Fathi M, Johansson A, Lundborg M, Orre L, Skold CM, Camner P. Functional and morphological differences between human alveolar and interstitial macrophages. Exp Mol Pathol (2001) 70(2):77–82. doi:10.1006/exmp.2000.2344
130. Cai Y, Sugimoto C, Arainga M, Alvarez X, Didier ES, Kuroda MJ. In vivo characterization of alveolar and interstitial lung macrophages in rhesus macaques: implications for understanding lung disease in humans. J Immunol (2014) 192(6):2821–9. doi:10.4049/jimmunol.1302269
131. Misharin AV, Morales-Nebreda L, Mutlu GM, Budinger GR, Perlman H. Flow cytometric analysis of macrophages and dendritic cell subsets in the mouse lung. Am J Respir Cell Mol Biol (2013) 49(4):503–10. doi:10.1165/rcmb.2013-0086MA
132. Wallis A, Spinks K. The diagnosis and management of interstitial lung diseases. BMJ (2015) 350:h2072. doi:10.1136/bmj.h2072
133. Papaiwannou A, Zarogoulidis P, Porpodis K, Spyratos D, Kioumis I, Pitsiou G, et al. Asthma-chronic obstructive pulmonary disease overlap syndrome (ACOS): current literature review. J Thorac Dis (2014) 6(Suppl 1):S146–51. doi:10.3978/j.issn.2072-1439.2014.03.04
134. Aaron SD. Management and prevention of exacerbations of COPD. BMJ (2014) 349:g5237. doi:10.1136/bmj.g5237
135. Thomassen MJ, Barna BP, Wiedemann HP, Farmer M, Ahmad M. Human alveolar macrophage function: differences between smokers and nonsmokers. J Leukoc Biol (1988) 44(5):313–8.
136. King TE Jr, Savici D, Campbell PA. Phagocytosis and killing of Listeria monocytogenes by alveolar macrophages: smokers versus nonsmokers. J Infect Dis (1988) 158(6):1309–16. doi:10.1093/infdis/158.6.1309
137. Rodriguez RJ, White RR, Senior RM, Levine EA. Elastase release from human alveolar macrophages: comparison between smokers and nonsmokers. Science (1977) 198(4314):313–4. doi:10.1126/science.910131
138. Hinman LM, Stevens CA, Matthay RA, Gee JB. Elastase and lysozyme activities in human alveolar macrophages. Effects of cigarette smoking. Am Rev Respir Dis (1980) 121(2):263–71. doi:10.1164/arrd.1980.121.2.263
139. Brown GP, Iwamoto GK, Monick MM, Hunninghake GW. Cigarette smoking decreases interleukin 1 release by human alveolar macrophages. Am J Physiol (1989) 256(2 Pt 1):C260–4.
140. Hinman LM, Stevens C, Matthay RA, Bernard J, Gee L. Angiotensin convertase activities in human alveolar macrophages: effects of cigarette smoking and sarcoidosis. Science (1979) 205(4402):202–3. doi:10.1126/science.221980
141. Pratt SA, Finley TN, Smith MH, Ladman AJ. A comparison of alveolar macrophages and pulmonary surfactant(?) obtained from the lungs of human smokers and nonsmokers by endobronchial lavage. Anat Rec (1969) 163(4):497–507. doi:10.1002/ar.1091630402
142. Harris JO, Swenson EW, Johnson JE III. Human alveolar macrophages: comparison of phagocytic ability, glucose utilization, and ultrastructure in smokers and nonsmokers. J Clin Invest (1970) 49(11):2086–96. doi:10.1172/JCI106426
143. Hoidal JR, Fox RB, LeMarbe PA, Perri R, Repine JE. Altered oxidative metabolic responses in vitro of alveolar macrophages from asymptomatic cigarette smokers. Am Rev Respir Dis (1981) 123(1):85–9. doi:10.1164/arrd.1981.123.1.85
