- 1Laboratory Cancer, Immune Control and Escape, Cordeliers Research Center, INSERM UMRS1138, Paris, France
- 2UMRS1138, University Pierre and Marie Curie, Paris, France
- 3UMRS1138, University Paris Descartes, Paris, France
- 4Division of Hematology, Oncology and Immunology, The Tisch Cancer Institute, Icahn School of Medicine at Mount Sinai, New York, NY, USA
It is now admitted that the immune system plays a major role in tumor control. Besides the existence of tumor-specific T cells and B cells, many studies have demonstrated that high numbers of tumor-infiltrating lymphocytes are associated with good clinical outcome. In addition, not only the density but also the organization of tumor-infiltrating immune cells has been shown to determine patient survival. Indeed, more and more studies describe the development within the tumor microenvironment of tertiary lymphoid structures (TLS), whose presence has a positive impact on tumor prognosis. TLS are transient ectopic lymphoid aggregates displaying the same organization and functionality as canonical secondary lymphoid organs, with T-cell-rich and B-cell-rich areas that are sites for the differentiation of effector and memory T cells and B cells. However, factors favoring the emergence of such structures within tumors still need to be fully characterized. In this review, we survey the state of the art of what is known about the general organization, induction, and functionality of TLS during chronic inflammation, and more especially in cancer, with a particular focus on the B-cell compartment. We detail the role played by TLS B cells in anti-tumor immunity, both as antigen-presenting cells and tumor antigen-specific antibody-secreting cells, and raise the question of the capacity of chemotherapeutic and immunotherapeutic agents to induce the development of TLS within tumors. Finally, we explore how to take advantage of our knowledge on TLS B cells to develop new therapeutic tools.
Introduction
Tumors represent a complex microenvironment that not only includes tumor cells but also stromal cells, blood vessels, and different immune cell populations, reflecting the capacity of the immune system to sense tumor cells (1). The major role played by the immune system in tumor surveillance and control has been extensively documented. Since the discovery of T cells, B cells, and antibodies specific for tumor antigens (2–6), several clinical studies have clearly demonstrated that a high density of T-cell or B-cell subsets within the tumor microenvironment is associated with increased patient survival (1, 7, 8), such as in colorectal cancer (9), primary cutaneous melanoma (10), breast cancer (11–14), non-small cell lung cancer (NSCLC) (15, 16), head and neck cancer (17), and ovarian cancer (18–20).
Regarding the development of adaptive anti-tumor immune responses (but it is also true for any other kind of disorders), the dogma was that infiltrating anti-tumor immune cells are educated to recognize tumor antigens and proliferate at distance of the tumor site, in conventional secondary lymphoid organs (SLOs), and are then recruited to the tumor microenvironment to exert their anti-tumor activity. SLOs are highly specialized tissues that arise at predetermined locations during embryogenesis and persist all over the life. They include lymph nodes (LNs), spleen and mucosal-associated lymphoid tissues (MALTs), namely Peyer’s patches, tonsils, and nasal-associated lymphoid tissues (NALTs) or bronchus-associated lymphoid tissues (BALTs). However, it is now established that immune responses can also take place at distance of SLOs, in tertiary lymphoid structures (TLS), also called tertiary lymphoid organs (TLOs) or, in the case of the lung, (T)i-BALTs, for (tumor-) induced BALTs (21–23). As opposed to SLOs, TLS are defined as transient ectopic lymphoid organizations that can develop after birth in non-lymphoid tissues, in situations of chronic inflammation. This phenomenon is usually termed lymphoid “neogenesis” by opposition to lymphoid organogenesis, which refers to SLO development (24, 25). TLS have already been observed in several infectious diseases, graft rejection, autoimmune disorders [including rheumatoid arthritis (RA) and multiple sclerosis], allergy, and cancer (25–27). They have also been described in different lung inflammatory diseases, such as fibrosis, pneumonia, hypersensitivity pneumonitis, diffuse pan-bronchiolitis, and tobacco-induced inflammation (28), and in the adventitia of human atherosclerotic arteries (29–31). It has been proposed that the presence of TLS can be observed in every situation when the inflammatory response initiated is unable to eradicate the associated antigen (32). This inability might be the result of an efficient escape of a pathogen antigen to immune surveillance, and/or of a permanent replenishment of a self or tumor antigen by the tissue. The difficulty in eradicating antigens would lead to a chronic inflammation, characterized by a sustained infiltration of immune cells, and to the development of TLS as sites for the generation of a local more efficient immune response. Because these structures turn off after the resolution of the inflammation, they have been proposed as a genuine inflammation marker (27). In autoimmune diseases and transplantation, the presence of TLS is respectively associated with disease exacerbation, poor outcome (33), chronic rejection, and anti-graft responses (34–37). On the opposite, T- and B-cell responses initiated within TLS during infections are associated with pathogen clearance, increased survival, and reduced morbidity (21). Finally, an increasing number of studies demonstrated over the past decades that the presence of TLS in the tumor microenvironment is associated with anti-tumor immune responses and prolonged patient survival (22, 27, 38–44). Yet, the events that influence immune cell infiltration and TLS formation are still incompletely understood.
In this review, we discuss the factors favoring the recruitment of immune cells and their organization in TLS by comparison with what is known about conventional SLOs. We detail the role played by TLS B cells in anti-tumor immunity, both as tumor antigen-specific antibody-secreting cells and antigen-presenting cells (APCs). Finally, we raise the question of the capacity of chemotherapeutic agents and/or new immunotherapeutic drugs to induce the development of TLS within tumors, and how to take advantage of what we know now from these structures to develop new therapeutic tools.
TLS Organization and Formation: How Far a TLS is Like a LN?
TLS Display the Same Organization as Conventional SLOs
LN, a prototypical SLO
Lymph nodes are encapsulated structures mainly composed of T cells and B cells, dendritic cells (DCs), a network of fibroblastic reticular cells (FRCs) and fibers (45), and two specialized lymphatic and vascular systems: lymphatic vessels and high endothelial venules (HEVs), the latter specifically allowing the recruitment of naive T and B lymphocytes, but also plasmacytoid DCs (pDCs) and the precursors of conventional (CD11chi) DCs (pre-cDCs) into the LN [reviewed in Ref. (46)].
Within LNs, B, and T cells are segregated into distinct functional areas: a B-cell area mainly composed of densely packed B cells and follicular DCs (FDCs) forming follicles, and a T-cell area mainly composed of less dense accumulation of T cells and DCs (25). While DCs prime T cells through the presentation of antigen-derived peptides within major histocompatibility complex (MHC) class I and II molecules, FDCs shape the B-cell response by presenting unprocessed antigens in the form of antigen-antibody complexes that they trap through their complement or fragment constant receptors (FcRs). After antigen encounter, activated B cells organize into a germinal center (GC), which is a site of active B-cell proliferation, class switch recombination (CSR), and somatic hyper-mutation (SHM), allowing the generation of B-cell clones highly specific for the antigen. A particular subset of T helper (Th) cells, namely T follicular helper (Tfh) cells, plays also a major role in GC responses, by delivering key signals for GC B-cell survival and differentiation [reviewed in Ref. (47)].
TLS, similarities and differences with LNs
Tertiary lymphoid structures were shown to display an overall organization very similar to that observed in SLOs. In NSCLC for example, we showed that TLS were composed of a T-cell-rich area, characterized by T cells forming clusters with mature DCs expressing the maturation marker DC-Lamp, and CD20+ B cells, organized in B-cell follicle (Figure 1) (22, 39). Like in conventional LNs, B-cell follicles were composed of a mantle of IgD+ naive B cells, surrounding a GC identified by highly proliferating Ki67+ B cells and a network of CD21+ FDCs. Expression of activation-induced cytidine deaminase (AID) and of the transcription repressor Bcl6 by GC B cells, associated with CSR and SHM activities, was reported in several studies (32, 39, 40, 48). Finally, the presence of CD68+ tingible-body macrophages (specialized in the clearance of apoptotic B cells) and CD3+ CD4+ CXCL13+ Tfh cells could also be detected within TLS B-cell follicles (39, 43), as well as that of differentiated plasma cells (PCs) (39, 49, 50).
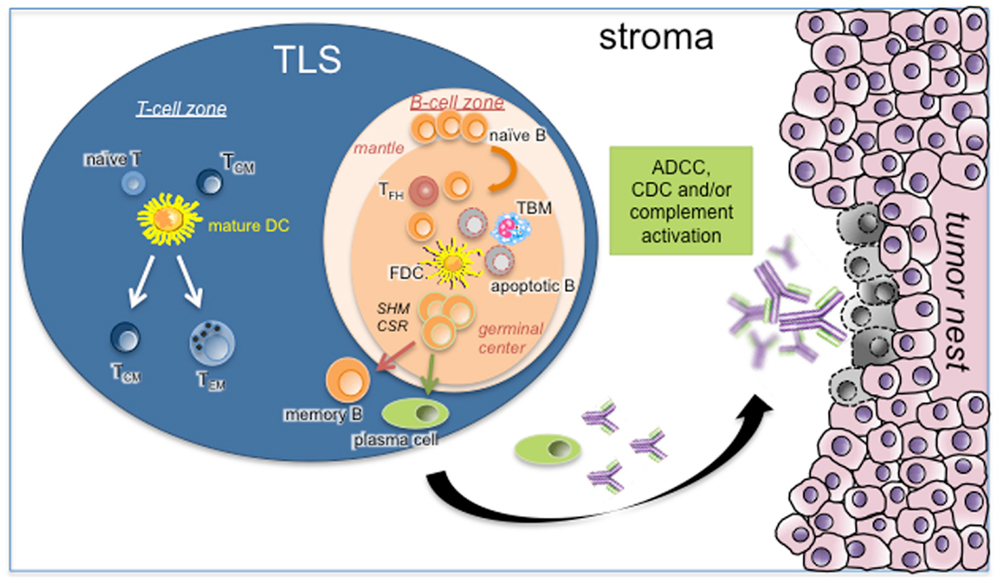
Figure 1. Role of TLS B cells in the initiation of a protective anti-tumor immune response. As in canonical SLOs, TLS may represent a critical site where specific T and B cells can undergo terminal differentiation into effector cells in the T- and B-cell-rich areas, respectively. In the latter case, germinal center B cells establish intimate interactions with FDCs and Tfh in the course of differentiation into memory B cells and PCs. This step is under the activation of CSR and SHM machineries. Apoptotic B cells will be phagocytosed by TBM whereas fully differentiated B cells will leave TLS B-cell follicle to the tumor stroma. After binding to their cognate tumor antigens, antibodies will elicit ADCC, CDC, and/or complement activation. Abbreviations: ADCC, antibody-dependent cellular cytotoxicity; CDC, complement-dependent cytotoxicity; CSR, class switch recombination; FDC, follicular dendritic cell; SHM, somatic hyper-mutation; TBM, tingible-body macrophage; TCM, central-memory T cell; TEM, effector-memory T cell; Tfh, follicular helper T cell; TLS, tertiary lymphoid structure.
Like in SLOs, the presence of TLS is frequently associated with that of HEVs. HEVs can be easily distinguished from other types of blood vessels, thanks to a cuboidal endothelial cell lining and a set of molecules expressed by endothelial cells: peripheral node addressins (PNAd), which interact with L-selectin (CD62L) on lymphocytes and allow lymphocyte tethering to HEV endothelial cells and rolling along the HEV lumen; chemokines, including CCL19, CCL21, CXCL10, CXCL12, and CXCL13, known to determine the trafficking of lymphocytes but also their segregation into distinct areas within the LN (detailed below); and integrins and immunoglobulin superfamily adhesion molecules, which support the binding and migration of lymphocytes across HEVs (51, 52). Because HEVs are crucial for the recruitment of lymphocytes into LNs, they are also supposed to play a major role in tumor infiltration by immune cells and TLS formation (40, 53–56). Indeed, we observed in lung cancer that most TLS T cells and B cells (except GC B cells) expressed the PNAd ligand CD62L (53). Similarly, Martinet et al. demonstrated that the presence of HEVs in melanoma tumors was associated with high levels of lymphocyte infiltration (54). They notably showed that lymphocytes specifically infiltrated HEV-rich areas of melanoma tumors, and that the density of HEVs was strongly correlated with DC-Lamp+ mature DC density and CD3+, CD8+, and CD20+ tumor-infiltrating lymphocyte densities (55).
Interestingly, a major difference between SLOs and TLS is the absence of natural killer (NK) cells within TLS, as demonstrated in NSCLC using the NKp46 marker (57). To date, the presence of NK cells has never been reported in TLS regardless of the pathology concerned (cancer, autoimmunity, transplantation, or infectious diseases). In LNs, NK cells colocalize with DCs in the T-cell area (58), and form clusters with DCs and T cells getting primed shortly after infection, during the early phases of the adaptive immune response (59–61). On one hand, activated DCs can enhance NK cell proliferation, IFNγ secretion, and cytotoxic functions through the production of cytokines such as IL-15, IL-12, IL-18, and IFNα/β. On the other end, NK cells can either promote DC maturation through the production of IFNγ and TNFα, or kill immature DCs [reviewed in Ref. (62)]. Similarly, NK cells are thought to provide an early source of IFNγ that is needed to polarize the T-cell response (63), while newly primed Ag-specific CD4+ T cells can directly activate NK cells through the secretion of IL-2 (60, 64). These interactions are supposed to play a crucial role in shaping both innate and adaptive immune responses in LNs (65). The fact that intra-tumoral NK cells are not observed within cancer-associated TLS (57, 66) indicates that tumor-infiltrating NK cells may not influence T-cell response, and are not the cellular source of IFNγ detected within TLS.
TLS Neogenesis, What Can We Learn from LN Organogenesis?
LN development
The development of LNs during embryogenesis depends on interactions between a unique subset of CD45+CD4+CD3- hematopoietic cells, named lymphoid tissue-inducer (LTi) cells and resident stromal cells, qualified as lymphoid tissue-organizer (LTo) cells (67). In mice, van de Pavert et al. showed that the initial interaction between these two cell subsets was triggered by CXCL13 produced by stromal LTo cells at sites of lymphoid tissue formation, inducible upon retinoic acid and neuronal stimulation, and able to attract CXCR5-expressing LTi cells (68). The engagement of lymphotoxin (LT), a member of the TNF family expressed as an LTα1β2 heterotrimer on LTi cells, with lymphotoxin β receptor (LTβR) on stromal cells, then leads to the activation of two different pathways of the NF-κB transcription factor, resulting in the expression of high levels of the intercellular and vascular cell adhesion molecules ICAM-1 and VCAM-1, production of lymphoid chemokines (CCL19, CCL21, and CXCL13) and cytokines, and the development of both HEVs and lymphatic vessels (51, 69–72). The local expression of lymphoid chemokines by stromal LTo cells allows the subsequent attraction and trafficking of B, T, and DCs to the LN (67, 68). These three homeostatic chemokines not only govern the recruitment but also the positioning of immune cells within the LN. While CCL19 and CCL21 are expressed by the T-cell-zone stromal cells, and interact both with CCR7 to orchestrate the homing of naive and central-memory T cells and DCs to the T-cell compartment, CXCL13 is expressed by the B-cell-zone stromal cells, and allows the recruitment of CXCR5+ B cells and Tfh to the follicular compartment (73). Of note, CXCL13 was also shown to promote LTα1β2 expression on the surface of LTi cells, which amplifies the LTβR signaling in stromal LTo cells, resulting in a positive and stable feedback loop (67). Another feedback loop involving CXCL13 and LTα1β2 was also observed between FDCs and B cells, which are themselves strong producers of LTα and LTβ (74). LTα1β2 expression by B cells plays notably a major role in the establishment of the FDC network and in B-cell follicle organization. However, it has been demonstrated that membrane-bound LTα1β2 on B cells was a key regulator of CXCL13 production by FDCs, which in turn induces an upregulation of LTα1β2 on B cells (75).
