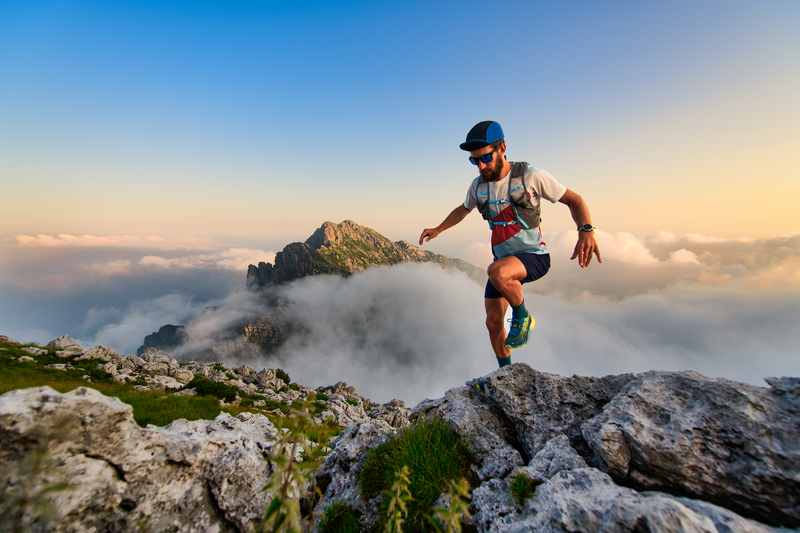
94% of researchers rate our articles as excellent or good
Learn more about the work of our research integrity team to safeguard the quality of each article we publish.
Find out more
REVIEW article
Front. Immunol. , 07 March 2012
Sec. Antigen Presenting Cell Biology
Volume 3 - 2012 | https://doi.org/10.3389/fimmu.2012.00037
This article is part of the Research Topic Molecular and cell-biological mechanisms of antigen cross-presentation View all 11 articles
Cross-presentation of endocytosed antigen as peptide/class I major histocompatibility complex complexes plays a central role in the elicitation of CD8+ T cell clones that mediate anti-viral and anti-tumor immune responses. While it has been clear that there are specific subsets of professional antigen presenting cells capable of antigen cross-presentation, identification of mechanisms involved is still ongoing. Especially amongst dendritic cells (DC), there are specialized subsets that are highly proficient at antigen cross-presentation. We here present a focused survey on the cell biological processes in the endosomal pathway that support antigen cross-presentation. This review highlights DC-intrinsic mechanisms that facilitate the cross-presentation of endocytosed antigen, including receptor-mediated uptake, maturation-induced endosomal sorting of membrane proteins, dynamic remodeling of endosomal structures and cell surface-directed endosomal trafficking. We will conclude with the description of pathogen-induced deviation of endosomal processing, and discuss how immune evasion strategies pertaining endosomal trafficking may preclude antigen cross-presentation.
Major histocompatibility complex (MHC) restriction of T lymphocytes was first reported by Doherty and Zinkernagel (1975). They provided experimental proof that T cells can only respond to peptide antigens when they are presented in complex with host-derived MHC molecules, existing in a class I and class II variant. CD8+ T cells can recognize peptide/class I MHC complexes, whereas CD4+ T cells can respond to peptide/class II MHC complexes. Soon thereafter, Bevan (1976) showed that the functional dichotomy of endogenous antigen presentation on class I MHC and exogenous antigen on class II MHC is not absolute. He demonstrated that minor histocompatibility antigens from transplanted cells (e.g., exogenous antigen) could prime cytotoxic CD8+ T cells in a host class I MHC-restricted manner and named this process cross-priming. More recent work showed that injected naive antigen-specific CD8+ T cells accumulate in the lymph nodes that drain tissues expressing a membrane-bound self-antigen in a class I MHC-dependent manner. CD8+ T cells can thus survey processed self-antigen delivered from non-lymphoid tissues, without leaving lymphoid organs (Kurts et al., 1996). In following years, the capability to present exogenous antigens via class I MHC was shown to be relevant for numerous cell-associated antigens in various settings, including viral, self, and tumor-associated antigens (Heath and Carbone, 2001). Antigen presentation that results in CD8+ T cell activation is now named cross-priming (Bevan, 1976), whereas T cell deletion or induction of anergy is called cross-tolerance (Albert et al., 2001; Bonifaz et al., 2002). Collectively the presentation of exogenous cell-associated antigens via class I MHC molecules to CD8+ T cells is called cross-presentation.
Under homeostatic conditions, cross-presentation of self-antigens harbors the risk of autoreactivity and is therefore strictly controlled. Under these circumstances, antigen cross-presentation is mostly confined to a specific subset of dendritic cells (DC), notably CD8α+ DC in mice (den Haan et al., 2000) and CD141+ DC in human (Bachem et al., 2010; Crozat et al., 2010; Jongbloed et al., 2010; Poulin et al., 2010). CD8α+ DEC205+ mouse DC not only excel in antigen cross-presentation, but are also specialized in the uptake of dying cells (Dudziak et al., 2007). Receptor-based antigen capture and cross-presentation was shown using a DEC205 antibody to which protein antigen was chemically coupled (Bonifaz et al., 2002; Bozzacco et al., 2007). Antigen internalized via DEC205 targeting results in the continuous, steady state capture and processing of antigen into peptide/class I MHC complexes in a manner that results in tolerogenic CD8+ T cell responses (Bonifaz et al., 2002). Besides DC, macrophages (Kovacsovics-Bankowski et al., 1993) and liver sinusoidal endothelial cells (Limmer et al., 2000) can also cross-present antigens in the steady state. In contrast, more cell types can cross-present antigen during inflammation. Mouse DC (Jung et al., 2002), macrophages (Kovacsovics-Bankowski et al., 1993), neutrophils (Beauvillain et al., 2007) and under specific conditions even B cells (Heit et al., 2004), have been demonstrated to cross-present antigen in vivo. Additionally, cell types that can cross-present antigen in vitro include basophils (Kim et al., 2009), γδ T cells (Brandes et al., 2009), mast cells (Stelekati et al., 2009), and endothelial cells (Bagai et al., 2005).
Although multiple cells can be involved in cross-priming in vivo, DC are especially important for this process, as shown by abrogated CD8+ T cell priming after depletion of CD11c+ cells (predominantly DC) in mice (Jung et al., 2002). Hence, this review focuses mainly on DC, for most cell-intrinsic mechanisms involved in cross-presentation of endocytosed antigen are described in this cell type.
In the last 15 years, at least 50 DC vaccination-based trials, aimed in part at harnessing effective CD8+ T cell responses were performed with overall minimal success (reviewed in Rosenberg et al., 2004). This review aims at providing insight in molecular mechanisms that are pivotal to cross-presentation.
Antigen recognition can trigger receptor-mediated endocytosis and can bring forth the ability of cells to cross-present the receptor-bound antigen (Sancho et al., 2009; Bachem et al., 2010; Jongbloed et al., 2010). In contrast to complement-opsonized antigen, immunoglobulin (Ig)-opsonized antigens are delivered in an endosomal compartment that favors cross-presentation by murine DC (Kim et al., 2008). Because Ig-opsonized antigen is predominantly endocytosed via Fc receptor and complement-opsonized antigen via complement receptor, this study indicates that antigen recognition dictates antigen delivery in distinct endosomal compartments. Ultimately, this may favor either the class I or class II MHC presentation pathway (reviewed in Flinsenberg et al., 2011).
Aside from antigen recognition, antigen size plays a role in its handling by phagocytes: particulate antigens that are larger than roughly 0.5 μm are internalized by phagocytosis, whereas smaller antigens are brought in by pinocytosis (Pratten and Lloyd, 1986; Mant et al., 2012). One major route of antigen internalization that yields cross-presentation seems to be phagocytosis, as particulate antigens are often more efficiently targeted for cross-presentation in comparison to their soluble counterparts (Graham et al., 2010). Thus, DC internalize antigens via distinct routes that are dictated by the structure of the antigen (i.e., particulate or soluble, size) and possible involvement of a recognizing endocytic receptor.
Receptor-mediated endocytosis is considered to be a highly efficient process that permits the selective retrieval of macromolecules present in the extracellular fluid (Brown et al., 1976). Such uptake depends on structural proteins that mediate the formation of lipid vacuoles, e.g., clathrin or caveolin (Mayor and Pagano, 2007). Within minutes of internalization from the plasma membrane into the endosomal pathway, antigen is located in vesicular compartments named early endosomes/phagosomes, characterized by a near neutral pH (pH 7.5; Savina et al., 2006) and presence of the small GTPase Rab5 (Simonsen et al., 1998). Endosomal maturation causes the fusion of early endosomes (EE) with late compartments, accompanied by transition of Rab5 expression to Rab7-positivity. The endosomal compartment is now renamed to late endosomes (LE) with their hallmark of a lowered pH of the endosomal lumen (pH 5.5). Further maturation of the LE leads to fusion with lysosomes in which the acidic environment (pH 4.7) and lysosomal proteases and hydrolases with low pH optima can mediate full degradation of luminal content (i.e., internalized antigen, but also cellular components for turnover). Especially DC harbor cellular mechanisms that prevent the rapid maturation-induced acidification of endosomal compartments, thereby allowing protein antigen fragments to remain intact for a prolonged time (as proteolytic activity by pH-sensitive proteases is restrained). Thereby, DC can cross-present antigen-derived peptides more efficiently than other phagocytes (Trombetta et al., 2003; Savina et al., 2006). Micro-organisms exploit these mechanisms to prevent their display as peptide/class I MHC complexes as immune evasion strategies (i.e., Mycobacteria and Salmonella; Jantsch et al., 2011; Johansen et al., 2011), as will be discussed in paragraph 6.
