- 1Surgery Branch, National Cancer Institute, Bethesda, MD, USA
- 2Division of Pediatrics, University of Texas MD Anderson Cancer Center, Houston, TX, USA
- 3The University of Texas Graduate School of Biomedical Sciences, UT MD Anderson Cancer Center, Houston, TX, USA
γδ T cells hold promise for adoptive immunotherapy because of their reactivity to bacteria, viruses, and tumors. However, these cells represent a small fraction (1–5%) of the peripheral T-cell pool and require activation and propagation to achieve clinical benefit. Aminobisphosphonates specifically expand the Vγ9Vδ2 subset of γδ T cells and have been used in clinical trials of cancer where objective responses were detected. The Vγ9Vδ2 T cell receptor (TCR) heterodimer binds multiple ligands and results in a multivalent attack by a monoclonal T cell population. Alternatively, populations of γδ T cells with oligoclonal or polyclonal TCR repertoire could be infused for broad-range specificity. However, this goal has been restricted by a lack of applicable expansion protocols for non-Vγ9Vδ2 cells. Recent advances using immobilized antigens, agonistic monoclonal antibodies (mAbs), tumor-derived artificial antigen presenting cells (aAPC), or combinations of activating mAbs and aAPC have been successful in expanding gamma delta T cells with oligoclonal or polyclonal TCR repertoires. Immobilized major histocompatibility complex Class-I chain-related A was a stimulus for γδ T cells expressing TCRδ1 isotypes, and plate-bound activating antibodies have expanded Vδ1 and Vδ2 cells ex vivo. Clinically sufficient quantities of TCRδ1, TCRδ2, and TCRδ1negTCRδ2neg have been produced following co-culture on aAPC, and these subsets displayed differences in memory phenotype and reactivity to tumors in vitro and in vivo. Gamma delta T cells are also amenable to genetic modification as evidenced by introduction of αβ TCRs, chimeric antigen receptors, and drug-resistance genes. This represents a promising future for the clinical application of oligoclonal or polyclonal γδ T cells in autologous and allogeneic settings that builds on current trials testing the safety and efficacy of Vγ9Vδ2 T cells.
Introduction
γδ T cells possess a combination of innate and adaptive immune cell qualities rendering them attractive for immunotherapy (1–3). They can produce inflammatory cytokines, directly lyse infected or malignant cells, and establish a memory response to attack pathogens upon re-exposure. γδ T cells are defined by expression of γ and δ heterodimer of T cell receptor (TCR) chains (TCRγ/TCRδ) that directs intracellular signaling through associated CD3 complexes (4). The γδ T-cell lineage (1–5% of circulating T cells) can be contrasted to the more prevalent αβ T cell lineage (~90%) in peripheral blood, which expresses TCRα/TCRβ heterodimers and also signals through associated CD3 complexes (5, 6). CD4 and CD8 co-receptors on αβ T cells assist binding of TCRαβ chains to the major histocompatibility complex (MHC) presenting processed peptides (7–9). In contrast, TCRγδ directly binds to an antigen’s superstructure independent of the MHC/peptide complexes and, as a result, CD4 and CD8 are uncommon on γδ T cells (10, 11). Given that antigen recognition is achieved outside of MHC/peptide-restriction, γδ T cells have predictable immune effector functions mediated through their TCR and have potential use as universal (“off-the-shelf”) allogeneic T-cell therapies (12).
Functional responses by γδ T cells can be stratified by the variable (V) region of the TCRδ chain. In humans, the TCRδ locus (TRD) lies within the TCRα locus (TRA). Three unique Vδ alleles, TRDV1, TRDV2, and TRDV3, code for TCRδ1, TCRδ2, and TCRδ3, respectively. Additionally, shared Vδ and Vα variable regions exist in TRDV4/TRAV14, TRDV5/TRAV29, TRDV6/TRAV23, TRDV7/TRAV36, and TRDV8/TRAV38-2 loci. Recombination of these shared V alleles with a TRA junction region (TRAJ) results in TCRα14, TCRα29, TCRα23, TCRα36, and TCRα38-2, respectively, but recombination of these shared V alleles with TRD junction (TRDJ) and diversity (TRDD) regions results in TCRδ4, TCRδ5, TCRδ6, TCRδ7, and TCRδ8, respectively (13). Expression of TCRγδ heterodimers on the T-cell surface in the thymus inhibits recombination of TCRβ-chain locus during the CD4negCD8neg stage thereby committing the T cell to the γδ T-cell lineage (14). This double negative status is typically maintained upon exit from the thymus, most likely because co-receptors are dispensable for functional TCRγδ binding to antigens (15). However, the thymus is not required to complete all γδ T-cell development, as many γδ T cells directly take up residence in peripheral tissues following exit from the bone marrow and exhibit immediate effector functions against pathogens (16). Thymus-independent “resident” γδ T cells can be found in the mucosa, tongue, vagina, intestine, lung, liver, and skin and can comprise up to 50% of the T-cell populations in intestinal epithelial lymphocytes (17, 18). In contrast, circulating γδ T cells can be found in the blood and lymphoid organs, and are dominated by γδ T cells preferentially expressing TCRδ2 isotype (commonly referred to as Vδ2 cells). Indeed, γδ T cells expressing the TCRδ1 isotype (commonly referred to as Vδ1 cells) are frequently found within tissues (19, 20). Vδ2 cells have preferred pairing with TCRγ9 (Vγ9Vδ2 cells), but broad γ-chain pairing is observed in Vδ1 cells and Vδ1negVδ2neg cells, a generic grouping of all other non-Vδ1/Vδ2 T cells (12, 19). Therefore, γδ T cells are distributed across an array of anatomical locations with a range of TCRγδ variable region expression.
Human TCRγδ ligands are MHC/peptide complex-independent and are therefore conserved amongst unrelated individuals. Most of the known human ligands are specific for TCRδ1 or TCRδ2. TCRγ1/TCRδ1 (alternatively termed Vγ1Vδ1) heterodimers have specificity for MHC Class-I chain-related A (MICA) (21, 22), a molecule participating in evasion of immune surveillance following viral infection and expressed on tumor cells as it is involved in the cellular stress response (23). MICA is also one of the ligands for NKG2D, which is expressed on γδ T cells, αβ T cells, and natural killer (NK) cells (23, 24). Both Vγ1Vδ1 and Vγ2Vδ1 recognize non-polymorphic MHC molecule CD1c (25), and Vγ5Vδ1 is a receptor for α-galactosylceramide-CD1d complexes commonly described in the activation of natural killer T (NKT) cells which, like γδ T cells, have both innate and adaptive immune functions and recognize conserved ligands amongst unrelated individuals (26, 27). γδ T cells can have specificity for virus as cytomegalovirus (CMV)-reactive Vγ8Vδ1 cells have been isolated from umbilical cord blood from infected newborns (28). Vδ1 cells have also been associated with immunity to human immunodeficiency virus (HIV), but the precise HIV ligands for TCRδ1 have not been determined (29). Bacterial alkylamines and Listeria monocytogenes are recognized by Vδ2 cells when paired with Vγ2 (30–32). Vγ9Vδ2 cells are the most extensively studied sub-group of human γδ T cells and their ligands include phosphoantigens [isopentenyl pyrophosphate (IPP)], F1-ATPase expressed on the cell surface, apolipoprotein A-I, and Mycobacterium tuberculosis (33–37). Moreover, Vγ9Vδ2 cells controlled and prevented lethal Epstein–Barr virus (EBV)-transformed leukemia xenografts in immunocompromised mice (4), and in vitro and in vivo data suggested that Vδ1 cells are also specific for EBV (38, 39). In contrast to Vδ1 and Vδ2 cells, very little is known about human γδ T cells expressing other TCRγδ alleles except for indirect evidence of Vδ3 cell’s immunity against CMV and HIV (40, 41). Given the multivalent nature of γδ T cells, harnessing γδ T cells populations with polyclonal TCR repertoire is attractive for adoptive immunotherapy.
γδ T-Cell Clinical Experience
Immunotherapy with γδ T cells requires their activation and expansion as they comprise only a small percentage of circulating T cells. Interleukin-2 (IL-2) and activating CD3 antibody (OKT3), commonly used for the propagation of αβ T cells directly from peripheral blood mononuclear cells (PBMC), do not reliably expand γδ T cells without further manipulation and so alternative approaches are needed. Aminobisphosphonates, e.g., Zoledronic Acid (Zol), used in the treatment of bone-related diseases, e.g., osteoporosis, resulted in in vivo propagation of γδ T cells, and the use of aminobisphosphonates has been subsequently translated into laboratory practice to grow γδ T cells ex vivo (Figure 1A) (42, 43). Aminobisphosphonates inhibit cholesterol synthesis and result in the accumulation of phosphoantigen intermediates in the mevalonate–CoA pathway, including IPP, a ligand for Vγ9Vδ2 (44). However, only the Vγ9Vδ2 T-cell subset is reactive to cells treated with phosphoantigens (45, 46). Synthetic phosphoantigens, e.g., bromohydrin pyrophosphate (BrHPP) (47) and 2-methyl-3-butenyl-1-pyrophosphate (2M3B1PP) (48), can mimic aminobisphosphonates and stimulate Vγ9Vδ2 T cells for proliferation.