HEV endothelial cells also express LTβR, and several studies have demonstrated that continuous engagement of LTβR on HEVs by LTα1β2+ cells is critical for the induction and maintenance of HEV gene expression and HEV endothelial cell morphology (70, 76). In mice, B cells (71, 77) and CD11c+ DCs (78) were both demonstrated as major sources of LTα1β2 for HEV regulation in lymphoid tissues. CD11c+ DCs in particular were shown to play an essential role in lymphocyte homing to the LNs, as a direct or indirect source of pro-angiogenic factors favoring the development of HEVs (79, 80). Webster et al. notably showed in immunized mice that CD11c+ DCs were able to induce increased vascular endothelial growth factor (VEGF) levels in LNs, favoring endothelial cell proliferation including HEV endothelial cell growth, associated with increased immune cell entry (81). In a further study, the same group showed that CD11c+ DC-induced vascular growth in this model is at least in part mediated by the production of IL-1β by CD11c+ DCs that can directly stimulate FRC proliferation and VEGF production (82). Similarly, activated B cells are able to secrete the pro-lymphangiogenic factor VEGF-A, and to drive lymphangiogenesis in inflamed LNs (83).
TLS neogenesis
The physiological events that lead to TLS formation are still unclear, in particular in cancer. Numerous studies in inflammatory disorders tried to determine if the same cell types and/or molecules involved in lymphoid organogenesis, could similarly be implicated in TLS neogenesis. If the presence of the bona fide LTi cells does not seem to be required for TLS induction in CCL21-transgenic LTi-deficient mice (84), many studies showed that key molecules, such as LT, CCL21, or CXCL13 chemokines, play also a major role in lymphoid neogenesis. Grabner et al. for example demonstrated in a mouse model of atherosclerosis that the activation through LTβR of medial smooth muscle cells (SMCs) in the abdominal aorta led to the expression of CCL19, CCL21, CXCL13, and CXCL16 chemokines, which in turn triggered the recruitment of lymphocytes to the adventitia and the development of TLS (85). In this study, authors suggest that the cellular source of LT would be CD11c+CD68+Ly6Clo monocytes that accumulate in atherosclerotic plaques. Interestingly, Thaunat et al. showed in a rat model of chronic allograft rejection based on aorta transplantation that macrophages, together with CD4+ and CD8+ T cells, are one of the first immune cell subsets infiltrating the adventitia few days after engraftment, simultaneously with the acquisition by some endothelial cells of a HEV-like phenotype (37). The same group demonstrated that macrophages, in particular M1-polarized pro-inflammatory macrophages that express high levels of LTα and TNFα, can act as LTi cells in diseased arteries and confer a LTo phenotype to vascular SMCs, but that this mechanism is TNF receptor 1/2 (TNFR1/2) signaling-dependent and LTβR-independent (86). They explained the discrepancies observed between their study and others claiming that LTβR is crucial for the formation of TLS by the major role played by LTβR in inducing CXCL13 production, cytokine not indispensable to induce TLS in their model. Similarly, Moyron-Quiroz et al. were able to observe the formation of i-BALTs in mice upon influenza infection even in the absence of LTα, but not as well organized as the ones generated in wild-type mice, with B-cell areas devoid of FDCs, few intermixed T cells, and no expression of PNAd (21). Finally, in human NSCLC, we were able to detect the expression of a set of chemoattractants associated with T lymphocytes in TLS, including the previously cited chemokines CCL19, CCL21, and CXCL13 (53).
In accordance with the aforementioned role of DCs in lymphocyte homing to LNs, it was suggested that DCs could promote lymphocyte tumor infiltration through the regulation of tumor HEVs (87). Indeed, Martinet et al. showed that LTβ was specifically overexpressed in breast cancers characterized by a high density of HEVs, and that the major producers of LTβ in the tumor microenvironment were DCs. They notably showed that LTβ expression correlated with that of HEV-associated chemokines, and that DC-Lamp+ DC density correlated with HEV density, lymphocyte infiltration, and favorable clinical outcome (88). In mice, CD11c+ DCs were shown to play a major role in the maintenance of i-BALTs. Halle et al. for example demonstrated a massive infiltration of CD11c+ DCs followed by i-BALT neogenesis in the lung of modified vaccinia virus Ankara (MVA)-infected mice, and that the depletion of these cells after i-BALT formation resulted in strong i-BALT size reduction (89). Similarly, GeurtsvanKessel et al. showed in a mouse model of influenza virus infection that CD11chi DCs are essential for the maintenance of virus i-BALTs after virus clearance, as DC depletion resulted in reduced i-BALT numbers, associated with reduced B-cell density within the remaining clusters, and altered humoral immunity (49). This effect was associated with LTβ expression by DCs, as blocking LTβR signaling had the same effect as DC depletion. Inhibition of LTβR signaling was associated with reduced secretion of CXCL13, suggesting that DCs maintain i-BALTs by providing a continuous source of LTβ that is necessary for high CXCL13 production and retention of B cells. Interestingly, authors showed that repeated injections of DCs also led to the development of i-BALTs into naïve mice suggesting that DCs may have a direct action in lymphoid neogenesis. Finally, Marinkovic et al. showed in a CCL21-transgenic-LTi-deficient mouse model that the formation of thyroid-associated TLS started by the clustering of host tissue DCs with newly entered mature CD3+ CD4+ T cells, followed by the formation of PNAd+ HEVs (84).
It has been suggested that Th17 cells could also play a major role in TLS induction. Th17 cells and associated cytokines are elevated in a number of autoimmune and inflammatory diseases, in which their function is well documented [reviewed in Ref. (90)]. Their role in cancer however is more uncertain, due to their strong plasticity [reviewed in Ref. (91)]. The putative role of Th17 cells in TLS development notably comes from the observation that Th17 cells share many developmental and effector markers with LTi cells. It includes the nuclear hormone receptor retinoic acid-related orphan receptor γt (RORγt), which promotes the production of IL-17 and IL-22 by Th17 cells, LTi cells, and other RORγt+ innate lymphoid cells (ILCs); the chemokine receptor CCR6, which favors the recruitment of Th17 cells to the site of inflammation in response to its ligand CCL20; and the ligand-dependent transcription factor, aryl hydrocarbon receptor (AHR). Above all, both cell subsets express LTα1β2 on the cell membrane [reviewed in Ref. (92)]. The role of Th17 cells in TLS induction was particularly studied in mouse models of lung infection or autoimmunity. Rangel-Moreno et al. for example demonstrated in mice sensitized with LPS that i-BALT formation was dependent on IL-17 production by T cells, including Th17 cells and γδ T cells, while LTi cells were not necessary (93). Opposite results were obtained by Fleige et al., who showed in MVA-infected mice that i-BALT formation was independent of IL-17 (94). The same authors further deciphered this duality in another study, in which they suggested that the role played by IL-17 in i-BALT development depends on the type of inflammation-inducing pathogen (95). Actually, they showed that while mice infected with MVA developed in their lungs highly organized i-BALTs, with densely packed B-cell follicles characterized by CXCL12-producing stromal cells and a network of CXCL13+ FDCs, mice infected with P. aeruginosa developed i-BALTs with B-cell follicles that contained CXCL12-expressing stromal cells but lacked FDCs. Authors demonstrated that in the case of P. aeruginosa infection, IL-17 drives the differentiation of lung stroma toward CXCL12-expressing cells, which allows B-cell recruitment and follicle formation even in the absence of FDCs. Again, the major source of IL-17 was identified here as being γδ T cells within i-BALTs. Still in favor of IL-17 involvement in TLS induction, Peters et al. showed in a mouse model of multiple sclerosis [experimental autoimmune encephalomyelitis (EAE)] that the transfer of differentiated myelin oligodendrocyte glycoprotein (MOG)-specific Th17 cells was more capable of inducing ectopic lymphoid structures than other Th subtypes (96). Ectopic lymphoid structure development was notably associated here with the acquired expression by transferred Th17 cells of Tfh cell-associated molecules, such as CXCR5, ICOS, and Bcl6, and dependent on the expression of IL-17 by Th17 cells. Finally, in humans, Deteix et al. suggested that Th17 cells, and IL-17 and IL-21 secretion by these cells, promote lymphoid neogenesis within human renal grafts, and are associated with fast chronic rejection (97). They notably showed that while rejected renal grafts from slow progressor patients were characterized by the presence of IL-10-secreting regulatory T cells (Tregs), which positively correlated with graft survival, rejected renal grafts from fast progressor patients were characterized by the presence of IL-17- and IL-21-secreting Th17 cells, which negatively correlated with graft survival. In addition, the presence of Th17 cells correlated with the presence of AID+ GC B cells suggesting that Th17 cells promote intragraft neogenesis through the production of IL-21, which in turn stimulates intragraft B cells, with deleterious consequences on kidney transplant.
In accordance with the aforementioned study, it has been proposed that Tregs could impair TLS formation. Kocks et al. for example observed that CCR7-deficient mice spontaneously develop very organized BALTs under steady-state conditions (98). Because BALT occurrence was reverted by the adoptive transfer of CCR7-expressing wild-type Tregs, authors suggested that Tregs may play a role in controlling BALT formation. It is emphasized in this study that BALTs may not be bona fide TLS, as they arise in absence of any inflammatory signals. In parallel, Hindley et al. showed in carcinogen-induced tumor-bearing mice that Treg depletion allowed the development of HEVs within tumors, associated with higher expression of LTα, LTβ, CCL19, CCL21, and CXCL13, higher CD8+ T-cell infiltration, and lower tumor growth (99). These observations are in accordance with the study of Martinet et al., who showed a decreased FoxP3+/CD3+ T-cell ratio in HEVhi human breast tumors (88).
Thus, if factors influencing TLS formation are still unclear, in particular in cancer, three critical events definitively appear to promote TLS formation: inflammatory cytokine production (such as LTα/β), lymphoid chemokine production, and HEV development (25). By their capacity to produce LT and pro-angiogenic factors, it appears that B cells and DCs may participate in the initiation and/or maintenance of TLS.
Double Face of B Cells in Tumor Immunity
Deleterious Effects of B Cells in Anti-Tumor Immunity
For a long time, the deleterious or positive role of B cells in anti-tumor immune response was a matter of debate. Many studies reported a pro-tumoral role of B cells in cancer patients and tumor mice models. For example, the detection of specific antibodies in the serum or in the tumor of breast cancer patients was associated with poor prognosis (100). In advanced colorectal cancer, a reduction of the tumor size following B-cell depletion could be observed in half of the patients treated with Rituximab, a humanized monoclonal antibody directed against human CD20 (101). Several mechanisms have been proposed to explain this pro-tumoral effect, using inactivated genes, xenograft experiments, adoptive cell transfer, or systemic B-cell depletion in mice models. The participation and/or the maintenance of a chronic inflammation by B cells leading to the growth of the tumor have notably been suggested (102). Also, when bound to antigens into immune complexes, antibodies can activate the complement system, which can trigger inflammation in B-cell deficient (μMT) or B-cell depleted mice. Indeed, the secretion of mediators like anaphylatoxins (i.e., C3 and C5a components) can induce the recruitment of inflammatory cells which in turn may provide a rich pro-angiogenic and pro-tumoral environment (103). Depending on the context, immune complexes can also promote tumor growth via the degradation of the extracellular matrix and the promotion of angiogenesis in a granulocyte- and macrophage-dependent manner (104). B cells were shown to directly inhibit cytotoxic T-cell responses in colorectal, melanoma, and thymoma tumor models in μMT mice (105). In addition, B-cell-derived factors, like TGF-β and IL-10, can favor the differentiation and the recruitment of Tregs, further amplifying the immunosuppressive environment (106, 107). Lastly, it has been proposed that LT produced by B cells could favor tumor metastasis in an NF-κB-independent manner (74). This phenomenon was notably demonstrated in a mouse model of castration-resistant prostate cancer, in which tumor cell death induced by castration elicited an inflammatory response, associated with inflammatory chemokine production and recruitment of immune cells. In this model, newly recruited mature B2 cells induced the activation of prostate cancer cells via LT-LTβR signaling, followed by IKK-α nuclear translocation and STAT3 activation, ultimately enhancing androgen-independent tumor growth and spreading. However, some of these studies, like ones in μMT mice, have to be interpreted with caution, as mice used in these experiments have also profound defects in TCR repertoire usage and strong disruption of lymphoid tissue architecture with diminished FDC, DC, and NK cell numbers.
Beneficial Effects of B Cells in Anti-Tumor Immunity
Recent studies readdressed this question using more relevant animal models. For instance, DiLillo et al. demonstrated a higher growth of B16 melanoma and lung metastasis, associated with decreased numbers of both CD4+ and CD8+ effector T cells, in mice depleted in B cells using an anti-CD20 antibody as compared with wild-type mice, demonstrating the key role of B cells in the induction and maintenance of a protective anti-tumor cellular immunity (108). In accordance with a beneficial role for tumor-infiltrating B cells in some mouse models, numerous clinical studies reported a positive correlation between the densities of total B cells and the clinical outcome of cancer patients, such as in NSCLC (15), primary cutaneous melanoma (10), breast cancer (11, 12, 14), and ovarian cancer (18). Some studies included the organization of tumor-infiltrating B cells as a supplementary criteria to consider in addition to the cell density. Since then, markers of TLS B-cell follicles (follicular B cells, Tfh cells, B-cell chemoattractant CXCL13) were all associated with prolonged cancer patient survival (39, 43, 44). In chronic obstructive pulmonary disease (COPD), TLS B cells have been shown to secrete both CXCL13 and LT (109). Thus, it is tempting to speculate that intra-tumoral B cells may also play a beneficial role via the maintenance of cancer-associated TLS which are associated with long-term survival. The organization of B cells into TLS B-cell follicles may thus better reflect the initiation of a local anti-tumor B-cell-mediated immunity (18, 39, 110, 111). Actually, molecular analyses of TLS-derived GC B cells from patients suffering from RA, primary Sjögren syndrome, or myasthenia gravis showed evidence of oligoclonal B-cell proliferation and SHM of immunoglobulin variable genes (112–115). In RA, the functionality of TLS was further demonstrated by the presence of anti-citrullinated protein/peptide (ACPA) PCs surrounding ectopic lymphoid structures in the synovial tissue of patients (50). In metastatic melanoma patients, TLS B cells were antigen experienced as demonstrated by the presence of clonal amplification, somatic mutation, and isotype switching (40). We similarly detected in NSCLC patients all stages of B-cell differentiation within tumors, in accordance with the presence of AID+ GC B cells, differentiated memory B cells and plasmablasts within TLS, and PCs within tumor stroma (Figure 1) (39). The demonstration of a direct correlation between the percentage of PCs and the density of TLS follicular B cells in NSCLC tumors, together with the capacity of tumor PCs to secrete antibodies specific for tumor antigens (discussed below) were further evidence of TLS B-cell functionality.
