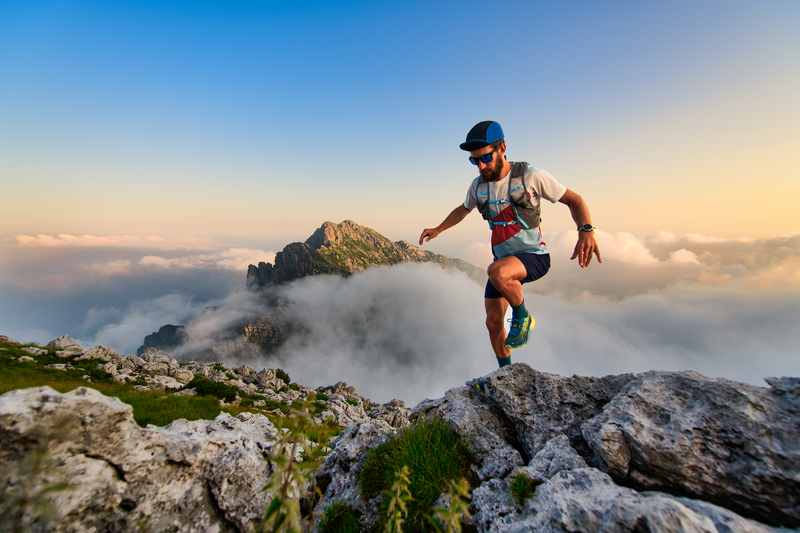
94% of researchers rate our articles as excellent or good
Learn more about the work of our research integrity team to safeguard the quality of each article we publish.
Find out more
ORIGINAL RESEARCH article
Front. Immunol. , 14 July 2014
Sec. Microbial Immunology
Volume 5 - 2014 | https://doi.org/10.3389/fimmu.2014.00324
This article is part of the Research Topic Causes for increased susceptibility to Mycobacterium tuberculosis-A close view of the immune system View all 8 articles
Human primary dendritic cells (DCs) are heterogeneous by phenotype, function, and tissue localization and distinct from inflammatory monocyte-derived DCs. Current information regarding the susceptibility and functional role of primary human DC subsets to Mycobacterium tuberculosis (Mtb) infection is limited. Here, we dissect the response of different primary DC subsets to Mtb infection. Myeloid CD11c+ cells and pDCs (C-type lectin 4C+ cells) were located in human lymph nodes (LNs) of tuberculosis (TB) patients by histochemistry. Rare CD141hi DCs (C-type lectin 9A+ cells) were also identified. Infection with live Mtb revealed a higher responsiveness of myeloid CD1c+ DCs compared to CD141hi DCs and pDCs. CD1c+ DCs produced interleukin (IL)-6, tumor necrosis factor α, and IL-1β but not IL-12p70, a cytokine important for Th1 activation and host defenses against Mtb. Yet, CD1c+ DCs were able to activate autologous naïve CD4+ T cells. By combining cell purification with fluorescence-activated cell sorting and gene expression profiling on rare cell populations, we detected in responding CD4+ T cells, genes related to effector-cytolytic functions and transcription factors associated with Th1, Th17, and Treg polarization, suggesting multifunctional properties in our experimental conditions. Finally, immunohistologic analyses revealed contact between CD11c+ cells and pDCs in LNs of TB patients and in vitro data suggest that cooperation between Mtb-infected CD1c+ DCs and pDCs favors stimulation of CD4+ T cells.
Tuberculosis (TB) is caused by the intracellular bacterial pathogen Mycobacterium tuberculosis (Mtb). Phagocytic cells such as macrophages engulf bacteria entering the lung and initiate a first line of defense, which controls Mtb growth and recruits pro-inflammatory cells (1). Activation of the adaptive immune responses, notably T cells, occurs only at later time points after infection and involves the migration of infected dendritic cells (DCs) to the draining lymph nodes (LNs) (2–4). Here, DCs prime naïve T cells leading to expansion and polarization of effector T cells and generation of memory T cells. Migration of DCs to LNs involves interleukin (IL)-12p40-dependent mechanisms and upregulation of CCR7 (5). Moreover, the bacterial antigens presented in the LNs need to reach a critical abundance to efficiently activate a specific CD4+ T cell response (4). As a corollary, inhibition of DC maturation and trafficking and, consequently, suboptimal antigen presentation, likely contribute to delayed CD4+ T cell responses.
Migratory and resident DCs are categorized in two main groups: myeloid (m)DCs (CD11c+) and plasmacytoid (p)DCs. Murine mDCs (CD11c+) comprise CD11b+ and CD11b– DCs (6). After aerosol infection with Mtb, murine CD11b+ DCs are the major subset harboring Mtb and trafficking from the lung to the mesenteric lymph nodes (MLNs) (7). However, interferon (IFN)-γ production of CD4+ T cells in the MLNs seems to be mediated by non-infected CD11blow/– cells rather than by CD11b+ DCs. Thus, so far, two unresolved questions remain to be answered: which DC subsets initiate the activation of naïve T cells in LNs and which type of T helper populations are primed in response to Mtb.
Functional specialization of DC subsets is determined by intrinsic properties such as pattern recognition receptors and external factors such as tissue localization, cytokine environment, and type of pathogen encountered. For example, in the lung, murine CD11b+(CD24+) DCs and the corresponding human homolog CD1c+ DCs, activate IL-17+ CD4+ T cells in response to Aspergillus fumigatus (8). On the contrary, human blood CD1c+ DCs acquire regulatory functions when stimulated with Escherichia coli (9). We showed that CD1c+ DCs produce pro-inflammatory cytokines in response to the TB vaccine Bacille Calmette–Guérin (BCG) and low levels of IL-10 (10).
Murine splenic CD11b– CD8α+ DCs and non-lymphoid tissue CD11b– CD103+ DCs are highly related to human CD141hi DCs (11). CD11b– CD8α+ DCs are susceptible to Listeria monocytogenes infection and their depletion enhances host defense (12, 13). CD141hi DCs are well characterized for cross-presentation and for their ability to present necrotic antigens by mean of C-type lectin 9A (CLEC9A) (14–16). This complex network of DC subsets emphasizes differential susceptibility of distinct DC populations to pathogens and pathogen-associated molecular patterns.