Peptides of 8–10 amino acids in length fit within the antigen-binding groove of class I MHC molecules, leading to a stable formation of peptide/class I MHC complexes (Urban et al., 1994; Rammensee, 1995). The proteasome is the foremost contributor to cleaved peptides for the classical class I MHC presentation pathway. Therefore its role in cross-presentation was assessed. The use of proteasome-selective inhibitors clarified the existence of both proteasome-dependent (Kovacsovics-Bankowski and Rock, 1995) and independent antigen processing (Shen et al., 2004; Saveanu et al., 2009) in distinct cross-presentation model systems. To date, two main routes leading to cross-presentation have broad experimental support: the cytosolic and vacuolar pathway. The cytosolic pathway proposes that endocytosed antigen is transported into the cytosol for proteasome/cytosolic peptidase-mediated degradation, whereas the vacuolar pathway relies on proteases for antigen processing within endosomes.
The cytosolic pathway model is supported by phagosome-to-cytosol translocation of OVA-beads (Kovacsovics-Bankowski and Rock, 1995), OVA-IgG and HRP-IgG immune complexes in murine cells (Rodriguez et al., 1999). Lin et al. (2008) demonstrated that cross-presentation competent CD8α+ and not the incapable CD8α− DC were sensitive for exogenously added cytochrome-c (cytochrome-c induces apoptosis when cytosolic concentrations are elevated). Antigen translocation from phagosome-to-cytosol involves processes that are antigen-specific, have antigen size-restrictions, may involve the reduction and unfolding of protein antigen and are Sec61 complex mediated (Rodriguez et al., 1999; Ackerman et al., 2006; Singh and Cresswell, 2010). In addition, cytosolic transfer of apoptotic peptides by neighboring cells and interacting dendritic cells can occur via gap-junctions into the cross-presenting cell (Pang et al., 2009).
The processing of antigen that is translocated into the cytosol involves the proteasome, as well as amino- and carboxy-terminal peptidases (Levy et al., 2002; Shen et al., 2011). The transporter associated with antigen processing (TAP) translocates the peptides into the endoplasmic reticulum (ER) which thereby enter the conventional class I MHC pathway (Ackerman et al., 2005), or back into the phagosomal pathway in an MyD88-dependent manner (Ackerman et al., 2003; Houde et al., 2003; Burgdorf et al., 2008). All necessary components to enable peptide trimming, loading, and translocation appear present and functional in early phagosomes (Houde et al., 2003; Ackerman et al., 2005). It was proposed that phagosome–ER fusion occurs to deliver the necessary components to the phagosome (Guermonprez et al., 2003). It now appears that rather than complete phagosome–ER fusion, which was disputed (Touret et al., 2005), only selective ER-derived components are delivered to phagosomes (Burgdorf et al., 2008; Cebrian et al., 2011). The SNARE Sec22b is shown to recruit ER-resident proteins to phagosomes that are necessary for phagosome-to-cytosol translocation (Cebrian et al., 2011).
Several groups demonstrated that peptide generation for cross-presentation may occur independent of the proteasome (Shen et al., 2004), while requiring endosomal acidification (Gromme et al., 1999; Di Pucchio et al., 2008). The proposed vacuolar pathway does not require phagosome-to-cytosol translocation, but relies on endosomal proteases for generation of antigenic peptides (Gromme et al., 1999; Di Pucchio et al., 2008). Shen et al. showed that cell-associated OVA can be degraded by both cathepsin S in the endosomal pathway or the cytosolic proteasome within one population of DC. This indicates that the proteasome-independent vacuolar pathway may co-exist with the cytosolic pathways. This possibility is supported by reports demonstrating that plasmacytoid DC cross-present in both proteasome-dependent and independent pathways (Hoeffel et al., 2007; Di Pucchio et al., 2008). In summary, antigen processing for cross-presentation depends on distinct proteolytic enzymes and may occur in the endosomal compartment as well as the cytosol.
These studies strengthen the concept that both antigen recognition and its physical characteristics affect antigen sorting into the given processing pathways, thereby influencing antigen presentation. Immunization studies showed that appropriate endosomal sorting is essential for efficient cross-presentation. Immunization with bead-coupled OVA caused CD8+ T cell responses and proliferation in an Fcγ receptor and DAP12-dependent manner. Cross-presentation of soluble OVA was independent of Fcγ receptors and DAP12 (Graham et al., 2007).
Early studies showed that TAP-dependent cross-presentation is sensitive to Brefeldin A through its ability to block ER-to-Golgi transport. These data fueled the initial proposal that peptide loading occurs in the ER (Kovacsovics-Bankowski and Rock, 1995; Fonteneau et al., 2003). However, the identification of Brefeldin A-independent antigen cross-presentation (Pfeifer et al., 1993; Belizaire and Unanue, 2009) and the discovery that components for peptide loading are present in phagosomes (Houde et al., 2003; Ackerman et al., 2005) suggest that peptide loading onto class I MHC may occur also outside of the ER. Class I MHC molecules are distributed in endosomal compartments, as shown in human melanoma epithelial cells (Mel JuSo cells) and lymphoblastoid cells (B-LCLs; Gromme et al., 1999; Kleijmeer et al., 2001). In contrast to endosomal class II MHC molecules, that can directly transit from the Golgi system to the endosomal pathway via association with the invariant chain chaperone, a major route for endosomal localization of class I MHC involves internalization from the plasma membrane. Peptide-class I MHC interactions are destabilized in late endosomal compartments (pH around 5.0), thereby facilitating peptide loading (Gromme et al., 1999). Further support came from TAP inhibition studies in which TAP function in the early and recycling endosomal (transferrin-positive) compartment was selectively disrupted (Burgdorf et al., 2008). Endosomal peptide loading would contribute to rapid cross-presentation of a selective set of endocytosed antigen-derived peptides, while decreasing the risk for competition with endogenous peptides that are assembled into peptide/class I MHC complexes in the ER.
For efficient endosomal peptide/class I MHC loading, class I MHC molecules must be delivered into the peptide loading compartment. Class I MHC molecules are constitutively internalized (Vega and Strominger, 1989). Mutational analysis of the cytoplasmic domain of class I MHC molecules identified several key residues that are essential for internalization (Lizee et al., 2003; Basha et al., 2008). An evolutionary-conserved tyrosine residue mediates the delivery into lysosomes (Lizee et al., 2003). This tyrosine residue is part of a known targeting motif YXX∅ (Y = tyrosine, X = any amino acid, ∅ = bulky hydrophobic amino acid) that has been shown to bind directly to adaptor protein (AP)-1, 2, or 3 (Bonifacino and Dell’Angelica, 1999). AP-tyrosine motif interaction results in selective incorporation of motif-containing cargo, such as the transferrin receptor, in clathrin-coated vesicles for uptake (Lizee et al., 2005). Besides endosomal targeting mediated by the tyrosine-based motif, the cytoplasmic domain of class I MHC molecules contains two or three conserved lysine residues (Duncan et al., 2006). Lysines are targets for ubiquitination that can also induce clathrin-mediated endocytosis. Studies on immune evasion strategies employed by Kaposi sarcoma associated herpesvirus (KSHV) identified two viral proteins, K3 and K5, that can downregulate cell surface bound class I MHC molecules via poly-ubiquitination (Lorenzo et al., 2002). Two human homologous proteins of K3 and K5, the membrane-associated RING-CH family MARCH IV and IX, are key regulators in class II MHC surface expression in B cells and DC (Gassart de et al., 2008). Moreover, MARCH IV and IX ubiquitinate class I MHC molecules and induce its internalization in an overexpression system (Bartee et al., 2004). Possibly these proteins can facilitate class I MHC endocytosis under physiological conditions, but this remains to be established. Thus, class I MHC molecules are taken up into the endosomal pathway of DC in a clathrin-dependent manner, enabling for sufficient amounts of endosomal class I MHC molecules to assemble into antigenic peptide/class I MHC complexes. As T cell activation requires presentation of multiple antigen-specific peptide/class I MHC complexes, the efficient transport of peptide/class I MHC complexes from peptide loading compartment to the cell surface is a further cross-presentation requirement that needs to be attained.
The intracellular location where peptide/class I MHC complexes are assembled dictates the trafficking route that is taken. Peptide loading within the ER probably results in transport via the biosynthetic pathway to the cell surface. In contrast, endosomal peptide/class I MHC assembly suggests an alternative route of transport.
The endosomal pathway contains both vesicular and tubular structures (Kleijmeer et al., 2001; Boes et al., 2002). During endocytosis, cell surface-derived membrane proteins and lipids are concomitantly taken up with antigen into endosomal vesicles (Scita and Di Fiore, 2010). To ensure steady surface display, most of the proteins and lipids are rapidly returned to the plasma membrane via the endosomal recycling pathway that consists of two main routes. Within minutes, retrograde recycling of membrane proteins from the EE to plasma membrane may occur, whereas a slower recycling route exists via juxtanuclear endosomal recycling compartments (ERC; Sonnichsen et al., 2000). It was estimated that cells internalize the equivalent of their cell surface one to five times per hour (Steinman et al., 1983), demonstrating the importance of endosomal recycling to normal cellular function.
There is experimental support that the recycling pathway may play a considerable role in antigen cross-presentation. Pharmacological inhibition of the recycling pathway by inclusion of primaquine in murine DC cultures abrogates cross-presentation of exogenous soluble antigen, without affecting class I MHC-mediated presentation of endogenously expressed antigen (Burgdorf et al., 2008). Similarly, Di Pucchio et al. (2008) report cross-presentation of a viral antigen by plasmacytoid DC in a Brefeldin A-resistant, but primaquine-sensitive manner. Furthermore, silencing of the small GTPases Rab3b and 3c, that colocalize with class I MHC molecules in recycling endosomes (RE) of DC2.4 cells, inhibits cross-presentation (Zou et al., 2009). Finally, mouse DC lacking class I MHC in recycling compartments due to expression of class I MHC with an aberrant tyrosine-based motif, are defective in cross-presentation (Lizee et al., 2003). Together, these reports demonstrate that interfering with the recycling pathway of class I MHC can abrogate cross-presentation, but the exact DC-intrinsic mechanisms for class I MHC molecule recycling that are involved in cross-presentation remain elusive.
The endosomal targeting of internalized antigen involves the selective recruitment of signaling molecules [i.e., EHD1 (Jovic et al., 2009) and Rab effector molecules (Hayakawa et al., 2007)]. One factor that regulate selective recruitment of signaling molecules is the small GTPase Arf6 (Brown et al., 2001). GDP/GTP cycling affects Arf6 function in membrane lipid and protein recycling. Active GTP-bound Arf6 localizes to the cytosolic side of the plasma membrane for clathrin-independent endocytosis, whereas GDP-bound Arf6 localized to tubular-like endosomal structures (Caplan et al., 2002).