In parallel of their capacity to produce antigen-specific antibodies, B cells can also act as powerful APCs for T-cell activation and memory T-cell development (116, 117). It is known that during antigen challenge, presentation of processed antigens by B cells to CD4+ T cells in the outer follicle is necessary to the full activation of B cells, and their differentiation into short-lived plasmablasts or their proliferation and organization into GC. Several ligand/receptor pairs are involved during the B-T-cell interaction and also determine the extent of primary expansion of CD4+ T cells, such as CD80-CD86/CD28, CD40/CD40L, and OX40L/OX40, expressed on the surface of B cells and T cells, respectively. Especially, interaction between CD40, that is constitutively expressed by B cells, and its ligand CD40L which is expressed by activated Th cells, is crucial for GC formation (47, 118). Besides of GC reaction, the contribution of B cells as APCs was demonstrated in many disorders, including autoimmunity and allograft rejection. In multiple sclerosis for instance, activated B cells were shown to stimulate activation and proliferation of myelin basic protein-specific autologous CD4+ T cells (119). Similarly, in non-obese diabetic (NOD) mice, Serreze et al. showed that B cells are necessary APCs for the initial priming of T-cell responses against the candidate pancreatic β cell autoantigen glutamic acid decarboxylase (GAD) (120). While NOD mice genetically deficient in B lymphocytes were resistant to T-cell-mediated autoimmune insulin-dependent diabetes mellitus, and failed to develop GAD-specific T-cell responses, repopulation of mice with B lymphocytes restored their ability to initiate anti-GAD T-cell immunity. This observation was confirmed by Bouaziz et al. who showed that B-cell depletion with an anti-CD20 antibody impaired CD4+ T-cell activation and clonal expansion in response to protein antigens and pathogen challenge, suggesting the requirement of both B cells and DCs for optimal antigen-specific CD4+ T-cell priming (121). This result is in accordance with our observation in NSCLC patients, in which high densities of both TLS mature DCs and TLS B cells correlated with longer survival (39). However, TLS mature DCHigh patients do not match perfectly with TLS B-cellHigh patients. The combination of both biomarkers was the best predictor for survival suggesting that both APCs may play a complementary role in the initiation of protective immune responses in NSCLC patients. The capacity of B cells to cross-present antigens to CD8+ T cells is also well established (122–124). We notably showed that 30-mer polypeptides derived from the cancer-testis antigen NY-ESO-1 were efficiently cross-presented by B cells and immunogenic, in that they induced the proliferation of nonamer-specific CD8+ T cells in vitro (122). Finally, B cells would have the capacity to reactivate T cells previously primed by DCs (125). According to Nielsen et al., B cells may similarly serve as APCs in cancer and thus promote anti-tumor T-cell responses (18). Actually, authors observed that tumor-infiltrating CD20+ B cells were often found in close proximity to CD8+ T cells, and that the presence of both CD20+ and CD8+ lymphocytes was associated with markedly prolonged survival of ovarian cancer patients compared with that of CD8+ T-cell infiltrate alone. In addition, they observed that tumor CD20+ B cells expressed several markers of antigen presentation, such as MHC Class I and II molecules, as well as the costimulatory molecules CD80 and CD86. In accordance with that study, a high expression of CD40, CD80, and HLA-DR was also reported by Yasuda et al. on tumor-infiltrating B cells in primary lung cancer (126). Finally, some tumor-infiltrating B-cell subsets may act as killer cells through the expression of granzyme B and TRAIL, induce a Th1 polarization via the secretion of IFNγ and to a lesser extend IL12p40, as well as present processed antigens to T cells through the expression of MHC class I, MHC class II, and costimulatory molecules, as demonstrated in hepatocellular carcinoma patients (127).
Lastly, the ligation of CD27 expressed by B cells to CD70 present on cytotoxic T lymphocytes (CTLs) was deemed critical to sustain B-cell activation and immunoglobulin synthesis in an antigen-independent manner, as well as to promote CTL survival and proliferation (128). In contrast to ovarian and hepatocellular carcinomas (18, 127), memory B cells infiltrating lung tumors are positive for CD27, which argues for an additional anti-tumor immune function of intra-tumoral B cells (39).
As for T-cell infiltrate, it is necessary to take into account the activation status and the cellular organization of intra-tumoral B cells within the tumor microenvironment. The presence of TLS may represent a privileged site where specific naïve B cells can undergo their final differentiation into effector B cells, i.e., memory B cells and PCs, and thus may play a pivotal role in cellular- and humoral-mediated anti-tumor immunity.
Antibody Responses to Tumor Antigens in the Periphery and In situ
It has been clearly established that tumor-infiltrating B cells, and in particular cancer-associated TLS B cells, contribute to tumor prognosis and are able to produce antibodies locally. Thus, the important question to address is what these antibodies do, what their specificity is, and whether produced antibodies may contribute to the clinical outcome. In the 1980s, the knowledge that tumors can elicit spontaneous humoral responses spurred the development of autologous typing to identify novel molecular targets (129, 130). One of the most prolific methods to discover tumor-associated antigens was serological expression cloning (SEREX), which originally used serum of a cancer patient to screen for targets expressed in the autologous tumor after expression cloning (131). This unbiased method led to the discovery of a series of novel immunogenic targets, many of which were validated to be only eliciting antibody responses in the context of tumors (132). NY-ESO-1, discovered by SEREX, emerged as a model human tumor antigen, with restricted expression in normal tissues to germline cells, but widespread aberrant expression in various tumor types, and therefore named a cancer-testis antigen (133). Members of this family include MAGE, LAGE, XAGE, SSX, and other antigens, all of which are intracellular molecules, with cytoplasmic and/or nuclear expression. These antigens are controlled by epigenetic mechanisms and can be induced by hypomethylation or deacetylation of promoter sequences (134). It is thought that as tumors grow, some cells may be releasing antigens and initiating B-cell responses, and it is well established that detection of antibodies against these tumor antigens is associated with tumor antigen expression, even though there is no evidence that resulting antibodies have a direct functional role against tumors (135). Not much is known about what initiate immunogenicity, and whether it happens at the tumor site from local TLS, or whether more classic SLOs are responsible, after APCs carrying tumor extracts migrate there. A positive correlation has been described between size of LN B-cell areas and tumor-infiltrating B cells in head and neck cancer patients treated with a mixture of cytokines (136), suggesting that an interplay may be happening across SLOs and local tumor environment. To address the question of the structure initiating humoral responses, one would need a dynamic follow-up of antigen and uptake, and a better characterization of TLS versus LN versus circulating B cells for their specificity and chemokine receptor expression.
While B cells at the tumor site may recognize tumor antigens, it is important to consider their role and potential clinical benefit in a dynamic fashion as tumors evolve over time. Expression of most cancer-testis antigens tends to increase with stage and grade of disease, as shown for melanoma, lung, myeloma, and ovarian tumors among others, thereby highlighting more aggressive, poorly differentiated tumors with usually worse outcome (137), but that also become immunogenic as a result of new antigenicity. A study has found that NY-ESO-1 protein expression in primary breast carcinoma and metastases correlates with brisk plasmacytic B-cell infiltration (138). It is likely however that by the time, the tumor has reached a large enough size to generate B-cell response, the resulting antibodies are insufficient to affect the disease. Recently, tumor-infiltrating B cells were found increased in prostate cancer tissue with high intra-tumoral density of B cells related to higher stage and potential for recurrence or progression (139). In addition, B cells from ascites of ovarian cancer are able to produce antibodies to known tumor antigens, though the presence of B cells in ascites has been shown to increase with stage and to correlate with poor prognosis, but to decrease by chemotherapeutic treatment (140).
It is therefore important to view antibody responses as diverse repertoires with polyfunctionality, not all of it associated with better prognosis. Historically, circulating antibodies to tumor-specific antigens were seen as a sign that cellular immunity was skewed in favor of humoral rather than cellular responses, while CTLs were preferred for their direct anti-tumor capacity (103, 141). Mutated TP53 was one of the first tumor-associated antigens to be identified serologically (142), but circulating CTL responses against TP53 appear to be rare (143). Despite abundant literature emphasizing the split between B cell and Th2-mediated immunity versus cytotoxic CD8+ T cell and Th1 immunity, this dichotomy may not be so clear for all tumor-associated antigens. In particular, NY-ESO-1, one of the most spontaneously immunogenic antigens from the cancer-testis family, is an example for which presence of specific circulating antibodies typically implies concomitant presence of circulating effector-like CD4+ and CD8+ T cells, in an integrated fashion (144, 145). Therefore, antibodies have been proposed as a surrogate for T-cell immunity to NY-ESO-1. We showed that patients seropositive for NY-ESO-1 have NY-ESO-1-specific T cells accumulating at the tumor site in ovarian cancer patients, though their activity was blunted by expression of co-inhibitory molecules CTLA-4, PD-1, and LAG-3 (146). This may explain the lack of anti-tumor effect, and therapeutic strategies to reverse such responses will be discussed later. Nevertheless, these results suggest that seropositivity could be a surrogate for the presence of tumor-specific T cells infiltrating tumors.
Serum reactivities against tumor antigens have been described in many cancer types, such as anti-NY-ESO-1 antibodies in sarcoma (147) and primary breast cancer (148), anti-XAGE-1b antibodies in NSCLC (3), anti-NY-ESO-1, anti-LAGE-1, and anti-P53 antibodies in ovarian cancer (18, 149). Very recently, Ohue et al. showed that cancer patients with serum antibody-reactivity against the tumor antigen XAGE1 had prolonged overall survival as compared with seronegative patients (3). Similarly, antibodies to MUC1 appeared to confer better survival in various tumors, while p53 antibodies did the opposite (150). Circulating antibody responses to NY-ESO-1, SOX2, Ubiquilin, and others have been proposed as biomarkers for early detection of NSCLC (151, 152), though few have been validated to date (153). Presence of circulating autoantibodies to tumor antigens has also been well described in paraneoplastic syndrome, where specificities shared between tumors and the nervous system abruptly lead to autoimmune pathology, albeit with some anti-tumor benefit (154).
Some very recent studies have provided evidence that tumor-specific humoral responses can be generated in situ, within TLS. To characterize the specificity of local B cells at the tumor site, we performed ex vivo cultures of NSCLC-infiltrating B cells and showed that they were able to produce high levels of IgG and IgA directed against tumor antigens of the cancer-testis antigen family, such as LAGE-1, MAGE antigens, and NY-ESO-1, but also P53. The reactivity we observed was polyclonal, as a same patient could display reactivities against several different tumor antigens (39). The presence of IgA at the tumor site is intriguing, as it differs from the usual IgG detected in the periphery, and detailed repertoire analyses are still needed to distinguish potential differences locally and systemically. More evidence of antigen-specific local B cells was found in ductal breast carcinoma where infiltrating B cells from TLS-like structures were microdissected and showed polyclonality and high mutation rates, with some dominant clones shared between various clusters of B cells suggesting affinity maturation in situ and seeding from site to site (155). Finally, Maletzki et al. observed in colorectal cancer that tumor-infiltrating B cells produced IgG able to bind to the cell surface of several tumor cell lines (156).
Once again though, mere presence of antibodies may not necessarily be sufficient to predict outcome, as not all B-cell responses are of equal quality. In lung cancer, a recent report indicated increased circulating regulatory B cells [Bregs, reviewed in Ref. (157)] but decreased Tregs compared to healthy donors, highlighting a novel population of B cells to consider. It is likely that the context of the original antigen stimulation (viral versus self, chronically versus acutely inflamed) will drive the differentiation of the B-cell repertoire, and this opens up strategies for manipulation with immunotherapies.
Toward TLS- and Antibody-Based Therapeutic Strategies in Cancer
It is assumed that the presence of TLS within tumors (except in renal cell carcinoma) is always associated with prolonged survival (27). Thus, a big challenge in the near future will be to develop new therapeutic tools, or to determine therapeutic combinations, able to favor the formation of such lymphoid structures in cancer patients.
Several studies have already reported the development of TLS as a consequence of antigen challenge during anti-tumor vaccination protocols. Lutz et al. for example observed the induction of TLS in pancreatic ductal adenocarcinoma (PDAC) after vaccination with GM-CSF-secreting pancreatic tumor vaccine (GVAX), a granulocyte-macrophage colony-stimulating factor (GM-CSF)-secreting, allogeneic PDAC vaccine (158). While PDAC is classically considered as a non-immunogenic neoplasm, tumors from patients vaccinated with GVAX presented organized and functional TLS as soon as 2 weeks following GVAX treatment. These TLS displayed all features of an ongoing immune response, including GC B-cell proliferation, T-cell activation, and Th1 transcription factor T-bet expression, associated with the induction of IFNγ-expressing effector T-cell responses. The number of TLS detected within PDAC tumors tended to increase when patients were treated with GVAX combined to the Treg-depleting chemotherapy cyclophosphamide, in accordance with studies showing that Tregs can suppress the development of TLS (88, 98, 99, 159). More interestingly, a downregulation of genes involved in the Treg pathway and a concomitant upregulation of genes involved in the Th17 pathway was detected in TLS of patients who survived longer and had enhanced immune responses. These results are in accordance with studies claiming that, as opposed to Tregs, Th17 cells and Th17 cytokines promote TLS development (92, 93, 96, 97). Similarly, Maldonado et al. observed the formation of TLS in patients with high-grade cervical intraepithelial neoplasias (CIN2/3) who received intramuscular therapeutic vaccination targeting HPV16 E6/E7 antigens (160). These TLS were associated with HEVs, immune cell activation and Th1 polarization signatures, and TCR clonality. In both studies, it seems that vaccination-induced antigen-specific T cells generated systemically were able to massively infiltrate the tumor microenvironment, and to initiate a local inflammatory reaction, further favoring the recruitment of immune cells and TLS neogenesis.