In addition, distinct DC subsets may communicate during infection to promote or inhibit T cell responses (17). Cross-talk of mDCs and pDCs promotes cytotoxic T cell activation and IL-12 production in response to herpes simplex virus or TLR9 ligand (18–20). Besides the crucial role of pDCs in viral infection (21), we have shown that pDCs are activated by BCG-infected CD1c+ DCs and enhance BCG-specific CD8+ T cell responses independently of TLR9 and type I IFN. Thus, cooperation of mDCs and pDCs occurs during bacterial infection.
We embarked on the characterization of human DC responses to Mtb infection by visualizing DC subsets in LNs of TB patients. We determined the responsiveness of CD1c+ DCs, CD141hi DCs, and pDCs to live Mtb infection and their ability to stimulate autologous naive CD4+ T cells.
For FACS sorting (FACS Aria II, BD Biosciences) and acquisition (FACSCanto II equipped with FACSDIVA Software, BD Biosciences) the following anti-human antibodies were used: BDCA-1-FITC, BDCA-4-PE, and BDCA-3-APC from Miltenyi Biotec; CD3 Alexa Fluor 700 (UCHT1), CD4 Pacific Blue (RPA-T4), CD11c Alexa Fluor 700 (B-ly6), CD14 Pacific Blue (M5E2), CD56 Pe-Cy7 (B159), and Annexin V APC from BD Biosciences; CD25 Alexa Fluor 488 (VT-072), CD19 Pe-Cy5 (HIB19), CD20 PerCP (2H7), CD45RA Alexa Fluor 700 (HI100), and HLA-DR BV 570 (L243), from Biolegend; CD123 eFluor450 (6H6) and Propidium Iodide (PI) from eBioscience; and CD40 PE (82111) and CD127 PE (40131) from R&D Systems. Cultures were performed using complete RPMI media 1640 (Life Technologies) in the presence of 5% human serum (Lonza) without antibiotics.
Experiments with donor material were approved by the Ethics Committee of the Charité University Hospital (Charité Universitätsmedizin) in Berlin, Germany [EA2/064/14]. Donations received from blood bank donors were anonymized. DCs were isolated from buffy coats obtained from the German Red Cross blood bank (DRK-Blutspendedienst Ost) by Ficoll–Hypaque gradient (Biochrom), as described previously (10). Briefly, DCs were enriched from PBMCs by MACS separation using BDCA-1-FITC, BDCA-4-PE, and BDCA-3-APC followed by incubation with FITC-, PE-, and APC-beads. The positive fraction was stained with lineage markers (lin) (α-CD3, α-CD19, α-CD20, α-CD56, α-CD14) and α-HLA-DR and DCs were further purified by cell sorting according to the following staining: pDCs (lin−HLA-DR+BDCA-4+BDCA-1−BDCA-3−), CD1c+ (=BDCA-1+) mDCs (lin−HLA-DR+BDCA-4−BDCA-1+BDCA-3–), and CD141hi (=BDCA-3+) mDCs (lin−HLA-DR+BDCA-4−BDCA-1−BDCA-3+). Naïve CD4+ T (CD3+) cells were enriched using MACS beads (Miltenyi Biotec) followed by sorting according to naïve (CD45RA+CD127highCD25–) and T cell markers. Sorted cells with purity higher than 98% were used for experiments.
A total of 25,000 CD1c+ DCs, CD141hi DCs, or pDCs were infected using virulent Mtb strain (H37Rv) expressing GFP at an MOI of five. After 2 h, extracellular bacteria were removed by extensive washing and cells were cultured for another 16 h unless otherwise indicated. In co-culture conditions, unstimulated pDCs were added to Mtb-infected mDC cultures, 2 h post-infection, at a 1:1 ratio and supernatants were harvested after 14 h. In some conditions, CD1c+ DCs were stimulated with 100 ng/mL Lipopolysaccharide (LPS; Sigma-Aldrich) and 2.5 μg/mL Resiquimod (R848; Invitrogen) for 16 h.
To study naïve CD4+ T cell proliferation, 250,000 naïve CD4 T cells were stimulated at a 1:10 ratio (mDC subset:T cell) with autologous DCs previously infected for 16 h with Mtb. Proliferation was visualized after 7 days by carboxyfluorescein succinimidyl ester (CFSE) dilution.
After Mtb infection or TLR stimulation, DCs were harvested and labeled with α-hCD123 and α-hCD11c antibodies that allow distinction between pDCs (CD123high/lowCD11c–) and CD1c+ DCs (CD123low/–CD11c+) in co-culture conditions. α-hCD141 was used to label CD141hi DCs. Apoptotic cells were detected as Annexin V+ PI– (early apoptotic cells) or Annexin V+ PI+ (late apoptotic cells). Necrotic cells were detected as PI+ cells. Mean fluorescence intensity of HLA-DR, CD40, and CD83 was showed after subtraction from baseline values of unstimulated conditions. Naïve CD4+ T cells were labeled with CFSE according to manufacturer’s instructions (Molecular Probes) and proliferation analyzed after 7 days of culture with autologous DCs. Proliferating cells were gated as CD4+CD3+CFSElow cells. Analysis was performed using FlowJo (TreeStar).
Unless otherwise indicated, ELISA was performed at 16 h post-stimulation (2 h infection and additional 14 h incubation). IFN-α was measured by VeriKine Human Interferon Alpha ELISA Kit (PBL Interferon Source), granzyme B (GrB) by PeliKine Compact Human Granzyme B Elisa Kit (Sanquin), IL-1β and IL-12p70 by R&D Systems, tumor necrosis factor (TNF)-α, and IL-6 by Ready-SET-Go kit (eBioscience).
