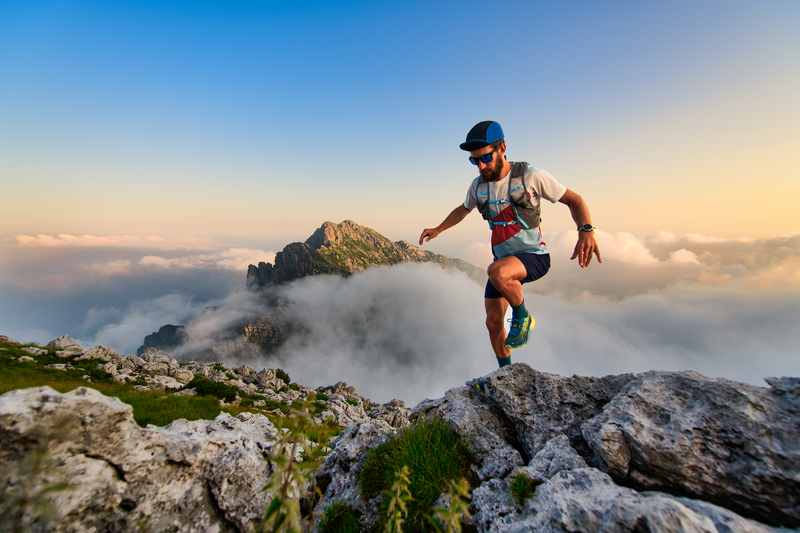
95% of researchers rate our articles as excellent or good
Learn more about the work of our research integrity team to safeguard the quality of each article we publish.
Find out more
REVIEW article
Front. Immunol. , 26 January 2015
Sec. T Cell Biology
Volume 6 - 2015 | https://doi.org/10.3389/fimmu.2015.00015
This article is part of the Research Topic Recent Advances in γδ T-Cell Biology: New Ligands, New Functions, Translational Perspective View all 28 articles
The contributions of γδ T-cells to immunity to infection or tumors critically depend on their activation and differentiation into effectors capable of secreting cytokines and killing infected or transformed cells. These processes are molecularly controlled by surface receptors that capture key extracellular cues and convey downstream intracellular signals that regulate γδ T-cell physiology. The understanding of how environmental signals are integrated by γδ T-cells is critical for their manipulation in clinical settings. Here, we discuss how different classes of surface receptors impact on human and murine γδ T-cell differentiation, activation, and expansion. In particular, we review the role of five receptor types: the T-cell receptor (TCR), costimulatory receptors, cytokine receptors, NK receptors, and inhibitory receptors. Some of the key players are the costimulatory receptors CD27 and CD28, which differentially impact on pro-inflammatory subsets of γδ T-cells; the cytokine receptors IL-2R, IL-7R, and IL-15R, which drive functional differentiation and expansion of γδ T-cells; the NK receptor NKG2D and its contribution to γδ T-cell cytotoxicity; and the inhibitory receptors PD-1 and BTLA that control γδ T-cell homeostasis. We discuss these and other receptors in the context of a five-step model of receptor signaling in γδ T-cell differentiation and activation, and discuss its implications for the manipulation of γδ T-cells in immunotherapy.
γδ cells endow the T-cell compartment with a rapid, innate-like reaction to insults, which places them in the afferent phase of the immune response. Namely, γδ T-cells are responsible for “lymphoid stress surveillance,” i.e., sensing and responding immediately to infections or non-microbial stress without the need of clonal expansion or de novo differentiation, in synchrony with prototypic innate immune responses (1). Critically, this implicates γδ T-cells in inflammation (2), autoimmunity (3), infectious diseases (4, 5), and tumor surveillance (6–8).
Many of the studies elucidating the physiological roles of γδ T-cells have been performed in murine models, where a major breakthrough has been the identification of pro-inflammatory subsets naturally producing either IFNγ or IL-17 (9–11). Moreover, these studies have been greatly facilitated by the identification of cell surface markers that segregate the two functional γδ T-cell subsets: CD27, CD122, and NK1.1 mark IFNγ-producing γδ cells, whereas their IL-17-expressing counterparts display a CD27− CCR6+ phenotype (9–11). Moreover, the two subsets show distinct Vγ chain usage in their TCR repertoires, with a bias toward Vγ1 among IFNγ-producing γδ cells, and an enrichment in Vγ4 and Vγ6 in IL-17-producing γδ cells (12).
In humans, γδ T-cells are primarily identified by their Vδ chain usage, with Vδ1+ cells predominating in the thymus and in peripheral tissues, while Vδ2+ cells (mostly co-expressing a Vγ9 chain) constitute the majority of blood-circulating γδ T-cells. Both human γδ T-cell subsets are highly prone to secrete IFNγ, but IL-17 can be induced in highly inflammatory conditions triggered by infections (13) or tumors (14, 15).
In both murine and human γδ T-cells, functional responses are initiated upon recognition of antigens that are likely induced by stress signals and sensed by either T-cell or natural killer receptors. Some γδ T-cell populations are also particularly responsive to cytokines or innate toll-like receptor (TLR) agonists (16, 17). Following proliferation and effector responses, the return to homeostasis is controlled by inhibitory receptors. Here, we discuss the various layers of contributions of T (TCR and costimulatory/inhibitory receptors), NK, and cytokine receptors to the activation and differentiation of effector γδ T-cell populations in mice and humans.
The γδTCR complex is composed by the γδTCR itself and various CD3 chains following the stoichiometry: TCRγδCD3δ2γδζ2 in humans and TCRγδCD3δ2γ2ζ2 in mice (18). The assembly of a γδTCR complex in thymic progenitors has immediate consequences for γδ T-cell development. The “strong” signals stemming from the γδTCR (when compared to the “weaker” pre-TCR signaling) drive γδ/αβ common precursors into the γδ lineage (19, 20). These “stronger” γδTCR signals associate with increased phosphorylation of ERK1/2, abundant calcium release and induction of early growth response (Egr) transcription factors (21, 22).
The TCR complex does not present intrinsic kinase activity but the intracellular signaling is initiated after phosphorylation of immunoreceptor tyrosine-based activation motifs (ITAMs) in the CD3 cytoplasmic domains by the Src-family kinases (SFKs) Lck and Fyn (23). The recruitment of these SFKs to the TCR complex in γδ T-cells remains obscure since these cells do not express the CD4 or CD8 co-receptors, which have been shown, in αβ T-cells, to be responsible for recruiting SFKs upon αβTCR ligation (23). Nonetheless, the importance of SFKs in γδ T-cells is underscored by the substantial phosphorylation of ERK upon inhibition of Csk, a potent inhibitor of SFKs (24).
SFK-mediated phosphorylation of the ITAMs on CD3 chains allows the recruitment, phospholylation, and activation of Zap70 that facilitates phosphorylation of the scaffolding proteins SLP-76 and LAT. This lead to the formation of a supramolecular signalosome that recruits the phospholipase PLCγ1, resulting in propagation of downstream signaling events (22). Here again, γδ T-cell signaling is different from αβ T-cells, since mutations on the binding site of PLCγ1 on LAT resulted in a severe block in murine αβ thymocyte development while γδ T-cell numbers were only modestly reduced in the thymus, intestine, and liver, and remained normal in the skin. Unexpectedly, a population of γδ T-cells in the secondary lymphoid organs in these mice underwent uncontrolled expansion and caused autoimmune pathology, suggesting distinct functions for LAT/PLCγ1-mediated signaling in subpopulations of γδ T-cells (21, 25).
In humans, the major γδ T-cell subset in the peripheral blood, Vγ9Vδ2 T-cells, are uniquely and specifically reactive to self- and foreign non-peptidic phosphorylated intermediates of isoprenoid synthesis – “phosphoantigens” or “phosphoagonists” (P-Ags) (26–28). These P-Ags were shown to trigger bona fide Vγ9Vδ2 TCR signaling in various studies. Cipriani and colleagues showed that the activation of Vγ9Vδ2 T-cells with the P-Ag isopentenyl pyrophosphate (IPP), induced rapid and persistent PKC-dependent phosphorylation of ERK1/2, p38 MAPK, and JNK, resulting in NF-κB and AP-1 activation as well as the release of MIP-1α, MIP-1β, IFN-γ, and TNF-α (29). Moreover, P-Ag stimulation and CD3-crosslinking produced identical phosphorylation of the signaling proteins Zap70, PI3K, LAT, ERK1/2, and p38 MAPK (30, 31); and induced highly sustained calcium signaling in Vγ9Vδ2 T-cells (32). Importantly, activation by P-Ags is the basis of current cancer immunotherapy strategies involving Vγ9Vδ2 T-cells (33).
Recent work has produced some puzzling results on the role of the γδTCR in the development of effector subsets of murine γδ T-cells (34–36), namely, CD27+ CD122+ γδ T-cells producing IFN-γ or CD27− CCR6+ γδ T-cells making IL-17 (9, 10). First, Chien and co-workers showed that T10/T22-specific γδ T-cells required thymic expression of their TCR ligand to differentiate into IFN-γ producers, in contrast with “ligand naïve” IL-17 producers (9). Consistent with this, TCR-dependent thymic selection was also shown to set the functional potential of dendritic epidermal T-cells (DETC) progenitors away from IL-17 production (37). Furthermore, peripheral IL-17-producing CD27− CCR6+ γδ T-cells were shown to expand and produce IL-17 independently of TCR activation (38). However, a subsequent study by Chien and collaborators demonstrated that a subset of phycoerythrin (PE)-specific γδ T-cells produced IL-17 specifically upon TCR ligation (39). Moreover, a recent study by Hayday and colleagues suggested that an impairment in Zap70 signaling (in SKG mice) mostly affected the development of IL-17+ rather than IFN-γ+ γδ T-cells (40). The authors further proposed that “innate-like” γδ T-cell populations, including IL-17 producers and some subsets of IFN-γ producers, receive strong TCR signals during thymic development to become hyporesponsive to TCR stimulation in the periphery (40). Future research should aim to resolve the apparent contradictions of the available data, namely, by clarifying the requirement on TCR ligand engagement, as well as the developmental effects of manipulating distinct γδTCR signaling pathways and their downstream (transcriptional and post-transcriptional) mechanisms on γδ T-cell subsets.