144. Karavitis J, Kovacs EJ. Macrophage phagocytosis: effects of environmental pollutants, alcohol, cigarette smoke, and other external factors. J Leukoc Biol (2011) 90(6):1065–78. doi:10.1189/jlb.0311114
145. Lee JS. Heterogeneity of lung mononuclear phagocytes in chronic obstructive pulmonary disease. J Innate Immun (2012) 4(5–6):489–97. doi:10.1159/000337434
146. Hassett DJ, Borchers MT, Panos RJ. Chronic obstructive pulmonary disease (COPD): evaluation from clinical, immunological and bacterial pathogenesis perspectives. J Microbiol (2014) 52(3):211–26. doi:10.1007/s12275-014-4068-2
147. Upham JW, Xi Y. Dendritic cells in human lung disease: recent advances. Chest (2017) 151(3):668–73. doi:10.1016/j.chest.2016.09.030
148. Hogg JC, Chu F, Utokaparch S, Woods R, Elliott WM, Buzatu L, et al. The nature of small-airway obstruction in chronic obstructive pulmonary disease. N Engl J Med (2004) 350(26):2645–53. doi:10.1056/NEJMoa032158
149. Yoshida T, Tuder RM. Pathobiology of cigarette smoke-induced chronic obstructive pulmonary disease. Physiol Rev (2007) 87(3):1047–82. doi:10.1152/physrev.00048.2006
150. Rennard SI, Fogarty C, Kelsen S, Long W, Ramsdell J, Allison J, et al. The safety and efficacy of infliximab in moderate to severe chronic obstructive pulmonary disease. Am J Respir Crit Care Med (2007) 175(9):926–34. doi:10.1164/rccm.200607-995OC
151. Berenson CS, Kruzel RL, Eberhardt E, Dolnick R, Minderman H, Wallace PK, et al. Impaired innate immune alveolar macrophage response and the predilection for COPD exacerbations. Thorax (2014) 69(9):811–8. doi:10.1136/thoraxjnl-2013-203669
152. Demedts IK, Bracke KR, Van Pottelberge G, Testelmans D, Verleden GM, Vermassen FE, et al. Accumulation of dendritic cells and increased CCL20 levels in the airways of patients with chronic obstructive pulmonary disease. Am J Respir Crit Care Med (2007) 175(10):998–1005. doi:10.1164/rccm.200608-1113OC
153. Vassallo R, Walters PR, Lamont J, Kottom TJ, Yi ES, Limper AH. Cigarette smoke promotes dendritic cell accumulation in COPD; a Lung Tissue Research Consortium Study. Respir Res (2010) 11:45. doi:10.1186/1465-9921-11-45
154. Freeman CM, Martinez FJ, Han MK, Ames TM, Chensue SW, Todt JC, et al. Lung dendritic cell expression of maturation molecules increases with worsening chronic obstructive pulmonary disease. Am J Respir Crit Care Med (2009) 180(12):1179–88. doi:10.1164/rccm.200904-0552OC
155. Kheradmand F, Shan M, Corry DB. Smoking gun: mature dendritic cells in human lung provide clues to chronic obstructive pulmonary disease. Am J Respir Crit Care Med (2009) 180(12):1166–7. doi:10.1164/rccm.200909-1391ED
156. Zanini A, Spanevello A, Baraldo S, Majori M, Della Patrona S, Gumiero F, et al. Decreased maturation of dendritic cells in the central airways of COPD patients is associated with VEGF, TGF-beta and vascularity. Respiration (2014) 87(3):234–42. doi:10.1159/000356749
157. Arellano-Orden E, Calero-Acuna C, Moreno-Mata N, Gomez-Izquierdo L, Sanchez-Lopez V, Lopez-Ramirez C, et al. Cigarette smoke decreases the maturation of lung myeloid dendritic cells. PLoS One (2016) 11(4):e0152737. doi:10.1371/journal.pone.0152737