Besides vaccination protocols, which aim to increase humoral and cellular immune responses against particular tumor antigens, several studies demonstrated that some chemotherapeutic drugs have the capacity to enhance tumor immunogenicity and recognition by the immune system. Kroemer and Zitvogel groups notably have extensively documented how anthracyclines and oxaliplatin are able to trigger an immunogenic form of tumor cell death, and thus to promote anti-tumor immune responses, through three molecular mechanisms (161): first, the translocation of calreticulin on the tumor cell surface, which results in tumor engulfment by DCs; and secondly, the release by dying tumor cells of HMGB1 protein and ATP, two danger signals that bind TLR4 and P2X7 on DCs, respectively, and increase their ability to present tumor antigens and to activate anti-tumor CD8+ T-cell responses (162–164). Similarly, some DNA demethylating agents showed capacities to increase tumor immunogenicity, by favoring the expression of cancer-testis antigens in cancer cells (165). Ayyoub et al. for example demonstrated that DNA demethylation induced by 5-aza-2′-deoxycytidine (5-AZA-CdR) increased or induced mRNA expression of several cancer-testis antigens in sarcoma cell lines, including MAGE-A10, SSX, NY-ESO-1, and LAGE-1, resulting in increased tumor cell recognition by CTLs (147). Considering the potential role played by DCs and B cells in TLS induction and/or maintenance, we can speculate that chemotherapeutic drugs favoring DC and/or B-cell activation may also have a beneficial effect on the development of TLS within the tumor.
Other developed strategies aim to directly induce the formation of TLS within tumor tissue, by targeting at the tumor site signals or chemokines known as favoring immune cell recruitment and/or lymphoid neogenesis. Schrama et al. used the property of LTα to promote TLS development in a mouse model of melanoma (166). They developed an anti-disialoganglioside GD2 specific antibody-LTα fusion protein, and demonstrated that this fusion protein was able to favor subcutaneous tumor and established pulmonary metastasis eradication, and to prolong survival. Twenty-eight days after treatment, they could observe the presence of T cells and B cells organized in lymphoid-like patterns within tumors, characterized by the presence of MHC class II+ cells resembling DCs, the presence of CD62L+ cells in close contact with PNAd+ HEVs. Lymphoid neogenesis in the treated mice was associated with anti-tumor T-cell clonal expansion. Similarly, Liang et al. used the properties of the lymphoid chemokine CCL21 to favor the recruitment of immune cells at the tumor site (167). In a mouse model of hepatocellular carcinoma, they showed that the transduction of tumor cells with a recombinant adeno-associated virus (rAAV) expressing CCL21, or intra-tumoral injections of rAAV-CCL21, allowed extensive infiltration of the tumor site by CD11c+ DCs and activated CD3+ CD69+ T cells. Finally, Irvine et al. discussed the possibility of using tissue engineering, and matrix or scaffold-based approaches in order to create an implantable microenvironment that would support the recruitment of immune cell and/or favor lymphoid neogenesis through, for instance, the slow release of chemoattractants (168).
We can hypothesize that strategies aiming at increasing or re-activating exhausted T-cell responses, by targeting immunoregulators such as CTLA-4 or PD-1, may also have bystander effects on TLS development. Beyond immune checkpoint inhibitors, next generation immunomodulators may also involve costimulatory agonists that can target both T and B cells, including 4-1BB, CD27, HVEM, and CD40 (169). Cross-linking capacity of B cells was shown to be important for the therapeutic effect of immunomodulatory antibodies such as anti-CD40 (170). These novel strategies will need to be studied in detail for their role on local immune infiltration and organization, through the design of presurgical, neoadjuvant clinical studies. Another immunomodulatory approach, beside systemic administration of antibodies targeting immune pathways, may be to proceed with intra-tumoral injections of pathogen-associated molecular patterns (PAMPs) or cytokines, to change the inflammatory environment of the tumor. BTLA was described as an inhibitor of some B-cell functions that is upregulated in the context of immunotherapies including CpG administration, but could be actionable with blocking of its interaction with natural ligand HVEM (171). Intra-tumoral injection of IL-12 was also shown to activate B cells and lead to the polarization of immunoglobulin secretion to Th1-associated IgG1, along with IFNγ production by B cells (17).
The detection of a humoral anti-tumor immune response initiated within TLS and its characterization open the way to the development of new vaccine and antibody-based therapeutic strategies. As discussed above, reactivity against tumor antigens belonging to the cancer-testis antigen family is a feature shared by several cancer types, making these antigens as potential targets in future protocols of immunotherapy. Despite their intracellular expression, vaccines or monoclonal antibodies directed against some of these antigens have already been validated in preclinical models. Noguchi et al. for example demonstrated in mice the anti-tumor effect of an anti-NY-ESO-1 antibody when associated with chemotherapy (172). In this study, they observed not only the generation of an anti-NY-ESO-1 CD8+ T-cell immune response, but also the differentiation of other antigen-specific CD8+ T cells, thanks to antigen spreading and DC activation by anti-NY-ESO-1 immune complexes (173).
MAGE-A3 and NY-ESO-1 have entered into clinical trials for various cancers, and belong to the top 10 of the cancer antigens referenced in the “Prioritization of Cancer Antigens” project proposed by the National Cancer Institute (NCI) (174–177). Several studies have already reported the development of both humoral and cellular specific immune responses in NY-ESO-1-vaccinated cancer patients (144, 178). In one study, it was suggested that patients with the highest humoral responses following vaccination with NY-ESO-1 protein and CpG were more likely to have associated induction of CD8+ T cells (179).
Finally, as described above, NY-ESO-1 is known to elicit spontaneous immune responses though their clinical benefit is not clear, since the effector T cells at the tumor site may be dysfunctional due to immune checkpoint inhibition (146). We asked if some of the success of CTLA-4 blockade could be attributed to a change in immune responses against NY-ESO-1, since it is a frequent antigen in melanoma. We found that patients with pre-existing serological immunity to NY-ESO-1, coupled with detectable CD8+ T cells in the periphery, were nearly twice as likely to experience clinical benefit to ipilimumab compared to patients without NY-ESO-1 immunity (180). Circulating antibody titers, CD8+ T-cell repertoire, and polyfunctionality to NY-ESO-1 increased after treatment, but it is still unknown how ipilimumab affected local tumor B and T-cell responses. However, the clinical benefit suggests that having the tools to recognize NY-ESO-1 at baseline will make it more likely that immunomodulatory treatment will work.
Concluding Remarks
Tertiary lymphoid structures in human tumors contribute to an environment conducive to the generation of B-cell diversity, with the help of T cells and DCs that leads to accumulation of tumor-specific immune responses inside or in proximity to the tumor. The presence of such lymphoid structures, including their B-cell component, underlines a population of patients that have already been primed to react against their tumors, albeit immunosurveillance has failed by the time the tumor is clinically detectable likely due to many counter-regulatory mechanisms. Nevertheless, TLS, along with T cells, mature DCs, and B cells, often predict eventual outcome in various diseases such as NSCLC, which suggests that having a primed tumor immune microenvironment, i.e., local immunocompetence, prior to treatment may facilitate various interventions and slow down progression. However, with progression also comes a series of counter-regulatory mechanisms that hamper the orchestrated anti-tumor immune responses, including the B-cell repertoire.
Many questions remain to be answered however, such as identifying whether immune responses are originally primed locally in TLS and become systemic, or conversely are generated in classic lymphoid organs and migrate to the disease site. Investigating differences in antigen cross-presentation, in antigen specificity locally and systemically, in quality and function between tumor B cells, LN B cells, and circulating B cells should help bring some answers.
Conflict of Interest Statement
The authors declare that the research was conducted in the absence of any commercial or financial relationships that could be construed as a potential conflict of interest.
Acknowledgments
We are grateful to Prof. W. H. Fridman, C. Fridman, D. Damotte, as well as Drs. J. Goc, R. Remark, A. Lupo, P. Validire, L. de Chaisemartin, and S. Hammond for their contribution to these projects. We also would like to acknowledge the clinicians and pathologists who participated in the publications referenced in the present review. This work was supported by the Institut National de la Santé et de la Recherche Médicale (INSERM), University Paris Descartes, University Pierre and Marie Curie, SIRIC Cancer Research and Personalized Medicine (CARPEM), the LabeX Immuno-Oncology, Institut National du Cancer and Canceropole Ile-de-France, Fondation ARC pour la Recherche sur le Cancer, and Cancer Research Institute as part of the Cancer Vaccine Collaborative.
Abbreviations
5-AZA-CdR, 5-aza-2′-deoxycytidine; ACPA, anti-citrullinated protein/peptide; ADCC, antibody-dependent cellular cytotoxicity; AHR, aryl hydrocarbon receptor; AID, activation-induced cytidine deaminase; APC, antigen-presenting cell; BALT, bronchus-associated lymphoid tissue; CDC, complement-dependent cytotoxicity; CSR, class switch recombination; CTL, cytotoxic T lymphocyte; DC, dendritic cell; EAE, experimental autoimmune encephalomyelitis; FcR, fragment constant receptor; FDC, follicular dendritic cell; FRC, fibroblastic reticular cell; GAD, glutamic acid decarboxylase; GC, germinal center; GD2, disialoganglioside 2; GM-CSF, granulocyte-macrophage colony-stimulating factor; GVAX, GM-CSF-secreting pancreatic tumor vaccine; HEV, high endothelial venule; i-BALT, induced BALT; ICAM-1, intercellular cell adhesion molecule 1; ILC, innate lymphoid cell; LN, lymph node; LTi, lymphoid tissue-inducer; LTo, lymphoid tissue-organizer; LTβ, lymphotoxin β; MALT, mucosal-associated lymphoid tissue; MHC, major histocompatibility complex; MOG, myelin oligodendrocyte glycoprotein; MVA, modified vaccinia virus Ankara; NALT, nasal-associated lymphoid tissue; NK, natural killer; NOD, non-obese diabetic; NSCLC, non-small cell lung cancer; PAMP, pathogen-associated molecular pattern; PC, plasma cell; PDAC, pancreatic ductal adenocarcinoma; pDC, plasmacytoid dendritic cell; PNAd, peripheral node addressin; RA, rheumatoid arthritis; rAAV, recombinant adeno-associated virus; SEREX, serological expression cloning; SHM, somatic hyper-mutation; SLO, secondary lymphoid organ; SMC, smooth muscle cell; Tfh, T follicular helper; Th, T helper; TLO, tertiary lymphoid organ; TLS, tertiary lymphoid structure; VCAM-1, vascular cell adhesion molecule 1; VEGF, vascular endothelial growth factor.
References
1. Fridman WH, Pagès F, Sautès-Fridman C, Galon J. The immune contexture in human tumours: impact on clinical outcome. Nat Rev Cancer (2012) 12:298–306. doi:10.1038/nrc3245
Pubmed Abstract | Pubmed Full Text | CrossRef Full Text | Google Scholar
2. Van der Bruggen P, Traversari C, Chomez P, Lurquin C, De Plaen E, Van den Eynde B, et al. A gene encoding an antigen recognized by cytolytic T lymphocytes on a human melanoma. Science (1991) 254:1643–7. doi:10.1126/science.1840703
Pubmed Abstract | Pubmed Full Text | CrossRef Full Text | Google Scholar
3. Ohue Y, Eikawa S, Okazaki N, Mizote Y, Isobe M, Uenaka A, et al. Spontaneous antibody, and CD4 and CD8 T-cell responses against XAGE-1b (GAGED2a) in non-small cell lung cancer patients. Int J Cancer (2012) 131:E649–58. doi:10.1002/ijc.27359
Pubmed Abstract | Pubmed Full Text | CrossRef Full Text | Google Scholar
4. Zippelius A, Gati A, Bartnick T, Walton S, Odermatt B, Jaeger E, et al. Melanocyte differentiation antigen RAB38/NY-MEL-1 induces frequent antibody responses exclusively in melanoma patients. Cancer Immunol Immunother (2007) 56:249–58. doi:10.1007/s00262-006-0177-z
Pubmed Abstract | Pubmed Full Text | CrossRef Full Text | Google Scholar
5. Walton SM, Gerlinger M, de la Rosa O, Nuber N, Knights A, Gati A, et al. Spontaneous CD8 T cell responses against the melanocyte differentiation antigen RAB38/NY-MEL-1 in melanoma patients. J Immunol (2006) 177:8212–8. doi:10.4049/jimmunol.177.11.8212
Pubmed Abstract | Pubmed Full Text | CrossRef Full Text | Google Scholar
6. Soussi T. p53 antibodies in the sera of patients with various types of cancer: a review. Cancer Res (2000) 60:1777–88.