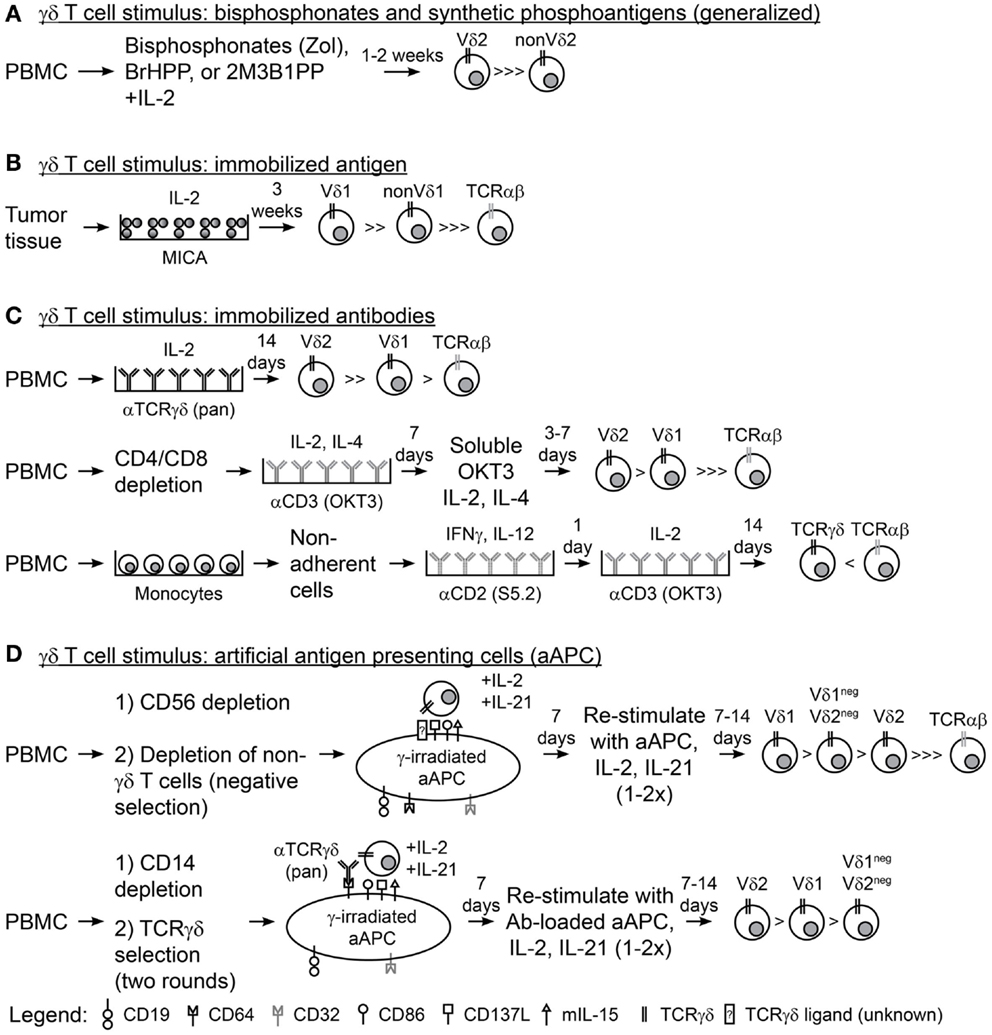
Figure 1. Methodologies for expanding γδ T cells ex vivo. (A) A generalized schematic for the use of aminobisphosphonates (Zol, zoledronic acid) or synthetic phosphoantigens (BrHPP, bromohydrin pyrophosphate; 2M3B1PP, 2-methyl-3-butenyl-1-pyrophosphate) and interleukin-2 (IL-2) to expand γδ T cells from peripheral blood mononuclear cells (PBMC). (B) Plate-bound MHC class-I chain-related (MICA) and IL-2 were used to expand γδ T cells from colon and ovarian tumor tissues. (C) Immobilized antibodies (Ab) were used to expand γδ T cells from PBMC in three scenarios: (top) PBMC directly stimulated with anti-pan-TCRγδ Ab and IL-2, (middle) PBMC depleted of CD4 and CD8 T cells followed by two rounds of stimulus with anti-CD3 Ab (OKT3), IL-2, and IL-4, and (bottom) PBMC were depleted of non-adherent cells, stimulated with anti-CD2 Ab (S5.2), interferon-γ (IFNγ), and IL-12, then stimulated with OKT3 and IL-2. (D) Schematic for the use of artificial antigen presenting cells (aAPC) to expand γδ T cells from PBMC in two scenarios: (top) PBMC was depleted of CD56+ NK cells then of other non-γδ T cells (TCRγ/δ+ magnetic bead kit) so that γδ T cell were isolated by “negative selection” and co-cultured recursively with aAPC, IL-2, and IL-21 for 2–3 rounds of stimulation; (bottom) PBMC was depleted of CD14+ monocytes and “positively selected” with TCRγδ magnetic beads then co-cultured recursively with anti-TCRγδ Ab-loaded aAPC, IL-2, and IL-21 for 2–3 rounds of stimulation.
These reagents have been transitioned to the clinic for investigational treatments of cancer and HIV (Table 1) (49, 50). Six trials have evaluated the ability of aminobisphosphonates or BrHPP to generate in vivo expansions of Vγ9Vδ2 T cells to fight leukemia/lymphoma (51, 52), melanoma (52), renal cell carcinoma (RCC) (52, 53), hormone-refractory prostate cancer (HRPC) (54), breast cancer (55), and HIV (56). These trials established safety of large Vγ9Vδ2 T cell expansions in vivo and generated a total of nine objective responses (11.3%; N = 80) but no complete responses (CR) as anti-tumor therapies. Six clinical trials have used either Zol, BrHPP, or 2M3B1PP to expand autologous Vγ9Vδ2 T cells ex vivo and these cells were directly infused (three trials with added IL-2 infusion and three without) for treatment of RCC (57–59), non-small cell lung cancer (NSCLC) (60, 61), and colorectal cancer (CRC) (62). Direct infusion of Vγ9Vδ2 T cells was established as a safe regimen and a total of eight objective responses (11.3%; N = 71) were detected, including one CR (1.4%; N = 71) (62). Three trials have evaluated the combination of adoptive transfer of ex vivo expanded Vγ9Vδ2 T cells followed by Zol administration to boost their in vivo proliferation. Multiple myeloma (63), RCC (64), and multiple metastatic tumors (melanoma, CRC, gastrointestinal tumors, ovarian cancer, breast cancer, cervical cancer, and bone cancer) (65) were treated with this combination, which was established to be safe, and four objective responses (13.8%; N = 29) were observed, two of which were CRs (6.9%; N = 29) treating intermediate-stage RCC (64) and breast cancer (65). Thus, adoptive transfer and in vivo expansions of Vγ9Vδ2 T cells are safe therapeutic modalities and can result in objective clinical responses in the treatment of cancer.
Allogeneic γδ T cells have also been infused but were part of heterogeneous cell populations (Table 1). Patients with acute myelogenous leukemia (AML) and acute lymphoblastic leukemia (ALL) were treated with αβ T cell-depleted hematopoietic stem cell transplant (HSCT), which resulted in 100 objective responses (65%; N = 153) with 36 durable CRs (24%; N = 153) (66–69). These complete remissions could be directly correlated to the elevated persistence of donor-derived Vδ1 cells in the peripheral blood of the patients, suggesting that these cells were involved in long-term clearance of leukemia. Increases in peripheral Vδ1 cells have also been correlated with CMV re-activation in patients with leukemia following allogeneic HSCT (40, 70). Most recently, haploidentical PBMC were depleted of CD4+ and CD8+ cells using magnetic beads and were administered to patients with refractory hematological malignancies followed by Zol and IL-2 infusions (71). Three of the four patients treated experienced short-lived CRs (2, 5, and 8 months) and the other patient died of infection 6 weeks after treatment. Expansion of γδ T cells was observed the week after treatment suggesting that they may have directed the anti-tumor response. Currently, clinical trials of direct infusion of activated, homogenous populations of Vδ1 cells, or other non-Vγ9Vδ2 cells have yet to be undertaken but hold promise as future avenues of medical intervention.
Ex vivo Propagation of Non-Vγ9Vδ2 γδ T Cells
Populations of γδ T cells outside of the Vγ9Vδ2 subset have been grown with immobilized TCRγδ agonists. Plate-bound recombinant MICA and IL-2 were used to sustain the proliferation of γδ T-cell cultures ex vivo from epithelial ovarian cancer and CRC tumor infiltrating lymphocytes (TILs) and resulted in high frequencies of Vδ1 cells (Figure 1B) (72). In addition, plate-bound pan-TCRγδ-specific antibody and IL-2 led to proliferation of both Vδ2 and Vδ1 cells (Vδ2 > > Vδ1) from peripheral blood derived from both healthy donors and patients with lung cancer or lymphoma (Figure 1C, top) (73, 74). Similarly, OKT3 has been used in combination with IL-2 and IL-4 to stimulate CD4/CD8-depleted T cells from healthy peripheral blood, which resulted in expansion of Vδ2 and Vδ1 cells (Vδ2 > Vδ1), albeit with reduced cell numbers compared to the TCRγδ monoclonal antibody (mAb)-stimulated cells (Figure 1C, middle) (75). A more complex cocktail of cytokines [IL-2, IL-12, and Interferon-γ (IFNγ)] has also been used with OKT3 and CD2-specific antibodies to expand γδ T cells, but the Vδ repertoires were not reported (Figure 1C, bottom) (76). Transition of these immobilized antigens and antibodies into clinical manufacture will streamline the application of these expansion strategies for γδ T cells and could be the source of clinical trials with non-Vγ9Vδ2 cells.