Gene expression levels were analyzed simultaneously using the 96.96 Dynamic Array Integrated Fluidic Circuits (IFCs) from Fluidigm. After 7 days of proliferation, CD4+CD3+CFSElow cells were sorted in triplicates of 100 cells and collected in a 96-well PCR plate (Eppendorf). The genes of interest were pre-amplified using a mix of TaqMan Gene expression Assays (Applied Biosystems). The cDNA and the single TaqMan assays were then loaded in a microfluidic chip using 96.96 IFC Controller HX according to manufacturer’s protocol. Quantitative PCR was performed with the BioMark™ HD System (Fluidigm). Data were exported with the Real-time PCR Analysis Software (Fluidigm) and analyzed with Microsoft Office Excel. ΔCt was referred to GAPDH (NM_001256799.1) transcript. The threshold for ΔCt calculation was set at Ct < 30 and Ct values >30 were excluded. To compare data from different donors and chips, fold change in transcripts (2–[(ΔCt)reference−ΔCt(value)]) was calculated relative to ΔCt of CD4+CD3+CFSElow cells stimulated with Mtb-infected CD1c+ DCs (ΔCtreference). The following transcripts were analyzed: GZMB (NM_004131.4), PRF1 (NM_000594.3), IRF4 (NM_001195286), CXCR3 (NM_001504), CCR7 (NM_001838), TBX21 (NM_013351), RORC (NM_001001523), GATA3 (NM_001 002295), and FOXP3 (NM_001114377).
Formalin-fixed paraffin-embedded tissue blocks of LN specimens from TB+ HIV– patients with pulmonary TB were obtained from the Lazzaro Spallanzani National Institute for Infectious Diseases (INMI), Translational Research Unit, Department of Epidemiology and Preclinical Research, Rome, Italy. Specimens belong to archived autopsies of patients with pulmonary TB and were Mtb culture-positive or had positive stains for acid-fast bacilli. In addition, LN tissue slides from patients with pulmonary TB were obtained from Bio-Cat GmbH, Heidelberg, Germany.
Lymph node tissues from subjects without TB but undergoing cancer screening (Bio-Cat GmbH, Heidelberg, Germany) were used as comparison group. Paraffin-embedded sections of 5-μm thickness were deparaffinized and rehydrated. Epitopes were heat-retrieved in a pressure cooker with Target Retrieval Solution, High pH (Dako), and tissue sections were blocked with 1% horse serum (PAA technologies), 5% donkey serum (PAA technologies), 5% sheep serum, and 1% bovine serum albumin (Sigma–Aldrich) in PBS with 0.05% Tween-20 (PBS-T) at room temperature for 45 min. Goat polyclonal antibody (Ab) against CLEC4C, sheep polyclonal Ab against CLEC9A (R&D Systems), rabbit polyclonal Ab against CD3 (Dako), CD20 and CD11c (Abcam), mouse monoclonal Ab against GrB (Monosan), were applied to tissue sections at 4°C, overnight. After three washes with PBS-T, sections were incubated with NL™ 557 donkey anti-goat or anti-sheep Ab (R&D Systems) at room temperature for 45 min, washed and then incubated with Alexa Fluor 647 goat anti-mouse and Alexa Fluor 488 goat anti-rabbit (Invitrogen) at room temperature for 45 min. After PBS-T wash, nuclei were stained with DAPI (Sigma) and tissue sections were mounted in Confocal Matrix (Imm Tech). Images were captured by a Leica DMR epifluorescence microscope equipped with Nikon Digital DX M1200F.
We performed statistical analysis using Graph Pad Prism 5 Software. Group data were tested for normal distribution (Shapiro–Wilk normality test). Wilcoxon signed-rank or Mann–Whitney tests were used for paired or unpaired observations, respectively. Analysis of Variance (ANOVA) was used to compare more than two sets of data. Lines and error bars represent mean ± SD. For the increase of GrB and IFN-α concentrations, significance of the interaction between treatment groups and time points was tested using permutation test from the R package lmPerm version 1.1.
We analyzed the distribution of DC populations in reactive LNs of individuals without TB (no-TB) and TB patients. In both groups, abundant pDCs (identified by CLEC4C staining) were detected in close vicinity to CD3+ T cells (Figure 1A) but were rare in B cell areas (Figure 1B). CD11c+ cells, including mDCs, were regularly distributed in LNs of individuals without TB while some clusters of cells were detected in TB patients (Figure 1C). Among mDCs, CD141hi DCs (identified by CLEC9A staining) were found in LNs of no-TB individuals within CD3+ and CD20+ cell areas (Figures 1D,E, left) but were rare in LNs of TB patients (Figures 1D,E, right). At higher magnitude single CLEC9A cells were clearly distinguishable and located in contact with CD3+ or CD20+ cells in TB patients (Figures 2A,B).
Figure 1. Immunostaining of DCs in LNs from TB patients and individuals without TB. (A–C) Immunostaining of pDCs (CLEC4C, red) and (A) T cells (CD3+, green); (B) B cells (CD20+, green); (C) CD11c+ cells (green). DAPI (blue) indicates cell nuclei. (D,E) Immunostaining of CD141hi DCs (CLEC9A, red); and (D) T cells (CD3+, green); or (E) B cells (CD20+, green). DAPI (blue) indicates cell nuclei. Magnification 10× or 20×. Left: reactive LNs from individuals without TB (no-TB LNs); right: LNs from TB patients (TB LNs). One representative experiment out of three controls (no-TB) and four TB cases shown.
Figure 2. Identification of CD141hi DCs in LNs of TB patients. Immunostaining of CD141hi DCs (CLEC9A) with (A) T cells (CD3+, green) and (B) B cells (CD20+, green). DAPI (blue) indicates cell nuclei. Magnification 40×. One representative experiment out of three TB cases shown.
We previously described that pDCs help CD1c+ DCs in the control of BCG infection and induction of mycobacteria-specific CD8 T cell response(s). In this context, pDCs produced GrB in high abundance but not type I IFN (10). We determined whether Mtb induces a similar cross-talk between pDCs and CD1c+ DCs. In contrast to BCG stimulation (10), low concentrations of IFN-α were detected in culture of pDCs with Mtb-infected CD1c+ DCs (MOI 5, 48 h post-infection) (Figure 3A, blue diamonds, p < 0.05 compared to Mtb-infected CD1c+ DCs monocultures). However, GrB production remained predominant (Figure 3A, red circles, p < 0.001 compare to Mtb-infected CD1c+ DCs monocultures) indicating that pDC response to mycobacterial infection is skewed toward GrB production and regulated by the state of activation of infected mDCs rather than by the type of microorganism (pathogenic Mtb vs. attenuated BCG) (10). Similarly, ex vivo co-staining of LNs of TB patients revealed discrete contact areas between CD11c+ DCs and pDCs (Figure 3B), as well as between CD3+ cells and pDCs (Figure 3C) and the presence of GrB+ pDCs (Figure 3C). This spatial distribution and functional capacity of pDCs in LNs points to a cross-talk between pDCs and mDCs during active TB.