7. Hanahan D, Weinberg RA. Hallmarks of cancer: the next generation. Cell (2011) 144:646–74. doi:10.1016/j.cell.2011.02.013
8. Bindea G, Mlecnik B, Tosolini M, Kirilovsky A, Waldner M, Obenauf AC, et al. Spatiotemporal dynamics of intratumoral immune cells reveal the immune landscape in human cancer. Immunity (2013) 39:782–95. doi:10.1016/j.immuni.2013.10.003
Pubmed Abstract | Pubmed Full Text | CrossRef Full Text | Google Scholar
9. Galon J, Costes A, Sanchez-Cabo F, Kirilovsky A, Mlecnik B, Lagorce-Pagès C, et al. Type, density, and location of immune cells within human colorectal tumors predict clinical outcome. Science (2006) 313:1960–4. doi:10.1126/science.1129139
Pubmed Abstract | Pubmed Full Text | CrossRef Full Text | Google Scholar
10. Ladányi A, Kiss J, Mohos A, Somlai B, Liszkay G, Gilde K, et al. Prognostic impact of B-cell density in cutaneous melanoma. Cancer Immunol Immunother (2011) 60:1729–38. doi:10.1007/s00262-011-1071-x
Pubmed Abstract | Pubmed Full Text | CrossRef Full Text | Google Scholar
11. Mahmoud SMA, Lee AHS, Paish EC, Macmillan RD, Ellis IO, Green AR. The prognostic significance of B lymphocytes in invasive carcinoma of the breast. Breast Cancer Res Treat (2012) 132:545–53. doi:10.1007/s10549-011-1620-1
Pubmed Abstract | Pubmed Full Text | CrossRef Full Text | Google Scholar
12. Simsa P, Teillaud J-L, Stott DI, Tóth J, Kotlan B. Tumor-infiltrating B cell immunoglobulin variable region gene usage in invasive ductal breast carcinoma. Pathol Oncol Res (2005) 11:92–7. doi:10.1007/BF02893374
Pubmed Abstract | Pubmed Full Text | CrossRef Full Text | Google Scholar
13. Kotlan B, Simsa P, Teillaud J-L, Fridman WH, Toth J, McKnight M, et al. Novel ganglioside antigen identified by B cells in human medullary breast carcinomas: the proof of principle concerning the tumor-infiltrating B lymphocytes. J Immunol (2005) 175:2278–85. doi:10.4049/jimmunol.175.4.2278
Pubmed Abstract | Pubmed Full Text | CrossRef Full Text | Google Scholar
14. Schmidt M, Böhm D, von Törne C, Steiner E, Puhl A, Pilch H, et al. The humoral immune system has a key prognostic impact in node-negative breast cancer. Cancer Res (2008) 68:5405–13. doi:10.1158/0008-5472.CAN-07-5206
Pubmed Abstract | Pubmed Full Text | CrossRef Full Text | Google Scholar
15. Al-Shibli KI, Donnem T, Al-Saad S, Persson M, Bremnes RM, Busund L-T. Prognostic effect of epithelial and stromal lymphocyte infiltration in non-small cell lung cancer. Clin Cancer Res (2008) 14:5220–7. doi:10.1158/1078-0432.CCR-08-0133
Pubmed Abstract | Pubmed Full Text | CrossRef Full Text | Google Scholar
16. Lohr M, Edlund K, Botling J, Hammad S, Hellwig B, Othman A, et al. The prognostic relevance of tumour-infiltrating plasma cells and immunoglobulin kappa C indicates an important role of the humoral immune response in non-small cell lung cancer. Cancer Lett (2013) 333:222–8. doi:10.1016/j.canlet.2013.01.036
Pubmed Abstract | Pubmed Full Text | CrossRef Full Text | Google Scholar
17. Van Herpen CM, van der Voort R, van der Laak JA, Klasen IS, de Graaf AO, van Kempen LC, et al. Intratumoral rhIL-12 administration in head and neck squamous cell carcinoma patients induces B cell activation. Int J Cancer (2008) 123:2354–61. doi:10.1002/ijc.23756
Pubmed Abstract | Pubmed Full Text | CrossRef Full Text | Google Scholar
18. Nielsen JS, Sahota RA, Milne K, Kost SE, Nesslinger NJ, Watson PH, et al. CD20+ tumor-infiltrating lymphocytes have an atypical CD27- memory phenotype and together with CD8+ T cells promote favorable prognosis in ovarian cancer. Clin Cancer Res (2012) 18:3281–92. doi:10.1158/1078-0432.CCR-12-0234
Pubmed Abstract | Pubmed Full Text | CrossRef Full Text | Google Scholar
19. Sato E, Olson SH, Ahn J, Bundy B, Nishikawa H, Qian F, et al. Intraepithelial CD8+ tumor-infiltrating lymphocytes and a high CD8+/regulatory T cell ratio are associated with favorable prognosis in ovarian cancer. Proc Natl Acad Sci U S A (2005) 102:18538–43. doi:10.1073/pnas.0509182102
Pubmed Abstract | Pubmed Full Text | CrossRef Full Text | Google Scholar
20. Zhang L, Conejo-Garcia JR, Katsaros D, Gimotty PA, Massobrio M, Regnani G, et al. Intratumoral T cells, recurrence, and survival in epithelial ovarian cancer. N Engl J Med (2003) 348:203–13. doi:10.1056/NEJMoa020177
21. Moyron-Quiroz JE, Rangel-Moreno J, Kusser K, Hartson L, Sprague F, Goodrich S, et al. Role of inducible bronchus associated lymphoid tissue (iBALT) in respiratory immunity. Nat Med (2004) 10:927–34. doi:10.1038/nm1091
Pubmed Abstract | Pubmed Full Text | CrossRef Full Text | Google Scholar
22. Dieu-Nosjean M-C, Antoine M, Danel C, Heudes D, Wislez M, Poulot V, et al. Long-term survival for patients with non-small-cell lung cancer with intratumoral lymphoid structures. J Clin Oncol (2008) 26:4410–7. doi:10.1200/JCO.2007.15.0284
Pubmed Abstract | Pubmed Full Text | CrossRef Full Text | Google Scholar
23. Rangel-Moreno J, Hartson L, Navarro C, Gaxiola M, Selman M, Randall TD. Inducible bronchus-associated lymphoid tissue (iBALT) in patients with pulmonary complications of rheumatoid arthritis. J Clin Invest (2006) 116:3183–94. doi:10.1172/JCI28756
Pubmed Abstract | Pubmed Full Text | CrossRef Full Text | Google Scholar
24. Kratz A, Campos-Neto A, Hanson MS, Ruddle NH. Chronic inflammation caused by lymphotoxin is lymphoid neogenesis. J Exp Med (1996) 183:1461–72. doi:10.1084/jem.183.4.1461
Pubmed Abstract | Pubmed Full Text | CrossRef Full Text | Google Scholar
25. Drayton DL, Liao S, Mounzer RH, Ruddle NH. Lymphoid organ development: from ontogeny to neogenesis. Nat Immunol (2006) 7:344–53. doi:10.1038/ni1330
Pubmed Abstract | Pubmed Full Text | CrossRef Full Text | Google Scholar
26. Aloisi F, Pujol-Borrell R. Lymphoid neogenesis in chronic inflammatory diseases. Nat Rev Immunol (2006) 6:205–17. doi:10.1038/nri1786
Pubmed Abstract | Pubmed Full Text | CrossRef Full Text | Google Scholar
27. Dieu-Nosjean MC, Goc J, Giraldo NA, Sautès-Fridman C, Fridman WH. Tertiary lymphoid structures in cancer and beyond. Trends Immunol (2014) 35(11):571–80. doi:10.1016/j.it.2014.09.006
28. Marchal-Sommé J, Uzunhan Y, Marchand-Adam S, Kambouchner M, Valeyre D, Crestani B, et al. Dendritic cells accumulate in human fibrotic interstitial lung disease. Am J Respir Crit Care Med (2007) 176:1007–14. doi:10.1164/rccm.200609-1347OC
Pubmed Abstract | Pubmed Full Text | CrossRef Full Text | Google Scholar
29. Houtkamp MA, de Boer OJ, van der Loos CM, van der Wal AC, Becker AE. Adventitial infiltrates associated with advanced atherosclerotic plaques: structural organization suggests generation of local humoral immune responses. J Pathol (2001) 193:263–9. doi:10.1002/1096-9896(2000)9999:9999<::AID-PATH774>3.0.CO;2-N
Pubmed Abstract | Pubmed Full Text | CrossRef Full Text | Google Scholar
30. Galkina E, Kadl A, Sanders J, Varughese D, Sarembock IJ, Ley K. Lymphocyte recruitment into the aortic wall before and during development of atherosclerosis is partially L-selectin dependent. J Exp Med (2006) 203:1273–82. doi:10.1084/jem.20052205
Pubmed Abstract | Pubmed Full Text | CrossRef Full Text | Google Scholar
31. Nicoletti A, Khallou-Laschet J, Guedj K, Clement M, Gaston A-T, Morvan M, et al. L19. Lymphoid neogenesis in vascular chronic inflammation. Presse Med (2013) 42:558–60. doi:10.1016/j.lpm.2013.01.018
32. Thaunat O, Patey N, Morelon E, Michel J-B, Nicoletti A. Lymphoid neogenesis in chronic rejection: the murderer is in the house. Curr Opin Immunol (2006) 18:576–9. doi:10.1016/j.coi.2006.07.006
Pubmed Abstract | Pubmed Full Text | CrossRef Full Text | Google Scholar
33. Neyt K, Perros F, GeurtsvanKessel CH, Hammad H, Lambrecht BN. Tertiary lymphoid organs in infection and autoimmunity. Trends Immunol (2012) 33:297–305. doi:10.1016/j.it.2012.04.006
Pubmed Abstract | Pubmed Full Text | CrossRef Full Text | Google Scholar
34. Baddoura FK, Nasr IW, Wrobel B, Li Q, Ruddle NH, Lakkis FG. Lymphoid neogenesis in murine cardiac allografts undergoing chronic rejection. Am J Transplant (2005) 5:510–6. doi:10.1111/j.1600-6143.2004.00714.x
Pubmed Abstract | Pubmed Full Text | CrossRef Full Text | Google Scholar
35. Kerjaschki D, Regele HM, Moosberger I, Nagy-Bojarski K, Watschinger B, Soleiman A, et al. Lymphatic neoangiogenesis in human kidney transplants is associated with immunologically active lymphocytic infiltrates. J Am Soc Nephrol (2004) 15:603–12. doi:10.1097/01.ASN.0000113316.52371.2E
Pubmed Abstract | Pubmed Full Text | CrossRef Full Text | Google Scholar
36. Thaunat O, Patey N, Caligiuri G, Gautreau C, Mamani-Matsuda M, Mekki Y, et al. Chronic rejection triggers the development of an aggressive intragraft immune response through recapitulation of lymphoid organogenesis. J Immunol (2010) 185:717–28. doi:10.4049/jimmunol.0903589
Pubmed Abstract | Pubmed Full Text | CrossRef Full Text | Google Scholar
37. Thaunat O, Field A-C, Dai J, Louedec L, Patey N, Bloch M-F, et al. Lymphoid neogenesis in chronic rejection: evidence for a local humoral alloimmune response. Proc Natl Acad Sci U S A (2005) 102:14723–8. doi:10.1073/pnas.0507223102
Pubmed Abstract | Pubmed Full Text | CrossRef Full Text | Google Scholar
38. Goc J, Germain C, Vo-Bourgais TKD, Lupo A, Klein C, Knockaert S, et al. Dendritic cells in tumor-associated tertiary lymphoid structures signal a Th1 cytotoxic immune contexture and license the good positive prognostic value of infiltrating CD8+ T cells. Cancer Res (2014) 74:705–15. doi:10.1158/0008-5472.CAN-13-1342
39. Germain C, Gnjatic S, Tamzalit F, Knockaert S, Remark R, Goc J, et al. Presence of B cells in tertiary lymphoid structures is associated with a protective immunity in patients with lung cancer. Am J Respir Crit Care Med (2014) 189:832–44. doi:10.1164/rccm.201309-1611OC
Pubmed Abstract | Pubmed Full Text | CrossRef Full Text | Google Scholar
40. Cipponi A, Mercier M, Seremet T, Baurain J-F, Théate I, van den Oord J, et al. Neogenesis of lymphoid structures and antibody responses occur in human melanoma metastases. Cancer Res (2012) 72:3997–4007. doi:10.1158/0008-5472.CAN-12-1377
Pubmed Abstract | Pubmed Full Text | CrossRef Full Text | Google Scholar
41. Messina JL, Fenstermacher DA, Eschrich S, Qu X, Berglund AE, Lloyd MC, et al. 12-Chemokine gene signature identifies lymph node-like structures in melanoma: potential for patient selection for immunotherapy? Sci Rep (2012) 2:765. doi:10.1038/srep00765
Pubmed Abstract | Pubmed Full Text | CrossRef Full Text | Google Scholar
42. Coppola D, Nebozhyn M, Khalil F, Dai H, Yeatman T, Loboda A, et al. Unique ectopic lymph node-like structures present in human primary colorectal carcinoma are identified by immune gene array profiling. Am J Pathol (2011) 179:37–45. doi:10.1016/j.ajpath.2011.03.007
Pubmed Abstract | Pubmed Full Text | CrossRef Full Text | Google Scholar
43. Gu-Trantien C, Loi S, Garaud S, Equeter C, Libin M, de Wind A, et al. CD4+ follicular helper T cell infiltration predicts breast cancer survival. J Clin Invest (2013) 123:2873–92. doi:10.1172/JCI67428
Pubmed Abstract | Pubmed Full Text | CrossRef Full Text | Google Scholar
44. Wirsing AM, Rikardsen OG, Steigen SE, Uhlin-Hansen L, Hadler-Olsen E. Characterisation and prognostic value of tertiary lymphoid structures in oral squamous cell carcinoma. BMC Clin Pathol (2014) 14:38. doi:10.1186/1472-6890-14-38
Pubmed Abstract | Pubmed Full Text | CrossRef Full Text | Google Scholar
45. Katakai T, Hara T, Sugai M, Gonda H, Shimizu A. Lymph node fibroblastic reticular cells construct the stromal reticulum via contact with lymphocytes. J Exp Med (2004) 200:783–95. doi:10.1084/jem.20040254
Pubmed Abstract | Pubmed Full Text | CrossRef Full Text | Google Scholar
46. Girard J-P, Moussion C, Förster R. HEVs, lymphatics and homeostatic immune cell trafficking in lymph nodes. Nat Rev Immunol (2012) 12:762–73. doi:10.1038/nri3298
Pubmed Abstract | Pubmed Full Text | CrossRef Full Text | Google Scholar
47. Gatto D, Brink R. The germinal center reaction. J Allergy Clin Immunol (2010) 126:898–907. doi:10.1016/j.jaci.2010.09.007
48. Gottlin EB, Bentley RC, Campa MJ, Pisetsky DS, Herndon JE, Patz EF. The association of intratumoral germinal centers with early-stage non-small cell lung cancer. J Thorac Oncol (2011) 6:1687–90. doi:10.1097/JTO.0b013e3182217bec
Pubmed Abstract | Pubmed Full Text | CrossRef Full Text | Google Scholar
49. GeurtsvanKessel CH, Willart MA, Bergen IM, van Rijt LS, Muskens F, Elewaut D, et al. Dendritic cells are crucial for maintenance of tertiary lymphoid structures in the lung of influenza virus-infected mice. J Exp Med (2009) 206:2339–49. doi:10.1084/jem.20090410
Pubmed Abstract | Pubmed Full Text | CrossRef Full Text | Google Scholar
50. Humby F, Bombardieri M, Manzo A, Kelly S, Blades MC, Kirkham B, et al. Ectopic lymphoid structures support ongoing production of class-switched autoantibodies in rheumatoid synovium. PLoS Med (2009) 6:e1. doi:10.1371/journal.pmed.0060001
Pubmed Abstract | Pubmed Full Text | CrossRef Full Text | Google Scholar
51. Hayasaka H, Taniguchi K, Fukai S, Miyasaka M. Neogenesis and development of the high endothelial venules that mediate lymphocyte trafficking. Cancer Sci (2010) 101:2302–8. doi:10.1111/j.1349-7006.2010.01687.x
Pubmed Abstract | Pubmed Full Text | CrossRef Full Text | Google Scholar
52. Miyasaka M, Tanaka T. Lymphocyte trafficking across high endothelial venules: dogmas and enigmas. Nat Rev Immunol (2004) 4:360–70. doi:10.1038/nri1354
53. de Chaisemartin L, Goc J, Damotte D, Validire P, Magdeleinat P, Alifano M, et al. Characterization of chemokines and adhesion molecules associated with T cell presence in tertiary lymphoid structures in human lung cancer. Cancer Res (2011) 71:6391–9. doi:10.1158/0008-5472.CAN-11-0952
Pubmed Abstract | Pubmed Full Text | CrossRef Full Text | Google Scholar
54. Martinet L, Garrido I, Girard J-P. Tumor high endothelial venules (HEVs) predict lymphocyte infiltration and favorable prognosis in breast cancer. Oncoimmunology (2012) 1:789–90. doi:10.4161/onci.19787
Pubmed Abstract | Pubmed Full Text | CrossRef Full Text | Google Scholar
55. Martinet L, Le Guellec S, Filleron T, Lamant L, Meyer N, Rochaix P, et al. High endothelial venules (HEVs) in human melanoma lesions: major gateways for tumor-infiltrating lymphocytes. Oncoimmunology (2012) 1:829–39. doi:10.4161/onci.20492
Pubmed Abstract | Pubmed Full Text | CrossRef Full Text | Google Scholar
56. Martinet L, Garrido I, Filleron T, Le Guellec S, Bellard E, Fournie J-J, et al. Human solid tumors contain high endothelial venules: association with T- and B-lymphocyte infiltration and favorable prognosis in breast cancer. Cancer Res (2011) 71:5678–87. doi:10.1158/0008-5472.CAN-11-0431
Pubmed Abstract | Pubmed Full Text | CrossRef Full Text | Google Scholar
57. Platonova S, Cherfils-Vicini J, Damotte D, Crozet L, Vieillard V, Validire P, et al. Profound coordinated alterations of intratumoral NK cell phenotype and function in lung carcinoma. Cancer Res (2011) 71:5412–22. doi:10.1158/0008-5472.CAN-10-4179
