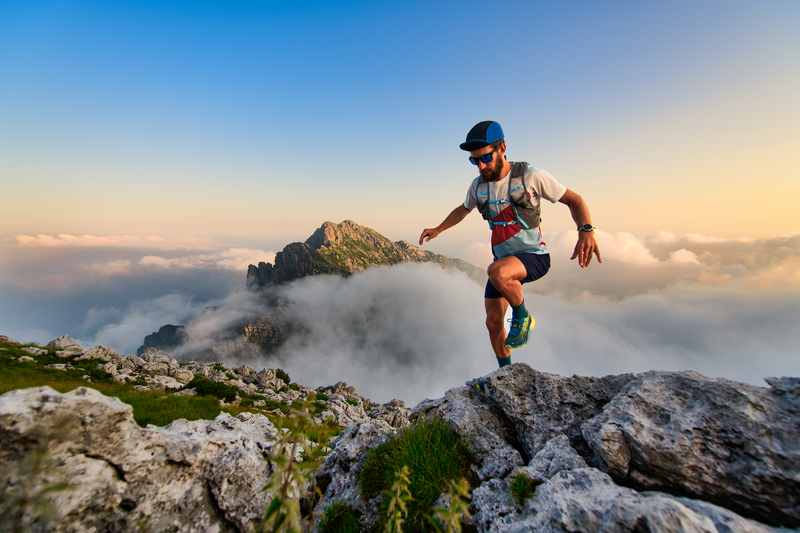
94% of researchers rate our articles as excellent or good
Learn more about the work of our research integrity team to safeguard the quality of each article we publish.
Find out more
REVIEW article
Front. Immunol. , 27 February 2012
Sec. Antigen Presenting Cell Biology
Volume 3 - 2012 | https://doi.org/10.3389/fimmu.2012.00026
This article is part of the Research Topic Molecular and cell-biological mechanisms of antigen cross-presentation View all 11 articles
Dendritic cells (DCs) are specialized antigen presenting cells that are exquisitely adapted to sense pathogens and induce the development of adaptive immune responses. They form a complex network of phenotypically and functionally distinct subsets. Within this network, individual DC subsets display highly specific roles in local immunosurveillance, migration, and antigen presentation. This division of labor amongst DCs offers great potential to tune the immune response by harnessing subset-specific attributes of DCs in the clinical setting. Until recently, our understanding of DC subsets has been limited and paralleled by poor clinical translation and efficacy. We have now begun to unravel how different DC subsets develop within a complex multilayered system. These findings open up exciting possibilities for targeted manipulation of DC subsets. Furthermore, ground-breaking developments overcoming a major translational obstacle – identification of similar DC populations in mouse and man – now sets the stage for significant advances in the field. Here we explore the determinants that underpin cellular and transcriptional heterogeneity within the DC network, how these influence DC distribution and localization at steady-state, and the capacity of DCs to present antigens via direct or cross-presentation during pathogen infection.
Dendritic cells (DCs) are a heterogeneous population of rare hematopoietic cells that are present in most tissues and are essential to the induction of both immunity and tolerance (Steinman and Witmer, 1978; Birnberg et al., 2008; Ohnmacht et al., 2009). They are organized as a specialized “network” that enables them to sample antigens from their environment which are then presented to other lymphocytes. As such, this elegant arm of the immune system is dedicated to shaping the immune response to peripheral antigens.
Dendritic cells are distinct from other immune cells as they are equipped with molecular machinery that enables them to very efficiently take up, process, and present antigens on major histocompatibility (MHC) class I and II molecules to T cells. In addition, they are equiped with a range of pathogen sensing molecules such as toll-like receptors (TLRs), nucleotide-binding oligomerization domain proteins, retinoid-inducible gene 1-like receptors, and C-type lectins that allow them to detect pathogen products and sense inflammation. Signaling through these receptors triggers migration of DCs from peripheral tissues to secondary lymphoid organs bringing DCs carrying antigens into close association with T cells. This pathway provides the critical link between the external environment (the major entry point for pathogens) and the sites where organized immune responses are induced, the lymph nodes (Randolph et al., 2008).
In recent years a number of distinct DC subsets have been defined. These subsets have been based largely on long standing criteria that relies on the expression of specific cell-surface markers. More recently, understanding the development and contributions of these DC subsets to immunity has been broadened significantly by insights to the ontogeny of the different subsets and the transcription factors that guide their development. Here we will highlight recent studies deciphering the transcriptional regulation that underpins DC heterogeneity which is critical in defense against pathogen infection.
On the October 3, 2011, DCs took center stage in the scientific world with the awarding of the Nobel Prize for Physiology or Medicine to Ralph Steinman together with Bruce Beutler and Jules Hoffmann for revolutionizing our understanding of the immune system by discovering key principles for its activation. Hoffman pioneered the discovery of sensing molecules in fruit flies enabling them to combat infection (Lemaitre et al., 1996), while Beutler uncovered the homologous receptors in mice that could detect pathogen products (Poltorak et al., 1998). More than a decade earlier, Ralph Steinman had discovered a rare cell type, the DC, within the immune system that had a unique capacity to efficiently activate immune cells (Steinman and Cohn, 1973). Since then, it has been shown that DCs express a wide range of innate receptors that enable pathogen sensing and the initiation of protective immunity.