Highly polyclonal γδ T cells have been generated through co-culture of patient or healthy donor γδ T cells with irradiated artificial antigen presenting cells (aAPC), IL-2, and IL-21 (77–80). The aAPC (clone#4) are derived from the chronic myelogenous leukemia (CML) cell line K562 following genetic modification with T-cell co-stimulatory molecules (CD86 and CD137L), Fc receptors for antibody loading (introduced CD64 and endogenous CD32), antigens (CD19), and cytokines (a membrane-bound IL-15), and have been produced as a master cell bank (MCB) (81). This MCB is currently used in the production of αβ T cells for cancer treatments in clinical trials at MD Anderson (NCT01653717, NCT01619761, NCT00968760, and NCT01497184) (79, 82, 83). γ-irradiation of aAPC prior to co-culture with T cells subjects the aAPC to death (typically at or within 3 days) thereby reducing the risk for unintended transfer of this tumor cell line into recipients (83). Deniger et al. demonstrated that circulating γδ T cells, containing a polyclonal TCRγδ repertoire, could be isolated from healthy donor venipuncture or umbilical cord blood by “unlabeled/negative” magnetic bead selection and recursively stimulated with irradiated aAPC, IL-2, and IL-21 (Figure 1D, top). The aAPC-expanded γδ T cells proliferated to numbers sufficient for clinical use while maintaining the expression of most TRDV and TRGV alleles and demonstrating TCRδ surface expression of Vδ1 > Vδ1negVδ2neg > Vδ2 (77). These polyclonal γδ T-cell cultures displayed broad tumor reactivity as they were able to lyse leukemia, ovarian cancer, pancreatic cancer, and colon cancer cells. Separation of the polyclonal cultures by TCRδ surface expression showed that each T-cell subset had anti-tumor reactivity and that a polyclonal γδ T-cell population led to the superior survival of mice with established ovarian cancer xenografts. Propagation of Vδ1negVδ2neg cells had not been previously achieved and this was the first evidence of the functional activity of this γδ T-cell sub-population. In a similar study, Fisher et al. isolated polyclonal γδ T cells from PBMC of healthy donors or patients with neuroblastoma by first depleting monocytes followed by “positive/labeled” selection with anti-TCRγδ-hapten antibody and anti-hapten microbeads (Figure 1D, bottom) (79). This study made use of the Fc receptors on the aAPC surface to load anti-TCRγδ antibody where isolated γδ T cells were co-cultured with the antibody-loaded aAPC. These expanded γδ T cells expressed multiple TRDV and TRGV alleles with surface TCRδ expression of Vδ2 > Vδ1 > Vδ1negVδ2neg. Using this mode of expansion, Vδ1 and Vδ2 were mediators of antibody-independent (AIC) and antibody-dependent cellular cytotoxicity (ADCC), respectively, to neuroblastoma tumor cells (as predicted by whether or not they expressed Fc receptor CD16). aAPC-expanded polyclonal γδ T cells could be used for anti-tumor therapies because aAPC are currently available as a clinical reagent. However, human application of aAPC/mAb-expanded γδ T cells could depend on interest in the use of the current MCB of aAPC, generation of new MCB of aAPC at institutions where there are currently none, and production of γδ T cell agonistic antibodies in good manufacturing practice (GMP) conditions. Clinical testing of these cells could potentially lead to more widespread acceptance and use of γδ T cells as adoptive cellular therapies.
Given that the aAPC can sustain the proliferation of non-Vγ9Vδ2 cells to large quantities, there is opportunity for clinical translation, laboratory testing of subsets to elucidate their functions, and correlative studies. A limiting factor in studying γδ T cells has been the lack of TCRδ and TCRγ isotype-specific antibodies outside of specificity for TCRδ1, TCRδ2, TCRγ9, and TCRδ3 (where commercially available). Mice can now be immunized to generate mAb specific for desired TCRγδ isotypes where commercial and academic use of these detection antibodies can have tangible outcomes, including diagnostic and/or prognostic profiling of γδ T cells resident within tumors. γδ T-cell clones could be generated through co-culture of single γδ T cells with aAPC, and this can facilitate studies to determine Vδ/Vγ pairing, corresponding TCRγδ ligands, and pathogenic reactivity. The ligands on the K562-derived aAPC that TCRγδ binds are not currently known. Likely candidates include IPP and MICA/B for TCRδ2 and TCRδ1, respectively (22, 35). Elucidation of these interactions could assist attempts to tailor the design of the aAPC for total γδ T-cell expansion, propagation of a particular γδ T-cell lineage, or polarization toward a certain γδ T-cell phenotype (84). As an example, CD27neg and CD27+ γδ T cells are associated with IL17 and IFNγ production, respectively (85–87), leading to the conclusion that expression of CD70, the CD27 ligand, on aAPC could potentially polarize these T cells toward a desired cytokine output. Thus, aAPC could be an excellent source for the study of fundamental γδ T-cell immunobiology and could yield answers not currently accessible because of limited starting cell numbers and ineffective polyclonal expansion protocols.
Genetic Modification of γδ T Cells for Therapeutic Use
γδ T cells are also amenable to genetic modification allowing for the introduction of genes to improve their therapeutic function. For instance, re-directed specificity of T cells can also be accomplished through the introduction of recombinant TCRs with defined antigen specificity. The conventional thought is that transfer of TCRα/TCRβ genes into γδ T cells or transfer of TCRγ/TCRδ genes into αβ T cells would not cause mis-pairing with the TCRα/TCRβ and TCRγ/TCRδ heterodimers, thereby mitigating the risk of generating inappropriate pairings such as TCRα/TCRδ, TCRα/TCRγ, TCRβ/TCRγ, or TCRβ/TCRδ heterodimers with unknown specificity (88). This mis-pairing hypothesis was modeled in mice with the ovalbumin-specific αβ TCR OT-I, which resulted in re-directed specificity of murine γδ T cells toward ovalbumin peptide, but whether or not the TCRs were actually mis-paired was not reported (89). Vγ2Vδ2 cells have been expanded with 2M3B1PP and infected with γ-retrovirus to transduce TCRαβ chains with specificity toward MAGE-A4 peptide, but co-transduction with CD8 was required in order to transfer significant MHC Class-I-restricted recognition of MAGE-A4 peptide-pulsed tumor cells (90, 91). Similar studies have transferred αβ TCRs specific for CMV pp65 peptide or minor histocompatibility antigens into γδ T cells rendering them reactive to antigen-appropriate tumor cells (92). In contrast to the above reports of introducing αβ TCRs into γδ T cells, the Vγ9Vδ2 TCR has been transferred into αβ T cells and rendered both CD4+ and CD8+ T cells reactive to multiple tumor cell lines (93). Chemotherapy (temozolomide)-resistant γδ T cells have been generated by lentiviral transduction of (6)-alkylguanine DNA alkyltransferase into Vγ9Vδ2 cells expanded on Zol (94). Chimeric antigen receptors (CARs) can be introduced into T cells and re-direct the T cell toward a specific antigen. CARs are formed by fusing a single chain antibody to one or more T-cell intracellular signaling domains, e.g., CD3ζ, CD28, and/or CD137 (95). The antibody confers specificity through its variable regions toward a particular antigen, e.g., CD19, GD2, HER2, etc., and CAR binding to the antigen transmits intracellular T-cell signals for antigen-dependent proliferation, cytokine production, and cytolysis (96, 97). Following expansion on Zol, Vγ9Vδ2 cells were efficiently transduced to express CD19- and GD2-specific CARs with γ-retroviral vectors and displayed re-directed specificity toward CD19+ and GD2+ tumor targets, respectively (98). Zol and γ-retroviruses engineered to transduce CD19- and GD2-specific CARs are available for human application, but have not been combined in a clinical trial to date. Thus, subsets of γδ T cells are amenable to viral gene transfer to improve their therapeutic impact.
In contrast to γ-retroviruses and lentiviruses, which require cell division for efficient transduction, non-viral Sleeping Beauty (SB) transposition transfers genes into quiescent T cells and allows manipulation of cells that are difficult to culture ex vivo (99–102). SB transposase enzyme was originally derived from fish that were undergoing active transposition in their evolutionary maturation and was adapted for human application (103). In short, a DNA transposon with flanking inverted repeats and direct repeats is ligated into the human genome at TA dinucleotide repeats by the SB transposase enzyme (104). TA dinucleotide repeats are widely distributed in the human genome, yielding potential for random integration into the genome, and have been shown to be safe in regards to transgene insertion in pre-clinical studies (99, 101, 105). This is of particular importance in gene therapy as inappropriate integration at gene start sites or promoters, within exons, or even distal to genes within enhancers or repressors may cause cellular transformation. Lentiviruses and γ-retroviruses have higher efficiency in transgene delivery than SB, but these vectors are known to integrate near genes or within genes (97). Application of SB to human clinical-grade T cells has been reduced to practice as a two DNA plasmid system, where one plasmid contains the SB transposon with the transgene of interest, e.g., CAR, and the other plasmid encodes a hyperactive SB transposase (106). Electro-transfer of the DNA plasmids by nucleofection into circulating (quiescent) PBMC results in transient expression of SB transposase that then ligates the transposon into the genome using a “cut-and-paste” mechanism. As soon as the SB transposase mRNA is degraded translation of SB transposase protein is halted, thereby negating additional transposition events. T cells with stable CAR expression can be encouraged through the co-culture of T cells on irradiated aAPC that express antigen for the CAR (83). This process, originally developed for αβ T cells, has been adapted for expression of CAR in γδ T cells (78). Resting PBMCs were electroporated with CD19-specific CAR transposon and SB11 transposase plasmids and sorted the following day to deplete non-γδ T cells with magnetic beads from the transfected mixture. Isolated γδ T cells were recursively stimulated with CD19+ aAPC along with IL-2 and IL-21, which resulted in the outgrowth of CAR+ γδ T cells with a highly polyclonal TCRγδ repertoire. Endogenous leukemia reactivity by the aAPC-expanded γδ T cells was improved through expression of CD19-specific CAR rendering these T cells bi-specific through CAR and TCRγδ. SB transposon and transposase are available as clinical reagents; therefore, clinical trials can test the safety and efficacy of bi-specific CAR+ γδ T cells.