Figure 3. Production of IFN-α and GrB after Mtb infection. (A) ELISA of IFN-α (blue diamonds) and granzyme B (GrB) (red circles), at 4, 24, and 48 h after infection with Mtb. Only results from Mtb-infected CD1+–pDC cultures are shown (no detectable concentration of either GrB or IFN-α was found in Mtb-infected CD1c+ DC monocultures). Three donors in one experiment are shown (permutation test). Immunostaining of (B) contact area between CD11c+ cells (blue) and pDCs (CLEC4C, red) and (C) GrB+-producing pDCs (in red) or GrB+-producing CD3+ (in blue). GrB is stained in green. Arrows indicate contact area (B) or CLEC4C–GrB co-staining (C). Magnification 100×. One representative experiment out of three TB cases shown.
To gain deeper insights into DC subset interplay during Mtb infection, CD1c+ DCs, CD141hi DCs, and pDCs were isolated from peripheral blood of healthy donors. The subsets were cultured with virulent Mtb-expressing GFP and analyzed for their ability to prime naïve CD4+ T cells. Autologous naïve CD4+ T cells proliferated in response to infected CD1c+ DCs but not to CD141hi DCs and pDCs (Figures 4A,B). These differences could be due to lower ability of CD141hi DCs and pDCs to phagocytose whole bacilli (Figure 4C) and consequently, to present antigens to T cells.
Figure 4. CD4+ T cell activation by CD1c+ DCs. (A) and (B) Naïve CD4 T cells were labeled with CFSE and proliferation was measured after 7 days of culture in the presence of autologous CD1c+ DCs ± pDCs (CD1c), CD141hi DCs ± pDCs (CD141hi), or pDCs alone (pDC) previously stimulated with live Mtb for 16 h. DC:T cell ratio was 1:10. (A) One representative flow cytometric CD4 T cell staining shown; lymphocytes were gated according to morphological parameters and doublets excluded. Proliferating CD4+ T cells were gated as CD4+CD3+CFSElow cells. (B) Mean ± SD of proliferating CD4+ T cells in response to CD1c+ DCs ± pDCs or CD141hi DCs ± pDCs of at least five donors in two independent experiments. (C) One representative gating strategy of Mtb-infected CD1c+ DCs (top left), CD141hi DCs (top middle), and pDCs (top right) and mean ± SD of Mtb-infected cells of at least eight donors in four independent experiments (bottom). Cells were infected at MOI 5 with Mtb-expressing GFP and stained for specific DC marker after 16 h. Infected cells were measured as percentage of GFP+ cells. Kruskal–Wallis ANOVA; *p < 0.05, **p < 0.01, ***p < 0.001.
Proliferation of autologous naïve CD4+ T cells in response to Mtb-infected CD1c+ DCs was enhanced by the presence of pDCs (Figures 4A,B). A similar trend was observed when pDCs were cultured with Mtb-infected CD141hi DCs although naive CD4+ T cells were more responsive to infected CD1c+ DCs (Figures 4A,B).
We then focused on the CD1c+ DC and pDC interaction. Apoptosis of CD1c+ DCs was not affected by pDCs excluding that pDCs killed CD1c+ DCs through type I IFN or GrB-mediated mechanisms (22, 23) (Figures 5A,B). Similarly, pDCs did not affect the number of Mtb-infected CD1c+ DCs (Figure 5C). Contact with Mtb-infected CD1c+ DCs elicited higher expression of HLA-DR and CD40 but not of CD83 on the surface of pDCs. (Figure 5D, bottom and data not shown). After infection, CD1c+ DCs expressed HLA-DR, CD40, and CD83 (Figure 5D, top) and produced IL-6, TNF-α, and IL-1β but not IL-23, TGF-β, or IL-12p70 (Figures 5E,F and data not shown). We excluded a functional defect in CD1c+ DCs since IL-12p70 was produced in the presence of LPS (TLR4 ligand) and R848 (TLR7/8 ligands) (24) and partially induced in infected cells triggered with R848 (Figure 5F). These data identify CD1c+ DCs as the most responsive DC subset to Mtb infection but also highlight their dependency on additional stimuli for optimal IL-12 production. Moreover the data reveal that the presence of pDCs favors CD4+ T cell expansion.
Figure 5. Survival, infection and cytokine production by CD1c+ DCs is not affected by pDCs. (A) One representative gating strategy used to visualize DC subsets and apoptotic–necrotic CD1c+ DCs. After exclusion of doublets, pDCs were gated as CD123high CD11c– cells and CD1c+ DCs as CD123low/–CD11c+ cells. CD1c+ DCs were then gated as early apoptotic cells (Annexin A+ PI–), late apoptotic cells (Annexin A+ PI+), and necrotic cells (PI+). (B) Relative number of apoptotic and necrotic CD1c+ DCs at different time points after Mtb infection in the presence (Mtb + pDCs) or absence of pDCs (Mtb). The number was obtained by normalizing the percentages of apoptotic–necrotic CD1c+ DCs after infection to the percentages of the respective controls (CD1c+ DCs ± pDCs in the absence of Mtb). (C) Percentage of Mtb-infected (GFP+)-CD1c+ DCs in the presence or absence of pDCs. (D) Mean fluorescence intensity of HLA-DR, CD40, and CD83 in Mtb-infected mono- (white bars) or co-cultures (black bars) gated on CD1c+ DCs (top) or pDCs (bottom panel) (Mann–Whitney test). (E) Cytokine production by pDCs, CD1c+ DCs, or CD1c-pDC cultures 16 h post-infection. (F) Production of IL-12p70 by CD1c+ DC or CD1c+ DC-pDC cultures 16 h post-infection in the absence (Mtb) or in the presence (Mtb/R848) of TLR7/8 ligands or after stimulation with TLR4 and TLR7/8 ligands (LPS/R848). Control (Cntr) indicated unstimulated cells. Data are obtained from six donors in two independent experiments. One-way ANOVA; *p < 0.05, ***p < 0.001.