Cardinal features of DCs are (i) their ability to efficiently take up and present self and pathogen-derived antigens to other cells of the immune system such as T cells and B cells, and (ii) their capacity to migrate from peripheral tissues such as skin and mucosa to secondary lymphoid tissues where they can activate lymphocytes and initiate the immune response. This migratory behavior is pivotal and provides a critical cellular link between the external environment where pathogens might enter the body and the secondary lymphoid tissues where immune responses are initiated.
A signature of DCs is their heterogeneity. The DC network is composed of multiple subtypes of DCs that vary in their origin, anatomical localization, lifespan, and function (Figure 1). Unraveling the developmental history of these subtypes has been complicated in part by the rarity of DCs in tissues (∼1% of cells) and their short lifespan. This was compounded by the early difficulties in establishing the growth factors and culture conditions necessary for generating large numbers in vitro. However, recent studies have made significant progress in clarifying a number of these steps.
Figure 1. Architecture of the DC network. The DC network is composed of multiple DC subsets that are broadly divided into cDCs, pDCs, MoDCs, and LCs which have distinct anatomical localizations in tissues. (A) Migratory DCs in peripheral tissues sample antigen from the periphery and then migrate through the lymphatic vessels to the afferent lymph node to present captured-processed antigens to the T cell within the T cell zone. In peripheral tissues, such as the skin, three main DC subsets are found. Dermal DCs are composed of the CD103+CD11b− DC and CD11b+ DC. Both arise from a pre-DC that homed to the tissue. Under conditions of inflammation, some CD11b+ DC can be derived from a monocytic precursor. In addition to the dermal DCs, the epidermis of the skin is populated by the LCs which are derived from a Ly6C+ progenitor. (B) In secondary lymphoid tissues such as spleen, CD4+, CD8α+, and CD4−CD8α− or double negative (DN) DCs are found. These subsets are also found in the draining lymph nodes which also receive the influx of the migratory CD11b+, CD103+CD11b− DCs, and LCs arriving from the peripheral tissues.
Simplistically, four major populations of DCs have been described (Figure 1), namely the conventional DCs (cDCs), plasmacytoid DCs (pDCs), Langerhans cells (LCs), and the monocyte-derived DCs (MoDCs).
Conventional DCs can be divided into two main groups of cells. They are (i) the migratory DCs and (ii) the lymphoid tissue-resident DCs (Figure 1).
Migratory DCs reside in peripheral tissues such as the skin and mucosa where they efficiently sample environmental antigens and then migrate from to the regional lymph node in afferent lymphatics to present antigens to T cells. They are composed of the dermal or interstitial DCs and can be divided into the CD11b+ and CD11b− DCs (Figure 1A). These DCs may also express the integrin αE, also known as CD103. CD103 is expressed on CD11b− DCs and can be found in a variety of others tissues. However, in intestinal tissues, CD103 is expressed on CD11b+ DCs. Despite a similarity in expression of surface molecules by these two DC subsets, the transcriptional machinery regulating these two populations is distinctly different as discussed below.
The second category of cDCs is composed of several subsets of DCs that are known as tissue-resident DCs. In contrast to their migratory counterparts, they do not circulate through peripheral tissues and thus can only process antigens found within the tissue in which they are localized (Figure 1B). To overcome this potentially limited access to antigen, migratory DCs can transfer antigens to lymphoid resident DCs who via the process of cross-presentation, provide an alternate strategy for the amplification of CD8+ T cell responses (Belz et al., 2004a; Allan et al., 2006).
Tissue-resident DCs are delineated by the expression of the surface molecules CD4 and CD8α and are found in secondary lymphoid organs such as the thymus, spleen, and lymph nodes. Three subsets have been defined which are (i) the CD4+ DCs, (ii) the CD8α+ DCs, and (iii) the CD4−CD8α− (double negative, DN) DCs. These subsets develop in situ from a common precursor generated in the bone marrow that homes to the lymphoid organs where they undergo further differentiation into mature DC subsets (Naik et al., 2006). Although there are a number of shared functions between these subsets, an interesting division of labor has emerged: CD8α+ DCs are highly efficient in direct and cross-presentation of soluble, cell-associated (Table 1), and pathogen-derived antigens to CD8+ T cells. Although CD4+ DCs and CD4−CD8α− DCs can also present MHC class I-restricted antigens in some settings (Kim and Braciale, 2009; Lukens et al., 2009). They are more potent in presenting MHC II antigens to CD4+ T cells (Allenspach et al., 2008; Mount et al., 2008).