A series of T-cell costimulatory receptors are known to induce qualitative and quantitative changes that lower activation thresholds, prevent “anergy” and enhance T-cell functions. Typical costimulatory receptors are type I transmembrane proteins that can be divided into two groups, based on their structural characteristics: immunoglobulin (Ig) or tumor necrosis factor receptor (TNFR) superfamilies. Ig superfamily members have a variable Ig-like extracellular domain and a short cytoplasmic tail, whereas TNFR family members present extracellular domains rich in six cysteine repeats (which form disulfide bridges) and a more complex cytoplasmic tail [reviewed in Ref. (41)]. These two main types of costimulatory receptors display different modes of intracellular signaling: whereas the CD28 family members associate directly with protein kinases (like PI3K or ITK), TNFR superfamily co-receptors require the adaptor proteins TRAF (TNFR-associated factor), namely TRAF2 and TRAF5, to link to downstream signaling mediators (Table 1). Here, based on their specific roles in γδ T-cells, we shall discuss CD28 (of the Ig superfamily) and the TNFR superfamily members, CD27, CD30, and CD137 (4-1BB).
The best studied costimulatory receptor, CD28, has historically yielded paradoxical results on γδ T-cells (46). We have recently readdressed this issue for both human and mouse γδ T-cells. We described that CD28 is constitutively expressed on lymphoid γδ T-cells and promotes survival and proliferation via IL-2 production. CD28 receptor agonists enhanced γδ T-cell expansion, which was conversely inhibited by blocking antibodies against its B7 ligands (42). Importantly, CD28-deficient mice displayed lower (relative to controls) numbers of total or activated γδ T-cells upon Plasmodium berghei infection, and failed to expand both their IFN-γ+ and IL-17+ subsets (42). In contrast, Hayes and colleagues reported that both functional γδ T-cell subsets differentiated and expanded normally in a Listeria model (80). It would be interesting to determine how variable is the dependence on CD28 costimulation for γδ T-cell responses to distinct infectious agents.
In naïve mice, while CD28 is not required for the development of either IFN-γ+ or IL-17+ γδ T-cell subsets (80), the TNFR superfamily member CD27 is selectively implicated in the generation of IFN-γ+ γδ T-cells (10). In fact, we showed that CD27 expression segregates IFN-γ+ (CD27+) and IL-17+ (CD27−) γδ T-cells. Most interestingly, these phenotypes are established in the thymus, and since embryonic stages. Based on the results from our (10) and Chien’s (9) teams, the development of IFN-γ-producing γδ T-cells seemingly requires strong TCR signaling and CD27 costimulation in the thymus.
Beyond its role in thymic differentiation, CD27 is critical for the expansion of peripheral IFN-γ-producing γδ T-cells upon infection with herpes viruses or malaria parasites in mice (81). We showed that, in the context of TCR stimulation and upon ligation to CD70, CD27 signaling activates the non-canonical NF-κB pathway and enhances the expression of anti-apoptotic and cell cycle-related genes, thus promoting murine γδ T-cell survival and proliferation (81).
We have also addressed the impact of CD27 costimulation on the activation of human γδ T-cells. Administration of soluble recombinant CD70 enhanced, whereas anti-CD27 (or anti-CD70) antibodies reduced, Vγ9Vδ2 T-cell expansion in vitro (82). Moreover, CD27 signals induced calcium fluxes and upregulated the expression of Cyclin D2 and the anti-apoptotic gene Bcl2a1. Given the typical IFN-γ secretion and cytotoxicity of activated Vγ9Vδ2 T-cells (30), our work suggests that the modulation of CD70–CD27 signals may be beneficial in the context of γδ T-cell-based cancer immunotherapy.
Upon activation, human γδ T-cells can also express another TNFR superfamily member, CD30 (83). CD30 signaling, which potentiated calcium fluxes induced by TCR activation, also enhanced pro-inflammatory cytokine production (50). Recently, Yoshikai and colleagues compared γδ T-cell homeostasis and response to Listeria monocytogenes in CD30-sufficient versus deficient mice. They demonstrated a selective depletion of IL-17-producing Vγ6+ T-cells in mucosal tissues in the steady-state and upon infection (84). This associated with reduced bacterial clearance, which could be rescued, alongside the IL-17+ Vγ6+ T-cell pool, by agonistic anti-CD30 antibody administration. In contrast, Lee et al. reported that agonistic anti-CD137 (4-1BB) antibodies promoted the expansion of IFN-γ+ Vγ1+ T-cells, which protected (in an IFN-γ-dependent manner) also from Listeria infection (52). This study also showed that 4-1BB was expressed and functional on activated human γδ T-cells, and its ligation upon cell transfer protected NOD/SCID mice against Listeria infection.
Interestingly, activated Vγ9Vδ2 T-cells also express high levels of 4-1BBL (CD137L) (85), which besides acting as a ligand for 4-1BB on T and NK-cells, may also participate in Vγ9Vδ2 T-cell activation due to its known reverse signaling ability (86). This may, in fact, also apply to CD70 (CD27-ligand), which is highly induced upon phosphoantigen-mediated stimulation of Vγ9Vδ2 T-cells (82, 87). These possibilities deserve further investigation.
Interleukins are key determinants of T-cell survival, proliferation, and differentiation. IL-7, IL-15, and IL-2 are essential for lymphocyte development and homeostasis; upon inflammation, other cytokines, namely, IL-1β, IL-12, IL-18, IL-21, and IL-23, take a central role in determining T-cell functions. Here, we review the main contributions of homeostatic and inflammatory cytokines specifically to γδ T-cell physiology.
IL-7 and IL-15 are seemingly the key determinants of murine γδ T-cell development (88–90) and homeostasis (91). A recent study that depleted IL-7 specifically from (Foxn1+) thymic epithelial cells showed that γδ T-cells were significantly reduced in the adult thymus and in the gut, whereas they were completely absent in the fetal thymus and epidermis (89). In the dermis, it was also IL-7, but not IL-15, that supported the development and survival of the resident γδ T-cell population (92). Conversely, in the gut, IL-15 seems to play the primordial role in sustaining the local intraepithelial γδ T-cell compartment (93).
Unexpectedly, IL-7 was recently reported to promote the selective expansion of murine IL-17-producing γδ T-cells (59). STAT3-dependent IL-7 signals allowed CD27− γδ T-cells to resist activation-induced cell death (AICD) and undergo proliferative responses to TCR agonists. Such an IL-7/IL-17 axis was also reported to be required for the γδ T-cell response to viral hepatitis infection in vivo (94). Moreover, IL-7 also seems to support the expansion of human IL-17-producing γδ T-cells (59).
We recently assessed the functional differentiation of human γδ thymocytes, which are >80% of the Vδ1 subtype. We observed that IL-15 and IL-2, but not IL-7, induced the cytotoxic type 1 (IFN-γ-producing) program in functionally immature γδ thymocytes (55). This was consistent with previous data on peripheral γδ T-cells isolated from cancer patients (95). However, additional reports on peripheral Vγ9Vδ2 T-cell cultures showed that IL-15 or IL-2 stimulation, despite efficient ERK and AKT activation, were not sufficient to induce effector responses; these required phosphoantigen-dependent TCR activation and downstream calcium mobilization (56, 96). Unexpectedly, in our cultures of γδ (mostly Vδ1) thymocytes, TCR stimulation was not required for neither ERK activation nor T-bet and eomesodermin induction and the acquisition of effector functions (55).
IL-2 and IL-15 play key roles in the peripheral expansion of Vγ9Vδ2 T-cells in response to microbial phosphoantigens or synthetic drugs like bisphosphonates (56, 97). This notwithstanding, it is important to note, toward the therapeutic application of Vγ9Vδ2 T-cells, that optimal effector responses seemingly require the combination of these cytokines with TCR agonists. Thus, recent work from Chen and colleagues demonstrated that the differentiation of cytotoxic type 1 Vγ9Vδ2 T-cells capable of controlling Mycobacterium tuberculosis infection in macaques required a phosphoantigen/IL-2 combination (98).
Effector γδ T-cell differentiation is also greatly impacted by inflammatory cytokines, particularly IL-12 and IL-18 that typically promote IFN-γ production; and IL-1β and IL-23 that mostly drive IL-17 production.