Concluding Remarks
Given that γδ T cells are unlikely to cause graft-versus-host disease (GVHD) because their TCR ligands (IPP, MICA, etc.) are not MHC-restricted, γδ T cells (with or without genetic modification) could be generated from healthy donors in a third party manufacturing facility and given in the allogeneic setting as an “off-the-shelf” therapeutic. Additionally, a “universal” bank of polyclonal γδ T cells could be established that was known to have high anti-tumor immunity or contain a particular set frequency of Vδ1, Vδ2, and Vδ1negVδ2neg populations to achieve superior efficacy (66). This could have specialized application in cases where T cells were difficult to manufacture, e.g., high tumor burden in blood or after extensive systemic (lymphodepleting) chemotherapy. Polyclonal γδ T cells could also be used as front-line therapy before addition of HSCT, CAR+ T cells, TILs, etc. in order to prime the tumor microenvironment for other adaptive immune cells with broader tumor specificity or to reveal neo-tumor antigens, including somatic non-synonymous mutations expressed only in the tumor (107–109). If immunity is restored in the recipients then the 3rd party γδ T-cell graft may be rejected, but there may still be a therapeutic window before this occurs. Both pro-tumor and anti-tumor effects of γδ T cells infiltrating the tumor microenvironment have been described (110, 111), and whether or not these cells could be useful for therapy could be delineated following expansion of γδ T cells from solid tumors on aAPC, which have been shown to expand TIL (αβ T cells) from metastatic melanoma (112). Tumor lysis by γδ T cells could lead to other resident cell types, e.g., NK cells, macrophages, αβ T cells, etc., to have renewed reactivity to the malignancy (113). Indeed, B-ALL cell lines coated with mAb were lysed by CD16+ Vγ9Vδ2 cells via ADCC, and subsequently the Vγ9Vδ2 had antigen presenting cell function to generate antigen-specific CD8+ αβ T cell responses to known B-ALL peptides, e.g., PAX5 (114, 115). Unknown is whether γδ T cells will be subjected to inhibition by regulatory T cells or other immunosuppressive forces. Some γδ T cells have been reported to have immunosuppressive function, and it would be of interest to identify these cells and eliminate them from the adoptive T-cell product prior to infusion (116). In summary, administration of graded doses of autologous and allogeneic, even 3rd party, γδ T cells in humans have tested and will continue to evaluate the ability of these lymphocytes to home and recycle effector function in the tumor microenvironment. Given the development of aminobisphosphonates, synthetic phosphoantigens, immobilized antigens, antibodies, and designer clinical-grade aAPC, it now appears practical to sculpt and expand γδ T cells to achieve a therapeutic effect.
Author Contributions
Drew C. Deniger, Judy S. Moyes, and Laurence J. N. Cooper wrote the manuscript.
Conflict of Interest Statement
Dr. Cooper founded and owns InCellerate, Inc. He has patents with Sangamo BioSciences with artificial nucleases. He consults with Targazyme, Inc. (formerly American Stem cells, Inc.), GE Healthcare, Ferring Pharmaceuticals, Inc., and Bristol-Myers Squibb. He receives honoraria from Miltenyi Biotec. Other authors declare no other competing financial interests.
Acknowledgments
Funding for this work was provided by Cancer Center Core Grant (CA16672); RO1 (CA124782, CA120956, CA141303; CA141303); P01 (CA148600); SPORE (CA83639); Albert J. Ward Foundation; Alex Lemonade Stand Foundation; American Legion Auxiliary, Burroughs Wellcome Fund; Cancer Answers; Cancer Prevention and Research Institute of Texas; Charles B. Goddard Foundation of Texas; CLL Global Research Foundation; DARPA (Defense Sciences Office); Department of Defense; Estate of Noelan L. Bibler; Gillson Longenbaugh Foundation; Harry T. Mangurian, Jr., Fund for Leukemia Immunotherapy; Khalifa Bin Zayed Al Nahyan Foundation; Leukemia and Lymphoma Society; Lymphoma Research Foundation; Miller Foundation; Moon Shot program at MDACC, Mr. Herb Simons; Mr. and Mrs. Joe H. Scales; Mr. Thomas Scott; National Foundation for Cancer Research; Pediatric Cancer Research Foundation; Sheikh Khalifa Bin Zayed Al Nahyan Institute for Personalized Cancer Therapy; R. W. Butcher Foundation, University of Texas MD Anderson Cancer Center Sister Institution Network Fund and Moon Shot Fund; William Lawrence and Blanche Hughes Children’s Foundation.
Abbreviations
2M3B1PP, 2-methyl-3-butenyl-1-pyrophosphate; AML, acute myeloid leukemia; BrHPP, bromohydrin pyrophosphate; CLL, chronic lymphocytic leukemia; CRC, colorectal cancer; EOC, epithelial ovarian cancer; FCL, follicle center lymphoma; GI-cancer, cancers from the gastrointestinal tract; HIV, human immunodeficiency virus; HRPC, hormone-refractory prostate cancer; IC, immunocytoma; MM, multiple myeloma; MZL, mantle zone lymphoma; N/D, not determined; NHL, T-cell non-Hodgkin lymphoma; NSCLC, non-small-cell lung cancer; RCC, renal cell carcinoma; TBI, total body irradiation; T-SPL, secondary plasma cell leukemia; Zol, zoledronic acid.
References
1. Vantourout P, Hayday A. Six-of-the-best: unique contributions of gammadelta T cells to immunology. Nat Rev Immunol (2013) 13(2):88–100. doi:10.1038/nri3384
Pubmed Abstract | Pubmed Full Text | CrossRef Full Text | Google Scholar
2. Kabelitz D. Gammadelta T-cells: cross-talk between innate and adaptive immunity. Cell Mol Life Sci (2011) 68(14):2331–3. doi:10.1007/s00018-011-0696-4
3. Hannani D, Ma Y, Yamazaki T, Dechanet-Merville J, Kroemer G, Zitvogel L. Harnessing gammadelta T cells in anticancer immunotherapy. Trends Immunol (2012) 33(5):199–206. doi:10.1016/j.it.2012.01.006
4. Xiang Z, Liu Y, Zheng J, Liu M, Lv A, Gao Y, et al. Targeted activation of human Vγ9Vδ2-T cells controls Epstein-Barr virus-induced B Cell lymphoproliferative disease. Cancer Cell (2014) 26(4):565–76. doi:10.1016/j.ccr.2014.07.026
Pubmed Abstract | Pubmed Full Text | CrossRef Full Text | Google Scholar
5. Smith-Garvin JE, Koretzky GA, Jordan MS. T cell activation. Annu Rev Immunol (2009) 27:591–619. doi:10.1146/annurev.immunol.021908.132706
6. Kalyan S, Kabelitz D. Defining the nature of human gammadelta T cells: a biographical sketch of the highly empathetic. Cell Mol Immunol (2013) 10(1):21–9. doi:10.1038/cmi.2012.44
Pubmed Abstract | Pubmed Full Text | CrossRef Full Text | Google Scholar
7. Koretzky GA. Multiple roles of CD4 and CD8 in T cell activation. J Immunol (2010) 185(5):2643–4. doi:10.4049/jimmunol.1090076
8. Van Laethem F, Tikhonova AN, Singer A. MHC restriction is imposed on a diverse T cell receptor repertoire by CD4 and CD8 co-receptors during thymic selection. Trends Immunol (2012) 33(9):437–41. doi:10.1016/j.it.2012.05.006
Pubmed Abstract | Pubmed Full Text | CrossRef Full Text | Google Scholar
9. Germain RN. T-cell development and the CD4-CD8 lineage decision. Nat Rev Immunol (2002) 2(5):309–22. doi:10.1038/nri798
Pubmed Abstract | Pubmed Full Text | CrossRef Full Text | Google Scholar
10. Sato K, Ohtsuka K, Watanabe H, Asakura H, Abo T. Detailed characterization of gamma delta T cells within the organs in mice: classification into three groups. Immunology (1993) 80(3):380–7.
11. Kabelitz D, Kalyan S, Oberg HH, Wesch D. Human Vdelta2 versus non-Vdelta2 gammadelta T cells in antitumor immunity. Oncoimmunology (2013) 2(3):e23304. doi:10.4161/onci.23304
Pubmed Abstract | Pubmed Full Text | CrossRef Full Text | Google Scholar
12. Kabelitz D, Wesch D, He W. Perspectives of gammadelta T cells in tumor immunology. Cancer Res (2007) 67(1):5–8. doi:10.1158/0008-5472.CAN-06-3069
Pubmed Abstract | Pubmed Full Text | CrossRef Full Text | Google Scholar
13. Lefranc MP. Nomenclature of the human T cell receptor genes. Curr Protoc Immunol (2001) Appendix 1:Appendix 1O. doi:10.1002/0471142735.ima01os40
Pubmed Abstract | Pubmed Full Text | CrossRef Full Text | Google Scholar
14. Xiong N, Raulet DH. Development and selection of gammadelta T cells. Immunol Rev (2007) 215:15–31. doi:10.1111/j.1600-065X.2006.00478.x
15. Hayday AC. Gammadelta T cells and the lymphoid stress-surveillance response. Immunity (2009) 31(2):184–96. doi:10.1016/j.immuni.2009.08.006
Pubmed Abstract | Pubmed Full Text | CrossRef Full Text | Google Scholar
16. Hao J, Wu X, Xia S, Li Z, Wen T, Zhao N, et al. Current progress in gammadelta T-cell biology. Cell Mol Immunol (2010) 7(6):409–13. doi:10.1038/cmi.2010.50
17. Ishikawa H, Naito T, Iwanaga T, Takahashi-Iwanaga H, Suematsu M, Hibi T, et al. Curriculum vitae of intestinal intraepithelial T cells: their developmental and behavioral characteristics. Immunol Rev (2007) 215:154–65. doi:10.1111/j.1600-065X.2006.00473.x
Pubmed Abstract | Pubmed Full Text | CrossRef Full Text | Google Scholar
18. Carding SR, Egan PJ. Gammadelta T cells: functional plasticity and heterogeneity. Nat Rev Immunol (2002) 2(5):336–45. doi:10.1038/nri797
Pubmed Abstract | Pubmed Full Text | CrossRef Full Text | Google Scholar
19. Bonneville M, O’Brien RL, Born WK. Gammadelta T cell effector functions: a blend of innate programming and acquired plasticity. Nat Rev Immunol (2010) 10(7):467–78. doi:10.1038/nri2781
Pubmed Abstract | Pubmed Full Text | CrossRef Full Text | Google Scholar
20. Chodaczek G, Papanna V, Zal MA, Zal T. Body-barrier surveillance by epidermal gammadelta TCRs. Nat Immunol (2012) 13(3):272–82. doi:10.1038/ni.2240
Pubmed Abstract | Pubmed Full Text | CrossRef Full Text | Google Scholar
21. Xu B, Pizarro JC, Holmes MA, McBeth C, Groh V, Spies T, et al. Crystal structure of a gammadelta T-cell receptor specific for the human MHC class I homolog MICA. Proc Natl Acad Sci U S A (2011) 108(6):2414–9. doi:10.1073/pnas.1015433108
Pubmed Abstract | Pubmed Full Text | CrossRef Full Text | Google Scholar
22. Li J, Cui L, He W. Distinct pattern of human Vdelta1 gammadelta T cells recognizing MICA. Cell Mol Immunol (2005) 2(4):253–8.