158. Tsoumakidou M, Tousa S, Semitekolou M, Panagiotou P, Panagiotou A, Morianos I, et al. Tolerogenic signaling by pulmonary CD1c+ dendritic cells induces regulatory T cells in patients with chronic obstructive pulmonary disease by IL-27/IL-10/inducible costimulator ligand. J Allergy Clin Immunol (2014) 134(4):944–54.e8. doi:10.1016/j.jaci.2014.05.045
159. Hoffmann F, Ender F, Schmudde I, Lewkowich IP, Kohl J, Konig P, et al. Origin, localization, and immunoregulatory properties of pulmonary phagocytes in allergic asthma. Front Immunol (2016) 7:107. doi:10.3389/fimmu.2016.00107
160. Hrusch CL, Tjota MY, Sperling AI. The role of dendritic cells and monocytes in the maintenance and loss of respiratory tolerance. Curr Allergy Asthma Rep (2015) 15(1):494. doi:10.1007/s11882-014-0494-9
161. Froidure A, Shen C, Pilette C. Dendritic cells revisited in human allergic rhinitis and asthma. Allergy (2016) 71(2):137–48. doi:10.1111/all.12770
162. Lambrecht BN, Persson EK, Hammad H. Myeloid cells in asthma. Microbiol Spectr (2017) 5(1):739–57. doi:10.1128/microbiolspec.MCHD-0053-2016
163. Lambrecht BN, De Veerman M, Coyle AJ, Gutierrez-Ramos JC, Thielemans K, Pauwels RA. Myeloid dendritic cells induce Th2 responses to inhaled antigen, leading to eosinophilic airway inflammation. J Clin Invest (2000) 106(4):551–9. doi:10.1172/JCI8107
164. Moller GM, Overbeek SE, Van Helden-Meeuwsen CG, Van Haarst JM, Prens EP, Mulder PG, et al. Increased numbers of dendritic cells in the bronchial mucosa of atopic asthmatic patients: downregulation by inhaled corticosteroids. Clin Exp Allergy (1996) 26(5):517–24. doi:10.1046/j.1365-2222.1996.d01-337.x
165. Jahnsen FL, Moloney ED, Hogan T, Upham JW, Burke CM, Holt PG. Rapid dendritic cell recruitment to the bronchial mucosa of patients with atopic asthma in response to local allergen challenge. Thorax (2001) 56(11):823–6. doi:10.1136/thorax.56.11.823
166. Upham JW, Denburg JA, O’Byrne PM. Rapid response of circulating myeloid dendritic cells to inhaled allergen in asthmatic subjects. Clin Exp Allergy (2002) 32(6):818–23. doi:10.1046/j.1365-2222.2002.01375.x
167. Brugha R, Mushtaq N, McCarthy NE, Stagg AJ, Grigg J. Respiratory tract dendritic cells in paediatric asthma. Clin Exp Allergy (2015) 45(3):624–31. doi:10.1111/cea.12457
168. Bratke K, Lommatzsch M, Julius P, Kuepper M, Kleine HD, Luttmann W, et al. Dendritic cell subsets in human bronchoalveolar lavage fluid after segmental allergen challenge. Thorax (2007) 62(2):168–75. doi:10.1136/thx.2006.067793
169. Greer AM, Matthay MA, Kukreja J, Bhakta NR, Nguyen CP, Wolters PJ, et al. Accumulation of BDCA1(+) dendritic cells in interstitial fibrotic lung diseases and Th2-high asthma. PLoS One (2014) 9(6):e99084. doi:10.1371/journal.pone.0099084
170. Lambrecht BN, Hammad H. Taking our breath away: dendritic cells in the pathogenesis of asthma. Nat Rev Immunol (2003) 3(12):994–1003. doi:10.1038/nri1249
171. Lambrecht BN, Hammad H. The immunology of asthma. Nat Immunol (2015) 16(1):45–56. doi:10.1038/ni.3049
172. Mautino G, Henriquet C, Gougat C, Le Cam A, Dayer JM, Bousquet J, et al. Increased expression of tissue inhibitor of metalloproteinase-1 and loss of correlation with matrix metalloproteinase-9 by macrophages in asthma. Lab Invest (1999) 79(1):39–47.