The CD8α+ and CD103+ DCs are cDCs that are of special interest due to their shared functional attributes in driving immune responses to pathogen infections, their capacity to cross-present antigens, and the potential to harness the human equivalents of these subsets for clinical use (Steinman, 2010). What distinguishes these two subsets functionally and transcriptionally has thus been an area of intense investigation.
The CD8α+ DC subset. CD8α+ DCs are distinct from other conventional murine DC subsets by their unique surface expression of a CD8αα homodimer. This non-migratory, lymphoid tissue-resident DC has been shown to be key drivers of cross-presentation to a range of experimental pathogen antigens both in vitro and in vivo. CD8α+ DCs have been found to be critical for cross-presentation of self-antigens resulting in the induction of immune tolerance (Belz et al., 2002a; Heath et al., 2004). This subset was also identified as the main subset involved in presenting pathogen-derived antigens (Allan et al., 2003; Belz et al., 2004a,b, 2005; Lemos et al., 2004; Neuenhahn et al., 2006; Edelson et al., 2011). A number of mechanisms have been proposed to explain their constitutive ability to cross-present antigens. These include the expression of a number of surface receptors, such as CD36 and Clec9a, that allow them to uptake dead or dying cells, specialized intracellular pathways, and regulation of the pH in the phagosome (Table 1). This antigen presenting function and their production of interleukin-12 (IL-12) distinguish the CD8α+ DCs from their CD8α− counterparts. Although initially cross-presentation by CD8α+ DCs was thought to be a unique property restricted solely to this subset, it is now clear that other DC subsets can act as potent cross-presenting cells (i.e., CD103+ DC subset and MoDCs, Table 1).
The CD103+ DC subset. This unusual DC subset that appeared to be related to CD8α+ DCs was first described in the influenza infection and was characterized by the lack of expression of CD8α and CD11b (CD8α−CD11b−; Belz et al., 2004b). It was found in a number of lymph nodes including the inguinal, brachial, superficial cervical, mediastinal, mesenteric, hepatic, and renal nodes. This DC subset presents viral antigens very efficiently suggesting that CD8α−CD11b− DCs may play an important role in T cell mediated immunity. It was postulated that these cells could traffic to the lymph nodes, thereby providing a critical link between antigen sampling in peripheral tissues and antigen presentation in the lymph node. Furthermore it was speculated that these cells would be capable of cross-presentation. Following this early report, it was discovered that these DCs expressed the molecules integrin αE (CD103) and langerin (Sung et al., 2006). Following on from the establishment of the importance of CD8α+ DC in promoting viral immunity (Allan et al., 2003), CD103+ (langerin+) dermal DCs, and lung CD103+ DCs have emerged as a potent migratory DC able to process and load self- and viral antigens onto MHC class I molecules (Sung et al., 2006; Bedoui et al., 2009).
Plasmacytoid DCs express several key molecules that distinguish them from the cDC subsets. These include the sialic acid-binding immunoglobulin like lectin H (Siglec-H), Lilra4 (also known as ILT7), bone marrow stromal antigen-2 (BST2, also known as tetherin) and blood DC antigen-2 (BDCA-2, also known as CLEC4C), and the CD45RA isoform (Reizis et al., 2011). The hallmark property of pDCs is their capacity to rapidly secrete type I interferons (IFN-α) during viral infection. This is in part attributable to their expression of TLR7 and TLR9 that enables sensing of nucleic acids during viral infection. The antigen presentation potency of pDCs is poor and although this can be enhanced during maturation (Colonna et al., 2004), their low expression of MHC and co-stimulatory molecules compared to their cDCs counterparts provide some explanation for their inefficiency in priming of T cells.
Langerhans cells are a unique DC subset found within the epidermis of the skin and mucosa and contain large tennis racquet shaped granules known as Birbeck granules of which langerin is a crucial component. Initially, it was thought that langerin expression was restricted solely to LCs, but it has more recently been discovered that DC subsets located in the lamina propria of skin, bronchi, and mucosa also express langerin (Bursch et al., 2007; Ginhoux et al., 2007; Poulin et al., 2007). Despite sharing the expression of langerin, other langerin+ DCs can arise along a different developmental pathway distinct from LCs (Merad et al., 2008). LCs develop from a local LY6C+ myelo-monocytic precursor cell population, and their development is dependent on TGF-β signaling (Chorro et al., 2009; Nagao et al., 2009), while langerin+ DC develop from a bone marrow precursor that is dependent on Fms-like tyrosine kinase 3 (Flt3) signaling. LCs are exquisitely positioned to provide the front line of defense of the immune system against external invading pathogens. As LCs are the only cells that express MHC II in the epidermis under non-inflammatory conditions and since they are able to migrate through the dermis to the skin-draining lymph nodes, LCs have been believed to be critical to promote immunity, i.e., after skin infection.