23. Bauer S, Groh V, Wu J, Steinle A, Phillips JH, Lanier LL, et al. Activation of NK cells and T cells by NKG2D, a receptor for stress-inducible MICA. Science (1999) 285(5428):727–9. doi:10.1126/science.285.5428.727
Pubmed Abstract | Pubmed Full Text | CrossRef Full Text | Google Scholar
24. Wrobel P, Shojaei H, Schittek B, Gieseler F, Wollenberg B, Kalthoff H, et al. Lysis of a broad range of epithelial tumour cells by human gamma delta T cells: involvement of NKG2D ligands and T-cell receptor- versus NKG2D-dependent recognition. Scand J Immunol (2007) 66(2–3):320–8. doi:10.1111/j.1365-3083.2007.01963.x
Pubmed Abstract | Pubmed Full Text | CrossRef Full Text | Google Scholar
25. Spada FM, Grant EP, Peters PJ, Sugita M, Melian A, Leslie DS, et al. Self-recognition of CD1 by gamma/delta T cells: implications for innate immunity. J Exp Med (2000) 191(6):937–48. doi:10.1084/jem.191.6.937
Pubmed Abstract | Pubmed Full Text | CrossRef Full Text | Google Scholar
26. Uldrich AP, Le Nours J, Pellicci DG, Gherardin NA, McPherson KG, Lim RT, et al. CD1d-lipid antigen recognition by the gammadelta TCR. Nat Immunol (2013) 14(11):1137–45. doi:10.1038/ni.2713
27. Paget C, Chow MT, Duret H, Mattarollo SR, Smyth MJ. Role of gammadelta T cells in alpha-galactosylceramide-mediated immunity. J Immunol (2012) 188(8):3928–39. doi:10.4049/jimmunol.1103582
Pubmed Abstract | Pubmed Full Text | CrossRef Full Text | Google Scholar
28. Vermijlen D, Brouwer M, Donner C, Liesnard C, Tackoen M, Van Rysselberge M, et al. Human cytomegalovirus elicits fetal gammadelta T cell responses in utero. J Exp Med (2010) 207(4):807–21. doi:10.1084/jem.20090348
Pubmed Abstract | Pubmed Full Text | CrossRef Full Text | Google Scholar
29. Wesch D, Hinz T, Kabelitz D. Analysis of the TCR Vgamma repertoire in healthy donors and HIV-1-infected individuals. Int Immunol (1998) 10(8):1067–75. doi:10.1093/intimm/10.8.1067
Pubmed Abstract | Pubmed Full Text | CrossRef Full Text | Google Scholar
30. Bukowski JF, Morita CT, Brenner MB. Human gamma delta T cells recognize alkylamines derived from microbes, edible plants, and tea: implications for innate immunity. Immunity (1999) 11(1):57–65. doi:10.1016/S1074-7613(00)80081-3
Pubmed Abstract | Pubmed Full Text | CrossRef Full Text | Google Scholar
31. Wang L, Kamath A, Das H, Li L, Bukowski JF. Antibacterial effect of human V gamma 2V delta 2 T cells in vivo. J Clin Invest (2001) 108(9):1349–57. doi:10.1172/JCI13584
Pubmed Abstract | Pubmed Full Text | CrossRef Full Text | Google Scholar
32. Munk ME, Elser C, Kaufmann SH. Human gamma/delta T-cell response to Listeria monocytogenes protein components in vitro. Immunology (1996) 87(2):230–5. doi:10.1046/j.1365-2567.1996.470549.x
Pubmed Abstract | Pubmed Full Text | CrossRef Full Text | Google Scholar
33. Green AE, Lissina A, Hutchinson SL, Hewitt RE, Temple B, James D, et al. Recognition of nonpeptide antigens by human V gamma 9V delta 2 T cells requires contact with cells of human origin. Clin Exp Immunol (2004) 136(3):472–82. doi:10.1111/j.1365-2249.2004.02472.x
Pubmed Abstract | Pubmed Full Text | CrossRef Full Text | Google Scholar
34. Mookerjee-Basu J, Vantourout P, Martinez LO, Perret B, Collet X, Perigaud C, et al. F1-adenosine triphosphatase displays properties characteristic of an antigen presentation molecule for Vgamma9Vdelta2 T cells. J Immunol (2010) 184(12):6920–8. doi:10.4049/jimmunol.0904024
Pubmed Abstract | Pubmed Full Text | CrossRef Full Text | Google Scholar
35. Vantourout P, Mookerjee-Basu J, Rolland C, Pont F, Martin H, Davrinche C, et al. Specific requirements for Vgamma9Vdelta2 T cell stimulation by a natural adenylated phosphoantigen. J Immunol (2009) 183(6):3848–57. doi:10.4049/jimmunol.0901085
Pubmed Abstract | Pubmed Full Text | CrossRef Full Text | Google Scholar
36. Scotet E, Martinez LO, Grant E, Barbaras R, Jeno P, Guiraud M, et al. Tumor recognition following Vgamma9Vdelta2 T cell receptor interactions with a surface F1-ATPase-related structure and apolipoprotein A-I. Immunity (2005) 22(1):71–80. doi:10.1016/j.immuni.2004.11.012
Pubmed Abstract | Pubmed Full Text | CrossRef Full Text | Google Scholar
37. Constant P, Davodeau F, Peyrat MA, Poquet Y, Puzo G, Bonneville M, et al. Stimulation of human gamma delta T cells by nonpeptidic mycobacterial ligands. Science (1994) 264(5156):267–70. doi:10.1126/science.8146660
Pubmed Abstract | Pubmed Full Text | CrossRef Full Text | Google Scholar
38. Farnault L, Gertner-Dardenne J, Gondois-Rey F, Michel G, Chambost H, Hirsch I, et al. Clinical evidence implicating gamma-delta T cells in EBV control following cord blood transplantation. Bone Marrow Transplant (2013) 48(11):1478–9. doi:10.1038/bmt.2013.75
39. Hacker G, Kromer S, Falk M, Heeg K, Wagner H, Pfeffer K. V delta 1+ subset of human gamma delta T cells responds to ligands expressed by EBV-infected Burkitt lymphoma cells and transformed B lymphocytes. J Immunol (1992) 149(12):3984–9.
40. Knight A, Madrigal AJ, Grace S, Sivakumaran J, Kottaridis P, Mackinnon S, et al. The role of Vdelta2-negative gammadelta T cells during cytomegalovirus reactivation in recipients of allogeneic stem cell transplantation. Blood (2010) 116(12):2164–72. doi:10.1182/blood-2010-01-255166
Pubmed Abstract | Pubmed Full Text | CrossRef Full Text | Google Scholar
41. Kabelitz D, Hinz T, Dobmeyer T, Mentzel U, Marx S, Bohme A, et al. Clonal expansion of Vgamma3/Vdelta3-expressing gammadelta T cells in an HIV-1/2-negative patient with CD4 T-cell deficiency. Br J Haematol (1997) 96(2):266–71. doi:10.1046/j.1365-2141.1997.d01-2027.x
Pubmed Abstract | Pubmed Full Text | CrossRef Full Text | Google Scholar
42. Thompson K, Roelofs AJ, Jauhiainen M, Monkkonen H, Monkkonen J, Rogers MJ. Activation of gammadelta T cells by bisphosphonates. Adv Exp Med Biol (2010) 658:11–20. doi:10.1007/978-1-4419-1050-9_2
43. Nagamine I, Yamaguchi Y, Ohara M, Ikeda T, Okada M. Induction of gamma delta T cells using zoledronate plus interleukin-2 in patients with metastatic cancer. Hiroshima J Med Sci (2009) 58(1):37–44.