173. Staples KJ, Hinks TS, Ward JA, Gunn V, Smith C, Djukanovic R. Phenotypic characterization of lung macrophages in asthmatic patients: overexpression of CCL17. J Allergy Clin Immunol (2012) 130(6):1404–12.e7. doi:10.1016/j.jaci.2012.07.023
174. Carmona EM, Kalra S, Ryu JH. Pulmonary sarcoidosis: diagnosis and treatment. Mayo Clin Proc (2016) 91(7):946–54. doi:10.1016/j.mayocp.2016.03.004
175. Terao I, Hashimoto S, Horie T. Effect of GM-CSF on TNF-alpha and IL-1-beta production by alveolar macrophages and peripheral blood monocytes from patients with sarcoidosis. Int Arch Allergy Immunol (1993) 102(3):242–8. doi:10.1159/000236532
176. Ziegenhagen MW, Benner UK, Zissel G, Zabel P, Schlaak M, Muller-Quernheim J. Sarcoidosis: TNF-alpha release from alveolar macrophages and serum level of sIL-2R are prognostic markers. Am J Respir Crit Care Med (1997) 156(5):1586–92. doi:10.1164/ajrccm.156.5.97-02050
177. Betensley A, Sharif R, Karamichos D. A systematic review of the role of dysfunctional wound healing in the pathogenesis and treatment of idiopathic pulmonary fibrosis. J Clin Med (2016) 6(1):2. doi:10.3390/jcm6010002
178. Rennard SI, Hunninghake GW, Bitterman PB, Crystal RG. Production of fibronectin by the human alveolar macrophage: mechanism for the recruitment of fibroblasts to sites of tissue injury in interstitial lung diseases. Proc Natl Acad Sci U S A (1981) 78(11):7147–51. doi:10.1073/pnas.78.11.7147
179. Prasse A, Pechkovsky DV, Toews GB, Jungraithmayr W, Kollert F, Goldmann T, et al. A vicious circle of alveolar macrophages and fibroblasts perpetuates pulmonary fibrosis via CCL18. Am J Respir Crit Care Med (2006) 173(7):781–92. doi:10.1164/rccm.200509-1518OC
180. Tsoumakidou M, Karagiannis KP, Bouloukaki I, Zakynthinos S, Tzanakis N, Siafakas NM. Increased bronchoalveolar lavage fluid CD1c expressing dendritic cells in idiopathic pulmonary fibrosis. Respiration (2009) 78(4):446–52. doi:10.1159/000226244
181. Marchal-Somme J, Uzunhan Y, Marchand-Adam S, Kambouchner M, Valeyre D, Crestani B, et al. Dendritic cells accumulate in human fibrotic interstitial lung disease. Am J Respir Crit Care Med (2007) 176(10):1007–14. doi:10.1164/rccm.200609-1347OC
182. Lommatzsch M, Bratke K, Bier A, Julius P, Kuepper M, Luttmann W, et al. Airway dendritic cell phenotypes in inflammatory diseases of the human lung. Eur Respir J (2007) 30(5):878–86. doi:10.1183/09031936.00036307
183. Spiteri MA, Clarke SW, Poulter LW. Phenotypic and functional changes in alveolar macrophages contribute to the pathogenesis of pulmonary sarcoidosis. Clin Exp Immunol (1988) 74(3):359–64.
184. Krombach F, Gerlach JT, Padovan C, Burges A, Behr J, Beinert T, et al. Characterization and quantification of alveolar monocyte-like cells in human chronic inflammatory lung disease. Eur Respir J (1996) 9(5):984–91. doi:10.1183/09031936.96.09050984
185. Zaba LC, Smith GP, Sanchez M, Prystowsky SD. Dendritic cells in the pathogenesis of sarcoidosis. Am J Respir Cell Mol Biol (2010) 42(1):32–9. doi:10.1165/rcmb.2009-0033TR
186. Marchal-Somme J, Uzunhan Y, Marchand-Adam S, Valeyre D, Soumelis V, Crestani B, et al. Cutting edge: nonproliferating mature immune cells form a novel type of organized lymphoid structure in idiopathic pulmonary fibrosis. J Immunol (2006) 176(10):5735–9. doi:10.4049/jimmunol.176.10.5735
187. Freynet O, Marchal-Somme J, Jean-Louis F, Mailleux A, Crestani B, Soler P, et al. Human lung fibroblasts may modulate dendritic cell phenotype and function: results from a pilot in vitro study. Respir Res (2016) 17:36. doi:10.1186/s12931-016-0345-4