To characterize the phenotype of responding CD4+ T cells, we performed gene expression profiling of sorted CD3+CD4+ CFSElow T cells by using a protocol that allows gene expression analysis of rare populations (100 cells). CD4+ T cell expansion was associated with upregulation of CXCR3 and downregulation of CCR7 gene expression (Figure 6A) consistent with a phenotype of activated T cells. In addition, proliferating CD4+ T cells upregulated the expression of IRF4, GrB (GZMB), and perforin (PRF1) suggesting that they express cytolytic functions (Figure 6B). Interestingly, gene expression profiling of CFSElow CD4+ T cells revealed upregulation of Tbet (TBX21), RORγt (RORC), and FOXP3 but not GATA3 transcripts (Figure 6C). The presence of pDCs did not modulate the gene expression profile of these transcription factors (Figures 6A–C). We conclude that effector CD4+ T cells activated by Mtb-infected CD1c+ DCs are heterogeneous and potentially polarized toward Th1, Th17, Treg cells but not Th2 cells.
Figure 6. Gene expression profile of CD4+ T cells. Fold changes of (A) CXCR3, CCR7, (B) IRF4, GrB (GZMB), perforin (PRF1), (C) Tbet (TBX21), RORγT (RORC), GATA3, and FOXP3 transcripts, measured in CD4+CD3+ CFSElow cells, in the presence (Mtb + pDC) or absence of pDCs (Mtb) and unstimulated CD4+CD3+ cells (−). The threshold for ΔCt calculation was set at Ct < 30 and Ct values >30 were excluded. ΔCt was calculated to GAPDH. To compare data from different donors and chips, fold change in transcripts (2−[(ΔCt)reference−ΔCt(value)]) was calculated relative to ΔCt of CD4+CD3+CFSElow cells stimulated with Mtb-infected CD1c+ DCs (ΔCtreference) since most of the transcripts were undetectable in unstimulated conditions (−). In unstimulated conditions (−), IRF4 and Tbet transcripts were detectable only in one and two samples out of six, respectively. Mean ±95% confidential interval of at least five donors in two independent experiments shown. Wilcoxon signed-rank test; *p < 0.05; n.d., non-detectable.
Dendritic cells are important players in the early phase of Mtb infection mostly by modulating the activation of T lymphocytes (25). The heterogeneity of DC subsets indicates a division of labor during infection that could impact on the quality of the T cell response. The role of primary DC subsets in Mtb infection, particularly in human TB, is incompletely understood. Here, we identify both mDCs and pDCs in LNs of TB patients. Few CLEC9A+ cells, the marker used to identify CD141hi DCs (15, 26, 27), are also found in T and B cell areas.
We demonstrate that CD1c+ DCs engulf and respond to live Mtb more efficiently than CD141hi DCs and pDCs. After infection, they upregulated HLA-DR and CD40, which are required for CD4+ T cell priming (28). CD1c+ DCs produced pro-inflammatory cytokines, but not IL-12p70. Optimal production of IL-12 by CD1c+ DCs has been found to depend on TLR 4/7/8 triggering and to be promoted by IFN-γ or CD40L (24, 29, 30). Moreover, macrophages were found to require a prime signal by IFN-γ to produce IL-12 in response of Mtb (31). Thus, it is likely that the lack of IL-12 is due to absence of adequate stimuli. It is known that Mtb interferes with macrophage and DC activation by modulating cytokine production and MHC class II expression (32–34). Recognition of mannosylated lipoarabinomannan (ManLam) by DC-SIGN also inhibits monocyte-derived (mo)DC functions and induces IL-10 (35, 36). Primary human DCs do not express DC-SIGN or mannose receptor (37, 38) and whether Mtb actively inhibits IL-12 signaling in primary CD1c+ DCs needs further investigation.
The inhibition of moDC functions is not absolute since moDCs still produce cytokines and induce Th1 cells (39, 40). Similarly, we found that CD1c+ DCs are still able to stimulate naïve CD4+ T cells to become effector T cells. Furthermore, bacterial numbers could affect functions of DCs, and consequently T cell activation: low numbers may delay T cell responses (4, 41), whereas high numbers of bacilli could inhibit DC function or induce T cell exhaustion (42). To understand the physiological state of activation of CD1c+ DCs and their antigen presentation capacity, a closer look at these cells in LNs or lungs of Mtb-infected individuals is essential albeit limited by scarce availability of human tissues.
We found that CD141hi DCs fail to directly activate naïve CD4+ T cells. However, mice lacking essential transcription factors for CD8α+ DC and tissue CD11b−CD103+ DC development are susceptible to Mtb infection (43, 44). Although these transcription factors influence the functions of other cell types, these studies suggest that CD8α+ and CD11b−CD103+ DCs are indeed involved in protection against TB. Since localization and cytokine environment affect DC function, it is likely that immature blood CD141hi DCs respond less efficiently than LN-resident CD141hi DCs to Mtb infection in the absence of additional stimuli. In fact, when properly stimulated with TLR ligands and cytokines, CD141hi DCs produce IFN-β, IFN-λ, and IL-12 (15, 24, 45) and can therefore participate in optimal Th induction. In addition, CD141hi DCs are potent cross-presenting cells (11, 15) and they may play a role in T cell activation by presenting antigens from bystander-infected cells rather than by direct antigen presentation. Intriguingly, priming of CD4+ T cells in LNs of infected mice has been found to be mediated by non-infected CD11b− DCs rather than Mtb-infected CD11b+ DCs (7). Whether this subset of CD11b− DCs also comprise murine CD103+ DCs still needs to be addressed. In human TB a deeper analysis of CD141hi DCs from LNs will be more informative in determining the relevance of CD141hi and whether they cooperate with Mtb-infected myeloid DCs to activate a T cell response.
We show that contact between CD11c+ cells and pDCs occurs in LNs of TB patients. Furthermore, by using the specific pDC marker CLEC4C we identified the presence of GrB–pDCs, thus supporting previous data (46). GrB production in response to BCG was associated with enhanced IL-1β release by CD1c+ DCs and reduced bacterial growth (10). This phenotype was not observed in response to Mtb. Mtb activates type I IFN in macrophages and moDCs (47), and type I IFN has been shown to inhibit IL-1β production (48). Despite the low levels of IFN-α detected, it is possible that Mtb triggered the type I IFN pathway thereby counteracting the effect of pDCs on IL-1β release. Whereas GrB–pDCs alone acquire suppressive functions (49) we demonstrate here that they did not kill CD1c+ DCs; rather they strongly supported CD4+ T cell proliferation.