This classical paradigm has been recently challenged. LCs are largely resistant to γ-irradiation, a characteristic that has been taken advantage of in deciphering the immunological relevance of LC over dermal DC in chimeric mice. This feature has allowed the generation of elegant chimeric mice in which LCs could be of host origin while dermal and other DCs were of donor origin. Thus the individual function of LCs could be addressed. LCs were found to be unable to initiate T cell dependent immunity when challenged with a herpes simplex virus (HSV) type 1 skin infection (Allan et al., 2003). Similarly, LCs from the vaginal mucosa are not able to present HSV type 2-derived antigens to CD4+ T cells (Zhao et al., 2003). Although the biological function of LCs in vivo still raises much debate, it is now clearer that a major role of LCs is in maintaining immune tolerance (Shklovskaya et al., 2011).
Under non-inflammatory conditions, monocytes can give rise to CD11b+ MoDCs in non-lymphoid organ, and their development is in part dependent on MCSF-R signaling (Bogunovic et al., 2009; Ginhoux et al., 2009). However the contribution of monocytes to the DC pool is by far more important under inflammatory condition. Pathogen recognition by CD11c−MHCII− blood monocytes leads to their differentiation into CD11c+MHCII+ MoDCs that express GR1/LY6C pointing to their monocytic origin (Leon et al., 2004; Hohl et al., 2009; Nakano et al., 2009), becoming thereafter the dominant DC population. Until recently, the contributions of MoDCs to pathogen infections have been largely ignored. Recent detailed analyses of their antigen-capturing, processing, and presentation capacity revealed that they are strikingly efficient at both direct and cross-presentation of antigens and at least under certain conditions as good as CD8α and CD103+ DCs (Cheong et al., 2010). Under inflammatory conditions, MoDCs have been found to be the main population presenting antigens in the T cell area (Ingersoll et al., 2011). Hence, blood monocytes serve as an emergency reservoir of antigen presenting cells that efficiently recognize pathogens and their associated danger signals and rapidly induce specialized antigen presentation machinery. Based on the short kinetic of differentiation observed in vivo, it is likely that so called “DC genes” are poised to enable rapid differentiation into MoDCs to efficiently counter pathogen attack. The characterization of new markers that clearly identify MoDCs from cDCs in inflamed tissues will open the door for a better understanding of the molecular mechanisms that drive monocyte differentiation into MoDCs (Cheong et al., 2010; Siddiqui et al., 2010).
The hematopoietic system is hierarchically organized (Figure 2). Long-term repopulating hematopoietic stem cells (HSC) constantly self renew but can also give rise to short-term repopulating cells that have lost much of their self-renewal capacity. These precursors differentiate further into the multi-lineage progenitor (MPP). MPP can give rise to both the common-lymphoid progenitor (CLP) and the common myeloid progenitor (CMP). Both CLP and CMP can differentiate into several DC subsets (Manz et al., 2001). This dual origin of DCs has provoked controversy in the field. However, recent studies have clarified the origin of DCs. Under steady-state conditions, the two main populations of DCs, the cDCs and pDCs, have both been shown to arise from a common dendritic cell precursor (also called the CDP) found in the bone marrow. CDPs are devoid of macrophage potential and exclusively give rise to DCs in vitro and in vivo (Naik et al., 2007; Onai et al., 2007). Once generated CDPs develop either into pDCs in the bone marrow, or give rise to pre-cDCs that are able to migrate from bone marrow to lymphoid and non-lymphoid organs to further mature into cDCs (Naik et al., 2006). The CDP is thought to originate from a bipotential macrophage/DC progenitor (MDP) that can generate either DCs or macrophages (Fogg et al., 2006).
Figure 2. Ontogeny of DC precursors. Short-term HSCs commit into multipotent progenitors that give rise to either a common-lymphoid progenitor (CLP) or a common myeloid progenitor (CMP). A population that lies downstream of CMP has been found to differentiate either into DC or macrophages, and was therefore named the macrophage-dendritic cell progenitor (MDP). Full commitment to the DC lineage is acquired at the CDP stage (common DC progenitor) where a CDP can either differentiate into plasmacytoid DCs (pDCs) or into a pre-DC. The latter will further differentiate into mature conventional DCs (cDCs) in the peripheral tissues, or secondary lymphoid organs.
Development of early DC precursors is directed by the interplay between extrinsic cytokine signals such as Flt3, macrophage colony-stimulating factor (M-CSF), granulocyte-macrophage colony-stimulating factor (GM-CSF), and transcription factors PU.1, Ikaros, and Gfi-1. A key determinant of the development of DC precursors at steady-state is the Flt3:Flt3L pathway. Mice that lack Flt3L, or its receptor Flt3, have a severe reduction in conventional and pDC subsets (McKenna, 2001; Waskow et al., 2008). Conditional expression, or exogenous administration of Flt3L, results in a dramatic expansion of DCs in secondary lymphoid tissues (Maraskovsky et al., 1996; Manfra et al., 2003). Thus, Flt3 and Flt3L are pivotal in driving DC ontogeny. Coordinate regulation of Flt3 signaling is mediated through Stat3 but is dispensable for differentiation of DCs via GM-CSF signaling which is important for driving the differentiation of monocyte-derived DC development in an inflammatory setting (Laouar et al., 2003; Onai et al., 2006).