The Rab family of small GTPases are considered key regulators of endocytic trafficking (Stenmark, 2009; Figure 1). Rab22a colocalizes with class I MHC in Arf6-associated tubules (Weigert et al., 2004), and the expression of dominant active or inactive versions of the Rab22a protein, or depletion of Rab22a, impairs class I MHC recycling to the cell surface (Weigert et al., 2004). Also other members of the Rab family are pivotal to class I MHC recycling. Both Rab35 (Allaire et al., 2010) and Rab11 (Sheff et al., 1999) are implicated in recycling from the ERC to the cell surface. Rab11 in complex with its effector Rab11-FIP2 interacts with one of four known mammalian C-terminal Eps15 homology (EH) domain containing proteins (EHD1; Naslavsky et al., 2006) that all play a role in endosomal trafficking (Naslavsky and Caplan, 2011). EHD1 is essential for recycling of both clathrin-dependent and independent endocytosed molecules, including but not restricted to class I MHC (Caplan et al., 2002) and class II MHC (Walseng et al., 2008).
Figure 1. Molecular mechanisms coordinating cargo recycling in the endosomal compartment. Antigens can be internalized by phagocytosis and (receptor-mediated) endocytosis and converge into early endosomes (EE). Class I MHC molecules are taken up by either clathrin-mediated endocytosis dependent on tyrosine-based internalization motif or poly-ubiquitination, or clathrin-independent but GTP-bound Arf6-dependent mechanism. The small GTPase Rab proteins dictate the selection of different effectors and binding partners, thereby directing cargo to distinct endosomal compartments, involving late endosomes (LE), lysosomes (lys), recycling endosomes (RE), and the endosomal recycling compartment (ERC). Rab3b and 3c are involved in rapid recycling of transferrin and are involved in cross-presentation. Rab4 together with Rab11 and Sorting Nexin 4 (SNX4) sort cargo into the ERC. Rab22a regulates class I MHC recycling via Arf6-positive tubules. Rab35 mediates recruitment of EHD1 for class I MHC recycling from early endosomes. EHD1 also colocalizes with Rab11 and its Rab11-FIP2, Arf6, and the Rab4 and Rab5 effector Rabenosyn-5. During Rab5-to-Rab7 transition, the retromer complex directs cargo to the trans-Golgi Network (TGN). The increased blue coloration illustrates the drop in endosomal pH. Further information and references are mentioned in the main text. = Class I MHC molecules;
= peptide;
= Clathrin;
= Rab;
= GTP-bound Arf6;
= GDP-bound Arf6.
Next to the recycling pathway via the juxtanuclear ERC, class I MHC molecules may also be directed toward the TGN for entering the biosynthetic pathway. Retrograde transport from the endosomal compartment to the TGN involves a hetero-pentameric complex called the retromer (reviewed in Bonifacino and Hurley, 2008). Thus far, however, interaction between class I MHC molecules and retromer complexes is not reported. However, depletion of the retromer-distinct sorting nexin SNX4 results in disruption of the ERC, and miss-sorting of the transferrin receptor to lysosomes. Therefore, SNX4 appears important for shuttling selective cargo between EE and the ERC (Traer et al., 2007). In conclusion, the ERC is a highly dynamic compartment composed of vesicular and tubular membrane structures, in which proper interplay between molecules including GTPases and Rab proteins contributes to antigen cross-presentation.
Membrane-associated cargo, including class I MHC molecules, is selectively transported to distinct endosomal compartments. But what regulates the structural support necessary for endosomal trafficking?
All eukaryotic cells have a filamentous network of cellular proteins, collectively termed the cytoskeleton. It mainly comprises three distinct classes of fibers: microfilaments, microtubules, and intermediate filaments. The cytoskeleton has multiple tasks. It gives the cell its rigidity and strength that helps maintaining cell shape. Moreover, it provides tracks that allow directed movement of organelles and their transport intermediates during intracellular trafficking processes. Microtubules are major components of the cytoskeleton, and are composed of α and β tubulin heterodimers. Disruption of these microtubules perturbs Arf6-associated recycling tubules (Radhakrishna and Donaldson, 1997), and thereby may abrogate endosomal transport of peptide/class I MHC complexes.
The continuous assembly and disassembly of microtubules creates a temporal and spatial dynamic network that allows for long-range endosomal transport (Jiang and Akhmanova, 2011). This network allows directional movements of motor proteins that associate with these microtubule tracks. Kinesins and dyneins are two families of such motor proteins. Most kinesins migrate over the microtubules in plus-end direction toward the cell periphery, whereas dyneins are directed to the minus-end, toward the microtubule-organizing center (MTOC; Wubbolts et al., 1999). Various cargo-selecting molecules or complexes from the endosomal compartment are linked to the motor proteins, allowing separation and movement of endosomal vacuoles over these microtubule tracks. For instance, several Rab proteins are associated directly to motor proteins, such as Rab14 with kinesin (Ueno et al., 2011) and Rab4 with dynein (Bielli et al., 2001). Similar to SNX4, most Rab proteins are indirectly linked to motor proteins via adaptor proteins, allowing separate trafficking processes in distinct responses. For example, Rab6 can interact with Bicaudal-related protein 1 (BICDR-1) or Bicaudal D-2 to associate with kinesin-3 or 1 respectively (Grigoriev et al., 2007; Schlager et al., 2010). Thus, endosomal small GTPase activity of Rab proteins can affect motor–microtubule interaction, thereby altering the segregation or guidance of cargo transport.
Directed assembly of microtubules may also allow for polarized trafficking and delivery of membrane proteins or (soluble mediator) cargo in high concentration to one specific spot. Upon cognate interaction between an antigen presenting cell (APC) and a T cell, the cytoskeleton forms a highly organized structure called the immunological synapse (IS). The IS is a region of spatially and temporally organized, highly concentrated motifs of membrane proteins and cytosolic molecules, formed at the T cell interaction site. The formation of the IS in DC is critical for subsequent T cell activation and depends on cytoskeletal rearrangement (Pulecio et al., 2008). Perturbation of the cytoskeleton abrogates IS formation and subsequent T cell activation (Al-Alwan et al., 2001). Endosomal compartments that transport class II MHC molecules converge at the IS upon cognate DC-T cell interaction (Boes et al., 2002, 2003; Bertho et al., 2003). In addition, a recent study demonstrates that ICAM-1, an adhesion molecule involved in strengthening the DC-T cell interaction, is targeted to the IS. This occurs either via the cell surface by cytoskeleton-dependent active transport, or via RE, where it colocalizes with class II MHC molecules. The latter pathway depends on continuous endocytosis and recycling of ICAM-1. Polarization of the recycling ICAM-1 to the DC-T cell interaction site in its turn depends on the high-affinity state of the ICAM-1 binding partner LFA-1 on T cells (Jo et al., 2010). This was not unexpected, as it was described earlier that blocking LFA-1 with an antibody on antigen-specific CD4+ T cells hampers remodeling of the endosomal class II MHC-containing compartment in murine DC (Bertho et al., 2003). Taken together, these data demonstrate that T cell-directed, cytoskeleton-supported recycling of antigen cargo is crucial for cellular immune responses.
In all cell types mentioned, the small GTPase CDC42 of the Rho family was shown to be responsible for MTOC polarization (Eng et al., 2007; Pulecio et al., 2010; Yuseff et al., 2011). Specifically, Pulecio et al. (2010) show that CDC42-mediated polarization mediates both MTOC polarization and directed transport of the cytokine IL-12 to the DC-T cell interaction site, which was crucial for antigen-specific CD8+ T cell proliferation and IFNγ production. Yuseff et al. (2011) demonstrated that atypical PKC is a downstream target of CDC42, required for MTOC polarization. CDC42 may be responsible for MTOC relocation by a mechanism that recruits the PAR6-atypical PKC complex to the plasma membrane in an Arf6-dependent manner, as was demonstrated to facilitate the establishment of polarity in migrating astrocytes (Etienne-Manneville and Hall, 2001; Osmani et al., 2010). Moreover, PAR6 overexpression reduced MTOC reorientation in murine macrophages (Eng et al., 2007). Taken these data together, CDC42-based polarity machinery plays an instrumental role in the polarization of the microtubule network and influences the direction of RE and other microtubule-associated trafficking.
Abrogated transport or recycling of class I and class II MHC complexes leads to immune-related disorders, as might be expected considering their importance in the initiation of immune- or tolerogenic responses. Indeed, an inefficient MHC transport leads to severe combined immunodeficiency as in patients with Bare Lymphocyte Syndrome type I. This disease can be caused by mutation in the TAP1, TAP2, or TAPBP genes, all leading to inefficient peptide/class I MHC transport and ultimately decreased cell surface expression (Salle de la et al., 1994). Hampering the transport of peptide/MHC complexes also plays a major role in viral infections. Herpes and Pox viruses can evade immune responses. They do this by several mechanisms including preventing the presentation of newly synthesized class I MHC molecules at the cell surface by blocking peptide translocation via TAP, block of peptide loading, retention of MHC/peptide complexes in the ER or their retrograde translocation into the cytosol for degradation, as illustrated in Figure 2 (van der Wal et al., 2002; Hansen and Bouvier, 2009; Horst et al., 2011). Increased MHC internalization also limits plasma membrane displayed peptide/class I MHC complexes and subsequent T cell activation (Zuo et al., 2011). Thus far no inborn mutations are known that correlate with mechanisms of MHC recycling or degradation. However, pathogens developed immune evasion strategies that interfere with endosomal transport of MHC or its recycling from the plasma membrane, with possible implications to antigen cross-presentation.