44. Chiplunkar S, Dhar S, Wesch D, Kabelitz D. gammadelta T cells in cancer immunotherapy: current status and future prospects. Immunotherapy (2009) 1(4):663–78. doi:10.2217/imt.09.27
Pubmed Abstract | Pubmed Full Text | CrossRef Full Text | Google Scholar
45. D’Asaro M, La Mendola C, Di Liberto D, Orlando V, Todaro M, Spina M, et al. V gamma 9V delta 2 T lymphocytes efficiently recognize and kill zoledronate-sensitized, imatinib-sensitive, and imatinib-resistant chronic myelogenous leukemia cells. J Immunol (2010) 184(6):3260–8. doi:10.4049/jimmunol.0903454
Pubmed Abstract | Pubmed Full Text | CrossRef Full Text | Google Scholar
46. Thedrez A, Harly C, Morice A, Salot S, Bonneville M, Scotet E. IL-21-mediated potentiation of antitumor cytolytic and proinflammatory responses of human V gamma 9V delta 2 T cells for adoptive immunotherapy. J Immunol (2009) 182(6):3423–31. doi:10.4049/jimmunol.0803068
Pubmed Abstract | Pubmed Full Text | CrossRef Full Text | Google Scholar
47. Chargui J, Combaret V, Scaglione V, Iacono I, Peri V, Valteau-Couanet D, et al. Bromohydrin pyrophosphate-stimulated Vgamma9delta2 T cells expanded ex vivo from patients with poor-prognosis neuroblastoma lyse autologous primary tumor cells. J Immunother (2010) 33(6):591–8. doi:10.1097/CJI.0b013e3181dda207
Pubmed Abstract | Pubmed Full Text | CrossRef Full Text | Google Scholar
48. Espinosa E, Belmant C, Pont F, Luciani B, Poupot R, Romagne F, et al. Chemical synthesis and biological activity of bromohydrin pyrophosphate, a potent stimulator of human gamma delta T cells. J Biol Chem (2001) 276(21):18337–44. doi:10.1074/jbc.M100495200
Pubmed Abstract | Pubmed Full Text | CrossRef Full Text | Google Scholar
49. Fisher JP, Heuijerjans J, Yan M, Gustafsson K, Anderson J. gammadelta T cells for cancer immunotherapy: a systematic review of clinical trials. Oncoimmunology (2014) 3(1):e27572. doi:10.4161/onci.27572
Pubmed Abstract | Pubmed Full Text | CrossRef Full Text | Google Scholar
50. Gomes AQ, Martins DS, Silva-Santos B. Targeting gammadelta T lymphocytes for cancer immunotherapy: from novel mechanistic insight to clinical application. Cancer Res (2010) 70(24):10024–7. doi:10.1158/0008-5472.CAN-10-3236
Pubmed Abstract | Pubmed Full Text | CrossRef Full Text | Google Scholar
51. Wilhelm M, Kunzmann V, Eckstein S, Reimer P, Weissinger F, Ruediger T, et al. Gammadelta T cells for immune therapy of patients with lymphoid malignancies. Blood (2003) 102(1):200–6. doi:10.1182/blood-2002-12-3665
Pubmed Abstract | Pubmed Full Text | CrossRef Full Text | Google Scholar
52. Kunzmann V, Smetak M, Kimmel B, Weigang-Koehler K, Goebeler M, Birkmann J, et al. Tumor-promoting versus tumor-antagonizing roles of gammadelta T cells in cancer immunotherapy: results from a prospective phase I/II trial. J Immunother (2012) 35(2):205–13. doi:10.1097/CJI.0b013e318245bb1e
Pubmed Abstract | Pubmed Full Text | CrossRef Full Text | Google Scholar
53. Lang JM, Kaikobad MR, Wallace M, Staab MJ, Horvath DL, Wilding G, et al. Pilot trial of interleukin-2 and zoledronic acid to augment gammadelta T cells as treatment for patients with refractory renal cell carcinoma. Cancer Immunol Immunother (2011) 60(10):1447–60. doi:10.1007/s00262-011-1049-8
Pubmed Abstract | Pubmed Full Text | CrossRef Full Text | Google Scholar
54. Dieli F, Vermijlen D, Fulfaro F, Caccamo N, Meraviglia S, Cicero G, et al. Targeting human {gamma}delta} T cells with zoledronate and interleukin-2 for immunotherapy of hormone-refractory prostate cancer. Cancer Res (2007) 67(15):7450–7. doi:10.1158/0008-5472.CAN-07-0199
Pubmed Abstract | Pubmed Full Text | CrossRef Full Text | Google Scholar
55. Meraviglia S, Eberl M, Vermijlen D, Todaro M, Buccheri S, Cicero G, et al. In vivo manipulation of Vgamma9Vdelta2 T cells with zoledronate and low-dose interleukin-2 for immunotherapy of advanced breast cancer patients. Clin Exp Immunol (2010) 161(2):290–7. doi:10.1111/j.1365-2249.2010.04167.x
Pubmed Abstract | Pubmed Full Text | CrossRef Full Text | Google Scholar
56. Poccia F, Gioia C, Martini F, Sacchi A, Piacentini P, Tempestilli M, et al. Zoledronic acid and interleukin-2 treatment improves immunocompetence in HIV-infected persons by activating Vgamma9Vdelta2 T cells. AIDS (2009) 23(5):555–65. doi:10.1097/QAD.0b013e3283244619
Pubmed Abstract | Pubmed Full Text | CrossRef Full Text | Google Scholar
57. Kobayashi H, Tanaka Y, Yagi J, Osaka Y, Nakazawa H, Uchiyama T, et al. Safety profile and anti-tumor effects of adoptive immunotherapy using gamma-delta T cells against advanced renal cell carcinoma: a pilot study. Cancer Immunol Immunother (2007) 56(4):469–76. doi:10.1007/s00262-006-0199-6
Pubmed Abstract | Pubmed Full Text | CrossRef Full Text | Google Scholar
58. Bennouna J, Bompas E, Neidhardt EM, Rolland F, Philip I, Galea C, et al. Phase-I study of Innacell gammadelta, an autologous cell-therapy product highly enriched in gamma9delta2 T lymphocytes, in combination with IL-2, in patients with metastatic renal cell carcinoma. Cancer Immunol Immunother (2008) 57(11):1599–609. doi:10.1007/s00262-008-0491-8
Pubmed Abstract | Pubmed Full Text | CrossRef Full Text | Google Scholar
59. Bennouna J, Levy V, Sicard H, Senellart H, Audrain M, Hiret S, et al. Phase I study of bromohydrin pyrophosphate (BrHPP, IPH 1101), a Vgamma9Vdelta2 T lymphocyte agonist in patients with solid tumors. Cancer Immunol Immunother (2010) 59(10):1521–30. doi:10.1007/s00262-010-0879-0
Pubmed Abstract | Pubmed Full Text | CrossRef Full Text | Google Scholar
60. Nakajima J, Murakawa T, Fukami T, Goto S, Kaneko T, Yoshida Y, et al. A phase I study of adoptive immunotherapy for recurrent non-small-cell lung cancer patients with autologous gammadelta T cells. Eur J Cardiothorac Surg (2010) 37(5):1191–7. doi:10.1016/j.ejcts.2009.11.051
Pubmed Abstract | Pubmed Full Text | CrossRef Full Text | Google Scholar
61. Sakamoto M, Nakajima J, Murakawa T, Fukami T, Yoshida Y, Murayama T, et al. Adoptive immunotherapy for advanced non-small cell lung cancer using zoledronate-expanded gammadeltaTcells: a phase I clinical study. J Immunother (2011) 34(2):202–11. doi:10.1097/CJI.0b013e318207ecfb
Pubmed Abstract | Pubmed Full Text | CrossRef Full Text | Google Scholar
62. Izumi T, Kondo M, Takahashi T, Fujieda N, Kondo A, Tamura N, et al. Ex vivo characterization of gammadelta T-cell repertoire in patients after adoptive transfer of Vgamma9Vdelta2 T cells expressing the interleukin-2 receptor beta-chain and the common gamma-chain. Cytotherapy (2013) 15(4):481–91. doi:10.1016/j.jcyt.2012.12.004
Pubmed Abstract | Pubmed Full Text | CrossRef Full Text | Google Scholar
63. Abe Y, Muto M, Nieda M, Nakagawa Y, Nicol A, Kaneko T, et al. Clinical and immunological evaluation of zoledronate-activated Vgamma9gammadelta T-cell-based immunotherapy for patients with multiple myeloma. Exp Hematol (2009) 37(8):956–68. doi:10.1016/j.exphem.2009.04.008
Pubmed Abstract | Pubmed Full Text | CrossRef Full Text | Google Scholar
64. Kobayashi H, Tanaka Y, Yagi J, Minato N, Tanabe K. Phase I/II study of adoptive transfer of gammadelta T cells in combination with zoledronic acid and IL-2 to patients with advanced renal cell carcinoma. Cancer Immunol Immunother (2011) 60(8):1075–84. doi:10.1007/s00262-011-1021-7
Pubmed Abstract | Pubmed Full Text | CrossRef Full Text | Google Scholar
65. Nicol AJ, Tokuyama H, Mattarollo SR, Hagi T, Suzuki K, Yokokawa K, et al. Clinical evaluation of autologous gamma delta T cell-based immunotherapy for metastatic solid tumours. Br J Cancer (2011) 105(6):778–86. doi:10.1038/bjc.2011.293
Pubmed Abstract | Pubmed Full Text | CrossRef Full Text | Google Scholar
66. Godder KT, Henslee-Downey PJ, Mehta J, Park BS, Chiang KY, Abhyankar S, et al. Long term disease-free survival in acute leukemia patients recovering with increased gammadelta T cells after partially mismatched related donor bone marrow transplantation. Bone Marrow Transplant (2007) 39(12):751–7. doi:10.1038/sj.bmt.1705650