High expression of IL-12Rβ expression on activated murine γδ T-cells guarantees a dominance of type 1 (IFN-γ+) over type 2 (IL-4+) effector fates (99). Type 1 differentiation is also predominant in human γδ T-cells, and can be further enhanced by IL-18 (100, 101) or IL-21 (102). The induction of a type 17 program in human γδ T-cells requires persistent stimulation with IL-23 for neonatal Vγ9Vδ2 T-cells (15); and IL-23 and IL-1β in the presence of TGF-β for adult Vγ9Vδ2 T-cells (13, 103). In mice, IL-1β and IL-23 are also the main drivers of abundant IL-17 production by peripheral γδ T-cells (3, 5, 81, 104–106), although recent data surprisingly suggest that IL-18 can replace IL-1β in combining with IL-23 to induce IL-17 expression (107). In contrast, IL-1β upstream of IL-1R seems essential for GM-CSF production by γδ T-cells (108).
Finally, IL-21 was recently suggested to endow human Vγ9Vδ2 T-cells with B-cell helper activity associated with a T follicular helper cell-like phenotype (60, 109), which may impact on the generation of high affinity antibodies against microbial infections.
An important key characteristic that allows the recognition of transformed cells by γδ T-cells is the expression of a wide set of germline-encoded receptors that were initially described in NK-cells and hence are collectively known as NK receptors (NKRs), including natural cytotoxicity receptors (NCRs).
The C-type lectin-like NK receptor group 2 member D (NKG2D) is the best studied NKR in γδ T-cells. NKG2D binds extracellularly to multiple ligands of the MIC(A–B) and ULBP (1–6) families in humans; and to H60, MULT1, and various RAE1 molecules in mice (110). NKG2D ligands are induced upon cellular stress, for example, downstream of the DNA-damage response pathway in tumor cells (111, 112). The biological significance of this recognition system is underlined by the increased susceptibility of NKG2D-deficient mice to tumor development (113).
Intracellularly, NKG2D binds to DNAX-activating protein of 10 kDa (DAP10), which carries an YXNM motif that after tyrosine phosphorylation recruits PI3K or a Grb2–Vav1–SOS1 signaling complex (Table 1). This motif is similar to that in CD28, and thus, NKG2D/DAP10 may provide T-cells with costimulatory signals that synergize with the ITAM-based TCR/CD3 complex (61). However, unlike αβ T-cells but similarly to NK-cells, γδ T-cells can express both DAP10 and DAP12 (62). The latter contains an ITAM motif, which after tyrosine phosphorylation recruits and activates Syk and ZAP70. Interestingly, only murine but not human NKG2D is able to associate with DAP12 (in addition to DAP10).
The controversy on a primary stimulatory versus costimulatory role of NKG2D in γδ T-cells has been discussed elsewhere (46, 114). Briefly, the costimulatory function of NKG2D in human Vγ9Vδ2 T-cells was supported by additive effects on TCR-mediated activation: an upregulation of cytokine production upon MICA-NKG2D interactions (115); and an increase in intracellular calcium mobilization and cytotoxic activity (32). However, other lines of evidence have suggested that NKG2D signals can activate γδ T-cells in the absence of TCR engagement: NKG2D ligation can upregulate CD69 expression in Vγ9Vδ2 T-cells to similar extent as TCR stimulation (116); NKG2D but not TCR blockade can inhibit Vγ9Vδ2 T-cell cytotoxicity against various hematological tumors (117); and murine DETC can target tumors upon recognition of NKG2D ligands (6, 118).
Another NKR implicated in tumor cell recognition by Vγ9Vδ2 T-cells is DNAX accessory molecule-1 (DNAM-1). DNAM-1 is an Ig-like family glycoprotein composed of a cytoplasmic domain containing three putative sites of phosphorylation by intracellular kinases. The phosphorylation of the Ser329 by protein kinase C (PKC) was shown to be critical for the association between DNAM-1 and LFA-1, which recruits the Fyn Src kinase to phosphorylate the Tyr322 of DNAM-1, thus initiating downstream signaling leading to SLP-76 and Vav1 phosphorylation (Table 1) (119). Antibody-mediated DNAM-1 blockade impaired Vγ9Vδ2 T-cell cytotoxicity and IFN-γ production against hepatocellular carcinoma lines expressing Nectin-like-5 (71).
Recently, we characterized a Vδ1+ T-cell population capable of targeting hematological tumors resistant to fully activated Vγ9Vδ2 T-cells (120). Unexpectedly, the enhanced killer function resulted from induced NCR expression, namely NKp30 and NKp44, which had been previously regarded as NK-specific markers. Although neither Vδ1+ nor Vδ2+ cells express NCRs constitutively, these can be upregulated selectively in Vδ1+ cells by PI3K/AKT-dependent signals provided by γc cytokines (IL-2 or IL-15) and TCR stimulation. Once expressed on the cell surface, NKp30 and NKp44 can signal via CD3ζ and DAP12, respectively (64). We further showed that NKp30 and NKp44 are both functional in NCR+ Vδ1+ T-cells and synergize with NKG2D to target lymphocytic leukemia cells (120).
In sum, NKRs seem critical for tumor recognition and deployment of the cytotoxic program that is endowed by TCR/γc cytokine-dependent differentiation, thus defining distinct mechanisms to be integrated in γδ T-cell-mediated cancer immunotherapy.
Beyond efficient activation and deployment of effector functions, it is necessary to negatively regulate the T-cell response in order to return to the homeostatic baseline. Inhibitory receptors like PD-1 or CTLA-4 are known to be critical for this contracting phase of the T-cell response and have become major clinical targets in cancer immunotherapy. Although γδ T-cells rarely express CTLA-4, they can upregulate PD-1 upon activation, while they constitutively express BTLA, and thus these two receptors may be the key to control γδ T-cell responses.
Programed death-1 (PD-1) is absent or low expressed on circulating Vγ9Vδ2 T-cells but is rapidly induced upon activation (121). The cytoplasmic tail of PD-1 contains conserved immunoreceptor tyrosine-based inhibitory motif (ITIM) and switch motif (ITSM), both of which are phosphorylated to recruit negative regulators that block Lck activity downstream of the TCR complex (122). Moreover, PD-1 ligation can augment the activity of the protein phosphatase and tensin homolog (PTEN), a cellular phosphatase that inhibits PI3K/AKT signaling and thus leads to impaired survival, proliferation, and IL-2 release (123). The expression of the ligand PD-L1 on tumor cells inhibited Vγ9Vδ2 T-cell cytotoxicity and IFN-γ production (121). However, zoledronate-induced accumulation of P-Ags in tumor cells and consequent Vγ9Vδ2 TCR activation seemed to overcome the inhibitory effect of PD-1/PD-L1 interactions. More research is required to understand the full extent to what PD-1 may control γδ T-cell functions and homeostasis.
B- and T-lymphocyte attenuator (BTLA) is another inhibitory receptor, member of the CD28 family and structurally related to PD-1 and CTLA-4. Binding to its ligand, herpesvirus entry mediator (HVEM), induces phosphorylation of the ITIM domain and association with SH2 domain-containing protein tyrosine phosphatase 1 (SHP-1) and SHP-2, which leads to attenuation of cellular activation and growth (124). Recent data showed that BTLA engagement with HVEM reduced P-Ag/TCR-mediated signaling and inhibited Vγ9Vδ2 T-cell proliferation, including in response to lymphoma cells (77). Conversely, BTLA-HVEM blockade using monoclonal antibodies enhanced Vγ9Vδ2 TCR signaling and may thus have therapeutic potential for the positive manipulation of γδ T-cells.
A detailed study on BTLA function in murine γδ T-cells has revealed a selective involvement in the homeostasis of the IL-17-producing CD27− γδ T-cell subset (78). Although these cells constitutively express low levels of BTLA, it is upregulated by IL-7 stimulation and thereby limits γδ T-cell numbers. Consequently, BTLA-deficient mice accumulated IL-17+ CD27− γδ T-cells and were more susceptible (than wild-type controls) to dermatitis, which could be reversed by agonist BTLA antibodies. Thus, BTLA may be an important target for controlling pathogenic γδ T-cells in inflammatory and autoimmune diseases.
A multitude of surface receptors has been shown to participate in γδ T-cell differentiation and activation. However, some crucial aspects remain to be elucidated, such as the identity of most γδTCR ligands. Most importantly, we must improve the transfer of past and current basic research into future protocols for γδ T-cell-based immunotherapy. In this context, some key questions are: how to balance γδTCR activation with “exhaustion” due to chronic stimulation? What can be achieved by manipulating the NK-like activation mode of γδ T-cells? Which costimulatory receptors should be modulated, and at what stages, to boost the desired γδ T-cell responses? Which combinations of cytokines enable the best effector γδ T-cells for each therapeutic application? Which receptors are most useful to tune down or switch off pathogenic effector γδ T-cells? The answers to these questions must be obtained in appropriate in vivo pre-clinical models and hopefully next in the clinic.
For now, we would like to propose that the five types of receptor signals reviewed here define five distinct layers of regulation of γδ T-cell differentiation, activation, and function. The γδTCR is critical for the initial stages of differentiation and for proliferative responses; both processes further require cytokine signals that promote cell survival, proliferation, and terminal effector function. Costimulatory and inhibitory receptors control the extent of γδ T-cell expansion, with interesting biases toward specific effector subsets. Finally, NK receptors play a decisive role in tumor cell targeting by γδ T-cells. Thus, we believe that the recognition of “stressed self” can be mediated by the γδTCR but also chiefly by NK receptors like NKG2D. As such, the characterization of both type of ligands on tumors may be critical to design protocols, select and monitor patients, and increase the chances of efficacious γδ T-cell-based cancer immunotherapies.
Bruno Silva-Santos is a co-founder and share holder of LymphAct S.A. The other co-authors declare that the research was conducted in the absence of any commercial or financial relationships that could be construed as a potential conflict of interest.