188. Forman JD, Klein JT, Silver RF, Liu MC, Greenlee BM, Moller DR. Selective activation and accumulation of oligoclonal V beta-specific T cells in active pulmonary sarcoidosis. J Clin Invest (1994) 94(4):1533–42. doi:10.1172/JCI117494
189. Mathew S, Bauer KL, Fischoeder A, Bhardwaj N, Oliver SJ. The anergic state in sarcoidosis is associated with diminished dendritic cell function. J Immunol (2008) 181(1):746–55. doi:10.4049/jimmunol.181.1.746
190. Broos CE, van Nimwegen M, Hoogsteden HC, Hendriks RW, Kool M, van den Blink B. Granuloma formation in pulmonary sarcoidosis. Front Immunol (2013) 4:437. doi:10.3389/fimmu.2013.00437
191. Sasindran SJ, Torrelles JB. Mycobacterium tuberculosis infection and inflammation: what is beneficial for the host and for the bacterium? Front Microbiol (2011) 2:2. doi:10.3389/fmicb.2011.00002
192. Engele M, Stossel E, Castiglione K, Schwerdtner N, Wagner M, Bolcskei P, et al. Induction of TNF in human alveolar macrophages as a potential evasion mechanism of virulent Mycobacterium tuberculosis. J Immunol (2002) 168(3):1328–37. doi:10.4049/jimmunol.168.3.1328
193. Buettner M, Meinken C, Bastian M, Bhat R, Stossel E, Faller G, et al. Inverse correlation of maturity and antibacterial activity in human dendritic cells. J Immunol (2005) 174(7):4203–9. doi:10.4049/jimmunol.174.7.4203
194. de Jong MD, Simmons CP, Thanh TT, Hien VM, Smith GJ, Chau TN, et al. Fatal outcome of human influenza A (H5N1) is associated with high viral load and hypercytokinemia. Nat Med (2006) 12(10):1203–7. doi:10.1038/nm1477
195. Hayden FG, Fritz R, Lobo MC, Alvord W, Strober W, Straus SE. Local and systemic cytokine responses during experimental human influenza A virus infection. Relation to symptom formation and host defense. J Clin Invest (1998) 101(3):643–9. doi:10.1172/JCI1355
196. Jorens PG, Van Damme J, De Backer W, Bossaert L, De Jongh RF, Herman AG, et al. Interleukin 8 (IL-8) in the bronchoalveolar lavage fluid from patients with the adult respiratory distress syndrome (ARDS) and patients at risk for ARDS. Cytokine (1992) 4(6):592–7. doi:10.1016/1043-4666(92)90025-M
197. Huang Y, Zhu W, Zeng X, Li S, Li X, Lu C. Innate and adaptive immune responses in patients with pandemic influenza A(H1N1)pdm09. Arch Virol (2013) 158(11):2267–72. doi:10.1007/s00705-013-1692-9
198. Gill MA, Palucka AK, Barton T, Ghaffar F, Jafri H, Banchereau J, et al. Mobilization of plasmacytoid and myeloid dendritic cells to mucosal sites in children with respiratory syncytial virus and other viral respiratory infections. J Infect Dis (2005) 191(7):1105–15. doi:10.1086/428589
199. Gill MA, Long K, Kwon T, Muniz L, Mejias A, Connolly J, et al. Differential recruitment of dendritic cells and monocytes to respiratory mucosal sites in children with influenza virus or respiratory syncytial virus infection. J Infect Dis (2008) 198(11):1667–76. doi:10.1086/593018
200. Oshansky CM, Gartland AJ, Wong SS, Jeevan T, Wang D, Roddam PL, et al. Mucosal immune responses predict clinical outcomes during influenza infection independently of age and viral load. Am J Respir Crit Care Med (2014) 189(4):449–62. doi:10.1164/rccm.201309-1616OC
201. van Riel D, Leijten LM, van der Eerden M, Hoogsteden HC, Boven LA, Lambrecht BN, et al. Highly pathogenic avian influenza virus H5N1 infects alveolar macrophages without virus production or excessive TNF-alpha induction. PLoS Pathog (2011) 7(6):e1002099. doi:10.1371/journal.ppat.1002099
202. Becker S, Quay J, Soukup J. Cytokine (tumor necrosis factor, IL-6, and IL-8) production by respiratory syncytial virus-infected human alveolar macrophages. J Immunol (1991) 147(12):4307–12.