The growth factor M-CSF is well known to promote the development of macrophages and monocytes. The first example for a function of M-CSF in DC development was uncovered by Ginhoux et al. (2006), who found that mice lacking the M-CSFR failed to generate LCs, highlighting the critical role of M-CSFR/M-CSF axis in the generation of this unique DC subset. Although this pathway is thought to be dispensable for the generation of cDC and pDC, recently, a novel role for M-CSF in DC development has been uncovered. pDCs and cDCs can be generated from Flt3L−/− bone marrow cells when cultured in presence of M-CSF (Fancke et al., 2008). In addition the injection of M-CSF into mice leads to increased pDCs and cDCs numbers. Nevertheless, the effect of M-CSF on steady-state DCs (except from the LC) is modest when compared with the influence of Flt3 in steady-state DC development. The M-CSFR/M-CSF axis would be predicted to be more critical in the generation of MoDCs during a pathogen attack, but this pathway remains to be fully elucidated.
The differentiation of hematopoietic precursor cells into different DC lineages is a well orchestrated process controlled in large part by transcription factors that are modulated by extracellular cues such as cytokines. The transcriptional networks that guide the development of B and T cells, particularly in early hematopoiesis, are relatively well defined. In contrast, the transcription factors that specifically regulate DC differentiation have only now begun to be elucidated. This has been facilitated by the use of lineage-specific knockout mice and elegant lineage tracing approaches.
These transcription factors can be broadly divided into those that are required or act early in DC development in DC progenitors (Gfi-1, Pu.1), and those whose major actions affect DC subset specification late in DC development (E2.2, Spi-B, IRF8, Id2, Nfil3, Batf3).
Gfi-1 is a zinc-finger critical for DC ontogeny. Mice lacking Gfi-1 show a global reduction in DC numbers but increased frequency of LCs (Rathinam et al., 2005). Interestingly, Gfi-1−/− bone marrow progenitors cultured in vitro in presence of Flt3L or GM-CSF fail to produce DC and instead develop into macrophages. Gfi-1 antagonizes PU.1 activity through direct protein interaction and it seems plausible that the aberrant macrophage potential observed in Gfi-1−/− bone marrow progenitors reflects failed repression of PU.1 binding to its positive regulatory elements, resulting in a macrophage rather than a DC fate (Spooner et al., 2009).
PU.1 (encoded by the gene Sfpi1) belongs to the Ets family of transcription factors. Until recently, its role in DCs has been unclear due to disparate studies – the first reporting a deficit in DC differentiation (Anderson et al., 2000) and the second reporting apparently normal DC development in the absence of PU.1 (Guerriero et al., 2000). These studies could not distinguish whether PU.1 played a role at the stage of DC commitment or whether its main action was in multipotent progenitors. Conditional deletion of PU.1 at different stages of DC development highlighted the requirement of PU.1 for DC commitment as differentiation was abrogated in absence of PU.1 (Carotta et al., 2010). Furthermore, PU.1 lies upstream of FLT3 and GM-CSFR and is required for the development of DCs via both pathways. Thus, PU.1 is a central player in the generation of both steady-state DCs and presumably inflammatory DCs although the complex interactions of PU.1 in coordinating DC differentiation remain to be fully explored.
While a number of transcription factors delineate the development of precursor cells that ultimately give rise to DCs, specification of individual DC subset identity appears to be a late event that is guided a handful of transcription factors (see Table 2). These can be broadly divided into three pathways of regulation – those transcription factors that predominantly influence (i) the pDCs (namely E2-2 and Spi-B), (ii) the CD8α+ and CD103+ lineages (IRF8, Id2, EB4BP, and Batf3), and (iii) the non-CD8α+ DC lineages (IRF2, IRF4).
E proteins constitute a family of basic helix-loop-helix (bHLH) transcription factor whose function have been most clearly defined in B lymphocytes (de Pooter and Kee, 2010). The expression of E proteins is modulated by inhibitor of DNA-binding (ID) proteins which can bind E proteins to prevent their binding to DNA targets. To date three mammalian E proteins have been described (E2a, HEB, E2-2) that act on their targeted sequence (CACCTG E-box) either as homodimers or heterodimers. The first indication that E proteins played important roles in pDC differentiation came from the study by Spits et al. (2000), where the overexpression of Id proteins, which sequester E proteins, impaired in vitro pDC development but left cDC differentiation intact. Subsequent analysis uncovered that E2-2, encoded by the gene Tcf4, was a key determinant of pDC differentiation. Germline or conditional deletion of E2-2 led to a complete loss of the pDC and abolished the ability of mice to respond to unmethylated DNA (Cisse et al., 2008). Through the binding of E2-2 to many pDC signature genes (Irf8, Irf7, SpiB, BDCA-2) it has been proposed as a master regulator of the pDC compartment. In line with this hypothesis, the depletion of E2-2 in mature pDC led to a predominance of cDC-like cells implying that E2-2 plays a pivotal role in maintaining pDC cell fate (Ghosh et al., 2010). However, in this setting it is difficult to separate the contribution of expansion of the pre-existing cDCs as molecular analyses showed enrichment of the conventional DC genes rather than a pure signature. Nevertheless, pDCs are particularly sensitive to E2-2 concentration as both E2-2-deficient mice and rare patients with haploinsufficiency (Pitt–Hopkins syndrome) show impaired pDC formation and function (Cisse et al., 2008).