We thank Daniel Correia for helpful discussions on these topics. Our work is supported by Fundação para a Ciência e Tecnologia (SFRH/BD/84123/2012 to Sérgio T. Ribeiro; SFRH/BPD/78135/2011 and EXPL/IMI-IMU/0170/2013 to Julie C. Ribot) and European Research Council (StG_260352 to Bruno Silva-Santos).
1. Hayday AC. γδ T cells and the lymphoid stress-surveillance response. Immunity (2009) 31:184–96. doi:10.1016/j.immuni.2009.08.006
Pubmed Abstract | Pubmed Full Text | CrossRef Full Text | Google Scholar
2. Martin B, Hirota K, Cua DJ, Stockinger B, Veldhoen M. Interleukin-17-producing γδ T cells selectively expand in response to pathogen products and environmental signals. Immunity (2014) 31:321–30. doi:10.1016/j.immuni.2009.06.020
Pubmed Abstract | Pubmed Full Text | CrossRef Full Text | Google Scholar
3. Sutton CE, Lalor SJ, Sweeney CM, Brereton CF, Lavelle EC, Mills KHG. Interleukin-1 and IL-23 induce innate IL-17 production from γδ T cells, amplifying Th17 responses and autoimmunity. Immunity (2009) 31:331–41. doi:10.1016/j.immuni.2009.08.001
Pubmed Abstract | Pubmed Full Text | CrossRef Full Text | Google Scholar
4. Ramsburg E, Tigelaar R, Craft J, Hayday A. Age-dependent requirement for gammadelta T cells in the primary but not secondary protective immune response against an intestinal parasite. J Exp Med (2003) 198:1403–14. doi:10.1084/jem.20030050
Pubmed Abstract | Pubmed Full Text | CrossRef Full Text | Google Scholar
5. Lockhart E, Green AM, Flynn JL. IL-17 production is dominated by gammadelta T cells rather than CD4 T cells during Mycobacterium tuberculosis infection. J Immunol (2006) 177:4662–9. doi:10.4049/jimmunol.177.7.4662
Pubmed Abstract | Pubmed Full Text | CrossRef Full Text | Google Scholar
6. Girardi M, Oppenheim DE, Steele CR, Lewis JM, Glusac E, Filler R, et al. Regulation of cutaneous malignancy by gammadelta T cells. Science (2001) 294:605–9. doi:10.1126/science.1063916
Pubmed Abstract | Pubmed Full Text | CrossRef Full Text | Google Scholar
7. Gao Y, Yang W, Pan M, Scully E, Girardi M, Augenlicht LH, et al. Gamma delta T cells provide an early source of interferon gamma in tumor immunity. J Exp Med (2003) 198:433–42. doi:10.1084/jem.20030584
Pubmed Abstract | Pubmed Full Text | CrossRef Full Text | Google Scholar
8. Lança T, Costa MF, Gonçalves-Sousa N, Rei M, Grosso AR, Penido C, et al. Protective role of the inflammatory CCR2/CCL2 chemokine pathway through recruitment of type 1 cytotoxic γδ T lymphocytes to tumor beds. J Immunol (2013) 190:6673–80. doi:10.4049/jimmunol.1300434
Pubmed Abstract | Pubmed Full Text | CrossRef Full Text | Google Scholar
9. Jensen KDC, Su X, Shin S, Li L, Youssef S, Yamasaki S, et al. Thymic selection determines gammadelta T cell effector fate: antigen-naive cells make interleukin-17 and antigen-experienced cells make interferon gamma. Immunity (2008) 29:90–100. doi:10.1016/j.immuni.2008.04.022
Pubmed Abstract | Pubmed Full Text | CrossRef Full Text | Google Scholar
10. Ribot JC, deBarros A, Pang DJ, Neves JF, Peperzak V, Roberts SJ, et al. CD27 is a thymic determinant of the balance between interferon-gamma- and interleukin 17-producing gammadelta T cell subsets. Nat Immunol (2009) 10:427–36. doi:10.1038/ni.1717
Pubmed Abstract | Pubmed Full Text | CrossRef Full Text | Google Scholar
11. Haas JD, González FHM, Schmitz S, Chennupati V, Föhse L, Kremmer E, et al. CCR6 and NK1.1 distinguish between IL-17A and IFN-gamma-producing gammadelta effector T cells. Eur J Immunol (2009) 39:3488–97. doi:10.1002/eji.200939922
Pubmed Abstract | Pubmed Full Text | CrossRef Full Text | Google Scholar
12. O’Brien RL, Born WK. gammadelta T cell subsets: a link between TCR and function? Semin Immunol (2010) 22:193–8. doi:10.1016/j.smim.2010.03.006
Pubmed Abstract | Pubmed Full Text | CrossRef Full Text | Google Scholar
13. Caccamo N, La Mendola C, Orlando V, Meraviglia S, Todaro M, Stassi G, et al. Differentiation, phenotype, and function of interleukin-17-producing human Vgamma9Vdelta2 T cells. Blood (2011) 118:129–38. doi:10.1182/blood-2011-01-331298
Pubmed Abstract | Pubmed Full Text | CrossRef Full Text | Google Scholar
14. Wu P, Wu D, Ni C, Ye J, Chen W, Hu G, et al. γδT17 cells promote the accumulation and expansion of myeloid-derived suppressor cells in human colorectal cancer. Immunity (2014) 40:785–800. doi:10.1016/j.immuni.2014.03.013
Pubmed Abstract | Pubmed Full Text | CrossRef Full Text | Google Scholar
15. Moens E, Brouwer M, Dimova T, Goldman M, Willems F, Vermijlen D. IL-23R and TCR signaling drives the generation of neonatal Vgamma9Vdelta2 T cells expressing high levels of cytotoxic mediators and producing IFN-gamma and IL-17. J Leukoc Biol (2011) 89:743–52. doi:10.1189/jlb.0910501
Pubmed Abstract | Pubmed Full Text | CrossRef Full Text | Google Scholar
16. Bonneville M, O’Brien RL, Born WK. Gammadelta T cell effector functions: a blend of innate programming and acquired plasticity. Nat Rev Immunol (2010) 10:467–78. doi:10.1038/nri2781
Pubmed Abstract | Pubmed Full Text | CrossRef Full Text | Google Scholar
17. Chien Y, Meyer C, Bonneville M. γδ T cells: first line of defense and beyond. Annu Rev Immunol (2014) 32:121–55. doi:10.1146/annurev-immunol-032713-120216
Pubmed Abstract | Pubmed Full Text | CrossRef Full Text | Google Scholar
18. Siegers GM, Swamy M, Fernández-Malavé E, Minguet S, Rathmann S, Guardo AC, et al. Different composition of the human and the mouse gammadelta T cell receptor explains different phenotypes of CD3gamma and CD3delta immunodeficiencies. J Exp Med (2007) 204:2537–44. doi:10.1084/jem.20070782102207c
Pubmed Abstract | Pubmed Full Text | CrossRef Full Text | Google Scholar
19. Hayes SM, Li L, Love PE. TCR signal strength influences alphabeta/gammadelta lineage fate. Immunity (2005) 22:583–93. doi:10.1016/j.immuni.2005.03.014
Pubmed Abstract | Pubmed Full Text | CrossRef Full Text | Google Scholar
20. Haks MC, Lefebvre JM, Lauritsen JPH, Carleton M, Rhodes M, Miyazaki T, et al. Attenuation of gammadeltaTCR signaling efficiently diverts thymocytes to the alphabeta lineage. Immunity (2005) 22:595–606. doi:10.1016/j.immuni.2005.04.003
Pubmed Abstract | Pubmed Full Text | CrossRef Full Text | Google Scholar
21. Hayes SM, Shores EW, Love PE. An architectural perspective on signaling by the pre-, alphabeta and gammadelta T cell receptors. Immunol Rev (2003) 191:28–37. doi:10.1034/j.1600-065X.2003.00011.x
Pubmed Abstract | Pubmed Full Text | CrossRef Full Text | Google Scholar
22. Smith-Garvin JE, Koretzky GA, Jordan MS. T cell activation. Annu Rev Immunol (2009) 27:591–619. doi:10.1146/annurev.immunol.021908.132706
Pubmed Abstract | Pubmed Full Text | CrossRef Full Text | Google Scholar
23. Kuhns MS, Badgandi HB. Piecing together the family portrait of TCR-CD3 complexes. Immunol Rev (2012) 250:120–43. doi:10.1111/imr.12000
Pubmed Abstract | Pubmed Full Text | CrossRef Full Text | Google Scholar
24. Tan YX, Manz BN, Freedman TS, Zhang C, Shokat KM, Weiss A. Inhibition of the kinase Csk in thymocytes reveals a requirement for actin remodeling in the initiation of full TCR signaling. Nat Immunol (2014) 15:186–94. doi:10.1038/ni.2772
Pubmed Abstract | Pubmed Full Text | CrossRef Full Text | Google Scholar
25. Sullivan SA, Zhu M, Bao S, Lewis CA, Ou-Yang C, Zhang W. The role of LAT-PLCγ1 interaction in γδ T cell development and homeostasis. J Immunol (2014) 192:2865–74. doi:10.4049/jimmunol.1302493
Pubmed Abstract | Pubmed Full Text | CrossRef Full Text | Google Scholar
26. Constant P, Davodeau F, Peyrat M, Poquet Y, Puzo G, Bonneville M, et al. Stimulation of human gamma delta T cells by nonpeptidic mycobacterial ligands. Science (1994) 264:267–70. doi:10.1126/science.8146660
Pubmed Abstract | Pubmed Full Text | CrossRef Full Text | Google Scholar
27. Tanaka Y, Morita CT, Nieves E, Brenner MB, Bloom BR. Natural and synthetic non-peptide antigens recognized by human gamma delta T cells. Nature (1995) 375:155–8. doi:10.1038/375155a0
Pubmed Abstract | Pubmed Full Text | CrossRef Full Text | Google Scholar
28. Gober H-J, Kistowska M, Angman L, Jeno P, Mori L, De Libero G. Human T cell receptor γδ cells recognize endogenous mevalonate metabolites in tumor cells. J Exp Med (2003) 197:163–8. doi:10.1084/jem.20021500