Figure 2. Viral evasion strategies aim at different pathways of MHC transport. 1: Inhibition of proteasomal processing (EBV); 2: Inhibition of TAP (EBV, HSV, CMV); 3: Inhibition of peptide transport (EBV, CMV, adeno); 4: Retaining MHC molecules in the ER (adeno, cowpox); 5: Target MHC for proteasomal degradation (CMV); 6: Target MHC from TGN to endo/lysosomal compartments (HIV); 7: Clathrin-dependent MHC internalization (EBV, KSHV, HIV, CMV); 8: Clathrin independent MHC internalization (HIV); 9: Arresting phagosomal maturation (mycobacteria, Salmonella, Chlamydia, and Leishmania); 10: Targeting MHC to the LE/lysosome (HSV); 11: Inhibition of retromer activity (HVS); 12: Inhibition of progression to the RE (CMV, HSV). = protein;
= peptide;
= TAP;
= Clathrin;
= Class I MHC molecules;
= proteasome;
= GTP-bound ARF6.
For example, EBV-derived BILF-1 and the previously mentioned K3 and K5 of KSHV decrease class I MHC surface expression by increasing its internalization, aiding the virus in escaping the immune system (Coscoy and Ganem, 2000; Ishido et al., 2000; Lorenzo et al., 2002; Mansouri et al., 2006; Zuo et al., 2011). HIV uses multiple strategies to evade the immune system (Roeth et al., 2004; Dikeakos et al., 2010). HIV-1 expresses the Nef protein that targets newly synthesized class I MHC from the TGN to the destructive lysosomal compartments, thereby preventing cell surface expression of peptide/class I MHC. Moreover, Nef increases the turnover of MHC surface molecules by targeting MHC to lysosomes via the clathrin-dependent retromer-mediated pathway (Peterlin and Trono, 2003). Additionally, Nef and host-derived sorting protein PACS1 interfere with MHC recycling by targeting and retaining MHC from the plasma membrane in the TGN via the previously described clathrin-independent ARF6 endocytic pathway (Blagoveshchenskaya et al., 2002). Recycling of MHC is also abrogated by Murine Cytomegalovirus, which induces an arrest of MHC in EE (Tomas et al., 2010). The retromer is also targeted for immune evasion as was reported for Herpesvirus Saimiri (HVS) infection. HVS-derived tyrosine kinase-interacting protein binds and redistributes the retromer subunit Vps35 from the EE to lysosomes, thereby inhibiting retromer activity. This is physiologically linked to CD4+ T cell downregulation and immortalization (Kingston et al., 2011), but possible retromer-targeted effects by HVS on cross-presentation remain to be shown.
The enormous number of viruses targeting peptide/MHC expression and endosomal trafficking illustrates its crucial role in anti-viral responses. Bacteria can also use these mechanisms to create an environment in which they can thrive (Duclos and Desjardins, 2000). Intracellular pathogens can replicate in vacuoles that retain an elevated pH, show limited hydrolytic activity, and intersect poorly with antigen presentation pathways. To achieve this, pathogens such as Mycobacteria hamper phagosome–lysosome fusion and Salmonella delays vacuolar acidification, thereby inducing arrest of phagosomal maturation (Jantsch et al., 2011; Johansen et al., 2011). Bacteria can also use endosomal remodeling and recycling for their own benefit. For example, Rab14 and syntaxin 6, which are together with IRAP involved in cross-presentation (Saveanu et al., 2009; Weimershaus et al., 2012), are recruited to Chlamydial inclusion vacuoles (Capmany et al., 2011; Moore et al., 2011). Also, a recent investigation demonstrates that Salmonella induces kinesin activity by the expression of Arl8B, an Arf family member (Kaniuk et al., 2011). Concomitant with increased kinesin activity, endosomal remodeling into tubular-like structures is promoted by Arl8B (Kaniuk et al., 2011), thereby creating an opportunity for Salmonella to transfer from cell to cell.
In summary, peptide/MHC surface expression is pivotal in initiating T cell responses and is therefore an important target in pathogen evasion strategies. Pathogens interfere with endosomal transport of MHC molecules to the plasma membrane, internalization of MHC and subsequent recycling or degradation. Knowledge of these processes is important in therapeutic interventions aiming at clearance of infections via appropriately activated MHC-restricted T cell responses. Drugs specifically targeting viral evasion molecules could re-establish proper peptide/MHC presentation, thereby allowing the immune system to clear the virus. Secondly, it is important to clarify evasion strategies employed by prevalent pathogens in future cellular vaccination developments, e.g., DC-based vaccine strategies, since such evasion could impair vaccine effectiveness.
Efficient cross-presentation of CD8+ T cells that initiates balanced anti-viral and anti-tumor immune responses depends on DC-intrinsic mechanisms that enable the sequential interaction of specific TCR molecules with peptide/MHC complexes in the context of activating or inhibiting (tolerogenic) signals. The molecular mechanisms described in this review all aid to ensure the quantity and quality of this DC-derived signal toward the CD8+ T cells.
Antigen recognition by specific receptors permits the selective and rapid retrieval of antigens present in the extracellular fluid, focusing the antigen pool that is directed toward cross-presentation. Additionally, targeting antigen to specific receptors allow it to target toward superior cross-presenting DC subsets (Sancho et al., 2009), or to overcome prior incapability of antigen cross-presentation (Klechevsky et al., 2010). Not surprisingly, targeting antigen increases antigen cross-presentation in vivo (National Library of Medicine US, 2010; Flynn et al., 2011) and is currently used in first phase clinical trials (140, DCVax-001). It now appears that next to efficient antigen uptake, receptor selection is instrumental for antigen delivery to cross-presentation competent compartments. Antigen introduction, as well as co-presence of “danger signals” appears to optimize, at least in some circumstances, the capability of selective endosomal compartments to support antigen cross-presentation, by recruitment of necessary components for cross-presentation (Naslavsky et al., 2006; Jancic et al., 2007; Burgdorf et al., 2008; Kim et al., 2008).
Dendritic cells maturation is accompanied by dramatic changes in cell shape. Since the cytoskeleton is responsible for cell shape, danger signaling is likely involved in cytoskeletal reorganization. Indeed, Toll-like receptor 4 (TLR4) signaling induces actin cytoskeleton remodeling in a MyD88-dependent manner (West et al., 2004). In addition, MTOC reorientation in DC by binding of antigen-specific T cells required TLR signaling (Pulecio et al., 2010). However, this could be an indirect effect due to the fact that mature DC form more stable synapses than immature DC (Benvenuti et al., 2004). Moreover, innate signals via MyD88 are demonstrated in murine DC to remodel the late endosomal compartment in which class II MHC peptide loading occurs (Boes et al., 2002). All together, these data demonstrate a beneficial role for innate signaling in presentation of antigens. However, exact molecular mechanisms that link innate signaling with directed cargo transportation remain elusive.
A large amount of viral immune evasion strategies generated by the evolutionary pressure of the endosomal recycling pathway on anti-viral responses suggests that efficient recycling of class I MHC molecules is essential for an effective CD8+ T cell response. An exon7-deleted variant of class I MHC clearly demonstrates that only a small delay in class I MHC recycling greatly affects CD8+ T cell responses (Rodriguez-Cruz et al., 2011): antigen cross-presentation by exon7-deleted class I MHC molecules-expressing cells results in more robust CD8+ T cell responses.
The quality of the MHC/TCR interaction (e.g., signal 1) affects DC-CD8+ T cell interaction strength, thereby affecting CD8+ T effector function (Bouma et al., 2011), memory differentiation (Teixeiro et al., 2009) and survival (Iezzi et al., 1999). Hence, primary immune deficiencies with defective quality of signal 1, such as Wiskott-Aldrich syndrome and DOCK8 immunodeficiency patients, share clinical characteristics (e.g., eczema, elevated IgE levels, cutaneous M. contagiosum or Papilloma and Herpes viral infections, and increased tumor incidence (Bosticardo et al., 2009; Zhang et al., 2009). Both Dock8 and WAS protein are important for T cell synapse formation (Dupre et al., 2002; Randall et al., 2011), and crucial for interactions between naive CD8+ T cells and DC (Pulecio et al., 2008; Randall et al., 2011). Thus, the endosomal recycling pathway may prove of importance for antigen cross-presentation and prevention of correlated diseases via distinct mechanisms, some of which are outlined above, and likely with more to be uncovered in the years to come.
The authors declare that the research was conducted in the absence of any commercial or financial relationships that could be construed as a potential conflict of interest.
We acknowledge financial support from the Wilhelmina Research Fund and an NWO/NGI Horizon grant (to Marianne Boes). We thank Michiel de Jong (www.TomatoesandPotatoes.com) for his aid to design the figures. We additionally thank Henk Schipper and Robert van der Burgh for critical reading of the manuscript, the members of the Boes laboratory, and members of the Center for Molecular and Cellular Intervention (CMCI) for helpful discussions.
Ackerman, A. L., Giodini, A., and Cresswell, P. (2006). A role for the endoplasmic reticulum protein retrotranslocation machinery during crosspresentation by dendritic cells. Immunity 25, 607–617.
Ackerman, A. L., Kyritsis, C., Tampe, R., and Cresswell, P. (2003). Early phagosomes in dendritic cells form a cellular compartment sufficient for cross presentation of exogenous antigens. Proc. Natl. Acad. Sci. U.S.A. 100, 12889–12894.
Ackerman, A. L., Kyritsis, C., Tampe, R., and Cresswell, P. (2005). Access of soluble antigens to the endoplasmic reticulum can explain cross-presentation by dendritic cells. Nat. Immunol. 6, 107–113.
Al-Alwan, M. M., Rowden, G., Lee, T. D., and West, K. A. (2001). The dendritic cell cytoskeleton is critical for the formation of the immunological synapse. J. Immunol. 166, 1452–1456.
Albert, M. L., Jegathesan, M., and Darnell, R. B. (2001). Dendritic cell maturation is required for the cross-tolerization of CD8+ T cells. Nat. Immunol. 2, 1010–1017.
Allaire, P. D., Marat, A. L., Dall’Armi, C., Di, P. G., McPherson, P. S., and Ritter, B. (2010). The connecdenn DENN domain: a GEF for Rab35 mediating cargo-specific exit from early endosomes. Mol. Cell 37, 370–382.