A second transcription factor that influences the development of pDCs is Spi-B, a member of Ets family transcription factor (Schotte et al., 2004). In contrast to the loss of PU.1, deficiency of Spi-B affects only the pDC compartment. Interestingly, over expression of Spi-B in human pDCs impairs Id2 expression and consequently E2-2 activity is enhanced suggesting that Id2 is a key regulator of the cDC/pDC balance (Nagasawa et al., 2008). However, the capacity for E2-2 to promote pDC differentiation was abolished in these DC when Spi-B expression was ablated showing that Spi-B and E2-2 are jointly necessary to efficiently drive development of human precursors into the pDC lineage (Nagasawa et al., 2008).
Interferon regulatory factor 8 (also called the interferon consensus sequence-binding protein, or ICSBP) regulates the development of both cDCs and pDCs and is highly expressed on the CDP. Mice deficient in IRF8 lack many mature DC subsets including CD8α+ DCs and LCs (Schiavoni et al., 2002, 2004). In addition, IRF8 controls functional features such as TLR9 and IFN-α production in pDCs and IL-12 production in CD8α+ DCs (Tailor et al., 2008). Irf8−/− mice are more susceptible to viral infection but also develop a myeloproliferative syndrome characterized by overproduction of granulocytes (Holtschke et al., 1996). This suggests that while IRF8 is important for conventional DC development, it may also be required for the generation or maintenance of the upstream MDP progenitor that can give rise to monocytes, cDCs, and pDCs.
The expression of Id2 is highest in CD8α+ and CD103+CD11b− DCs but it is nevertheless broadly expressed by all conventional DC subsets (Ginhoux et al., 2009; Jackson et al., 2011). Loss of Id2 results in the failure of these two subsets, CD8α+ and CD103+ DCs, to develop in skin-draining lymph nodes and spleen. CD103+CD11b+ DCs found in the lymph nodes draining the gut together with CD4+ and CD4−CD8α− DCs in lymphoid tissues, appear to develop normally (Hacker et al., 2003; Ginhoux et al., 2009; Edelson et al., 2010). Through its action in regulating E protein binding, Id2 appears to plays a pivotal role in the maintenance of conventional DC identity. Multiple E proteins are expressed by DCs so precisely how these are regulated, and which E proteins (E2A, E2-2, or HEB) are the critical targets of Id2 is unclear. Understanding the balance between E proteins and Id proteins in cDCs will be required to understand why Id2 plays such an key role in CD8α+ and CD103+CD11b− DCs and how differentiation of such subsets might be enhanced.
E4BP4 is a mammalian basic leucine zipper (bZIP) transcription factor that is required for the development of NK cells, and is a key regulator of cytokine production in other hematopoietic lineages (Gascoyne et al., 2009; Kamizono et al., 2009; Kashiwada et al., 2011; Kobayashi et al., 2011). In NK cells E4BP4 acts in a dose dependent manner downstream of the IL-15 receptor and regulates Id2 expression (Gascoyne et al., 2009; Kamizono et al., 2009). Thus, it has been found that induction of E4BP4 is important for the development of CD8α+ DCs (Kashiwada et al., 2011). E4BP4−/− mice exhibit impaired IL-12 production upon TLR3 activation, and failed to cross-prime CD8+ T cells against cell-associated antigens. CDPs deficient in E4BP4 displayed reduced BATF3 expression and enforced expression in these cells rescued CD8α+ DCs development in vitro (Kashiwada et al., 2011). Thus, E4BP4 is emerging as an important regulator of conventional DC development.
Batf3 is a bZIP transcription factor (also known as Jun dimerization protein p21SNFT) that represses NFAT–AP1 activity by competing with FOS for JUN dimerization (Dorsey et al., 1995; Echlin et al., 2000). It provoked enormous interest in the DC field when it was shown to play a critical role in DC differentiation. Indeed, it was the first description of a transcription factor that appeared to have an exclusive role in the development of the CD8α+ DC subset. The fact that deletion of Batf3 resulted in increased susceptibility to various pathogen infections such as West Nile virus, influenza virus, Listeria monocytogenes, and Toxoplasma gondii (Hildner et al., 2008; Desch et al., 2011; Edelson et al., 2011; Mashayekhi et al., 2011) and critically impaired cross-priming was striking (Hildner et al., 2008). More recently it has been described to be involved in the development of migratory cross-presenting CD103+CD11b− DCs in peripheral lymphoid tissues (Edelson et al., 2010), but not in gut lymphoid tissue CD103+CD11b+ DCs (Bar-On et al., 2010). However, although Batf3−/− mice show a reduction in the frequency of CD8α+ DCs, particularly in the spleen, it is now clear that CD8α+ DCs are indeed present in the absence of Batf3 (Edelson et al., 2011; Jackson et al., 2011). Thus in contrast to the early suggestions, Batf3 appears to exclusively regulate the development of CD103+ DC while CD8α+ DC precursors develop although they seem unable to cross-present exogenous antigens to CD8+ T cells. This suggests that some stages in DC maturation may be perturbed in the absence of Batf3.