Pubmed Abstract | Pubmed Full Text | CrossRef Full Text | Google Scholar
29. Cipriani B, Knowles H, Chen L, Battistini L, Brosnan CF. Involvement of classical and novel protein kinase C isoforms in the response of human Vγ9Vδ2 T cells to phosphate antigens. J Immunol (2002) 169:5761–70. doi:10.4049/jimmunol.169.10.5761
Pubmed Abstract | Pubmed Full Text | CrossRef Full Text | Google Scholar
30. Correia DV, D’Orey F, Cardoso BA, Lança T, Grosso AR, DeBarros A, et al. Highly active microbial phosphoantigen induces rapid yet sustained MEK/Erk- and PI-3K/Akt-mediated signal transduction in anti-tumor human γδ T-cells. PLoS One (2009) 4:e5657. doi:10.1371/journal.pone.0005657
Pubmed Abstract | Pubmed Full Text | CrossRef Full Text | Google Scholar
31. Nedellec S, Bonneville M, Scotet E. Human Vgamma9Vdelta2 T cells: from signals to functions. Semin Immunol (2010) 22:199–206. doi:10.1016/j.smim.2010.04.004
Pubmed Abstract | Pubmed Full Text | CrossRef Full Text | Google Scholar
32. Nedellec S, Sabourin C, Bonneville M, Scotet E. NKG2D costimulates human Vγ9Vδ2 T cell antitumor cytotoxicity through protein kinase Cθ-dependent modulation of early TCR-induced calcium and transduction signals. J Immunol (2010) 185:55–63. doi:10.4049/jimmunol.1000373
Pubmed Abstract | Pubmed Full Text | CrossRef Full Text | Google Scholar
33. Gomes A, Martins D, Silva-Santos B. Targeting γδ T lymphocytes for cancer immunotherapy from novel mechanistic insight to clinical application. Cancer Res (2010) 70:10024–7. doi:10.1158/0008-5472.CAN-10-3236
Pubmed Abstract | Pubmed Full Text | CrossRef Full Text | Google Scholar
34. Turchinovich G, Pennington D. T cell receptor signalling in γδ cell development: strength isn’t everything. Trends Immunol (2011) 32:567–73. doi:10.1016/j.it.2011.09.005
Pubmed Abstract | Pubmed Full Text | CrossRef Full Text | Google Scholar
35. Chien Y, Zeng X, Prinz I. The natural and the inducible: interleukin (IL)-17-producing γδ T cells. Trends Immunol (2013) 34:151–4. doi:10.1016/j.it.2012.11.004
Pubmed Abstract | Pubmed Full Text | CrossRef Full Text | Google Scholar
36. Fahl SP, Coffey F, Wiest DL. Origins of γδ T cell effector subsets: a riddle wrapped in an enigma. J Immunol (2014) 193:4289–94. doi:10.4049/jimmunol.1401813
Pubmed Abstract | Pubmed Full Text | CrossRef Full Text | Google Scholar
37. Turchinovich G, Hayday AC. Skint-1 identifies a common molecular mechanism for the development of interferon-γ-secreting versus interleukin-17-secreting γδ T cells. Immunity (2011) 35:59–68. doi:10.1016/j.immuni.2011.04.018
Pubmed Abstract | Pubmed Full Text | CrossRef Full Text | Google Scholar
38. Kapsenberg ML. Gammadelta T cell receptors without a job. Immunity (2009) 31:181–3. doi:10.1016/j.immuni.2009.08.004
Pubmed Abstract | Pubmed Full Text | CrossRef Full Text | Google Scholar
39. Zeng X, Wei Y-L, Huang J, Newell EW, Yu H, Kidd BA, et al. γδ T cells recognize a microbial encoded B cell antigen to initiate a rapid antigen-specific interleukin-17 response. Immunity (2012) 37:524–34. doi:10.1016/j.immuni.2012.06.011
Pubmed Abstract | Pubmed Full Text | CrossRef Full Text | Google Scholar
40. Wencker M, Turchinovich G, Di Marco Barros R, Deban L, Jandke A, Cope A, et al. Innate-like T cells straddle innate and adaptive immunity by altering antigen-receptor responsiveness. Nat Immunol (2014) 15:80–7. doi:10.1038/ni.2773
Pubmed Abstract | Pubmed Full Text | CrossRef Full Text | Google Scholar
41. Duttagupta PA, Boesteanu AC, Katsikis PD. Costimulation signals for memory CD8+ T cells during viral infections. Crit Rev Immunol (2009) 29:469–86. doi:10.1615/CritRevImmunol.v29.i6.20
Pubmed Abstract | Pubmed Full Text | CrossRef Full Text | Google Scholar
42. Ribot JC, DeBarros A, Mancio-Silva L, Pamplona A, Silva-Santos B. B7-CD28 costimulatory signals control the survival and proliferation of murine and human γδ T cells via interleukin-2 production. J Immunol (2012) 189:1202–8. doi:10.4049/jimmunol.1200268
Pubmed Abstract | Pubmed Full Text | CrossRef Full Text | Google Scholar
43. Kane LP, Andres PG, Howland KC, Abbas AK, Weiss A. Akt provides the CD28 costimulatory signal for up-regulation of IL-2 and IFN-gamma but not TH2 cytokines. Nat Immunol (2001) 2:37–44. doi:10.1038/83144
Pubmed Abstract | Pubmed Full Text | CrossRef Full Text | Google Scholar
44. Takeda K, Harada Y, Watanabe R, Inutake Y, Ogawa S, Onuki K, et al. CD28 stimulation triggers NF-kappaB activation through the CARMA1-PKCtheta-Grb2/Gads axis. Int Immunol (2008) 20:1507–15. doi:10.1093/intimm/dxn108
Pubmed Abstract | Pubmed Full Text | CrossRef Full Text | Google Scholar
45. Watanabe R, Harada Y, Takeda K, Takahashi J, Ohnuki K, Ogawa S, et al. Grb2 and Gads exhibit different interactions with CD28 and play distinct roles in CD28-mediated costimulation. J Immunol (2006) 177:1085–91. doi:10.4049/jimmunol.177.2.1085
Pubmed Abstract | Pubmed Full Text | CrossRef Full Text | Google Scholar
46. Ribot JC, Debarros A, Silva-Santos B. Searching for “signal 2”: costimulation requirements of γδ T cells. Cell Mol Life Sci (2011) 68:2345–55. doi:10.1007/s00018-011-0698-2
Pubmed Abstract | Pubmed Full Text | CrossRef Full Text | Google Scholar
47. Gravestein LA, Amsen D, Boes M, Calvo CR, Kruisbeek AM, Borst J. The TNF receptor family member CD27 signals to Jun N-terminal kinase via Traf-2. Eur J Immunol (1998) 28:2208–16. doi:10.1002/(SICI)1521-4141(199807)28:07<2208::AID-IMMU2208>3.0.CO;2-L
Pubmed Abstract | Pubmed Full Text | CrossRef Full Text | Google Scholar
48. Denoeud J, Moser M. Role of CD27/CD70 pathway of activation in immunity and tolerance. J Leukoc Biol (2011) 89:195–203. doi:10.1189/jlb.0610351
Pubmed Abstract | Pubmed Full Text | CrossRef Full Text | Google Scholar
49. Xue L, Chu F, Cheng Y, Sun X, Borthakur A, Ramarao M, et al. Siva-1 binds to and inhibits BCL-X(L)-mediated protection against UV radiation-induced apoptosis. Proc Natl Acad Sci U S A (2002) 99:6925–30. doi:10.1073/pnas.102182299
Pubmed Abstract | Pubmed Full Text | CrossRef Full Text | Google Scholar
50. Biswas P, Rovere P, De Filippi C, Heltai S, Smith C, Dagna L, et al. Engagement of CD30 shapes the secretion of cytokines by human gamma delta T cells. Eur J Immunol (2000) 30:2172–80. doi:10.1002/1521-4141(2000)30:18<2172::AID-IMMU2172>3.0.CO;2-5
Pubmed Abstract | Pubmed Full Text | CrossRef Full Text | Google Scholar
51. Biswas P, Mantelli B, Delfanti F, Ferrarini M, Poli G, Lazzarin A. CD30 ligation differentially affects CXCR4-dependent HIV-1 replication and soluble CD30 secretion in non-Hodgkin cell lines and in gamma delta T lymphocytes. Eur J Immunol (2003) 33:3136–45. doi:10.1002/eji.200324344
Pubmed Abstract | Pubmed Full Text | CrossRef Full Text | Google Scholar
52. Lee SJ, Kim YH, Hwang SH, Kim YI, Han IS, Vinay DS, et al. 4-1BB signal stimulates the activation, expansion, and effector functions of γδ T cells in mice and humans. Eur J Immunol (2013) 43:1839–48. doi:10.1002/eji.201242842
Pubmed Abstract | Pubmed Full Text | CrossRef Full Text | Google Scholar
53. Wang C, Lin GHY, McPherson AJ, Watts TH. Immune regulation by 4-1BB and 4-1BBL: complexities and challenges. Immunol Rev (2009) 229:192–215. doi:10.1111/j.1600-065X.2009.00765.x
Pubmed Abstract | Pubmed Full Text | CrossRef Full Text | Google Scholar
54. Jang IK, Lee ZH, Kim YJ, Kim SH, Kwon BS. Human 4-1BB (CD137) signals are mediated by TRAF2 and activate nuclear factor-kappa B. Biochem Biophys Res Commun (1998) 242:613–20. doi:10.1006/bbrc.1997.8016
Pubmed Abstract | Pubmed Full Text | CrossRef Full Text | Google Scholar
55. Ribot JC, Ribeiro ST, Correia DV, Sousa AE, Silva-Santos B. Human thymocytes are functionally immature and differentiate into cytotoxic type 1 effector T cells upon IL-2/IL-15 signaling. J Immunol (2014) 192(5):2237–43. doi:10.4049/jimmunol.1303119
Pubmed Abstract | Pubmed Full Text | CrossRef Full Text | Google Scholar
56. García VE, Jullien D, Song M, Uyemura K, Shuai K, Morita CT, et al. IL-15 enhances the response of human gamma delta T cells to nonpeptide [correction of nonpetide] microbial antigens. J Immunol (1998) 160:4322–9.