Bachem, A., Guttler, S., Hartung, E., Ebstein, F., Schaefer, M., Tannert, A., Salama, A., Movassaghi, K., Opitz, C., Mages, H. W., Henn, V., Kloetzel, P. M., Gurka, S., and Kroczek, R. A. (2010). Superior antigen cross-presentation and XCR1 expression define human CD11c+ CD141+ cells as homologues of mouse CD8+ dendritic cells. J. Exp. Med. 207, 1273–1281.
Bagai, R., Valujskikh, A., Canaday, D. H., Bailey, E., Lalli, P. N., Harding, C. V., and Heeger, P. S. (2005). Mouse endothelial cells cross-present lymphocyte-derived antigen on class I MHC via a. J. Immunol. 174, 7711–7715.
Bartee, E., Mansouri, M., Hovey Nerenberg, B. T., Gouveia, K., and Fruh, K. (2004). Downregulation of major histocompatibility complex class I by human ubiquitin ligases related to viral immune evasion proteins. J. Virol. 78, 1109–1120.
Basha, G., Lizee, G., Reinicke, A. T., Seipp, R. P., Omilusik, K. D., and Jefferies, W. A. (2008). MHC class I endosomal and lysosomal trafficking coincides with exogenous antigen loading in dendritic cells. PLoS ONE 3, e3247. doi:10.1371/journal.pone.0003247
Beauvillain, C., Delneste, Y., Scotet, M., Peres, A., Gascan, H., Guermonprez, P., Barnaba, V., and Jeannin, P. (2007). Neutrophils efficiently cross-prime naive T cells in vivo. Blood 110, 2965–2973.
Belizaire, R., and Unanue, E. R. (2009). Targeting proteins to distinct subcellular compartments reveals unique requirements for MHC class I and II presentation. Proc. Natl. Acad. Sci. U.S.A. 106, 17463–17468.
Benvenuti, F., Lagaudriere-Gesbert, C., Grandjean, I., Jancic, C., Hivroz, C., Trautmann, A., Lantz, O., and Amigorena, S. (2004). Dendritic cell maturation controls adhesion, synapse formation, and the duration of the interactions with naive T lymphocytes. J. Immunol. 172, 292–301.
Bertho, N., Cerny, J., Kim, Y. M., Fiebiger, E., Ploegh, H., and Boes, M. (2003). Requirements for T cell-polarized tubulation of class II+ compartments in dendritic cells. J. Immunol. 171, 5689–5696.
Bevan, M. J. (1976). Cross-priming for a secondary cytotoxic response to minor H antigens with H-2 congenic cells which do not cross-react in the cytotoxic assay. J. Exp. Med. 143, 1283–1288.
Bielli, A., Thornqvist, P. O., Hendrick, A. G., Finn, R., Fitzgerald, K., and McCaffrey, M. W. (2001). The small GTPase Rab4A interacts with the central region of cytoplasmic dynein light intermediate chain-1. Biochem. Biophys. Res. Commun. 281, 1141–1153.
Blagoveshchenskaya, A. D., Thomas, L., Feliciangeli, S. F., Hung, C. H., and Thomas, G. (2002). HIV-1 Nef downregulates MHC-I by a PACS-1- and PI3K-regulated ARF6 endocytic pathway. Cell 111, 853–866.
Boes, M., Bertho, N., Cerny, J., Op den, B. M., Kirchhausen, T., and Ploegh, H. (2003). T cells induce extended class II MHC compartments in dendritic cells in a Toll-like receptor-dependent manner. J. Immunol. 171, 4081–4088.
Boes, M., Cerny, J., Massol, R., Op den, B. M., Kirchhausen, T., Chen, J., and Ploegh, H. L. (2002). T-cell engagement of dendritic cells rapidly rearranges MHC class II transport. Nature 418, 983–988.
Bonifacino, J. S., and Dell’Angelica, E. C. (1999). Molecular bases for the recognition of tyrosine-based sorting signals. J. Cell Biol. 145, 923–926.
Bonifaz, L., Bonnyay, D., Mahnke, K., Rivera, M., Nussenzweig, M. C., and Steinman, R. M. (2002). Efficient targeting of protein antigen to the dendritic cell receptor DEC-205 in the steady state leads to antigen presentation on major histocompatibility complex class I products and peripheral CD8+ T cell tolerance. J. Exp. Med. 196, 1627–1638.
Bosticardo, M., Marangoni, F., Aiuti, A., Villa, A., and Grazia, R. M. (2009). Recent advances in understanding the pathophysiology of Wiskott-Aldrich syndrome. Blood 113, 6288–6295.
Bouma, G., Mendoza-Naranjo, A., Blundell, M. P., de, F. E., Parsley, K. L., Burns, S. O., and Thrasher, A. J. (2011). Cytoskeletal remodeling mediated by WASp in dendritic cells is necessary for normal immune synapse formation and T-cell priming. Blood 118, 2492–2501.
Bozzacco, L., Trumpfheller, C., Siegal, F. P., Mehandru, S., Markowitz, M., Carrington, M., Nussenzweig, M. C., Piperno, A. G., and Steinman, R. M. (2007). DEC-205 receptor on dendritic cells mediates presentation of HIV gag protein to CD8+ T cells in a spectrum of human MHC I haplotypes. Proc. Natl. Acad. Sci. U.S.A. 104, 1289–1294.
Brandes, M., Willimann, K., Bioley, G., Levy, N., Eberl, M., Luo, M., Tampe, R., Levy, F., Romero, P., and Moser, B. (2009). Cross-presenting human gammadelta T cells induce robust CD8+ alphabeta T cell responses. Proc. Natl. Acad. Sci. U.S.A. 106, 2307–2312.
Brown, F. D., Rozelle, A. L., Yin, H. L., Balla, T., and Donaldson, J. G. (2001). Phosphatidylinositol 4,5-bisphosphate and Arf6-regulated membrane traffic. J. Cell Biol. 154, 1007–1017.
Brown, M. S., Ho, Y. K., and Goldstein, J. L. (1976). The low-density lipoprotein pathway in human fibroblasts: relation between cell surface receptor binding and endocytosis of low-density lipoprotein. Ann. N. Y. Acad. Sci. 275, 244–257.
Burgdorf, S., Scholz, C., Kautz, A., Tampe, R., and Kurts, C. (2008). Spatial and mechanistic separation of cross-presentation and endogenous antigen presentation. Nat. Immunol. 9, 558–566.
Caplan, S., Naslavsky, N., Hartnell, L. M., Lodge, R., Polishchuk, R. S., Donaldson, J. G., and Bonifacino, J. S. (2002). A tubular EHD1-containing compartment involved in the recycling of major histocompatibility complex class I molecules to the plasma membrane. EMBO J. 21, 2557–2567.
Capmany, A., Leiva, N., and Damiani, M. T. (2011). Golgi-associated Rab14, a new regulator for Chlamydia trachomatis infection outcome. Commun. Integr. Biol. 4, 590–593.
Cebrian, I., Visentin, G., Blanchard, N., Jouve, M., Bobard, A., Moita, C., Enninga, J., Moita, L. F., Amigorena, S., and Savina, A. (2011). Sec22b regulates phagosomal maturation and antigen crosspresentation by dendritic cells. Cell 74, 1355–1368.
Coscoy, L., and Ganem, D. (2000). Kaposi’s sarcoma-associated herpesvirus encodes two proteins that block cell surface display of MHC class I chains by enhancing their endocytosis. Proc. Natl. Acad. Sci. U.S.A. 97, 8051–8056.
Crozat, K., Guiton, R., Contreras, V., Feuillet, V., Dutertre, C. A., Ventre, E., Vu Manh, T. P., Baranek, T., Storset, A. K., Marvel, J., Boudinot, P., Hosmalin, A., Schwartz-Cornil, I., and Dalod, M. (2010). The XC chemokine receptor 1 is a conserved selective marker of mammalian cells homologous to mouse CD8alpha+ dendritic cells. J. Exp. Med. 207, 1283–1292.
den Haan, J. M., Lehar, S. M., and Bevan, M. J. (2000). CD8(+) but not CD8(−) dendritic cells cross-prime cytotoxic T cells in vivo. J. Exp. Med. 192, 1685–1696.
Di Pucchio, T., Chatterjee, B., Smed-Sorensen, A., Clayton, S., Palazzo, A., Montes, M., Xue, Y., Mellman, I., Banchereau, J., and Connolly, J. E. (2008). Direct proteasome-independent cross-presentation of viral antigen by plasmacytoid dendritic cells on major histocompatibility complex class I. Nat. Immunol. 9, 551–557.
Dikeakos, J. D., Atkins, K. M., Thomas, L., Emert-Sedlak, L., Byeon, I. J., Jung, J., Ahn, J., Wortman, M. D., Kukull, B., Saito, M., Koizumi, H., Williamson, D. M., Hiyoshi, M., Barklis, E., Takiguchi, M., Suzu, S., Gronenborn, A. M., Smithgall, T. E., and Thomas, G. (2010). Small molecule inhibition of HIV-1-induced MHC-I down-regulation identifies a temporally regulated switch in Nef action. Mol. Biol. Cell 21, 3279–3292.
Doherty, P. C., and Zinkernagel, R. M. (1975). H-2 compatibility is required for T-cell-mediated lysis of target cells infected with lymphocytic choriomeningitis virus. J. Exp. Med. 141, 502–507.
Duclos, S., and Desjardins, M. (2000). Subversion of a young phagosome: the survival strategies of intracellular pathogens. Cell. Microbiol. 2, 365–377.
Dudziak, D., Kamphorst, A. O., Heidkamp, G. F., Buchholz, V. R., Trumpfheller, C., Yamazaki, S., Cheong, C., Liu, K., Lee, H. W., Park, C. G., Steinman, R. M., and Nussenzweig, M. C. (2007). Differential antigen processing by dendritic cell subsets in vivo. Science 315, 107–111.
Duncan, L. M., Piper, S., Dodd, R. B., Saville, M. K., Sanderson, C. M., Luzio, J. P., and Lehner, P. J. (2006). Lysine-63-linked ubiquitination is required for endolysosomal degradation of class I molecules. EMBO J. 25, 1635–1645.