67. Lamb LS Jr, Gee AP, Hazlett LJ, Musk P, Parrish RS, O’Hanlon TP, et al. Influence of T cell depletion method on circulating gammadelta T cell reconstitution and potential role in the graft-versus-leukemia effect. Cytotherapy (1999) 1(1):7–19. doi:10.1080/0032472031000141295
Pubmed Abstract | Pubmed Full Text | CrossRef Full Text | Google Scholar
68. Lamb LS Jr, Henslee-Downey PJ, Parrish RS, Godder K, Thompson J, Lee C, et al. Increased frequency of TCR gamma delta + T cells in disease-free survivors following T cell-depleted, partially mismatched, related donor bone marrow transplantation for leukemia. J Hematother (1996) 5(5):503–9. doi:10.1089/scd.1.1996.5.503
Pubmed Abstract | Pubmed Full Text | CrossRef Full Text | Google Scholar
69. Lamb LS Jr, Musk P, Ye Z, van Rhee F, Geier SS, Tong JJ, et al. Human gammadelta(+) T lymphocytes have in vitro graft vs leukemia activity in the absence of an allogeneic response. Bone Marrow Transplant (2001) 27(6):601–6. doi:10.1038/sj.bmt.1702830
Pubmed Abstract | Pubmed Full Text | CrossRef Full Text | Google Scholar
70. Scheper W, van Dorp S, Kersting S, Pietersma F, Lindemans C, Hol S, et al. gammadeltaT cells elicited by CMV reactivation after allo-SCT cross-recognize CMV and leukemia. Leukemia (2013) 27(6):1328–38. doi:10.1038/leu.2012.374
Pubmed Abstract | Pubmed Full Text | CrossRef Full Text | Google Scholar
71. Wilhelm M, Smetak M, Schaefer-Eckart K, Kimmel B, Birkmann J, Einsele H, et al. Successful adoptive transfer and in vivo expansion of haploidentical gammadelta T cells. J Transl Med (2014) 12:45. doi:10.1186/1479-5876-12-45
Pubmed Abstract | Pubmed Full Text | CrossRef Full Text | Google Scholar
72. Qi J, Zhang J, Zhang S, Cui L, He W. Immobilized MICA could expand human Vdelta1 gammadelta T cells in vitro that displayed major histocompatibility complex class I chain-related A-dependent cytotoxicity to human epithelial carcinomas. Scand J Immunol (2003) 58(2):211–20. doi:10.1046/j.1365-3083.2003.01288.x
Pubmed Abstract | Pubmed Full Text | CrossRef Full Text | Google Scholar
73. Kang N, Zhou J, Zhang T, Wang L, Lu F, Cui Y, et al. Adoptive immunotherapy of lung cancer with immobilized anti-TCRgammadelta antibody-expanded human gammadelta T-cells in peripheral blood. Cancer Biol Ther (2009) 8(16):1540–9. doi:10.4161/cbt.8.16.8950
Pubmed Abstract | Pubmed Full Text | CrossRef Full Text | Google Scholar
74. Zhou J, Kang N, Cui L, Ba D, He W. Anti-gammadelta TCR antibody-expanded gammadelta T cells: a better choice for the adoptive immunotherapy of lymphoid malignancies. Cell Mol Immunol (2012) 9(1):34–44. doi:10.1038/cmi.2011.16
Pubmed Abstract | Pubmed Full Text | CrossRef Full Text | Google Scholar
75. Dokouhaki P, Han M, Joe B, Li M, Johnston MR, Tsao MS, et al. Adoptive immunotherapy of cancer using ex vivo expanded human gammadelta T cells: a new approach. Cancer Lett (2010) 297(1):126–36. doi:10.1016/j.canlet.2010.05.005
Pubmed Abstract | Pubmed Full Text | CrossRef Full Text | Google Scholar
76. Lopez RD, Xu S, Guo B, Negrin RS, Waller EK. CD2-mediated IL-12-dependent signals render human gamma delta-T cells resistant to mitogen-induced apoptosis, permitting the large-scale ex vivo expansion of functionally distinct lymphocytes: implications for the development of adoptive immunotherapy strategies. Blood (2000) 96(12):3827–37.
77. Deniger DC, Maiti S, Mi T, Switzer K, Ramachandran V, Hurton LV, et al. Activating and propagating polyclonal gamma delta T cells with broad specificity for malignancies. Clin Cancer Res (2014) 20(22):5708–19. doi:10.1158/1078-0432.CCR-13-3451
Pubmed Abstract | Pubmed Full Text | CrossRef Full Text | Google Scholar
78. Deniger DC, Switzer K, Mi T, Maiti S, Hurton L, Singh H, et al. Bispecific T-cells expressing polyclonal repertoire of endogenous gammadelta T-cell receptors and introduced CD19-specific chimeric antigen receptor. Mol Ther (2013) 21(3):638–47. doi:10.1038/mt.2012.267
Pubmed Abstract | Pubmed Full Text | CrossRef Full Text | Google Scholar
79. Fisher J, Yan M, Heuijerjans J, Carter L, Abolhassani A, Frosch J, et al. Neuroblastoma killing properties of V-δ 2 and V-δ2 negative gamma delta T cells following expansion by artificial antigen presenting cells. Clin Cancer Res (2014) 20(22):5720–32. doi:10.1158/1078-0432.CCR-13-3464
Pubmed Abstract | Pubmed Full Text | CrossRef Full Text | Google Scholar
80. Dechanet-Merville J. Promising cell-based immunotherapy using gamma delta T cells: together is better. Clin Cancer Res (2014) 20(22):5573–5. doi:10.1158/1078-0432.CCR-14-1371
Pubmed Abstract | Pubmed Full Text | CrossRef Full Text | Google Scholar
81. O’Connor CM, Sheppard S, Hartline CA, Huls H, Johnson M, Palla SL, et al. Adoptive T-cell therapy improves treatment of canine non-Hodgkin lymphoma post chemotherapy. Sci Rep (2012) 2:249. doi:10.1038/srep00249
Pubmed Abstract | Pubmed Full Text | CrossRef Full Text | Google Scholar
82. Singh H, Figliola MJ, Dawson MJ, Olivares S, Zhang L, Yang G, et al. Manufacture of clinical-grade CD19-specific T cells stably expressing chimeric antigen receptor using sleeping beauty system and artificial antigen presenting cells. PLoS One (2013) 8(5):e64138. doi:10.1371/journal.pone.0064138
83. Huls MH, Figliola MJ, Dawson MJ, Olivares S, Kebriaei P, Shpall EJ, et al. Clinical application of sleeping beauty and artificial antigen presenting cells to genetically modify T cells from peripheral and umbilical cord blood. J Vis Exp (2013) 72:e50070. doi:10.3791/50070
Pubmed Abstract | Pubmed Full Text | CrossRef Full Text | Google Scholar
84. Rushworth D, Jena B, Olivares S, Maiti S, Briggs N, Somanchi S, et al. Universal artificial antigen presenting cells to selectively propagate T cells expressing chimeric antigen receptor independent of specificity. J Immunother (2014) 37(4):204–13. doi:10.1097/CJI.0000000000000032
Pubmed Abstract | Pubmed Full Text | CrossRef Full Text | Google Scholar
85. Caccamo N, La Mendola C, Orlando V, Meraviglia S, Todaro M, Stassi G, et al. Differentiation, phenotype, and function of interleukin-17-producing human Vgamma9Vdelta2 T cells. Blood (2011) 118(1):129–38. doi:10.1182/blood-2011-01-331298
Pubmed Abstract | Pubmed Full Text | CrossRef Full Text | Google Scholar
86. DeBarros A, Chaves-Ferreira M, d’Orey F, Ribot JC, Silva-Santos B. CD70-CD27 interactions provide survival and proliferative signals that regulate T cell receptor-driven activation of human gammadelta peripheral blood lymphocytes. Eur J Immunol (2011) 41(1):195–201. doi:10.1002/eji.201040905
Pubmed Abstract | Pubmed Full Text | CrossRef Full Text | Google Scholar
87. Ribot JC, deBarros A, Pang DJ, Neves JF, Peperzak V, Roberts SJ, et al. CD27 is a thymic determinant of the balance between interferon-gamma- and interleukin 17-producing gammadelta T cell subsets. Nat Immunol (2009) 10(4):427–36. doi:10.1038/ni.1717
Pubmed Abstract | Pubmed Full Text | CrossRef Full Text | Google Scholar
88. Heemskerk MH. T-cell receptor gene transfer for the treatment of leukemia and other tumors. Haematologica (2010) 95(1):15–9. doi:10.3324/haematol.2009.016022
89. van der Veken LT, Coccoris M, Swart E, Falkenburg JH, Schumacher TN, Heemskerk MH. Alpha beta T cell receptor transfer to gamma delta T cells generates functional effector cells without mixed TCR dimers in vivo. J Immunol (2009) 182(1):164–70. doi:10.4049/jimmunol.182.1.164
Pubmed Abstract | Pubmed Full Text | CrossRef Full Text | Google Scholar
90. Hiasa A, Nishikawa H, Hirayama M, Kitano S, Okamoto S, Chono H, et al. Rapid alphabeta TCR-mediated responses in gammadelta T cells transduced with cancer-specific TCR genes. Gene Ther (2009) 16(5):620–8. doi:10.1038/gt.2009.6
Pubmed Abstract | Pubmed Full Text | CrossRef Full Text | Google Scholar
91. Murayama M, Tanaka Y, Yagi J, Uchiyama T, Ogawa K. Antitumor activity and some immunological properties of gammadelta T-cells from patients with gastrointestinal carcinomas. Anticancer Res (2008) 28(5B):2921–31.