57. Li H, David Pauza C. Interplay of T-cell receptor and interleukin-2 signalling in Vγ2Vδ2 T-cell cytotoxicity. Immunology (2011) 132:96–103. doi:10.1111/j.1365-2567.2010.03343.x
Pubmed Abstract | Pubmed Full Text | CrossRef Full Text | Google Scholar
58. Liao W, Lin J-X, Leonard WJ. Interleukin-2 at the crossroads of effector responses, tolerance, and immunotherapy. Immunity (2013) 38:13–25. doi:10.1016/j.immuni.2013.01.004
Pubmed Abstract | Pubmed Full Text | CrossRef Full Text | Google Scholar
59. Michel ML, Pang DJ, Haque SF, Potocnik AJ, Pennington DJ, Hayday AC. Interleukin 7 (IL-7) selectively promotes mouse and human IL-17-producing gammadelta cells. Proc Natl Acad Sci U S A (2012) 109:17549–54. doi:10.1073/pnas.1204327109
Pubmed Abstract | Pubmed Full Text | CrossRef Full Text | Google Scholar
60. Bansal RR, Mackay CR, Moser B, Eberl M. IL-21 enhances the potential of human γδ T cells to provide B-cell help. Eur J Immunol (2012) 42:110–9. doi:10.1002/eji.201142017
Pubmed Abstract | Pubmed Full Text | CrossRef Full Text | Google Scholar
61. Long EO. Versatile signaling through NKG2D. Nat Immunol (2002) 3:1119–20. doi:10.1038/ni1202-1119
62. Lanier LL. DAP10- and DAP12-associated receptors in innate immunity. Immunol Rev (2009) 227:150–60. doi:10.1111/j.1600-065X.2008.00720.x
Pubmed Abstract | Pubmed Full Text | CrossRef Full Text | Google Scholar
63. Poggi A, Zancolli M, Boero S, Catellani S, Musso A, Zocchi MR. Differential survival of γδT cells, αβT cells and NK cells upon engagement of NKG2D by NKG2DL-expressing leukemic cells. Int J Cancer (2011) 129:387–96. doi:10.1002/ijc.25682
Pubmed Abstract | Pubmed Full Text | CrossRef Full Text | Google Scholar
64. Hudspeth K, Silva-Santos B, Mavilio D. Natural cytotoxicity receptors: broader expression patterns and functions in innate and adaptive immune cells. Front Immunol (2013) 4:69. doi:10.3389/fimmu.2013.00069
Pubmed Abstract | Pubmed Full Text | CrossRef Full Text | Google Scholar
65. Brandt CS, Baratin M, Yi EC, Kennedy J, Gao Z, Fox B, et al. The B7 family member B7-H6 is a tumor cell ligand for the activating natural killer cell receptor NKp30 in humans. J Exp Med (2009) 206:1495–503. doi:10.1084/jem.20090681
66. Martinet L, Jean C, Dietrich G, Fournié J-J, Poupot R. PGE2 inhibits natural killer and gamma delta T cell cytotoxicity triggered by NKR and TCR through a cAMP-mediated PKA type I-dependent signaling. Biochem Pharmacol (2010) 80:838–45. doi:10.1016/j.bcp.2010.05.002
Pubmed Abstract | Pubmed Full Text | CrossRef Full Text | Google Scholar
67. Hudspeth K, Fogli M, Correia DV, Mikulak J, Roberto A, Della Bella S, et al. Engagement of NKp30 on Vδ1 T cells induces the production of CCL3, CCL4, and CCL5 and suppresses HIV-1 replication. Blood (2012) 119:4013–6. doi:10.1182/blood-2011-11-390153
Pubmed Abstract | Pubmed Full Text | CrossRef Full Text | Google Scholar
68. Baychelier F, Sennepin A, Ermonval M, Dorgham K, Debré P, Vieillard V. Identification of a cellular ligand for the natural cytotoxicity receptor NKp44. Blood (2013) 122:2935–42. doi:10.1182/blood-2013-03-489054
Pubmed Abstract | Pubmed Full Text | CrossRef Full Text | Google Scholar
69. Vieillard V, Baychelier F, Debré P. NKp44L: a new tool for fighting cancer. Oncoimmunology (2014) 3:e27988. doi:10.4161/onci.27988
Pubmed Abstract | Pubmed Full Text | CrossRef Full Text | Google Scholar
70. McVicar DW, Taylor LS, Gosselin P, Willette-Brown J, Mikhael AI, Geahlen RL, et al. DAP12-mediated signal transduction in natural killer cells. A dominant role For The Syk protein-tyrosine kinase. J Biol Chem (1998) 273:32934–42. doi:10.1074/jbc.273.49.32934
Pubmed Abstract | Pubmed Full Text | CrossRef Full Text | Google Scholar
71. Toutirais O, Cabillic F, Le Friec G, Salot S, Loyer P, Le Gallo M, et al. DNAX accessory molecule-1 (CD226) promotes human hepatocellular carcinoma cell lysis by Vgamma9Vdelta2 T cells. Eur J Immunol (2009) 39:1361–8. doi:10.1002/eji.200838409
Pubmed Abstract | Pubmed Full Text | CrossRef Full Text | Google Scholar
72. Long EO, Kim HS, Liu D, Peterson ME, Rajagopalan S. Controlling natural killer cell responses: integration of signals for activation and inhibition. Annu Rev Immunol (2013) 31:227–58. doi:10.1146/annurev-immunol-020711-075005
Pubmed Abstract | Pubmed Full Text | CrossRef Full Text | Google Scholar
73. Parry RV, Chemnitz JM, Frauwirth KA, Lanfranco AR, Braunstein I, Kobayashi SV, et al. CTLA-4 and PD-1 receptors inhibit T-cell activation by distinct mechanisms. Mol Cell Biol (2005) 25:9543–53. doi:10.1128/MCB.25.21.9543-9553.2005
Pubmed Abstract | Pubmed Full Text | CrossRef Full Text | Google Scholar
74. Chemnitz JM, Parry RV, Nichols KE, June CH, Riley JL. SHP-1 and SHP-2 associate with immunoreceptor tyrosine-based switch motif of programmed death 1 upon primary human T cell stimulation, but only receptor ligation prevents T cell activation. J Immunol (2004) 173:945–54. doi:10.4049/jimmunol.173.2.945
Pubmed Abstract | Pubmed Full Text | CrossRef Full Text | Google Scholar
75. Patsoukis N, Li L, Sari D, Petkova V, Boussiotis VA. PD-1 increases PTEN phosphatase activity while decreasing PTEN protein stability by inhibiting CK2. Mol Cell Biol (2013) 33:3091–8. doi:10.1128/MCB.00319-13
Pubmed Abstract | Pubmed Full Text | CrossRef Full Text | Google Scholar
76. Patsoukis N, Brown J, Petkova V, Liu F, Li L, Boussiotis VA. Selective effects of PD-1 on Akt and Ras pathways regulate molecular components of the cell cycle and inhibit T cell proliferation. Sci Signal (2012) 5:ra46. doi:10.1126/scisignal.2002796
Pubmed Abstract | Pubmed Full Text | CrossRef Full Text | Google Scholar
77. Gertner-dardenne J, Fauriat C, Orlanducci F, Thibult M, Pastor S, Fitzgibbon J, et al. The co-receptor BTLA negatively regulates human Vγ9Vδ2 T-cell proliferation: a potential way of immune escape for lymphoma cells. Blood (2013) 122:922–31. doi:10.1182/blood-2012-11-464685
Pubmed Abstract | Pubmed Full Text | CrossRef Full Text | Google Scholar
78. Bekiaris V, Sedý JR, Macauley MG, Rhode-Kurnow A, Ware CF. The inhibitory receptor BTLA controls γδ T cell homeostasis and inflammatory responses. Immunity (2013) 39:1082–94. doi:10.1016/j.immuni.2013.10.017
Pubmed Abstract | Pubmed Full Text | CrossRef Full Text | Google Scholar
79. Watanabe N, Gavrieli M, Sedy JR, Yang J, Fallarino F, Loftin SK, et al. BTLA is a lymphocyte inhibitory receptor with similarities to CTLA-4 and PD-1. Nat Immunol (2003) 4:670–9. doi:10.1038/ni944
Pubmed Abstract | Pubmed Full Text | CrossRef Full Text | Google Scholar
80. Laird RM, Wolf BJ, Princiotta MF, Hayes SM. γδ T cells acquire effector fates in the thymus and differentiate into cytokine-producing effectors in a Listeria model of infection independently of CD28 costimulation. PLoS One (2013) 8:e63178. doi:10.1371/journal.pone.0063178
Pubmed Abstract | Pubmed Full Text | CrossRef Full Text | Google Scholar
81. Ribot JC, Chaves-Ferreira M, D’Orey F, Wencker M, Gonçalves-Sousa N, Decalf J, et al. Cutting edge: adaptive versus innate receptor signals selectively control the pool sizes of murine IFN-γ- or IL-17-producing γδ T cells upon infection. J Immunol (2010) 185:6421–5. doi:10.4049/jimmunol.1002283
Pubmed Abstract | Pubmed Full Text | CrossRef Full Text | Google Scholar