The presence of pDCs apparently did not affect activation of responding CD4+ T cells – at least at the gene transcript level analyzed here. Activated CD4+ T cells expressed transcripts of cytotoxic effector molecules such as perforin and GrB. Transcription factors for Th1, Th17, and Treg, but not Th2, cells were also upregulated. It has been shown that a large proportion of memory T cells in latent Mtb infection express a unique CXCR3+CCR6+ Th1 phenotype (50) but it remains unclear whether they are derived from Th1 or Th17 lineages. While we found that interplay of infected CD1c+ DCs and pDCs induced CD4+ T cell proliferation, further studies on activated CD4+ T cells and antigen specificity will determine their features and if they differentiate into memory T cells.
Taken together, these data suggest that communication between Mtb-susceptible and resistant DC subsets, plays an essential role in host defense to TB, thus calling for deeper investigations. Consequently, we propose that, while CD1c+ DCs are the more responsive DC subset to Mtb infection, pDCs help Mtb-infected CD1c+ DCs by intensifying stimulation during priming of naïve CD4+ T cells.
Laura Lozza designed and performed research, analyzed data, and wrote the paper. Maura Farinacci and Marina Bechtle contributed to experimental design, performed experiments and analysis, and revised the manuscript. Manuela Stäber and Ulrike Zedler performed experiments and analyzed data. Andrea Baiocchini and Franca del Nonno contribute to data acquisition, and revised the manuscript. Stefan H. E. Kaufmann supervised the study, contributed to experimental design, and writing of the paper.
The authors declare that the research was conducted in the absence of any commercial or financial relationships that could be construed as a potential conflict of interest.
The authors would like to thank Jenny Kirsch and Toralf Kaiser from the Flow Cytometry Core Facility, January Weiner 3rd for helping with statistical analysis and Mary Louise Grossman for excellent editorial assistance. This work was supported in part by the European Union’s Seventh Framework Programme (EU FP7) project “NEWTBVAC” (Health-F3-2009-241745).
1. Guilliams M, Lambrecht BN, Hammad H. Division of labor between lung dendritic cells and macrophages in the defense against pulmonary infections. Mucosal Immunol (2013) 6:464–73. doi: 10.1038/mi.2013.14
2. Gallegos AM, Pamer EG, Glickman MS. Delayed protection by ESAT-6-specific effector CD4+ T cells after airborne M. tuberculosis infection. J Exp Med (2008) 205:2359–68. doi:10.1084/jem.20080353
3. Reiley WW, Calayag MD, Wittmer ST, Huntington JL, Pearl JE, Fountain JJ, et al. ESAT-6-specific CD4 T cell responses to aerosol Mycobacterium tuberculosis infection are initiated in the mediastinal lymph nodes. Proc Natl Acad Sci U S A (2008) 105:10961–6. doi:10.1073/pnas.0801496105
4. Wolf AJ, Desvignes L, Linas B, Banaiee N, Tamura T, Takatsu K, et al. Initiation of the adaptive immune response to Mycobacterium tuberculosis depends on antigen production in the local lymph node, not the lungs. J Exp Med (2008) 205:105–15. doi:10.1084/jem.20071367
5. Khader SA, Partida-Sanchez S, Bell G, Jelley-Gibbs DM, Swain S, Pearl JE, et al. Interleukin 12p40 is required for dendritic cell migration and T cell priming after Mycobacterium tuberculosis infection. J Exp Med (2006) 203:1805–15. doi:10.1084/jem.20052545
6. Haniffa M, Collin M, Ginhoux F. Ontogeny and functional specialization of dendritic cells in human and mouse. Adv Immunol (2013) 120:1–49. doi:10.1016/B978-0-12-417028-5.00001-6
7. Wolf AJ, Linas B, Trevejo-Nunez GJ, Kincaid E, Tamura T, Takatsu K, et al. Mycobacterium tuberculosis infects dendritic cells with high frequency and impairs their function in vivo. J Immunol (2007) 179:2509–19. doi:10.4049/jimmunol.179.4.2509
8. Schlitzer A, Mcgovern N, Teo P, Zelante T, Atarashi K, Low D, et al. IRF4 transcription factor-dependent CD11b+ dendritic cells in human and mouse control mucosal IL-17 cytokine responses. Immunity (2013) 38:970–83. doi:10.1016/j.immuni.2013.04.011
9. Kassianos AJ, Hardy MY, Ju X, Vijayan D, Ding Y, Vulink AJ, et al. Human CD1c (BDCA-1)+ myeloid dendritic cells secrete IL-10 and display an immuno-regulatory phenotype and function in response to Escherichia coli. Eur J Immunol (2012) 42:1512–22. doi:10.1002/eji.201142098
10. Lozza L, Farinacci M, Fae K, Bechtle M, Staber M, Dorhoi A, et al. Crosstalk between human DC subsets promotes antibacterial activity and CD8+ T-cell stimulation in response to bacille Calmette-Guérin. Eur J Immunol (2014) 44:80–92. doi:10.1002/eji.201343797
11. Haniffa M, Shin A, Bigley V, Mcgovern N, Teo P, See P, et al. Human tissues contain CD141hi cross-presenting dendritic cells with functional homology to mouse CD103+ nonlymphoid dendritic cells. Immunity (2012) 37:60–73. doi:10.1016/j.immuni.2012.04.012
12. Neuenhahn M, Kerksiek KM, Nauerth M, Suhre MH, Schiemann M, Gebhardt FE, et al. CD8alpha+ dendritic cells are required for efficient entry of Listeria monocytogenes into the spleen. Immunity (2006) 25:619–30. doi:10.1016/j.immuni.2006.07.017
13. Autenrieth SE, Warnke P, Wabnitz GH, Lucero Estrada C, Pasquevich KA, Drechsler D, et al. Depletion of dendritic cells enhances innate anti-bacterial host defense through modulation of phagocyte homeostasis. PLoS Pathog (2012) 8:e1002552. doi:10.1371/journal.ppat.1002552