92. van der Veken LT, Hagedoorn RS, van Loenen MM, Willemze R, Falkenburg JH, Heemskerk MH. Alphabeta T-cell receptor engineered gammadelta T cells mediate effective antileukemic reactivity. Cancer Res (2006) 66(6):3331–7. doi:10.1158/0008-5472.CAN-05-4190
Pubmed Abstract | Pubmed Full Text | CrossRef Full Text | Google Scholar
93. Marcu-Malina V, Heijhuurs S, van Buuren M, Hartkamp L, Strand S, Sebestyen Z, et al. Redirecting alphabeta T cells against cancer cells by transfer of a broadly tumor-reactive gammadeltaT-cell receptor. Blood (2011) 118(1):50–9. doi:10.1182/blood-2010-12-325993
Pubmed Abstract | Pubmed Full Text | CrossRef Full Text | Google Scholar
94. Lamb LS Jr, Bowersock J, Dasgupta A, Gillespie GY, Su Y, Johnson A, et al. Engineered drug resistant gammadelta T cells kill glioblastoma cell lines during a chemotherapy challenge: a strategy for combining chemo- and immunotherapy. PLoS One (2013) 8(1):e51805. doi:10.1371/journal.pone.0051805
Pubmed Abstract | Pubmed Full Text | CrossRef Full Text | Google Scholar
95. June CH. Principles of adoptive T cell cancer therapy. J Clin Invest (2007) 117(5):1204–12. doi:10.1172/JCI32446
Pubmed Abstract | Pubmed Full Text | CrossRef Full Text | Google Scholar
96. Singh H, Huls H, Kebriaei P, Cooper LJ. A new approach to gene therapy using sleeping beauty to genetically modify clinical-grade T cells to target CD19. Immunol Rev (2014) 257(1):181–90. doi:10.1111/imr.12137
Pubmed Abstract | Pubmed Full Text | CrossRef Full Text | Google Scholar
97. Jena B, Dotti G, Cooper LJ. Redirecting T-cell specificity by introducing a tumor-specific chimeric antigen receptor. Blood (2010) 116(7):1035–44. doi:10.1182/blood-2010-01-043737
Pubmed Abstract | Pubmed Full Text | CrossRef Full Text | Google Scholar
98. Rischer M, Pscherer S, Duwe S, Vormoor J, Jurgens H, Rossig C. Human gammadelta T cells as mediators of chimeric-receptor redirected anti-tumour immunity. Br J Haematol (2004) 126(4):583–92. doi:10.1111/j.1365-2141.2004.05077.x
Pubmed Abstract | Pubmed Full Text | CrossRef Full Text | Google Scholar
99. Hackett PB Jr, Aronovich EL, Hunter D, Urness M, Bell JB, Kass SJ, et al. Efficacy and safety of sleeping beauty transposon-mediated gene transfer in preclinical animal studies. Curr Gene Ther (2011) 11(5):341–9. doi:10.2174/156652311797415827
Pubmed Abstract | Pubmed Full Text | CrossRef Full Text | Google Scholar
100. Hackett PB, Largaespada DA, Cooper LJ. A transposon and transposase system for human application. Mol Ther (2010) 18(4):674–83. doi:10.1038/mt.2010.2
Pubmed Abstract | Pubmed Full Text | CrossRef Full Text | Google Scholar
101. Hackett PB, Largaespada DA, Switzer KC, Cooper LJ. Evaluating risks of insertional mutagenesis by DNA transposons in gene therapy. Transl Res (2013) 161(4):265–83. doi:10.1016/j.trsl.2012.12.005
Pubmed Abstract | Pubmed Full Text | CrossRef Full Text | Google Scholar
102. Maiti SN, Huls H, Singh H, Dawson M, Figliola M, Olivares S, et al. Sleeping beauty system to redirect T-cell specificity for human applications. J Immunother (2013) 36(2):112–23. doi:10.1097/CJI.0b013e3182811ce9
Pubmed Abstract | Pubmed Full Text | CrossRef Full Text | Google Scholar
103. Wadman SA, Clark KJ, Hackett PB. Fishing for answers with transposons. Mar Biotechnol (NY) (2005) 7(3):135–41. doi:10.1007/s10126-004-0068-2
Pubmed Abstract | Pubmed Full Text | CrossRef Full Text | Google Scholar
104. Liu G, Aronovich EL, Cui Z, Whitley CB, Hackett PB. Excision of sleeping beauty transposons: parameters and applications to gene therapy. J Gene Med (2004) 6(5):574–83. doi:10.1002/jgm.486
Pubmed Abstract | Pubmed Full Text | CrossRef Full Text | Google Scholar
105. Liu G, Geurts AM, Yae K, Srinivasan AR, Fahrenkrug SC, Largaespada DA, et al. Target-site preferences of sleeping beauty transposons. J Mol Biol (2005) 346(1):161–73. doi:10.1016/j.jmb.2004.09.086
Pubmed Abstract | Pubmed Full Text | CrossRef Full Text | Google Scholar
106. Singh H, Manuri PR, Olivares S, Dara N, Dawson MJ, Huls H, et al. Redirecting specificity of T-cell populations for CD19 using the sleeping beauty system. Cancer Res (2008) 68(8):2961–71. doi:10.1158/0008-5472.CAN-07-5600
Pubmed Abstract | Pubmed Full Text | CrossRef Full Text | Google Scholar
107. Tran E, Rosenberg SA. T-cell therapy against cancer mutations. Oncotarget (2014) 5(13):4579–80.
108. Tran E, Turcotte S, Gros A, Robbins PF, Lu YC, Dudley ME, et al. Cancer immunotherapy based on mutation-specific CD4+ T cells in a patient with epithelial cancer. Science (2014) 344(6184):641–5. doi:10.1126/science.1251102
Pubmed Abstract | Pubmed Full Text | CrossRef Full Text | Google Scholar
109. Robbins PF, Lu YC, El-Gamil M, Li YF, Gross C, Gartner J, et al. Mining exomic sequencing data to identify mutated antigens recognized by adoptively transferred tumor-reactive T cells. Nat Med (2013) 19(6):747–52. doi:10.1038/nm.3161
Pubmed Abstract | Pubmed Full Text | CrossRef Full Text | Google Scholar
110. Peng G, Wang HY, Peng W, Kiniwa Y, Seo KH, Wang RF. Tumor-infiltrating gammadelta T cells suppress T and dendritic cell function via mechanisms controlled by a unique toll-like receptor signaling pathway. Immunity (2007) 27(2):334–48. doi:10.1016/j.immuni.2007.05.020
Pubmed Abstract | Pubmed Full Text | CrossRef Full Text | Google Scholar
111. Raspollini MR, Castiglione F, Rossi Degl’innocenti D, Amunni G, Villanucci A, Garbini F, et al. Tumour-infiltrating gamma/delta T-lymphocytes are correlated with a brief disease-free interval in advanced ovarian serous carcinoma. Ann Oncol (2005) 16(4):590–6. doi:10.1093/annonc/mdi112
Pubmed Abstract | Pubmed Full Text | CrossRef Full Text | Google Scholar
112. Forget MA, Malu S, Liu H, Toth C, Maiti S, Kale C, et al. Activation and propagation of tumor-infiltrating lymphocytes on clinical-grade designer artificial antigen-presenting cells for adoptive immunotherapy of melanoma. J Immunother (2014) 37(9):448–60. doi:10.1097/CJI.0000000000000056
Pubmed Abstract | Pubmed Full Text | CrossRef Full Text | Google Scholar
113. Anderson J, Gustafsson K, Himoudi N. Licensing of killer dendritic cells in mouse and humans: functional similarities between IKDC and human blood gammadelta T-lymphocytes. J Immunotoxicol (2012) 9(3):259–66. doi:10.3109/1547691X.2012.685528
Pubmed Abstract | Pubmed Full Text | CrossRef Full Text | Google Scholar
114. Himoudi N, Morgenstern DA, Yan M, Vernay B, Saraiva L, Wu Y, et al. Human gammadelta T lymphocytes are licensed for professional antigen presentation by interaction with opsonized target cells. J Immunol (2012) 188(4):1708–16. doi:10.4049/jimmunol.1102654
Pubmed Abstract | Pubmed Full Text | CrossRef Full Text | Google Scholar
115. Anderson J, Gustafsson K, Himoudi N, Yan M, Heuijerjans J. Licensing of gammadeltaT cells for professional antigen presentation: a new role for antibodies in regulation of antitumor immune responses. Oncoimmunology (2012) 1(9):1652–4. doi:10.4161/onci.21971
Pubmed Abstract | Pubmed Full Text | CrossRef Full Text | Google Scholar
116. Kang N, Tang L, Li X, Wu D, Li W, Chen X, et al. Identification and characterization of Foxp3(+) gammadelta T cells in mouse and human. Immunol Lett (2009) 125(2):105–13. doi:10.1016/j.imlet.2009.06.005
Pubmed Abstract | Pubmed Full Text | CrossRef Full Text | Google Scholar
Keywords: cancer, immunotherapy, γδ T cells, adoptive T-cell therapy, T-cell receptor, allogeneic transplantation, chimeric antigen receptors, artificial APC
Citation: Deniger DC, Moyes JS and Cooper LJN (2014) Clinical applications of gamma delta T cells with multivalent immunity. Front. Immunol. 5:636. doi: 10.3389/fimmu.2014.00636
Received: 29 September 2014; Accepted: 28 November 2014;
Published online: 11 December 2014.
Edited by:
Julie Dechanet-Merville, Centre National de la Recherche Scientifique (CNRS), FranceReviewed by:
Sid P. Kerkar, National Institutes of Health, USAMary Poupot, Institut National de la Santé et de la Recherche Médicale, France
Copyright: © 2014 Deniger, Moyes and Cooper. This is an open-access article distributed under the terms of the Creative Commons Attribution License (CC BY). The use, distribution or reproduction in other forums is permitted, provided the original author(s) or licensor are credited and that the original publication in this journal is cited, in accordance with accepted academic practice. No use, distribution or reproduction is permitted which does not comply with these terms.
*Correspondence: Laurence J. N. Cooper, Unit 907, Division of Pediatrics, University of Texas MD Anderson Cancer Center, 1515 Holcombe Blvd., Houston, TX 77030, USA e-mail:bGpuY29vcGVyQG1kYW5kZXJzb24ub3Jn