Until recently, the capacity to cross-present antigens was a feature attributed almost exclusively to CD8α+ and CD103+ DCs. This distinction gained some foothold with the notion that particular DC subsets possess unique molecular machinery intrinsic to these subsets exclusively enabling them to cross-present (Schnorrer et al., 2006). However, in other settings, it was clear that non-CD8α+ lineage cells could also perform such functions. This was poignantly highlighted by the systematic characterization of the capacity for MoDCs to efficiently cross-present antigens, particularly in the inflammatory setting, demonstrated that induction of cross-presenting machinery could occur in different DC subsets when subjected to specific stimuli (Cheong et al., 2010).
Non-CD8α+ DC lineages are composed of DN and CD4+ DCs together with MoDCs. Much less is known about the transcription factors that regulate the fate decisions of these subsets although it is clear that at least the conventional subsets in this group are significantly influenced by IRF2 (Hida et al., 2000; Honda et al., 2004; Ichikawa et al., 2004; Arakura et al., 2007), IRF4 (Suzuki et al., 2004; Tamura et al., 2005), and RelB (Burkly et al., 1995; Wu et al., 1998). At least some of these pathways are also likely to regulate MoDCs. This could potentially happen through the role of IRF4 in coordinating signals from GM-CSF stimulation through the NF-κB pathway (Gilliet et al., 2002). It will be important to dissect the network of transcription factors that drive not only CD8α− DC development but also function. This latter feature seems particularly important where a property such as cross-presentation is inducible and transcription factors such as Id2 and Batf3 are broadly expressed in the non-CD8α− DC lineages but not obviously required for the development of these subsets. They may indeed be important for maturation or localization of DCs.
Key steps in understanding DC subsets has come from detailed phenotypic characterization made possible through the development of novel antibodies combined with the power of flow cytometry. These descriptions have been significantly extended by elucidation of the anatomical localization of particular DC subset, the precursors from which they arise and the identification of unique or restricted functions that can now be attributed to particular DC subsets. For example, CD8α+ and CD103+ DCs are the dominant DC subsets that cross-present soluble and cell-associated antigens and present viral antigens to CD8+ T cells (den Haan and Bevan, 2002; Allan et al., 2003; Belz et al., 2004a,b, 2005; Bedoui et al., 2009). While a number of seminal studies that have identified a new DC subset or function are critical to the development of the field, they have not always led to clarification of the contributions of DCs, or DC subsets, to immunoregulation of responses. This arises largely because surface markers or receptors are expressed by multiple DC subsets and in many instances, a broader range of immune cells. Thus, with regard to the capacity to cross-present antigens, MoDCs have recently also been discovered to be very efficient in shuttling antigen into this pathway, particularly in an inflammatory setting (Cheong et al., 2010).
One approach to this problem is to focus on the molecular wiring of cells – that is, the transcription factors – to develop a definitive map of DC identity (Satpathy et al., 2011). This approach indeed offers a way forward. However, deletion of specific DC populations is premised on the notion that presence or absence of a transcription factor results in a complete loss or alternately, exaggeration of DC subsets or their functions. Loss of some transcription factors such as Irf4, Irf8, and Id2 seem to demonstrate relatively definitive outcomes with complete loss of some DC subsets. Certainly, mice engineered to allow conditional deletion of a gene in a cell-specific manner (e.g., driven by CD11c) allows analysis of the intrinsic requirement for factors allows delineation of phenotypes that may arise due to extrinsic signals. Similar approaches have also been used where a cell-specific promoter drives conditional deletion of a cell type. A key example of this is the development of mice in which CD11c+ cells can be deleted by treating them with diptheria toxin (CD11c–DTR mice; Jung et al., 2002). This provides an elegant model for examination of cDCs but it was quickly realized that other cell types (such as inflamed lung epithelial cells) expressed CD11c and therefore generation of bone marrow chimeric mice was necessary to fully utilize this model without confounding effects (Sapoznikov and Jung, 2008).