Pubmed Abstract | Pubmed Full Text | CrossRef Full Text | Google Scholar
58. Ferlazzo G, Pack M, Thomas D, Paludan C, Schmid D, Strowig T, et al. Distinct roles of IL-12 and IL-15 in human natural killer cell activation by dendritic cells from secondary lymphoid organs. Proc Natl Acad Sci U S A (2004) 101:16606–11. doi:10.1073/pnas.0407522101
Pubmed Abstract | Pubmed Full Text | CrossRef Full Text | Google Scholar
59. Bajénoff M, Breart B, Huang AYC, Qi H, Cazareth J, Braud VM, et al. Natural killer cell behavior in lymph nodes revealed by static and real-time imaging. J Exp Med (2006) 203:619–31. doi:10.1084/jem.20051474
Pubmed Abstract | Pubmed Full Text | CrossRef Full Text | Google Scholar
60. Bihl F, Pecheur J, Bréart B, Poupon G, Cazareth J, Julia V, et al. Primed antigen-specific CD4+ T cells are required for NK cell activation in vivo upon Leishmania major infection. J Immunol (2010) 185:2174–81. doi:10.4049/jimmunol.1001486
Pubmed Abstract | Pubmed Full Text | CrossRef Full Text | Google Scholar
61. Beuneu H, Deguine J, Breart B, Mandelboim O, Di Santo JP, Bousso P. Dynamic behavior of NK cells during activation in lymph nodes. Blood (2009) 114:3227–34. doi:10.1182/blood-2009-06-228759
62. Walzer T, Dalod M, Robbins SH, Zitvogel L, Vivier E. Natural-killer cells and dendritic cells: “l’union fait la force.”. Blood (2005) 106:2252–8. doi:10.1182/blood-2005-03-1154
Pubmed Abstract | Pubmed Full Text | CrossRef Full Text | Google Scholar
63. Martín-Fontecha A, Thomsen LL, Brett S, Gerard C, Lipp M, Lanzavecchia A, et al. Induced recruitment of NK cells to lymph nodes provides IFN-gamma for T(H)1 priming. Nat Immunol (2004) 5:1260–5. doi:10.1038/ni1138
Pubmed Abstract | Pubmed Full Text | CrossRef Full Text | Google Scholar
64. Bihl F, Germain C, Luci C, Braud VM. Mechanisms of NK cell activation: CD4(+) T cells enter the scene. Cell Mol Life Sci (2011) 68:3457–67. doi:10.1007/s00018-011-0796-1
Pubmed Abstract | Pubmed Full Text | CrossRef Full Text | Google Scholar
65. Moretta A, Marcenaro E, Parolini S, Ferlazzo G, Moretta L. NK cells at the interface between innate and adaptive immunity. Cell Death Differ (2007) 15:226–33. doi:10.1038/sj.cdd.4402170
66. Mamessier E, Sylvain A, Thibult M-L, Houvenaeghel G, Jacquemier J, Castellano R, et al. Human breast cancer cells enhance self tolerance by promoting evasion from NK cell antitumor immunity. J Clin Invest (2011) 121:3609–22. doi:10.1172/JCI45816
Pubmed Abstract | Pubmed Full Text | CrossRef Full Text | Google Scholar
67. Mebius RE. Organogenesis of lymphoid tissues. Nat Rev Immunol (2003) 3:292–303. doi:10.1038/nri1054
68. Van de Pavert SA, Olivier BJ, Goverse G, Vondenhoff MF, Greuter M, Beke P, et al. Chemokine CXCL13 is essential for lymph node initiation and is induced by retinoic acid and neuronal stimulation. Nat Immunol (2009) 10:1193–9. doi:10.1038/ni.1789
Pubmed Abstract | Pubmed Full Text | CrossRef Full Text | Google Scholar
69. Ngo VN, Korner H, Gunn MD, Schmidt KN, Riminton DS, Cooper MD, et al. Lymphotoxin alpha/beta and tumor necrosis factor are required for stromal cell expression of homing chemokines in B and T cell areas of the spleen. J Exp Med (1999) 189:403–12. doi:10.1084/jem.189.2.403
Pubmed Abstract | Pubmed Full Text | CrossRef Full Text | Google Scholar
70. Drayton DL, Ying X, Lee J, Lesslauer W, Ruddle NH. Ectopic LT alpha beta directs lymphoid organ neogenesis with concomitant expression of peripheral node addressin and a HEV-restricted sulfotransferase. J Exp Med (2003) 197:1153–63. doi:10.1084/jem.20021761
Pubmed Abstract | Pubmed Full Text | CrossRef Full Text | Google Scholar
71. Liao S, Ruddle NH. Synchrony of high endothelial venules and lymphatic vessels revealed by immunization. J Immunol (2006) 177:3369–79. doi:10.4049/jimmunol.177.5.3369
Pubmed Abstract | Pubmed Full Text | CrossRef Full Text | Google Scholar
72. Onder L, Danuser R, Scandella E, Firner S, Chai Q, Hehlgans T, et al. Endothelial cell-specific lymphotoxin-β receptor signaling is critical for lymph node and high endothelial venule formation. J Exp Med (2013) 210:465–73. doi:10.1084/jem.20121462
Pubmed Abstract | Pubmed Full Text | CrossRef Full Text | Google Scholar
73. Cyster JG. Lymphoid organ development and cell migration. Immunol Rev (2003) 195:5–14. doi:10.1034/j.1600-065X.2003.00075.x
74. Ammirante M, Luo J-L, Grivennikov S, Nedospasov S, Karin M. B-cell-derived lymphotoxin promotes castration-resistant prostate cancer. Nature (2010) 464:302–5. doi:10.1038/nature08782
Pubmed Abstract | Pubmed Full Text | CrossRef Full Text | Google Scholar
75. Ansel KM, Ngo VN, Hyman PL, Luther SA, Förster R, Sedgwick JD, et al. A chemokine-driven positive feedback loop organizes lymphoid follicles. Nature (2000) 406:309–14. doi:10.1038/35018581
Pubmed Abstract | Pubmed Full Text | CrossRef Full Text | Google Scholar
76. Browning JL, Allaire N, Ngam-Ek A, Notidis E, Hunt J, Perrin S, et al. Lymphotoxin-beta receptor signaling is required for the homeostatic control of HEV differentiation and function. Immunity (2005) 23:539–50. doi:10.1016/j.immuni.2005.10.002
Pubmed Abstract | Pubmed Full Text | CrossRef Full Text | Google Scholar
77. Kumar V, Scandella E, Danuser R, Onder L, Nitschké M, Fukui Y, et al. Global lymphoid tissue remodeling during a viral infection is orchestrated by a B cell-lymphotoxin-dependent pathway. Blood (2010) 115:4725–33. doi:10.1182/blood-2009-10-250118
Pubmed Abstract | Pubmed Full Text | CrossRef Full Text | Google Scholar
78. Moussion C, Girard J-P. Dendritic cells control lymphocyte entry to lymph nodes through high endothelial venules. Nature (2011) 479:542–6. doi:10.1038/nature10540
Pubmed Abstract | Pubmed Full Text | CrossRef Full Text | Google Scholar
79. Wendland M, Willenzon S, Kocks J, Davalos-Misslitz AC, Hammerschmidt SI, Schumann K, et al. Lymph node T cell homeostasis relies on steady state homing of dendritic cells. Immunity (2011) 35:945–57. doi:10.1016/j.immuni.2011.10.017
Pubmed Abstract | Pubmed Full Text | CrossRef Full Text | Google Scholar
80. Sozzani S, Rusnati M, Riboldi E, Mitola S, Presta M. Dendritic cell-endothelial cell cross-talk in angiogenesis. Trends Immunol (2007) 28:385–92. doi:10.1016/j.it.2007.07.006
Pubmed Abstract | Pubmed Full Text | CrossRef Full Text | Google Scholar
81. Webster B, Ekland EH, Agle LM, Chyou S, Ruggieri R, Lu TT. Regulation of lymph node vascular growth by dendritic cells. J Exp Med (2006) 203:1903–13. doi:10.1084/jem.20052272
Pubmed Abstract | Pubmed Full Text | CrossRef Full Text | Google Scholar
82. Benahmed F, Chyou S, Dasoveanu D, Chen J, Kumar V, Iwakura Y, et al. Multiple CD11c+ cells collaboratively express IL-1β to modulate stromal vascular endothelial growth factor and lymph node vascular-stromal growth. J Immunol (2014) 192:4153–63. doi:10.4049/jimmunol.1301765
Pubmed Abstract | Pubmed Full Text | CrossRef Full Text | Google Scholar
83. Angeli V, Ginhoux F, Llodrà J, Quemeneur L, Frenette PS, Skobe M, et al. B cell-driven lymphangiogenesis in inflamed lymph nodes enhances dendritic cell mobilization. Immunity (2006) 24:203–15. doi:10.1016/j.immuni.2006.01.003
Pubmed Abstract | Pubmed Full Text | CrossRef Full Text | Google Scholar
84. Marinkovic T, Garin A, Yokota Y, Fu Y-X, Ruddle NH, Furtado GC, et al. Interaction of mature CD3+CD4+ T cells with dendritic cells triggers the development of tertiary lymphoid structures in the thyroid. J Clin Invest (2006) 116:2622–32. doi:10.1172/JCI28993
Pubmed Abstract | Pubmed Full Text | CrossRef Full Text | Google Scholar
85. Gräbner R, Lötzer K, Döpping S, Hildner M, Radke D, Beer M, et al. Lymphotoxin beta receptor signaling promotes tertiary lymphoid organogenesis in the aorta adventitia of aged ApoE-/- mice. J Exp Med (2009) 206:233–48. doi:10.1084/jem.20080752
Pubmed Abstract | Pubmed Full Text | CrossRef Full Text | Google Scholar
86. Guedj K, Khallou-Laschet J, Clement M, Morvan M, Gaston A-T, Fornasa G, et al. M1 macrophages act as LTβR-independent lymphoid tissue inducer cells during atherosclerosis-related lymphoid neogenesis. Cardiovasc Res (2014) 101:434–43. doi:10.1093/cvr/cvt263
Pubmed Abstract | Pubmed Full Text | CrossRef Full Text | Google Scholar
87. Martinet L, Girard J-P. Regulation of tumor-associated high-endothelial venules by dendritic cells. Oncoimmunology (2013) 2:e26470. doi:10.4161/onci.26470
Pubmed Abstract | Pubmed Full Text | CrossRef Full Text | Google Scholar
88. Martinet L, Filleron T, Le Guellec S, Rochaix P, Garrido I, Girard J-P. High endothelial venule blood vessels for tumor-infiltrating lymphocytes are associated with lymphotoxin β-producing dendritic cells in human breast cancer. J Immunol (2013) 191:2001–8. doi:10.4049/jimmunol.1300872
Pubmed Abstract | Pubmed Full Text | CrossRef Full Text | Google Scholar
89. Halle S, Dujardin HC, Bakocevic N, Fleige H, Danzer H, Willenzon S, et al. Induced bronchus-associated lymphoid tissue serves as a general priming site for T cells and is maintained by dendritic cells. J Exp Med (2009) 206:2593–601. doi:10.1084/jem.20091472
Pubmed Abstract | Pubmed Full Text | CrossRef Full Text | Google Scholar
90. Sallusto F, Lanzavecchia A. Human Th17 cells in infection and autoimmunity. Microbes Infect Inst Pasteur (2009) 11:620–4. doi:10.1016/j.micinf.2009.04.004
Pubmed Abstract | Pubmed Full Text | CrossRef Full Text | Google Scholar
91. Alizadeh D, Katsanis E, Larmonier N. The multifaceted role of Th17 lymphocytes and their associated cytokines in cancer. Clin Dev Immunol (2013) 2013:957878. doi:10.1155/2013/957878
Pubmed Abstract | Pubmed Full Text | CrossRef Full Text | Google Scholar
92. Grogan JL, Ouyang W. A role for Th17 cells in the regulation of tertiary lymphoid follicles. Eur J Immunol (2012) 42:2255–62. doi:10.1002/eji.201242656
Pubmed Abstract | Pubmed Full Text | CrossRef Full Text | Google Scholar
93. Rangel-Moreno J, Carragher DM, de la Luz Garcia-Hernandez M, Hwang JY, Kusser K, Hartson L, et al. The development of inducible bronchus-associated lymphoid tissue depends on IL-17. Nat Immunol (2011) 12:639–46. doi:10.1038/ni.2053
Pubmed Abstract | Pubmed Full Text | CrossRef Full Text | Google Scholar
94. Fleige H, Haas JD, Stahl FR, Willenzon S, Prinz I, Förster R. Induction of BALT in the absence of IL-17. Nat Immunol (2012) 13:1. doi:10.1038/ni.2167
95. Fleige H, Ravens S, Moschovakis GL, Bölter J, Willenzon S, Sutter G, et al. IL-17-induced CXCL12 recruits B cells and induces follicle formation in BALT in the absence of differentiated FDCs. J Exp Med (2014) 211:643–51. doi:10.1084/jem.20131737
Pubmed Abstract | Pubmed Full Text | CrossRef Full Text | Google Scholar
96. Peters A, Pitcher LA, Sullivan JM, Mitsdoerffer M, Acton SE, Franz B, et al. Th17 cells induce ectopic lymphoid follicles in central nervous system tissue inflammation. Immunity (2011) 35:986–96. doi:10.1016/j.immuni.2011.10.015
Pubmed Abstract | Pubmed Full Text | CrossRef Full Text | Google Scholar
97. Deteix C, Attuil-Audenis V, Duthey A, Patey N, McGregor B, Dubois V, et al. Intragraft Th17 infiltrate promotes lymphoid neogenesis and hastens clinical chronic rejection. J Immunol (2010) 184:5344–51. doi:10.4049/jimmunol.0902999
Pubmed Abstract | Pubmed Full Text | CrossRef Full Text | Google Scholar
98. Kocks JR, Davalos-Misslitz ACM, Hintzen G, Ohl L, Förster R. Regulatory T cells interfere with the development of bronchus-associated lymphoid tissue. J Exp Med (2007) 204:723–34. doi:10.1084/jem.20061424
Pubmed Abstract | Pubmed Full Text | CrossRef Full Text | Google Scholar
99. Hindley JP, Jones E, Smart K, Bridgeman H, Lauder SN, Ondondo B, et al. T-cell trafficking facilitated by high endothelial venules is required for tumor control after regulatory T-cell depletion. Cancer Res (2012) 72:5473–82. doi:10.1158/0008-5472.CAN-12-1912
Pubmed Abstract | Pubmed Full Text | CrossRef Full Text | Google Scholar
100. DeNardo DG, Coussens LM. Inflammation and breast cancer. Balancing immune response: crosstalk between adaptive and innate immune cells during breast cancer progression. Breast Cancer Res (2007) 9:212. doi:10.1186/bcr1746
Pubmed Abstract | Pubmed Full Text | CrossRef Full Text | Google Scholar
101. Barbera-Guillem E, Nelson MB, Barr B, Nyhus JK, May KF Jr, Feng L, et al. B lymphocyte pathology in human colorectal cancer. Experimental and clinical therapeutic effects of partial B cell depletion. Cancer Immunol Immunother (2000) 48:541–9. doi:10.1007/PL00006672
Pubmed Abstract | Pubmed Full Text | CrossRef Full Text | Google Scholar
102. De Visser KE, Korets LV, Coussens LM. De novo carcinogenesis promoted by chronic inflammation is B lymphocyte dependent. Cancer Cell (2005) 7:411–23. doi:10.1016/j.ccr.2005.04.014
Pubmed Abstract | Pubmed Full Text | CrossRef Full Text | Google Scholar
103. Tan T-T, Coussens LM. Humoral immunity, inflammation and cancer. Curr Opin Immunol (2007) 19:209–16. doi:10.1016/j.coi.2007.01.001
104. Barbera-Guillem E, May KF Jr, Nyhus JK, Nelson MB. Promotion of tumor invasion by cooperation of granulocytes and macrophages activated by anti-tumor antibodies. Neoplasia (1999) 1:453. doi:10.1038/sj.neo.7900054
Pubmed Abstract | Pubmed Full Text | CrossRef Full Text | Google Scholar
105. DeNardo DG, Andreu P, Coussens LM. Interactions between lymphocytes and myeloid cells regulate pro- versus anti-tumor immunity. Cancer Metastasis Rev (2010) 29:309–16. doi:10.1007/s10555-010-9223-6
Pubmed Abstract | Pubmed Full Text | CrossRef Full Text | Google Scholar
106. Olkhanud PB, Damdinsuren B, Bodogai M, Gress RE, Sen R, Wejksza K, et al. Tumor-evoked regulatory B cells promote breast cancer metastasis by converting resting CD4+ T cells to T-regulatory cells. Cancer Res (2011) 71:3505–15. doi:10.1158/0008-5472.CAN-10-4316
Pubmed Abstract | Pubmed Full Text | CrossRef Full Text | Google Scholar
107. Horikawa M, Minard-Colin V, Matsushita T, Tedder TF. Regulatory B cell production of IL-10 inhibits lymphoma depletion during CD20 immunotherapy in mice. J Clin Invest (2011) 121:4268–80. doi:10.1172/JCI59266
Pubmed Abstract | Pubmed Full Text | CrossRef Full Text | Google Scholar
108. DiLillo DJ, Yanaba K, Tedder TF. B cells are required for optimal CD4+ and CD8+ T cell tumor immunity: therapeutic B cell depletion enhances B16 melanoma growth in mice. J Immunol (2010) 184:4006–16. doi:10.4049/jimmunol.0903009
Pubmed Abstract | Pubmed Full Text | CrossRef Full Text | Google Scholar
109. Litsiou E, Semitekolou M, Galani IE, Morianos I, Tsoutsa A, Kara P, et al. CXCL13 production in B cells via Toll-like receptor/lymphotoxin receptor signaling is involved in lymphoid neogenesis in chronic obstructive pulmonary disease. Am J Respir Crit Care Med (2013) 187:1194–202. doi:10.1164/rccm.201208-1543OC
Pubmed Abstract | Pubmed Full Text | CrossRef Full Text | Google Scholar
110. Kim HJ, Krenn V, Steinhauser G, Berek C. Plasma cell development in synovial germinal centers in patients with rheumatoid and reactive arthritis. J Immunol (1999) 162:3053–62.