14. Sancho D, Joffre OP, Keller AM, Rogers NC, Martinez D, Hernanz-Falcon P, et al. Identification of a dendritic cell receptor that couples sensing of necrosis to immunity. Nature (2009) 458:899–903. doi:10.1038/nature07750
15. Jongbloed SL, Kassianos AJ, Mcdonald KJ, Clark GJ, Ju X, Angel CE, et al. Human CD141+ (BDCA-3)+ dendritic cells (DCs) represent a unique myeloid DC subset that cross-presents necrotic cell antigens. J Exp Med (2010) 207:1247–60. doi:10.1084/jem.20092140
16. Zhang JG, Czabotar PE, Policheni AN, Caminschi I, Wan SS, Kitsoulis S, et al. The dendritic cell receptor Clec9A binds damaged cells via exposed actin filaments. Immunity (2012) 36:646–57. doi:10.1016/j.immuni.2012.03.009
17. Pulendran B, Tang H, Denning TL. Division of labor, plasticity, and crosstalk between dendritic cell subsets. Curr Opin Immunol (2008) 20:61–7. doi:10.1016/j.coi.2007.10.009
18. Yoneyama H, Matsuno K, Toda E, Nishiwaki T, Matsuo N, Nakano A, et al. Plasmacytoid DCs help lymph node DCs to induce anti-HSV CTLs. J Exp Med (2005) 202:425–35. doi:10.1084/jem.20041961
19. Kuwajima S, Sato T, Ishida K, Tada H, Tezuka H, Ohteki T. Interleukin 15-dependent crosstalk between conventional and plasmacytoid dendritic cells is essential for CpG-induced immune activation. Nat Immunol (2006) 7:740–6. doi:10.1038/ni1348
20. Johnson S, Zhan Y, Sutherland RM, Mount AM, Bedoui S, Brady JL, et al. Selected toll-like receptor ligands and viruses promote helper-independent cytotoxic T cell priming by upregulating CD40L on dendritic cells. Immunity (2009) 30:218–27. doi:10.1016/j.immuni.2008.11.015
21. Cella M, Jarrossay D, Facchetti F, Alebardi O, Nakajima H, Lanzavecchia A, et al. Plasmacytoid monocytes migrate to inflamed lymph nodes and produce large amounts of type I interferon. Nat Med (1999) 5:919–23. doi:10.1038/11360
22. Kalb ML, Glaser A, Stary G, Koszik F, Stingl G. TRAIL(+) human plasmacytoid dendritic cells kill tumor cells in vitro: mechanisms of imiquimod- and IFN-alpha-mediated antitumor reactivity. J Immunol (2012) 188:1583–91. doi:10.4049/jimmunol.1102437
23. Tel J, Smits EL, Anguille S, Joshi RN, Figdor CG, De Vries IJ. Human plasmacytoid dendritic cells are equipped with antigen-presenting and tumoricidal capacities. Blood (2012) 120:3936–44. doi:10.1182/blood-2012-06-435941
24. Nizzoli G, Krietsch J, Weick A, Steinfelder S, Facciotti F, Gruarin P, et al. Human CD1c+ dendritic cells secrete high levels of IL-12 and potently prime cytotoxic T-cell responses. Blood (2013) 122:932–42. doi:10.1182/blood-2013-04-495424
25. Prendergast KA, Kirman JR. Dendritic cell subsets in mycobacterial infection: control of bacterial growth and T cell responses. Tuberculosis (Edinb) (2013) 93:115–22. doi:10.1016/j.tube.2012.10.008
26. Caminschi I, Proietto AI, Ahmet F, Kitsoulis S, Shin Teh J, Lo JC, et al. The dendritic cell subtype-restricted C-type lectin Clec9A is a target for vaccine enhancement. Blood (2008) 112:3264–73. doi:10.1182/blood-2008-05-155176
27. Huysamen C, Willment JA, Dennehy KM, Brown GD. CLEC9A is a novel activation C-type lectin-like receptor expressed on BDCA3+ dendritic cells and a subset of monocytes. J Biol Chem (2008) 283:16693–701. doi:10.1074/jbc.M709923200
28. Grewal IS, Xu J, Flavell RA. Impairment of antigen-specific T-cell priming in mice lacking CD40 ligand. Nature (1995) 378:617–20. doi:10.1038/378617a0
29. Napolitani G, Rinaldi A, Bertoni F, Sallusto F, Lanzavecchia A. Selected toll-like receptor agonist combinations synergistically trigger a T helper type 1-polarizing program in dendritic cells. Nat Immunol (2005) 6:769–76. doi:10.1038/ni1223
30. Gerosa F, Baldani-Guerra B, Lyakh LA, Batoni G, Esin S, Winkler-Pickett RT, et al. Differential regulation of interleukin 12 and interleukin 23 production in human dendritic cells. J Exp Med (2008) 205:1447–61. doi:10.1084/jem.20071450
31. Flesch IE, Hess JH, Huang S, Aguet M, Rothe J, Bluethmann H, et al. Early interleukin 12 production by macrophages in response to mycobacterial infection depends on interferon gamma and tumor necrosis factor alpha. J Exp Med (1995) 181:1615–21. doi:10.1084/jem.181.5.1615
32. Madan-Lala R, Sia JK, King R, Adekambi T, Monin L, Khader SA, et al. Mycobacterium tuberculosis impairs dendritic cell functions through the serine hydrolase hip1. J Immunol (2014) 192:4263–72. doi:10.4049/jimmunol.1303185
33. Koul A, Herget T, Klebl B, Ullrich A. Interplay between mycobacteria and host signalling pathways. Nat Rev Microbiol (2004) 2:189–202. doi:10.1038/nrmicro840
34. Harding CV, Boom WH. Regulation of antigen presentation by Mycobacterium tuberculosis: a role for toll-like receptors. Nat Rev Microbiol (2010) 8:296–307. doi:10.1038/nrmicro2321
35. Geijtenbeek TB, Van Vliet SJ, Koppel EA, Sanchez-Hernandez M, Vandenbroucke-Grauls CM, Appelmelk B, et al. Mycobacteria target DC-SIGN to suppress dendritic cell function. J Exp Med (2003) 197:7–17. doi:10.1084/jem.20021229
36. Tailleux L, Schwartz O, Herrmann JL, Pivert E, Jackson M, Amara A, et al. DC-SIGN is the major Mycobacterium tuberculosis receptor on human dendritic cells. J Exp Med (2003) 197:121–7. doi:10.1084/jem.20021468
37. Osugi Y, Vuckovic S, Hart DN. Myeloid blood CD11c(+) dendritic cells and monocyte-derived dendritic cells differ in their ability to stimulate T lymphocytes. Blood (2002) 100:2858–66. doi:10.1182/blood.V100.8.2858