82. DeBarros A, Chaves-Ferreira M, D’Orey F, Ribot JC, Silva-Santos B. CD70-CD27 interactions provide survival and proliferative signals that regulate T cell receptor-driven activation of human gammadelta peripheral blood lymphocytes. Eur J Immunol (2011) 41:195–201. doi:10.1002/eji.201040905
Pubmed Abstract | Pubmed Full Text | CrossRef Full Text | Google Scholar
83. Ferrarini M, Delfanti F, Gianolini M, Rizzi C, Alfano M, Lazzarin A, et al. NF-κB modulates sensitivity to apoptosis, proinflammatory and migratory potential in short- versus long-term cultured human γδ lymphocytes. J Immunol (2008) 181:5857–64. doi:10.4049/jimmunol.181.9.5857
Pubmed Abstract | Pubmed Full Text | CrossRef Full Text | Google Scholar
84. Sun X, Shibata K, Yamada H, Guo Y, Muta H, Podack ER, et al. CD30L/CD30 is critical for maintenance of IL-17A-producing γδ T cells bearing Vγ6 in mucosa-associated tissues in mice. Mucosal Immunol (2013) 6:1191–201. doi:10.1038/mi.2013.18
Pubmed Abstract | Pubmed Full Text | CrossRef Full Text | Google Scholar
85. Maniar A, Zhang X, Lin W, Gastman BR, Pauza CD, Strome SE, et al. Human gammadelta T lymphocytes induce robust NK cell-mediated antitumor cytotoxicity through CD137 engagement. Blood (2010) 116:1726–33. doi:10.1182/blood-2009-07-234211
Pubmed Abstract | Pubmed Full Text | CrossRef Full Text | Google Scholar
86. Shao Z, Schwarz H. CD137 ligand, a member of the tumor necrosis factor family, regulates immune responses via reverse signal transduction. J Leukoc Biol (2011) 89:21–9. doi:10.1189/jlb.0510315
Pubmed Abstract | Pubmed Full Text | CrossRef Full Text | Google Scholar
87. Brandes M, Willimann K, Lang AB, Nam K-H, Jin C, Brenner MB, et al. Flexible migration program regulates gamma delta T-cell involvement in humoral immunity. Blood (2003) 102:3693–701. doi:10.1182/blood-2003-04-1016
Pubmed Abstract | Pubmed Full Text | CrossRef Full Text | Google Scholar
88. Malissen M, Pereira P, Gerber DJ, Malissen B, DiSanto JP. The common cytokine receptor gamma chain controls survival of gamma/delta T cells. J Exp Med (1997) 186:1277–85. doi:10.1084/jem.186.8.1277
Pubmed Abstract | Pubmed Full Text | CrossRef Full Text | Google Scholar
89. Shitara S, Hara T, Liang B, Wagatsuma K, Zuklys S, Holländer GA, et al. IL-7 produced by thymic epithelial cells plays a major role in the development of thymocytes and TCRγδ+ intraepithelial lymphocytes. J Immunol (2013) 190:6173–9. doi:10.4049/jimmunol.1202573
Pubmed Abstract | Pubmed Full Text | CrossRef Full Text | Google Scholar
90. He YW, Malek TR. Interleukin-7 receptor alpha is essential for the development of gamma delta + T cells, but not natural killer cells. J Exp Med (1996) 184:289–93. doi:10.1084/jem.184.1.289
Pubmed Abstract | Pubmed Full Text | CrossRef Full Text | Google Scholar
91. Baccala R, Witherden D, Gonzalez-Quintial R, Dummer W, Surh CD, Havran WL, et al. Gamma delta T cell homeostasis is controlled by IL-7 and IL-15 together with subset-specific factors. J Immunol (2005) 174:4606–12. doi:10.4049/jimmunol.174.8.4606
Pubmed Abstract | Pubmed Full Text | CrossRef Full Text | Google Scholar
92. Sumaria N, Roediger B, Ng LG, Qin J, Pinto R, Cavanagh LL, et al. Cutaneous immunosurveillance by self-renewing dermal gammadelta T cells. J Exp Med (2011) 208:505–18. doi:10.1084/jem.20101824
Pubmed Abstract | Pubmed Full Text | CrossRef Full Text | Google Scholar
93. Zhao H, Nguyen H, Kang J. Interleukin 15 controls the generation of the restricted T cell receptor repertoire of gamma delta intestinal intraepithelial lymphocytes. Nat Immunol (2005) 6:1263–71. doi:10.1038/ni1267
Pubmed Abstract | Pubmed Full Text | CrossRef Full Text | Google Scholar
94. Hou L, Jie Z, Desai M, Liang Y, Soong L, Wang T, et al. Early IL-17 production by intrahepatic T cells is important for adaptive immune responses in viral hepatitis. J Immunol (2013) 190:621–9. doi:10.4049/jimmunol.1201970
Pubmed Abstract | Pubmed Full Text | CrossRef Full Text | Google Scholar
95. Yamaguchi T, Suzuki Y, Katakura R, Ebina T, Yokoyama J, Fujimiya Y. Interleukin-15 effectively potentiates the in vitro tumor-specific activity and proliferation of peripheral blood γδT cells isolated from glioblastoma patients. Cancer Immunol Immunother (1998) 47:97–103. doi:10.1007/s002620050509
Pubmed Abstract | Pubmed Full Text | CrossRef Full Text | Google Scholar
96. Li H, Pauza CD. HIV envelope-mediated, CCR5/α4β7-dependent killing of CD4-negative γδ T cells which are lost during progression to AIDS. Blood (2011) 118:5824–31. doi:10.1182/blood-2011-05-356535
Pubmed Abstract | Pubmed Full Text | CrossRef Full Text | Google Scholar
97. Casetti R, Perretta G, Taglioni A, Mattei M, Colizzi V, Dieli F, et al. Drug-induced expansion and differentiation of Vgamma9Vdelta2 T cells in vivo: the role of exogenous IL-2. J Immunol (2005) 175:1593–8. doi:10.4049/jimmunol.175.3.1593
Pubmed Abstract | Pubmed Full Text | CrossRef Full Text | Google Scholar
98. Chen CY, Yao S, Huang D, Wei H, Sicard H, Zeng G, et al. Phosphoantigen/IL2 expansion and differentiation of Vγ2Vδ2 T cells increase resistance to tuberculosis in nonhuman primates. PLoS Pathog (2013) 9:e1003501. doi:10.1371/journal.ppat.1003501
Pubmed Abstract | Pubmed Full Text | CrossRef Full Text | Google Scholar
99. Yin Z, Zhang DH, Welte T, Bahtiyar G, Jung S, Liu L, et al. Dominance of IL-12 over IL-4 in gamma delta T cell differentiation leads to default production of IFN-gamma: failure to down-regulate IL-12 receptor beta 2-chain expression. J Immunol (2000) 164:3056–64. doi:10.4049/jimmunol.164.6.3056
Pubmed Abstract | Pubmed Full Text | CrossRef Full Text | Google Scholar
100. Li W, Kubo S, Okuda A, Yamamoto H, Ueda H, Tanaka T, et al. Effect of IL-18 on expansion of gammadelta T cells stimulated by zoledronate and IL-2. J Immunother (2010) 33:287–96. doi:10.1097/CJI.0b013e3181c80ffa
Pubmed Abstract | Pubmed Full Text | CrossRef Full Text | Google Scholar
101. Tsuda J, Li W, Yamanishi H, Yamamoto H, Okuda A, Kubo S, et al. Involvement of CD56brightCD11c+ cells in IL-18-mediated expansion of human γδ T cells. J Immunol (2011) 186:2003–12. doi:10.4049/jimmunol.1001919
Pubmed Abstract | Pubmed Full Text | CrossRef Full Text | Google Scholar
102. Thedrez A, Harly C, Morice A, Salot S, Bonneville M, Scotet E. IL-21-mediated potentiation of antitumor cytolytic and proinflammatory responses of human V gamma 9V delta 2 T cells for adoptive immunotherapy. J Immunol (2009) 182:3423–31. doi:10.4049/jimmunol.0803068
Pubmed Abstract | Pubmed Full Text | CrossRef Full Text | Google Scholar
103. Ness-Schwickerath KJ, Jin C, Morita CT. Cytokine requirements for the differentiation and expansion of IL-17A- and IL-22-producing human Vgamma2Vdelta2 T cells. J Immunol (2010) 184:7268–80. doi:10.4049/jimmunol.1000600
Pubmed Abstract | Pubmed Full Text | CrossRef Full Text | Google Scholar
104. Duan J, Chung H, Troy E, Kasper DL. Microbial colonization drives expansion of IL-1 receptor 1-expressing and IL-17-producing gamma/delta T cells. Cell Host Microbe (2010) 7:140–50. doi:10.1016/j.chom.2010.01.005
Pubmed Abstract | Pubmed Full Text | CrossRef Full Text | Google Scholar