Dupre, L., Aiuti, A., Trifari, S., Martino, S., Saracco, P., Bordignon, C., and Roncarolo, M. G. (2002). Wiskott-Aldrich syndrome protein regulates lipid raft dynamics during immunological synapse formation. Immunity 17, 157–166.
Eng, E. W., Bettio, A., Ibrahim, J., and Harrison, R. E. (2007). MTOC reorientation occurs during FcgammaR-mediated phagocytosis in macrophages. Mol. Biol. Cell 18, 2389–2399.
Etienne-Manneville, S., and Hall, A. (2001). Integrin-mediated activation of Cdc42 controls cell polarity in migrating astrocytes through PKCzeta. Cell 106, 489–498.
Flinsenberg, T. W., Compeer, E. B., Boelens, J. J., and Boes, M. (2011). Antigen cross-presentation: extending recent laboratory findings to therapeutic intervention. Clin. Exp. Immunol. 165, 8–18.
Flynn, B. J., Kastenmuller, K., Wille-Reece, U., Tomaras, G. D., Alam, M., Lindsay, R. W., Salazar, A. M., Perdiguero, B., Gomez, C. E., Wagner, R., Esteban, M., Park, C. G., Trumpfheller, C., Keler, T., Pantaleo, G., Steinman, R. M., and Seder, R. (2011). Immunization with HIV Gag targeted to dendritic cells followed by recombinant New York vaccinia virus induces robust T-cell immunity in nonhuman primates. Proc. Natl. Acad. Sci. U.S.A. 108, 7131–7136.
Fonteneau, J. F., Kavanagh, D. G., Lirvall, M., Sanders, C., Cover, T. L., Bhardwaj, N., and Larsson, M. (2003). Characterization of the MHC class I cross-presentation pathway for cell-associated antigens by human dendritic cells. Blood 102, 4448–4455.
Gassart de, A., Camosseto, V., Thibodeau, J., Ceppi, M., Catalan, N., Pierre, P., and Gatti, E. (2008). MHC class II stabilization at the surface of human dendritic cells is the result of maturation-dependent MARCH I down-regulation. Proc. Natl. Acad. Sci. U.S.A. 105, 3491–3496.
Graham, D. B., Akilesh, H. M., Gmyrek, G. B., Piccio, L., Gilfillan, S., Sim, J., Belizaire, R., Carrero, J. A., Wang, Y., Blaufuss, G. S., Sandoval, G., Fujikawa, K., Cross, A. H., Russell, J. H., Cella, M., and Swat, W. (2010). ITAM signaling in dendritic cells controls T helper cell priming by regulating MHC class II recycling. Blood 116, 3208–3218.
Graham, D. B., Stephenson, L. M., Lam, S. K., Brim, K., Lee, H. M., Bautista, J., Gilfillan, S., Akilesh, S., Fujikawa, K., and Swat, W. (2007). An ITAM-signaling pathway controls cross-presentation of particulate but not soluble antigens in dendritic cells. J. Exp. Med. 204, 2889–2897.
Grigoriev, I., Splinter, D., Keijzer, N., Wulf, P. S., Demmers, J., Ohtsuka, T., Modesti, M., Maly, I. V., Grosveld, F., Hoogenraad, C. C., and Akhmanova, A. (2007). Rab6 regulates transport and targeting of exocytotic carriers. Dev. Cell 13, 305–314.
Gromme, M., Uytdehaag, F. G., Janssen, H., Calafat, J., van Binnendijk, R. S., Kenter, M. J., Tulp, A., Verwoerd, D., and Neefjes, J. (1999). Recycling MHC class I molecules and endosomal peptide loading. Proc. Natl. Acad. Sci. U.S.A. 96, 10326–10331.
Guermonprez, P., Saveanu, L., Kleijmeer, M., Davoust, J., van, E. P., and Amigorena, S. (2003). ER-phagosome fusion defines an MHC class I cross-presentation compartment in dendritic cells. Nature 425, 397–402.
Hansen, T. H., and Bouvier, M. (2009). MHC class I antigen presentation: learning from viral evasion strategies. Nat. Rev. Immunol. 9, 503–513.
Hayakawa, A., Hayes, S., Leonard, D., Lambright, D., and Corvera, S. (2007). Evolutionarily conserved structural and functional roles of the FYVE domain. Biochem. Soc. Symp. 74, 95–105.
Heath, W. R., and Carbone, F. R. (2001). Cross-presentation in viral immunity and self-tolerance. Nat. Rev. Immunol. 1, 126–134.
Heit, A., Huster, K. M., Schmitz, F., Schiemann, M., Busch, D. H., and Wagner, H. (2004). CpG-DNA aided cross-priming by cross-presenting B cells. J. Immunol. 172, 1501–1507.
Hoeffel, G., Ripoche, A. C., Matheoud, D., Nascimbeni, M., Escriou, N., Lebon, P., Heshmati, F., Guillet, J. G., Gannage, M., Caillat-Zucman, S., Casartelli, N., Schwartz, O., De la, S. H., Hanau, D., Hosmalin, A., and Maranon, C. (2007). Antigen crosspresentation by human plasmacytoid dendritic cells. Immunity 27, 481–492.
Horst, D., Verweij, M. C., Davison, A. J., Ressing, M. E., and Wiertz, E. J. (2011). Viral evasion of T cell immunity: ancient mechanisms offering new applications. Curr. Opin. Immunol. 23, 96–103.
Houde, M., Bertholet, S., Gagnon, E., Brunet, S., Goyette, G., Laplante, A., Princiotta, M. F., Thibault, P., Sacks, D., and Desjardins, M. (2003). Phagosomes are competent organelles for antigen cross-presentation. Nature 425, 402–406.
Iezzi, G., Scotet, E., Scheidegger, D., and Lanzavecchia, A. (1999). The interplay between the duration of TCR and cytokine signaling determines T cell polarization. Eur. J. Immunol. 29, 4092–4101.
Ishido, S., Wang, C., Lee, B. S., Cohen, G. B., and Jung, J. U. (2000). Downregulation of major histocompatibility complex class I molecules by Kaposi’s sarcoma-associated herpesvirus K3 and K5 proteins. J. Virol. 74, 5300–5309.
Jancic, C., Savina, A., Wasmeier, C., Tolmachova, T., El-Benna, J., Dang, P. M., Pascolo, S., Gougerot-Pocidalo, M. A., Raposo, G., Seabra, M. C., and Amigorena, S. (2007). Rab27a regulates phagosomal pH and NADPH oxidase recruitment to dendritic cell phagosomes. Nat. Cell Biol. 9, 367–378.
Jantsch, J., Chikkaballi, D., and Hensel, M. (2011). Cellular aspects of immunity to intracellular Salmonella enterica. Immunol. Rev. 240, 185–195.
Jiang, K., and Akhmanova, A. (2011). Microtubule tip-interacting proteins: a view from both ends. Curr. Opin. Cell Biol. 23, 94–101.
Jo, J. H., Kwon, M. S., Choi, H. O., Oh, H. M., Kim, H. J., and Jun, C. D. (2010). Recycling and LFA-1-dependent trafficking of ICAM-1 to the immunological synapse. J. Cell. Biochem. 111, 1125–1137.
Johansen, P., Fettelschoss, A., Amstutz, B., Selchow, P., Waeckerle-Men, Y., Keller, P., Deretic, V., Held, L., Kundig, T. M., Bottger, E. C., and Sander, P. (2011). Relief from Zmp1-mediated arrest of phagosome maturation is associated with facilitated presentation and enhanced immunogenicity of mycobacterial antigens. Clin. Vaccine Immunol. 18, 907–913.
Jongbloed, S. L., Kassianos, A. J., McDonald, K. J., Clark, G. J., Ju, X., Angel, C. E., Chen, C. J., Dunbar, P. R., Wadley, R. B., Jeet, V., Vulink, A. J., Hart, D. N., and Radford, K. J. (2010). Human CD141+ (BDCA-3)+ dendritic cells (DCs) represent a unique myeloid DC subset that cross-presents necrotic cell antigens. J. Exp. Med. 207, 1247–1260.
Jovic, M., Kieken, F., Naslavsky, N., Sorgen, P. L., and Caplan, S. (2009). Eps15 homology domain 1-associated tubules contain phosphatidylinositol-4-phosphate and phosphatidylinositol-(4,5)-bisphosphate and are required for efficient recycling. Mol. Biol. Cell 20, 2731–2743.
Jung, S., Unutmaz, D., Wong, P., Sano, G., De los, S. K., Sparwasser, T., Wu, S., Vuthoori, S., Ko, K., Zavala, F., Pamer, E. G., Littman, D. R., and Lang, R. A. (2002). In vivo depletion of CD11c+ dendritic cells abrogates priming of CD8+ T cells by exogenous cell-associated antigens. Immunity 17, 211–220.
Kaniuk, N. A., Canadien, V., Bagshaw, R. D., Bakowski, M., Braun, V., Landekic, M., Mitra, S., Huang, J., Heo, W. D., Meyer, T., Pelletier, L., ndrews-Polymenis, H., McClelland, M., Pawson, T., Grinstein, S., and Brumell, J. H. (2011). Salmonella exploits Arl8B-directed kinesin activity to promote endosome tubulation and cell-to-cell transfer. Cell. Microbiol. 13, 1812–1823.
Kim, S., Shen, T., and Min, B. (2009). Basophils can directly present or cross-present antigen to CD8 lymphocytes and alter CD8 T cell differentiation into IL-10-producing phenotypes. J. Immunol. 183, 3033–3039.
Kim, S. H., Visser, A., Cruijsen, C., van der Velden, A. W., and Boes, M. (2008). Recruitment of Rab27a to phagosomes controls microbial antigen cross-presentation by dendritic cells. Infect. Immun. 76, 5373–5380.
Kingston, D., Chang, H., Ensser, A., Lee, H. R., Lee, J., Lee, S. H., Jung, J. U., and Cho, N. H. (2011). Inhibition of retromer activity by Herpesvirus Saimiri tip leads to CD4 downregulation and efficient T cell transformation. J. Virol. 85, 10627–10638.