38. Piccioli D, Tavarini S, Borgogni E, Steri V, Nuti S, Sammicheli C, et al. Functional specialization of human circulating CD16 and CD1c myeloid dendritic-cell subsets. Blood (2007) 109:5371–9. doi:10.1182/blood-2006-08-038422
39. Giacomini E, Iona E, Ferroni L, Miettinen M, Fattorini L, Orefici G, et al. Infection of human macrophages and dendritic cells with Mycobacterium tuberculosis induces a differential cytokine gene expression that modulates T cell response. J Immunol (2001) 166:7033–41. doi:10.4049/jimmunol.166.12.7033
40. Hickman SP, Chan J, Salgame P. Mycobacterium tuberculosis induces differential cytokine production from dendritic cells and macrophages with divergent effects on naive T cell polarization. J Immunol (2002) 168:4636–42. doi:10.4049/jimmunol.168.9.4636
41. Shaler CR, Horvath C, Lai R, Xing Z. Understanding delayed T-cell priming, lung recruitment, and airway luminal T-cell responses in host defense against pulmonary tuberculosis. Clin Dev Immunol (2012) 2012:628293. doi:10.1155/2012/628293
42. Day CL, Abrahams DA, Lerumo L, Janse Van Rensburg E, Stone L, O’rie T, et al. Functional capacity of Mycobacterium tuberculosis-specific T cell responses in humans is associated with mycobacterial load. J Immunol (2011) 187:2222–32. doi:10.4049/jimmunol.1101122
43. Marquis JF, Lacourse R, Ryan L, North RJ, Gros P. Disseminated and rapidly fatal tuberculosis in mice bearing a defective allele at IFN regulatory factor 8. J Immunol (2009) 182:3008–15. doi:10.4049/jimmunol.0800680
44. Tussiwand R, Lee WL, Murphy TL, Mashayekhi M, Wumesh KC, Albring JC, et al. Compensatory dendritic cell development mediated by BATF-IRF interactions. Nature (2012) 490:502–7. doi:10.1038/nature11531
45. Poulin LF, Salio M, Griessinger E, Anjos-Afonso F, Craciun L, Chen JL, et al. Characterization of human DNGR-1+ BDCA3+ leukocytes as putative equivalents of mouse CD8alpha+ dendritic cells. J Exp Med (2010) 207:1261–71. doi:10.1084/jem.20092618
46. Facchetti F, De Wolf-Peeters C, De Vos R, Van Den Oord JJ, Pulford KA, Desmet VJ. Plasmacytoid monocytes (so-called plasmacytoid T cells) in granulomatous lymphadenitis. Hum Pathol (1989) 20:588–93. doi:10.1016/0046-8177(89)90248-7
47. Remoli ME, Giacomini E, Lutfalla G, Dondi E, Orefici G, Battistini A, et al. Selective expression of type I IFN genes in human dendritic cells infected with Mycobacterium tuberculosis. J Immunol (2002) 169:366–74. doi:10.4049/jimmunol.169.1.366
48. Guarda G, Braun M, Staehli F, Tardivel A, Mattmann C, Forster I, et al. Type I interferon inhibits interleukin-1 production and inflammasome activation. Immunity (2011) 34:213–23. doi:10.1016/j.immuni.2011.02.006
49. Jahrsdorfer B, Vollmer A, Blackwell SE, Maier J, Sontheimer K, Beyer T, et al. Granzyme B produced by human plasmacytoid dendritic cells suppresses T-cell expansion. Blood (2010) 115:1156–65. doi:10.1182/blood-2009-07-235382
50. Lindestam Arlehamn CS, Gerasimova A, Mele F, Henderson R, Swann J, Greenbaum JA, et al. Memory T cells in latent Mycobacterium tuberculosis infection are directed against three antigenic islands and largely contained in a CXCR3+CCR6+ Th1 subset. PLoS Pathog (2013) 9:e1003130. doi:10.1371/journal.ppat.1003130
Keywords: Mycobacterium tuberculosis, human, CD1c+ DCs, CD141hi DCs, plasmacytoid DCs, CD4+ T cells
Citation: Lozza L, Farinacci M, Bechtle M, Stäber M, Zedler U, Baiocchini A, del Nonno F and Kaufmann SHE (2014) Communication between human dendritic cell subsets in tuberculosis: requirements for naive CD4+ T cell stimulation. Front. Immunol. 5:324. doi: 10.3389/fimmu.2014.00324
Received: 24 April 2014; Accepted: 27 June 2014;
Published online: 14 July 2014.
Edited by:
Vishwanath Venketaraman, Western University of Health Sciences, USAReviewed by:
Kristen J. Radford, University of Queensland, AustraliaCopyright: © 2014 Lozza, Farinacci, Bechtle, Stäber, Zedler, Baiocchini, del Nonno and Kaufmann. This is an open-access article distributed under the terms of the Creative Commons Attribution License (CC BY). The use, distribution or reproduction in other forums is permitted, provided the original author(s) or licensor are credited and that the original publication in this journal is cited, in accordance with accepted academic practice. No use, distribution or reproduction is permitted which does not comply with these terms.
*Correspondence: Laura Lozza and Stefan H. E. Kaufmann, Department of Immunology, Max Planck Institute for Infection Biology, Charitéplatz 1, Berlin D-10117, Germany e-mail:bG96emFAbXBpaWItYmVybGluLm1wZy5kZQ==;a2F1Zm1hbm5AbXBpaWItYmVybGluLm1wZy5kZQ==
†Present address: Maura Farinacci, Charité, Campus Virchow-Klinikum, Berlin, Germany
‡Maura Farinacci and Marina Bechtle have contributed equally to this work.
Disclaimer: All claims expressed in this article are solely those of the authors and do not necessarily represent those of their affiliated organizations, or those of the publisher, the editors and the reviewers. Any product that may be evaluated in this article or claim that may be made by its manufacturer is not guaranteed or endorsed by the publisher.
Research integrity at Frontiers
Learn more about the work of our research integrity team to safeguard the quality of each article we publish.