111. Qin Y, Duquette P, Zhang Y, Talbot P, Poole R, Antel J. Clonal expansion and somatic hypermutation of V(H) genes of B cells from cerebrospinal fluid in multiple sclerosis. J Clin Invest (1998) 102:1045–50. doi:10.1172/JCI3568
Pubmed Abstract | Pubmed Full Text | CrossRef Full Text | Google Scholar
112. Schröder AE, Greiner A, Seyfert C, Berek C. Differentiation of B cells in the nonlymphoid tissue of the synovial membrane of patients with rheumatoid arthritis. Proc Natl Acad Sci U S A (1996) 93:221–5. doi:10.1073/pnas.93.1.221
Pubmed Abstract | Pubmed Full Text | CrossRef Full Text | Google Scholar
113. Dörner T, Hansen A, Jacobi A, Lipsky PE. Immunoglobulin repertoire analysis provides new insights into the immunopathogenesis of Sjögren’s syndrome. Autoimmun Rev (2002) 1:119–24. doi:10.1016/S1568-9972(02)00029-0
Pubmed Abstract | Pubmed Full Text | CrossRef Full Text | Google Scholar
114. Sims GP, Shiono H, Willcox N, Stott DI. Somatic hypermutation and selection of B cells in thymic germinal centers responding to acetylcholine receptor in myasthenia gravis. J Immunol (2001) 167:1935–44. doi:10.4049/jimmunol.167.4.1935
Pubmed Abstract | Pubmed Full Text | CrossRef Full Text | Google Scholar
115. Stott DI, Hiepe F, Hummel M, Steinhauser G, Berek C. Antigen-driven clonal proliferation of B cells within the target tissue of an autoimmune disease. The salivary glands of patients with Sjögren’s syndrome. J Clin Invest (1998) 102:938–46. doi:10.1172/JCI3234
Pubmed Abstract | Pubmed Full Text | CrossRef Full Text | Google Scholar
116. Coughlin CM, Vance BA, Grupp SA, Vonderheide RH. RNA-transfected CD40-activated B cells induce functional T-cell responses against viral and tumor antigen targets: implications for pediatric immunotherapy. Blood (2004) 103:2046–54. doi:10.1182/blood-2003-07-2379
Pubmed Abstract | Pubmed Full Text | CrossRef Full Text | Google Scholar
117. Yuseff M-I, Pierobon P, Reversat A, Lennon-Duménil A-M. How B cells capture, process and present antigens: a crucial role for cell polarity. Nat Rev Immunol (2013) 13:475–86. doi:10.1038/nri3469
Pubmed Abstract | Pubmed Full Text | CrossRef Full Text | Google Scholar
118. Galibert L, Burdin N, de Saint-Vis B, Garrone P, Van Kooten C, Banchereau J, et al. CD40 and B cell antigen receptor dual triggering of resting B lymphocytes turns on a partial germinal center phenotype. J Exp Med (1996) 183:77–85. doi:10.1084/jem.183.1.77
Pubmed Abstract | Pubmed Full Text | CrossRef Full Text | Google Scholar
119. Harp CT, Lovett-Racke AE, Racke MK, Frohman EM, Monson NL. Impact of myelin-specific antigen presenting B cells on T cell activation in multiple sclerosis. Clin Immunol (2008) 128:382–91. doi:10.1016/j.clim.2008.05.002
Pubmed Abstract | Pubmed Full Text | CrossRef Full Text | Google Scholar
120. Serreze DV, Fleming SA, Chapman HD, Richard SD, Leiter EH, Tisch RM. B lymphocytes are critical antigen-presenting cells for the initiation of T cell-mediated autoimmune diabetes in nonobese diabetic mice. J Immunol (1998) 161:3912–8.
121. Bouaziz J-D, Yanaba K, Venturi GM, Wang Y, Tisch RM, Poe JC, et al. Therapeutic B cell depletion impairs adaptive and autoreactive CD4+ T cell activation in mice. Proc Natl Acad Sci U S A (2007) 104:20878–83. doi:10.1073/pnas.0709205105
Pubmed Abstract | Pubmed Full Text | CrossRef Full Text | Google Scholar
122. Gnjatic S, Atanackovic D, Matsuo M, Jäger E, Lee SY, Valmori D, et al. Cross-presentation of HLA class I epitopes from exogenous NY-ESO-1 polypeptides by nonprofessional APCs. J Immunol (2003) 170:1191–6. doi:10.4049/jimmunol.170.3.1191
Pubmed Abstract | Pubmed Full Text | CrossRef Full Text | Google Scholar
123. Ahmadi T, Flies A, Efebera Y, Sherr DH. CD40 Ligand-activated, antigen-specific B cells are comparable to mature dendritic cells in presenting protein antigens and major histocompatibility complex class I- and class II-binding peptides. Immunology (2008) 124:129–40. doi:10.1111/j.1365-2567.2007.02749.x
Pubmed Abstract | Pubmed Full Text | CrossRef Full Text | Google Scholar
124. De Wit J, Souwer Y, Jorritsma T, Klaasse Bos H, ten Brinke A, Neefjes J, et al. Antigen-specific B cells reactivate an effective cytotoxic T cell response against phagocytosed Salmonella through cross-presentation. PLoS One (2010) 5:e13016. doi:10.1371/journal.pone.0013016
Pubmed Abstract | Pubmed Full Text | CrossRef Full Text | Google Scholar
125. Ronchese F, Hausmann B. B lymphocytes in vivo fail to prime naive T cells but can stimulate antigen-experienced T lymphocytes. J Exp Med (1993) 177:679–90. doi:10.1084/jem.177.3.679
Pubmed Abstract | Pubmed Full Text | CrossRef Full Text | Google Scholar
126. Yasuda M, Takenoyama M, Obata Y, Sugaya M, So T, Hanagiri T, et al. Tumor-infiltrating B lymphocytes as a potential source of identifying tumor antigen in human lung cancer. Cancer Res (2002) 62:1751–6.
127. Shi J-Y, Gao Q, Wang Z-C, Zhou J, Wang X-Y, Min Z-H, et al. Margin-infiltrating CD20(+) B cells display an atypical memory phenotype and correlate with favorable prognosis in hepatocellular carcinoma. Clin Cancer Res (2013) 19:5994–6005. doi:10.1158/1078-0432.CCR-12-3497
Pubmed Abstract | Pubmed Full Text | CrossRef Full Text | Google Scholar
128. Deola S, Panelli MC, Maric D, Selleri S, Dmitrieva NI, Voss CY, et al. Helper B cells promote cytotoxic T cell survival and proliferation independently of antigen presentation through CD27/CD70 interactions. J Immunol (2008) 180:1362–72. doi:10.4049/jimmunol.180.3.1362
Pubmed Abstract | Pubmed Full Text | CrossRef Full Text | Google Scholar
129. Shiku H, Takahashi T. Autologous typing: a tedious but orthodox approach for defining human tumor antigens with clarity. Cancer Immun (2012) 12:3.
130. Old LJ. Cancer immunology: the search for specificity – G. H. A. Clowes memorial lecture. Cancer Res (1981) 41:361–75.
131. Türeci O, Sahin U, Pfreundschuh M. Serological analysis of human tumor antigens: molecular definition and implications. Mol Med Today (1997) 3:342–9. doi:10.1016/S1357-4310(97)01081-2
Pubmed Abstract | Pubmed Full Text | CrossRef Full Text | Google Scholar
132. Scanlan MJ, Gure AO, Jungbluth AA, Old LJ, Chen Y-T. Cancer/testis antigens: an expanding family of targets for cancer immunotherapy. Immunol Rev (2002) 188:22–32. doi:10.1034/j.1600-065X.2002.18803.x
Pubmed Abstract | Pubmed Full Text | CrossRef Full Text | Google Scholar
133. Gnjatic S, Nishikawa H, Jungbluth AA, Güre AO, Ritter G, Jäger E, et al. NY-ESO-1: review of an immunogenic tumor antigen. Adv Cancer Res (2006) 95:1–30. doi:10.1016/S0065-230X(06)95001-5
Pubmed Abstract | Pubmed Full Text | CrossRef Full Text | Google Scholar
134. Simpson AJG, Caballero OL, Jungbluth A, Chen Y-T, Old LJ. Cancer/testis antigens, gametogenesis and cancer. Nat Rev Cancer (2005) 5:615–25. doi:10.1038/nrc1669
Pubmed Abstract | Pubmed Full Text | CrossRef Full Text | Google Scholar
135. Stockert E, Jäger E, Chen Y-T, Scanlan MJ, Gout I, Karbach J, et al. A survey of the humoral immune response of cancer patients to a panel of human tumor antigens. J Exp Med (1998) 187:1349. doi:10.1084/jem.187.8.1349
Pubmed Abstract | Pubmed Full Text | CrossRef Full Text | Google Scholar
136. Meneses A, Verastegui E, Barrera JL, de la Garza J, Hadden JW. Lymph node histology in head and neck cancer: impact of immunotherapy with IRX-2. Int Immunopharmacol (2003) 3:1083–91. doi:10.1016/S1567-5769(03)00017-1
Pubmed Abstract | Pubmed Full Text | CrossRef Full Text | Google Scholar
137. Gure AO, Chua R, Williamson B, Gonen M, Ferrera CA, Gnjatic S, et al. Cancer-testis genes are coordinately expressed and are markers of poor outcome in non-small cell lung cancer. Clin Cancer Res (2005) 11:8055–62. doi:10.1158/1078-0432.CCR-05-1203
Pubmed Abstract | Pubmed Full Text | CrossRef Full Text | Google Scholar
138. Theurillat J-P, Ingold F, Frei C, Zippelius A, Varga Z, Seifert B, et al. NY-ESO-1 protein expression in primary breast carcinoma and metastases: correlation with CD8+ T-cell and CD79a+ plasmacytic/B-cell infiltration. Int J Cancer (2007) 120:2411–7. doi:10.1002/ijc.22376
Pubmed Abstract | Pubmed Full Text | CrossRef Full Text | Google Scholar
139. Woo JR, Liss MA, Muldong MT, Palazzi K, Strasner A, Ammirante M, et al. Tumor infiltrating B-cells are increased in prostate cancer tissue. J Transl Med (2014) 12:30. doi:10.1186/1479-5876-12-30
Pubmed Abstract | Pubmed Full Text | CrossRef Full Text | Google Scholar
140. Dong HP, Elstrand MB, Holth A, Silins I, Berner A, Trope CG, et al. NK- and B-cell infiltration correlates with worse outcome in metastatic ovarian carcinoma. Am J Clin Pathol (2006) 125:451–8. doi:10.1309/15B6-6DQM-FYYM-78CJ
Pubmed Abstract | Pubmed Full Text | CrossRef Full Text | Google Scholar
141. Reilly RT, Emens LA, Jaffee EM. Humoral and cellular immune responses: independent forces or collaborators in the fight against cancer? Curr Opin Investig Drugs (2001) 2:133–5.