Klechevsky, E., Flamar, A. L., Cao, Y., Blanck, J. P., Liu, M., O’Bar, A., Gouna-Deciat, O., Klucar, P., Thompson-Snipes, L., Zurawski, S., Reiter, Y., Palucka, A. K., Zurawski, G., and Banchereau, J. (2010). Cross-priming CD8+ T cells by targeting antigens to human dendritic cells through DCIR. Blood 116, 1685–1697.
Kleijmeer, M. J., Escola, J. M., Uytdehaag, F. G., Jakobson, E., Griffith, J. M., Osterhaus, A. D., Stoorvogel, W., Melief, C. J., Rabouille, C., and Geuze, H. J. (2001). Antigen loading of MHC class I molecules in the endocytic tract. Traffic 2, 124–137.
Kovacsovics-Bankowski, M., Clark, K., Benacerraf, B., and Rock, K. L. (1993). Efficient major histocompatibility complex class I presentation of exogenous antigen upon phagocytosis by macrophages. Proc. Natl. Acad. Sci. U.S.A. 90, 4942–4946.
Kovacsovics-Bankowski, M., and Rock, K. L. (1995). A phagosome-to-cytosol pathway for exogenous antigens presented on MHC class I molecules. Science 267, 243–246.
Kurts, C., Heath, W. R., Carbone, F. R., Allison, J., Miller, J. F., and Kosaka, H. (1996). Constitutive class I-restricted exogenous presentation of self antigens in vivo. J. Exp. Med. 184, 923–930.
Levy, F., Burri, L., Morel, S., Peitrequin, A. L., Levy, N., Bachi, A., Hellman, U., Van den Eynde, B. J., and Servis, C. (2002). The final N-terminal trimming of a subaminoterminal proline-containing HLA class I-restricted antigenic peptide in the cytosol is mediated by two peptidases. J. Immunol. 169, 4161–4171.
Limmer, A., Ohl, J., Kurts, C., Ljunggren, H. G., Reiss, Y., Groettrup, M., Momburg, F., Arnold, B., and Knolle, P. A. (2000). Efficient presentation of exogenous antigen by liver endothelial cells to CD8+ T cells results in antigen-specific T-cell tolerance. Nat. Med. 6, 1348–1354.
Lin, M. L., Zhan, Y., Villadangos, J. A., and Lew, A. M. (2008). The cell biology of cross-presentation and the role of dendritic cell subsets. Immunol. Cell Biol. 86, 353–362.
Lizee, G., Basha, G., and Jefferies, W. A. (2005). Tails of wonder: endocytic-sorting motifs key for exogenous antigen presentation. Trends Immunol. 26, 141–149.
Lizee, G., Basha, G., Tiong, J., Julien, J. P., Tian, M., Biron, K. E., and Jefferies, W. A. (2003). Control of dendritic cell cross-presentation by the major histocompatibility complex class I cytoplasmic domain. Nat. Immunol. 4, 1065–1073.
Lorenzo, M. E., Jung, J. U., and Ploegh, H. L. (2002). Kaposi’s sarcoma-associated herpesvirus K3 utilizes the ubiquitin-proteasome system in routing class major histocompatibility complexes to late endocytic compartments. J. Virol. 76, 5522–5531.
Mansouri, M., Douglas, J., Rose, P. P., Gouveia, K., Thomas, G., Means, R. E., Moses, A. V., and Fruh, K. (2006). Kaposi sarcoma herpesvirus K5 removes CD31/PECAM from endothelial cells. Blood 108, 1932–1940.
Mant, A., Chinnery, F., Elliott, T., and Williams, A. P. (2012). The pathway of cross-presentation is influenced by the particle size of phagocytosed antigen. Immunology. doi:10.1111/j.1365-2567.2012.03558.x. [Epub ahead of print].
Mayor, S., and Pagano, R. E. (2007). Pathways of clathrin-independent endocytosis. Nat. Rev. Mol. Cell Biol. 8, 603–612.
Moore, E. R., Mead, D. J., Dooley, C. A., Sager, J., and Hackstadt, T. (2011). The trans-Golgi SNARE syntaxin 6 is recruited to the chlamydial inclusion membrane. Microbiology 157, 830–838.
Naslavsky, N., and Caplan, S. (2011). EHD proteins: key conductors of endocytic transport. Trends Cell Biol. 21, 122–131.
Naslavsky, N., Rahajeng, J., Sharma, M., Jovic, M., and Caplan, S. (2006). Interactions between EHD proteins and Rab11-FIP2: a role for EHD3 in early endosomal transport. Mol. Biol. Cell 17, 163–177.
National Library of Medicine US. (2010). A Randomized, Placebo-Controlled, Dose-Escalating, Double-Blinded Phase I Study to Evaluate the Safety and Immunogenicity of Anti-DEC-205 Monoclonal Antibody (Mab) Targeted HIV Gag p24 Vaccine (DCVax-001) with Poly ICLC (Hiltonol) as Adjuvant in HIV-Uninfected Healthy Volunteers. Bethesda, MD: ClinicalTrials. gov. [May, 19; Identifier: NCT01127464; Internet]. Available at: http://clinicaltrials.gov/ct2/show/NCT01127464
Osmani, N., Peglion, F., Chavrier, P., and Etienne-Manneville, S. (2010). Cdc42 localization and cell polarity depend on membrane traffic. J. Cell Biol. 191, 1261–1269.
Pang, B., Neijssen, J., Qiao, X., Janssen, L., Janssen, H., Lippuner, C., and Neefjes, J. (2009). Direct antigen presentation and gap junction mediated cross-presentation during apoptosis. J. Immunol. 183, 1083–1090.
Peterlin, B. M., and Trono, D. (2003). Hide, shield and strike back: how HIV-infected cells avoid immune eradication. Nat. Rev. Immunol. 3, 97–107.
Pfeifer, J. D., Wick, M. J., Roberts, R. L., Findlay, K., Normark, S. J., and Harding, C. V. (1993). Phagocytic processing of bacterial antigens for class I MHC presentation to T cells. Nature 361, 359–362.
Poulin, L. F., Salio, M., Griessinger, E., njos-Afonso, F., Craciun, L., Chen, J. L., Keller, A. M., Joffre, O., Zelenay, S., Nye, E., Le, M. A., Faure, F., Donckier, V., Sancho, D., Cerundolo, V., Bonnet, D., and Reis e Sousa, C. (2010). Characterization of human DNGR-1+ BDCA3+ leukocytes as putative equivalents of mouse CD8alpha+ dendritic cells. J. Exp. Med. 207, 1261–1271.
Pratten, M. K., and Lloyd, J. B. (1986). Pinocytosis and phagocytosis: the effect of size of a particulate substrate on its mode of capture by rat peritoneal macrophages cultured in vitro. Biochim. Biophys. Acta 881, 307–313.
Pulecio, J., Petrovic, J., Prete, F., Chiaruttini, G., Lennon-Dumenil, A. M., Desdouets, C., Gasman, S., Burrone, O. R., and Benvenuti, F. (2010). Cdc42-mediated MTOC polarization in dendritic cells controls targeted delivery of cytokines at the immune synapse. J. Exp. Med. 207, 2719–2732.
Pulecio, J., Tagliani, E., Scholer, A., Prete, F., Fetler, L., Burrone, O. R., and Benvenuti, F. (2008). Expression of Wiskott-Aldrich syndrome protein in dendritic cells regulates synapse formation and activation of naive CD8+ T cells. J. Immunol. 181, 1135–1142.
Radhakrishna, H., and Donaldson, J. G. (1997). ADP-ribosylation factor 6 regulates a novel plasma membrane recycling pathway. J. Cell Biol. 139, 49–61.
Rammensee, H. G. (1995). Chemistry of peptides associated with MHC class I and class II molecules. Curr. Opin. Immunol. 7, 85–96.
Randall, K. L., Chan, S. S., Ma, C. S., Fung, I., Mei, Y., Yabas, M., Tan, A., Arkwright, P. D., Al, S. W., Lugo Reyes, S. O., Yamazaki-Nakashimada, M. A., Garcia-Cruz, L., Smart, J. M., Picard, C., Okada, S., Jouanguy, E., Casanova, J. L., Lambe, T., Cornall, R. J., Russell, S., Oliaro, J., Tangye, S. G., Bertram, E. M., and Goodnow, C. C. (2011). DOCK8 deficiency impairs CD8 T cell survival and function in humans and mice. J. Exp. Med. 208, 2305–2320.
Rodriguez, A., Regnault, A., Kleijmeer, M., Ricciardi-Castagnoli, P., and Amigorena, S. (1999). Selective transport of internalized antigens to the cytosol for MHC class I presentation in dendritic cells. Nat. Cell Biol. 1, 362–368.
Rodriguez-Cruz, T. G., Liu, S., Khalili, J. S., Whittington, M., Zhang, M., Overwijk, W., and Lizee, G. (2011). Natural splice variant of MHC class I cytoplasmic tail enhances dendritic cell-induced CD8+ T-cell responses and boosts anti-tumor immunity. PLoS ONE 6, e22939. doi:10.1371/journal.pone.0022939
Roeth, J. F., Williams, M., Kasper, M. R., Filzen, T. M., and Collins, K. L. (2004). HIV-1 Nef disrupts MHC-I trafficking by recruiting AP-1 to the MHC-I cytoplasmic tail. J. Cell Biol. 167, 903–913.
Rosenberg, S. A., Yang, J. C., and Restifo, N. P. (2004). Cancer immunotherapy: moving beyond current vaccines. Nat. Med. 10, 909–915.
Salle de la, H., Hanau, D., Fricker, D., Urlacher, A., Kelly, A., Salamero, J., Powis, S. H., Donato, L., Bausinger, H., and Laforet, M. (1994). Homozygous human TAP peptide transporter mutation in HLA class I deficiency. Science 265, 237–241.
Sancho, D., Joffre, O. P., Keller, A. M., Rogers, N. C., Martinez, D., Hernanz-Falcon, P., Rosewell, I., and Reis e Sousa, C. (2009). Identification of a dendritic cell receptor that couples sensing of necrosis to immunity. Nature 458, 899–903.