105. Shibata K, Yamada H, Hara H, Kishihara K, Yoshikai Y. Resident Vdelta1+ gammadelta T cells control early infiltration of neutrophils after Escherichia coli infection via IL-17 production. J Immunol (2007) 178:4466–72. doi:10.4049/jimmunol.178.7.4466
Pubmed Abstract | Pubmed Full Text | CrossRef Full Text | Google Scholar
106. Riol-Blanco L, Lazarevic V, Awasthi A, Mitsdoerffer M, Wilson BS, Croxford A, et al. IL-23 receptor regulates unconventional IL-17-producing T cells that control bacterial infections. J Immunol (2010) 184:1710–20. doi:10.4049/jimmunol.0902796
Pubmed Abstract | Pubmed Full Text | CrossRef Full Text | Google Scholar
107. Lalor SJ, Dungan LS, Sutton CE, Basdeo SA, Fletcher JM, Mills KHG. Caspase-1-processed cytokines IL-1beta and IL-18 promote IL-17 production by gammadelta and CD4 T cells that mediate autoimmunity. J Immunol (2011) 186:5738–48. doi:10.4049/jimmunol.1003597
Pubmed Abstract | Pubmed Full Text | CrossRef Full Text | Google Scholar
108. Lukens JR, Barr MJ, Chaplin DD, Chi H, Kanneganti T-D. Inflammasome-derived IL-1β regulates the production of GM-CSF by CD4(+) T cells and γδ T cells. J Immunol (2012) 188:3107–15. doi:10.4049/jimmunol.1103308
Pubmed Abstract | Pubmed Full Text | CrossRef Full Text | Google Scholar
109. Caccamo N, Todaro M, La Manna MP, Sireci G, Stassi G, Dieli F. IL-21 regulates the differentiation of a human γδ T cell subset equipped with B cell helper activity. PLoS One (2012) 7:e41940. doi:10.1371/journal.pone.0041940
Pubmed Abstract | Pubmed Full Text | CrossRef Full Text | Google Scholar
110. Eagle RA, Trowsdale J. Promiscuity and the single receptor: NKG2D. Nat Rev Immunol (2007) 7:737–44. doi:10.1038/nri2144
Pubmed Abstract | Pubmed Full Text | CrossRef Full Text | Google Scholar
111. Gasser S, Orsulic S, Brown EJ, Raulet DH. The DNA damage pathway regulates innate immune system ligands of the NKG2D receptor. Nature (2005) 436:1186–90. doi:10.1038/nature03884
Pubmed Abstract | Pubmed Full Text | CrossRef Full Text | Google Scholar
112. Champsaur M, Lanier LL. Effect of NKG2D ligand expression on host immune responses. Immunol Rev (2010) 235:267–85. doi:10.1111/j.0105-2896.2010.00893.x
Pubmed Abstract | Pubmed Full Text | CrossRef Full Text | Google Scholar
113. Guerra N, Tan YX, Joncker NT, Choy A, Gallardo F, Xiong N, et al. NKG2D-deficient mice are defective in tumor surveillance in models of spontaneous malignancy. Immunity (2008) 28:571–80. doi:10.1016/j.immuni.2008.02.016
Pubmed Abstract | Pubmed Full Text | CrossRef Full Text | Google Scholar
114. Correia DV, Lopes A, Silva-Santos B. Tumor cell recognition by γδ T lymphocytes: T-cell receptor vs. NK-cell receptors. Oncoimmunology (2013) 2:e22892. doi:10.4161/onci.22892
Pubmed Abstract | Pubmed Full Text | CrossRef Full Text | Google Scholar
115. Das H, Groh V, Kuijl C, Sugita M, Morita CT, Spies T, et al. MICA engagement by human Vgamma2Vdelta2 T cells enhances their antigen-dependent effector function. Immunity (2001) 15:83–93. doi:10.1016/S1074-7613(01)00168-6
Pubmed Abstract | Pubmed Full Text | CrossRef Full Text | Google Scholar
116. Rincon-Orozco B, Kunzmann V, Wrobel P, Kabelitz D, Steinle A, Herrmann T. Activation of V gamma 9V delta 2 T cells by NKG2D. J Immunol (2005) 175:2144–51. doi:10.4049/jimmunol.175.4.2144
Pubmed Abstract | Pubmed Full Text | CrossRef Full Text | Google Scholar
117. Lança T, Correia DV, Moita CF, Raquel H, Neves-Costa A, Ferreira C, et al. The MHC class Ib protein ULBP1 is a nonredundant determinant of leukemia/lymphoma susceptibility to gammadelta T-cell cytotoxicity. Blood (2010) 115:2407–11. doi:10.1182/blood-2009-08-237123
Pubmed Abstract | Pubmed Full Text | CrossRef Full Text | Google Scholar
118. Nitahara A, Shimura H, Ito A, Tomiyama K, Ito M, Kawai K. NKG2D ligation without T cell receptor engagement triggers both cytotoxicity and cytokine production in dendritic epidermal T cells. J Invest Dermatol (2006) 126:1052–8. doi:10.1038/sj.jid.5700112
Pubmed Abstract | Pubmed Full Text | CrossRef Full Text | Google Scholar
119. De Andrade LF, Smyth MJ, Martinet L. DNAM-1 control of natural killer cells functions through nectin and nectin-like proteins. Immunol Cell Biol (2014) 92:237–44. doi:10.1038/icb.2013.95
Pubmed Abstract | Pubmed Full Text | CrossRef Full Text | Google Scholar
120. Correia DV, Fogli M, Hudspeth K, da Silva MG, Mavilio D, Silva-Santos B. Differentiation of human peripheral blood Vδ1+ T cells expressing the natural cytotoxicity receptor NKp30 for recognition of lymphoid leukemia cells. Blood (2011) 118:992–1001. doi:10.1182/blood-2011-02-339135
Pubmed Abstract | Pubmed Full Text | CrossRef Full Text | Google Scholar
121. Iwasaki M, Tanaka Y, Kobayashi H, Murata-Hirai K, Miyabe H, Sugie T, et al. Expression and function of PD-1 in human γδ T cells that recognize phosphoantigens. Eur J Immunol (2011) 41:345–55. doi:10.1002/eji.201040959
Pubmed Abstract | Pubmed Full Text | CrossRef Full Text | Google Scholar
122. Kulpa DA, Lawani M, Cooper A, Peretz Y, Ahlers J, Sékaly R-P. PD-1 coinhibitory signals: the link between pathogenesis and protection. Semin Immunol (2013) 25:219–27. doi:10.1016/j.smim.2013.02.002
Pubmed Abstract | Pubmed Full Text | CrossRef Full Text | Google Scholar
123. Pedoeem A, Azoulay-Alfaguter I, Strazza M, Silverman GJ, Mor A. Programmed death-1 pathway in cancer and autoimmunity. Clin Immunol (2014) 153:145–52. doi:10.1016/j.clim.2014.04.010
124. McGrath MM, Najafian N. The role of coinhibitory signaling pathways in transplantation and tolerance. Front Immunol (2012) 3:47. doi:10.3389/fimmu.2012.00047
Pubmed Abstract | Pubmed Full Text | CrossRef Full Text | Google Scholar
Keywords: γδ T-cells, T-cell receptor, T-cell costimulation, cytokines, natural killer receptors
Citation: Ribeiro ST, Ribot JC and Silva-Santos B (2015) Five layers of receptor signaling in γδ T-cell differentiation and activation. Front. Immunol. 6:15. doi: 10.3389/fimmu.2015.00015
Received: 21 October 2014; Accepted: 08 January 2015;
Published online: 26 January 2015.
Edited by:
Dieter Kabelitz, Christian-Albrechts University Kiel, GermanyCopyright: © 2015 Ribeiro, Ribot and Silva-Santos. This is an open-access article distributed under the terms of the Creative Commons Attribution License (CC BY). The use, distribution or reproduction in other forums is permitted, provided the original author(s) or licensor are credited and that the original publication in this journal is cited, in accordance with accepted academic practice. No use, distribution or reproduction is permitted which does not comply with these terms.
*Correspondence: Bruno Silva-Santos, Faculdade de Medicina, Instituto de Medicina Molecular, Universidade de Lisboa, Avenida Prof. Egas Moniz, Lisboa 1649-028, Portugal e-mail:YnNzYW50b3NAbWVkaWNpbmEudWxpc2JvYS5wdA==
Disclaimer: All claims expressed in this article are solely those of the authors and do not necessarily represent those of their affiliated organizations, or those of the publisher, the editors and the reviewers. Any product that may be evaluated in this article or claim that may be made by its manufacturer is not guaranteed or endorsed by the publisher.
Research integrity at Frontiers
Learn more about the work of our research integrity team to safeguard the quality of each article we publish.