142. DeLeo AB, Jay G, Appella E, Dubois GC, Law LW, Old LJ. Detection of a transformation-related antigen in chemically induced sarcomas and other transformed cells of the mouse. Proc Natl Acad Sci U S A (1979) 76:2420–4. doi:10.1073/pnas.76.5.2420
Pubmed Abstract | Pubmed Full Text | CrossRef Full Text | Google Scholar
143. Tsuji T, Matsuzaki J, Ritter E, Miliotto A, Ritter G, Odunsi K, et al. Split T cell tolerance against a self/tumor antigen: spontaneous CD4+ but not CD8+ T cell responses against p53 in cancer patients and healthy donors. PLoS One (2011) 6:e23651. doi:10.1371/journal.pone.0023651
Pubmed Abstract | Pubmed Full Text | CrossRef Full Text | Google Scholar
144. Jäger E, Nagata Y, Gnjatic S, Wada H, Stockert E, Karbach J, et al. Monitoring CD8 T cell responses to NY-ESO-1: correlation of humoral and cellular immune responses. Proc Natl Acad Sci U S A (2000) 97:4760–5. doi:10.1073/pnas.97.9.4760
Pubmed Abstract | Pubmed Full Text | CrossRef Full Text | Google Scholar
145. Gnjatic S, Atanackovic D, Jäger E, Matsuo M, Selvakumar A, Altorki NK, et al. Survey of naturally occurring CD4+ T cell responses against NY-ESO-1 in cancer patients: correlation with antibody responses. Proc Natl Acad Sci U S A (2003) 100:8862–7. doi:10.1073/pnas.1133324100
Pubmed Abstract | Pubmed Full Text | CrossRef Full Text | Google Scholar
146. Matsuzaki J, Gnjatic S, Mhawech-Fauceglia P, Beck A, Miller A, Tsuji T, et al. Tumor-infiltrating NY-ESO-1-specific CD8+ T cells are negatively regulated by LAG-3 and PD-1 in human ovarian cancer. Proc Natl Acad Sci U S A (2010) 107:7875–80. doi:10.1073/pnas.1003345107
Pubmed Abstract | Pubmed Full Text | CrossRef Full Text | Google Scholar
147. Ayyoub M, Taub RN, Keohan M-L, Hesdorffer M, Metthez G, Memeo L, et al. The frequent expression of cancer/testis antigens provides opportunities for immunotherapeutic targeting of sarcoma. Cancer Immun (2004) 4:7.
148. Hamaï A, Duperrier-Amouriaux K, Pignon P, Raimbaud I, Memeo L, Colarossi C, et al. Antibody responses to NY-ESO-1 in primary breast cancer identify a subtype target for immunotherapy. PLoS One (2011) 6:e21129. doi:10.1371/journal.pone.0021129
Pubmed Abstract | Pubmed Full Text | CrossRef Full Text | Google Scholar
149. Gnjatic S, Ritter E, Buchler MW, Giese NA, Brors B, Frei C, et al. Seromic profiling of ovarian and pancreatic cancer. Proc Natl Acad Sci U S A (2010) 107:5088–93. doi:10.1073/pnas.0914213107
Pubmed Abstract | Pubmed Full Text | CrossRef Full Text | Google Scholar
150. Reuschenbach M, von Knebel Doeberitz M, Wentzensen N. A systematic review of humoral immune responses against tumor antigens. Cancer Immunol Immunother (2009) 58:1535–44. doi:10.1007/s00262-009-0733-4
151. Healey GF, Lam S, Boyle P, Hamilton-Fairley G, Peek LJ, Robertson JFR. Signal stratification of autoantibody levels in serum samples and its application to the early detection of lung cancer. J Thorac Dis (2013) 5:618–25. doi:10.3978/j.issn.2072-1439.2013.08.65
Pubmed Abstract | Pubmed Full Text | CrossRef Full Text | Google Scholar
152. Leidinger P, Keller A, Heisel S, Ludwig N, Rheinheimer S, Klein V, et al. Identification of lung cancer with high sensitivity and specificity by blood testing. Respir Res (2010) 11:18. doi:10.1186/1465-9921-11-18
Pubmed Abstract | Pubmed Full Text | CrossRef Full Text | Google Scholar
153. Desmetz C, Mange A, Maudelonde T, Solassol J. Autoantibody signatures: progress and perspectives for early cancer detection. J Cell Mol Med (2011) 15:2013–24. doi:10.1111/j.1582-4934.2011.01355.x
Pubmed Abstract | Pubmed Full Text | CrossRef Full Text | Google Scholar
154. Posner JB. Immunology of paraneoplastic syndromes: overview. Ann N Y Acad Sci (2003) 998:178–86. doi:10.1196/annals.1254.018
Pubmed Abstract | Pubmed Full Text | CrossRef Full Text | Google Scholar
155. Nzula S, Going JJ, Stott DI. Antigen-driven clonal proliferation, somatic hypermutation, and selection of B lymphocytes infiltrating human ductal breast carcinomas. Cancer Res (2003) 63:3275–80.
156. Maletzki C, Jahnke A, Ostwald C, Klar E, Prall F, Linnebacher M. Ex-vivo clonally expanded B lymphocytes infiltrating colorectal carcinoma are of mature immunophenotype and produce functional IgG. PLoS One (2012) 7:e32639. doi:10.1371/journal.pone.0032639
Pubmed Abstract | Pubmed Full Text | CrossRef Full Text | Google Scholar
157. Balkwill F, Montfort A, Capasso M. B regulatory cells in cancer. Trends Immunol (2013) 34:169–73. doi:10.1016/j.it.2012.10.007
158. Lutz ER, Wu AA, Bigelow E, Sharma R, Mo G, Soares K, et al. Immunotherapy converts nonimmunogenic pancreatic tumors into immunogenic foci of immune regulation. Cancer Immunol Res (2014) 2:616–31. doi:10.1158/2326-6066.CIR-14-0027
Pubmed Abstract | Pubmed Full Text | CrossRef Full Text | Google Scholar
159. Foo SY, Phipps S. Regulation of inducible BALT formation and contribution to immunity and pathology. Mucosal Immunol (2010) 3:537–44. doi:10.1038/mi.2010.52
Pubmed Abstract | Pubmed Full Text | CrossRef Full Text | Google Scholar
160. Maldonado L, Teague JE, Morrow MP, Jotova I, Wu TC, Wang C, et al. Intramuscular therapeutic vaccination targeting HPV16 induces T cell responses that localize in mucosal lesions. Sci Transl Med (2014) 6:221ra13. doi:10.1126/scitranslmed.3007323
Pubmed Abstract | Pubmed Full Text | CrossRef Full Text | Google Scholar
161. Zitvogel L, Galluzzi L, Smyth MJ, Kroemer G. Mechanism of action of conventional and targeted anticancer therapies: reinstating immunosurveillance. Immunity (2013) 39:74–88. doi:10.1016/j.immuni.2013.06.014
Pubmed Abstract | Pubmed Full Text | CrossRef Full Text | Google Scholar
162. Apetoh L, Ghiringhelli F, Tesniere A, Obeid M, Ortiz C, Criollo A, et al. Toll-like receptor 4-dependent contribution of the immune system to anticancer chemotherapy and radiotherapy. Nat Med (2007) 13:1050–9. doi:10.1038/nm1622
163. Obeid M, Tesniere A, Ghiringhelli F, Fimia GM, Apetoh L, Perfettini J-L, et al. Calreticulin exposure dictates the immunogenicity of cancer cell death. Nat Med (2007) 13:54–61. doi:10.1038/nm1523
164. Ghiringhelli F, Apetoh L, Tesniere A, Aymeric L, Ma Y, Ortiz C, et al. Activation of the NLRP3 inflammasome in dendritic cells induces IL-1beta-dependent adaptive immunity against tumors. Nat Med (2009) 15:1170–8. doi:10.1038/nm.2028
Pubmed Abstract | Pubmed Full Text | CrossRef Full Text | Google Scholar
165. De Smet C, De Backer O, Faraoni I, Lurquin C, Brasseur F, Boon T. The activation of human gene MAGE-1 in tumor cells is correlated with genome-wide demethylation. Proc Natl Acad Sci U S A (1996) 93:7149–53. doi:10.1073/pnas.93.14.7149
Pubmed Abstract | Pubmed Full Text | CrossRef Full Text | Google Scholar
166. Schrama D, thor Straten P, Fischer WH, McLellan AD, Bröcker EB, Reisfeld RA, et al. Targeting of lymphotoxin-alpha to the tumor elicits an efficient immune response associated with induction of peripheral lymphoid-like tissue. Immunity (2001) 14:111–21. doi:10.1016/S1074-7613(01)00094-2
Pubmed Abstract | Pubmed Full Text | CrossRef Full Text | Google Scholar
167. Liang C, Zhong C, Sun R, Liu B, Huang C, Qin J, et al. Local expression of secondary lymphoid tissue chemokine delivered by adeno-associated virus within the tumor bed stimulates strong anti-liver tumor immunity. J Virol (2007) 81:9502–11. doi:10.1128/JVI.00208-07
Pubmed Abstract | Pubmed Full Text | CrossRef Full Text | Google Scholar
168. Irvine DJ, Stachowiak AN, Hori Y. Lymphoid tissue engineering: invoking lymphoid tissue neogenesis in immunotherapy and models of immunity. Semin Immunol (2008) 20:137–46. doi:10.1016/j.smim.2007.10.010
Pubmed Abstract | Pubmed Full Text | CrossRef Full Text | Google Scholar
169. Schaer DA, Hirschhorn-Cymerman D, Wolchok JD. Targeting tumor-necrosis factor receptor pathways for tumor immunotherapy. J Immunother Cancer (2014) 2:7. doi:10.1186/2051-1426-2-7
Pubmed Abstract | Pubmed Full Text | CrossRef Full Text | Google Scholar
170. White AL, Dou L, Chan HTC, Field VL, Mockridge CI, Moss K, et al. Fcγ receptor dependency of agonistic CD40 antibody in lymphoma therapy can be overcome through antibody multimerization. J Immunol (2014) 193:1828–35. doi:10.4049/jimmunol.1303204
Pubmed Abstract | Pubmed Full Text | CrossRef Full Text | Google Scholar
171. Thibult M-L, Rivals J-P, Mamessier E, Gertner-Dardenne J, Pastor S, Speiser DE, et al. CpG-ODN-induced sustained expression of BTLA mediating selective inhibition of human B cells. J Mol Med (Berl) (2013) 91:195–205. doi:10.1007/s00109-012-0943-7
Pubmed Abstract | Pubmed Full Text | CrossRef Full Text | Google Scholar
172. Noguchi T, Kato T, Wang L, Maeda Y, Ikeda H, Sato E, et al. Intracellular tumor-associated antigens represent effective targets for passive immunotherapy. Cancer Res (2012) 72:1672–82. doi:10.1158/0008-5472.CAN-11-3072
Pubmed Abstract | Pubmed Full Text | CrossRef Full Text | Google Scholar
173. Kalergis AM, Ravetch JV. Inducing tumor immunity through the selective engagement of activating Fcgamma receptors on dendritic cells. J Exp Med (2002) 195:1653–9. doi:10.1084/jem.20020338
Pubmed Abstract | Pubmed Full Text | CrossRef Full Text | Google Scholar
174. Chiriva-Internati M, Pandey A, Saba R, Kim M, Saadeh C, Lukman T, et al. Cancer testis antigens: a novel target in lung cancer. Int Rev Immunol (2012) 31:321–43. doi:10.3109/08830185.2012.723512
Pubmed Abstract | Pubmed Full Text | CrossRef Full Text | Google Scholar
175. Ghafouri-Fard S, Shamsi R, Seifi-Alan M, Javaheri M, Tabarestani S. Cancer-testis genes as candidates for immunotherapy in breast cancer. Immunotherapy (2014) 6:165–79. doi:10.2217/imt.13.165
176. Odunsi K, Jungbluth AA, Stockert E, Qian F, Gnjatic S, Tammela J, et al. NY-ESO-1 and LAGE-1 cancer-testis antigens are potential targets for immunotherapy in epithelial ovarian cancer. Cancer Res (2003) 63:6076–83.
177. Cheever MA, Allison JP, Ferris AS, Finn OJ, Hastings BM, Hecht TT, et al. The prioritization of cancer antigens: a national cancer institute pilot project for the acceleration of translational research. Clin Cancer Res (2009) 15:5323–37. doi:10.1158/1078-0432.CCR-09-0737
Pubmed Abstract | Pubmed Full Text | CrossRef Full Text | Google Scholar
178. Bioley G, Guillaume P, Luescher I, Bhardwaj N, Mears G, Old L, et al. Vaccination with a recombinant protein encoding the tumor-specific antigen NY-ESO-1 elicits an A2/157-165-specific CTL repertoire structurally distinct and of reduced tumor reactivity than that elicited by spontaneous immune responses to NY-ESO-1-expressing Tumors. J Immunother (2009) 32:161–8. doi:10.1097/CJI.0b013e31819302f6
Pubmed Abstract | Pubmed Full Text | CrossRef Full Text | Google Scholar
179. Valmori D, Souleimanian NE, Tosello V, Bhardwaj N, Adams S, O’Neill D, et al. Vaccination with NY-ESO-1 protein and CpG in Montanide induces integrated antibody/Th1 responses and CD8 T cells through cross- priming. Proc Natl Acad Sci U S A (2007) 104:8947–52. doi:10.1073/pnas.0703395104
Pubmed Abstract | Pubmed Full Text | CrossRef Full Text | Google Scholar
180. Yuan J, Adamow M, Ginsberg BA, Rasalan TS, Ritter E, Gallardo HF, et al. Integrated NY-ESO-1 antibody and CD8+ T-cell responses correlate with clinical benefit in advanced melanoma patients treated with ipilimumab. Proc Natl Acad Sci U S A (2011) 108:16723–8. doi:10.1073/pnas.1110814108
Pubmed Abstract | Pubmed Full Text | CrossRef Full Text | Google Scholar
Keywords: tertiary lymphoid structure, cancer, B cell, antibody, dendritic cell, anti-tumor immunity, biomarker, immunotherapy
Citation: Germain C, Gnjatic S and Dieu-Nosjean M-C (2015) Tertiary lymphoid structure-associated B cells are key players in anti-tumor immunity. Front. Immunol. 6:67. doi: 10.3389/fimmu.2015.00067
Received: 02 December 2014; Paper pending published: 04 January 2015;
Accepted: 02 February 2015; Published online: 23 February 2015.
Edited by:
José Mordoh, Fundación Instituto Leloir, ArgentinaReviewed by:
María Marcela Barrio, Fundación Cáncer FUCA, ArgentinaTheresa L. Whiteside, University of Pittsburgh Cancer Institute, USA
Copyright: © 2015 Germain, Gnjatic and Dieu-Nosjean. This is an open-access article distributed under the terms of the Creative Commons Attribution License (CC BY). The use, distribution or reproduction in other forums is permitted, provided the original author(s) or licensor are credited and that the original publication in this journal is cited, in accordance with accepted academic practice. No use, distribution or reproduction is permitted which does not comply with these terms.
*Correspondence: Marie-Caroline Dieu-Nosjean, Laboratory Cancer, Immune Control and Escape, Cordeliers Research Center, INSERM UMRS1138, 15, rue de l’école de Médecine, Paris Cedex 06 F-75270, France e-mail:mc.dieu-nosjean@crc.jussieu.fr