Saveanu, L., Carroll, O., Weimershaus, M., Guermonprez, P., Firat, E., Lindo, V., Greer, F., Davoust, J., Kratzer, R., Keller, S. R., Niedermann, G., and van, E. P. (2009). IRAP identifies an endosomal compartment required for MHC class I cross-presentation. Science 325, 213–217.
Savina, A., Jancic, C., Hugues, S., Guermonprez, P., Vargas, P., Moura, I. C., Lennon-Dumenil, A. M., Seabra, M. C., Raposo, G., and Amigorena, S. (2006). NOX2 controls phagosomal pH to regulate antigen processing during crosspresentation by dendritic cells. Cell 126, 205–218.
Schlager, M. A., Kapitein, L. C., Grigoriev, I., Burzynski, G. M., Wulf, P. S., Keijzer, N., de, G. E., Fukuda, M., Shepherd, I. T., Akhmanova, A., and Hoogenraad, C. C. (2010). Pericentrosomal targeting of Rab6 secretory vesicles by bicaudal-D-related protein 1 (BICDR-1) regulates neuritogenesis. EMBO J. 29, 1637–1651.
Sheff, D. R., Daro, E. A., Hull, M., and Mellman, I. (1999). The receptor recycling pathway contains two distinct populations of early endosomes with different sorting functions. J. Cell Biol. 145, 123–139.
Shen, L., Sigal, L. J., Boes, M., and Rock, K. L. (2004). Important role of cathepsin S in generating peptides for TAP-independent MHC class I crosspresentation in vivo. Immunity 21, 155–165.
Shen, X. Z., Billet, S., Lin, C., Okwan-Duodu, D., Chen, X., Lukacher, A. E., and Bernstein, K. E. (2011). The carboxypeptidase ACE shapes the MHC class I peptide repertoire. Nat. Immunol. 12, 1078–1085.
Simonsen, A., Lippe, R., Christoforidis, S., Gaullier, J. M., Brech, A., Callaghan, J., Toh, B. H., Murphy, C., Zerial, M., and Stenmark, H. (1998). EEA1 links PI(3)K function to Rab5 regulation of endosome fusion. Nature 394, 494–498.
Singh, R., and Cresswell, P. (2010). Defective cross-presentation of viral antigens in GILT-free mice. Science 328, 1394–1398.
Sonnichsen, B., De, R. S., Nielsen, E., Rietdorf, J., and Zerial, M. (2000). Distinct membrane domains on endosomes in the recycling pathway visualized by multicolor imaging of Rab4, Rab5, and Rab11. J. Cell Biol. 149, 901–914.
Steinman, R. M., Mellman, I. S., Muller, W. A., and Cohn, Z. A. (1983). Endocytosis and the recycling of plasma membrane. J. Cell Biol. 96, 1–27.
Stelekati, E., Bahri, R., D’Orlando, O., Orinska, Z., Mittrucker, H. W., Langenhaun, R., Glatzel, M., Bollinger, A., Paus, R., and Bulfone-Paus, S. (2009). Mast cell-mediated antigen presentation regulates CD8+ T cell effector functions. Immunity 31, 665–676.
Teixeiro, E., Daniels, M. A., Hamilton, S. E., Schrum, A. G., Bragado, R., Jameson, S. C., and Palmer, E. (2009). Different T cell receptor signals determine CD8+ memory versus effector development. Science 323, 502–505.
Tomas, M. I., Kucic, N., Mahmutefendic, H., Blagojevic, G., and Lucin, P. (2010). Murine cytomegalovirus perturbs endosomal trafficking of major histocompatibility complex class I molecules in the early phase of infection. J. Virol. 84, 11101–11112.
Touret, N., Paroutis, P., Terebiznik, M., Harrison, R. E., Trombetta, S., Pypaert, M., Chow, A., Jiang, A., Shaw, J., Yip, C., Moore, H. P., van der, W. N., Houben, D., Peters, P. J., de, C. C., Mellman, I., and Grinstein, S. (2005). Quantitative and dynamic assessment of the contribution of the ER to phagosome formation. Cell 123, 157–170.
Traer, C. J., Rutherford, A. C., Palmer, K. J., Wassmer, T., Oakley, J., Attar, N., Carlton, J. G., Kremerskothen, J., Stephens, D. J., and Cullen, P. J. (2007). SNX4 coordinates endosomal sorting of TfnR with dynein-mediated transport into the endocytic recycling compartment. Nat. Cell Biol. 9, 1370–1380.
Trombetta, E. S., Ebersold, M., Garrett, W., Pypaert, M., and Mellman, I. (2003). Activation of lysosomal function during dendritic cell maturation. Science 299, 1400–1403.
Ueno, H., Huang, X., Tanaka, Y., and Hirokawa, N. (2011). KIF16B/Rab14 molecular motor complex is critical for early embryonic development by transporting FGF receptor. Dev. Cell 20, 60–71.
Urban, R. G., Chicz, R. M., Lane, W. S., Strominger, J. L., Rehm, A., Kenter, M. J., Uytdehaag, F. G., Ploegh, H., Uchanska-Ziegler, B., and Ziegler, A. (1994). A subset of HLA-B27 molecules contains peptides much longer than nonamers. Proc. Natl. Acad. Sci. U.S.A. 91, 1534–1538.
van der Wal, F. J., Kikkert, M., and Wiertz, E. (2002). The HCMV gene products US2 and US11 target MHC class I molecules for degradation in the cytosol. Curr. Top. Microbiol. Immunol. 269, 37–55.
Vega, M. A., and Strominger, J. L. (1989). Constitutive endocytosis of HLA class I antigens requires a specific portion of the intracytoplasmic tail that shares structural features with other endocytosed molecules. Proc. Natl. Acad. Sci. U.S.A. 86, 2688–2692.
Walseng, E., Bakke, O., and Roche, P. A. (2008). Major histocompatibility complex class II-peptide complexes internalize using a clathrin- and dynamin-independent endocytosis pathway. J. Biol. Chem. 283, 14717–14727.
Weigert, R., Yeung, A. C., Li, J., and Donaldson, J. G. (2004). Rab22a regulates the recycling of membrane proteins internalized independently of clathrin. Mol. Biol. Cell 15, 3758–3770.
Weimershaus, M., Maschalidi, S., Sepulveda, F., Manoury, B., van, E. P., and Saveanu, L. (2012). Conventional dendritic cells require IRAP-Rab14 endosomes for efficient cross-presentation. J. Immunol. 188, 1840–1846.
West, M. A., Wallin, R. P., Matthews, S. P., Svensson, H. G., Zaru, R., Ljunggren, H. G., Prescott, A. R., and Watts, C. (2004). Enhanced dendritic cell antigen capture via toll-like receptor-induced actin remodeling. Science 305, 1153–1157.
Wubbolts, R., Fernandez-Borja, M., Jordens, I., Reits, E., Dusseljee, S., Echeverri, C., Vallee, R. B., and Neefjes, J. (1999). Opposing motor activities of dynein and kinesin determine retention and transport of MHC class II-containing compartments. J. Cell Sci. 112(Pt 6), 785–795.
Yuseff, M. I., Reversat, A., Lankar, D., Diaz, J., Fanget, I., Pierobon, P., Randrian, V., Larochette, N., Vascotto, F., Desdouets, C., Jauffred, B., Bellaiche, Y., Gasman, S., Darchen, F., Desnos, C., and Lennon-Dumenil, A. M. (2011). Polarized secretion of lysosomes at the B cell synapse couples antigen extraction to processing and presentation. Immunity 35, 361–374.
Zhang, Q., Davis, J. C., Lamborn, I. T., Freeman, A. F., Jing, H., Favreau, A. J., Matthews, H. F., Davis, J., Turner, M. L., Uzel, G., Holland, S. M., and Su, H. C. (2009). Combined immunodeficiency associated with DOCK8 mutations. N. Engl. J. Med. 361, 2046–2055.
Zou, L., Zhou, J., Zhang, J., Li, J., Liu, N., Chai, L., Li, N., Liu, T., Li, L., Xie, Z., Liu, H., Wan, Y., and Wu, Y. (2009). The GTPase Rab3b/3c-positive recycling vesicles are involved in cross-presentation in dendritic cells. Proc. Natl. Acad. Sci. U.S.A. 106, 15801–15806.
Zuo, J., Quinn, L. L., Tamblyn, J., Thomas, W. A., Feederle, R., Delecluse, H. J., Hislop, A. D., and Rowe, M. (2011). The Epstein-Barr virus-encoded BILF1 protein modulates immune recognition of endogenously processed antigen by targeting major histocompatibility complex class I molecules trafficking on both the exocytic and endocytic pathways. J. Virol. 85, 1604–1614.
Keywords: cross-presentation, endosomal recycling compartment, Rab GTPase, microtubule-organizing center, endosomal remodeling
Citation: Compeer EB, Flinsenberg TWH, van der Grein SG and Boes M (2012) Antigen processing and remodeling of the endosomal pathway: requirements for antigen cross-presentation. Front. Immun. 3:37. doi: 10.3389/fimmu.2012.00037
Received: 09 December 2011; Accepted: 16 February 2012;
Published online: 07 March 2012.
Edited by:
Christian Kurts, Friedrich Wilhelms-Universität Bonn, GermanyReviewed by:
Veronika Lukacs-Kornek, Harvard Medical School, USACopyright: © 2012 Compeer, Flinsenberg, van der Grein and Boes. This is an open-access article distributed under the terms of the Creative Commons Attribution Non Commercial License, which permits non-commercial use, distribution, and reproduction in other forums, provided the original authors and source are credited.
*Correspondence: Marianne Boes, Department of Pediatric Immunology, Cellular Immunology laboratory, University Medical Center Utrecht / Wilhelmina Children’s Hospital, Utrecht University, Lundlaan 6, Utrecht, 3508 AB, Netherlands. e-mail:bS5sLmJvZXNAdW1jdXRyZWNodC5ubA==
Disclaimer: All claims expressed in this article are solely those of the authors and do not necessarily represent those of their affiliated organizations, or those of the publisher, the editors and the reviewers. Any product that may be evaluated in this article or claim that may be made by its manufacturer is not guaranteed or endorsed by the publisher.
Research integrity at Frontiers
Learn more about the work of our research integrity team to safeguard the quality of each article we publish.