203. Remark R, Becker C, Gomez JE, Damotte D, Dieu-Nosjean MC, Sautes-Fridman C, et al. The non-small cell lung cancer immune contexture. A major determinant of tumor characteristics and patient outcome. Am J Respir Crit Care Med (2015) 191(4):377–90. doi:10.1164/rccm.201409-1671PP
204. Fridman WH, Pages F, Sautes-Fridman C, Galon J. The immune contexture in human tumours: impact on clinical outcome. Nat Rev Cancer (2012) 12(4):298–306. doi:10.1038/nrc3245
205. Dieu-Nosjean MC, Antoine M, Danel C, Heudes D, Wislez M, Poulot V, et al. Long-term survival for patients with non-small-cell lung cancer with intratumoral lymphoid structures. J Clin Oncol (2008) 26(27):4410–7. doi:10.1200/JCO.2007.15.0284
206. Goc J, Germain C, Vo-Bourgais TK, Lupo A, Klein C, Knockaert S, et al. Dendritic cells in tumor-associated tertiary lymphoid structures signal a Th1 cytotoxic immune contexture and license the positive prognostic value of infiltrating CD8+ T cells. Cancer Res (2014) 74(3):705–15. doi:10.1158/0008-5472.CAN-13-1342
207. Mu CY, Huang JA, Chen Y, Chen C, Zhang XG. High expression of PD-L1 in lung cancer may contribute to poor prognosis and tumor cells immune escape through suppressing tumor infiltrating dendritic cells maturation. Med Oncol (2011) 28(3):682–8. doi:10.1007/s12032-010-9515-2
208. Perrot I, Blanchard D, Freymond N, Isaac S, Guibert B, Pacheco Y, et al. Dendritic cells infiltrating human non-small cell lung cancer are blocked at immature stage. J Immunol (2007) 178(5):2763–9. doi:10.4049/jimmunol.178.5.2763
209. Valecha GK, Vennepureddy A, Ibrahim U, Safa F, Samra B, Atallah JP. Anti-PD-1/PD-L1 antibodies in non-small cell lung cancer: the era of immunotherapy. Expert Rev Anticancer Ther (2017) 17(1):47–59. doi:10.1080/14737140.2017.1259574
210. ImmGen C. Open-source ImmGen: mononuclear phagocytes. Nat Immunol (2016) 17(7):741. doi:10.1038/ni.3478
Keywords: respiratory, pulmonary, monocytes, dendritic cells, macrophages, bronchoalveolar lavage, lung tissue, bronchial tissue
Citation: Baharom F, Rankin G, Blomberg A and Smed-Sörensen A (2017) Human Lung Mononuclear Phagocytes in Health and Disease. Front. Immunol. 8:499. doi: 10.3389/fimmu.2017.00499
Received: 15 February 2017; Accepted: 11 April 2017;
Published: 01 May 2017
Edited by:
Nils Yngve Lycke, University of Gothenburg, SwedenReviewed by:
Carlos Alberto Guzman, National Research Centre for Biotechnology, GermanyCopyright: © 2017 Baharom, Rankin, Blomberg and Smed-Sörensen. This is an open-access article distributed under the terms of the Creative Commons Attribution License (CC BY). The use, distribution or reproduction in other forums is permitted, provided the original author(s) or licensor are credited and that the original publication in this journal is cited, in accordance with accepted academic practice. No use, distribution or reproduction is permitted which does not comply with these terms.
*Correspondence: Anna Smed-Sörensen, YW5uYS5zbWVkLnNvcmVuc2VuQGtpLnNl
Disclaimer: All claims expressed in this article are solely those of the authors and do not necessarily represent those of their affiliated organizations, or those of the publisher, the editors and the reviewers. Any product that may be evaluated in this article or claim that may be made by its manufacturer is not guaranteed or endorsed by the publisher.
Research integrity at Frontiers
Learn more about the work of our research integrity team to safeguard the quality of each article we publish.