In biology, this “black and white” model seldom reflects reality and it is not clear that DC differentiation into subsets is a purely linear process. Loss of the ability to detect a DC subset depends mainly on the same tools we have used to identify them in the first instance. These approaches do not generally distinguish between two important stages of developmental arrest – firstly, complete ablation of a lineage, and secondly, the formation of the immediate precursors of that lineage that then fail to receive the necessary signals for DC progenitors to develop into fully matured cells in the peripheral tissues. The former situation identifies factors that are definitively required for the formation of a DC subset; the latter allows detailed dissection of the factors that influence the maturation and survival of DC subsets in the periphery. Distinguishing between these two cases is facilitated by the use of genetically modified mice in which a transcription factor is linked to a fluorescent reporter allowing rare precursor cells to be identified when most of the population does not survive to maturity. This was first identified in mice expressing green fluorescent protein linked to Ror(c)t, an important transcription factor for the development of lymphoid-tissue inducer (LTi) cells (Schmutz et al., 2009; Chappaz and Finke, 2010). Treatment of these reporter mice with IL-7/IL-7R mAb complexes revealed that progenitor cells were in fact formed in the absence of Ror(c)t but they did not develop into cells that could colonize and form secondary lymphoid tissues due to impaired IL-7 signaling. Similarly, Batf3 was initially described (Hildner et al., 2008) to unequivocally regulate the development of CD8α+ DCs and was later discovered to also regulate CD103+ DCs (Edelson et al., 2010). By analyzing the expression patterns of ID2 in Batf3−/− mice using the Id2GFP/GFP strain (Jackson et al., 2011), it was discovered that both in vitro and in vivo CD8α+ DC precursors do develop in the absence of Batf3 although their persistence, particularly in spleen, was diminished (Edelson et al., 2011; Jackson et al., 2011). These two examples highlight that careful dissection of phenotypes is critical and that complementary methods to analyze the expression of transcription factors and consequences of their loss provides a real strength in tackling the difficult questions of the future.
Overall, the factors that determine differentiation patterns of individual DC subsets with unique functions are complex. Establishment of the features that define an individual DC subset’s identity, their maintenance and differentiation is unlikely to be simplistic and rely on a single phenotypic marker or transcription factor. A more likely scenario is that DC subset development is determined by combinatorial interactions between transcription factors to guide fate decisions (Lin et al., 2010; Wilson et al., 2010). Analyses of genome wide DNA-binding data will be critical for understanding precisely how these different transcription factors work together to define the different populations of DCs that are necessary to provide protective immunity.
Our current understanding of DC commitment and differentiation at a steady-state and during pathogen infection is limited, especially when compared to the extensive understanding of regulatory networks that guide fate decisions in B cells and CD4+ T cells. In addition, the mechanisms that guide the emergence inflammatory DCs and the induction of protective cross-presenting machinery offer a fertile field for discovery in the future. Collectively, understanding the complex and multilayered circuitry that integrates DC location, expression of surface molecules, components of the antigen processing and presentation machinery together with and understanding of the molecular wiring, and potential rewiring of DC subsets will be essential. This information will lead to a more complete picture of the roles of DCs and the DC network in maintaining immune tolerance and immunity and may prove pivotal to improvements in the clinical efficacy of therapeutic approaches. Solving the following, and other, critical questions in the field of DC biology will be instrumental in progressing discoveries in the field.
1. Do individual transcription factors, or alternately, combinatorial transcriptional programs involving networks of transcription factors determine DC subset specification?
2. How do DC subsets that emerge during inflammatory setting reflect those identified at steady-state?
3. How is the constitutive cross-presenting machinery that maintains tolerance through presentation of self-antigens at steady-state, and is dominant in CD8α+ and CD103+ DCs during viral infection, induced in multiple DC subsets during inflammation or pathogen challenge?
4. Can this inductive process be harnessed and how?
5. How does the MDP engage the “DC program”?
6. How does DC lifespan affect immunity?
7. How is developmental plasticity is maintained to ensure immunity?
The challenge now is to develop the skill sets, tools, and technologies that will enable us to embark on dissection of these core features of DCs to open the pathway for targeted use of this cell type.
The authors declare that the research was conducted in the absence of any commercial or financial relationships that could be construed as a potential conflict of interest.
The authors thank Y. Zhan, M. Pang, S. Nutt, and A. Kallies for helpful discussions and critical reading of the manuscript. This work is supported by the National Health and Medical Research Council (NHMRC) of Australia. Gabrielle T. Belz is supported by a Sylvia and Charles Viertel Foundation Fellowship and Rhys S. Allan is supported by an NHMRC International Postdoctoral Fellowship. This work was made possible through Victorian State Government Operational Infrastructure Support and Australian Government NHMRC IRIIS.
APCs, antigen presenting cells; DCs, dendritic cells; HSV, herpes simplex virus; IL, interleukin; LP, lamina propria; MHC, major histocompatibility complex; n.d., not determined.
Albert, M. L., Pearce, S. F., Francisco, L. M., Sauter, B., Roy, P., Silverstein, R. L., and Bhardwaj, N. (1998). Immature dendritic cells phagocytose apoptotic cells via alphavbeta5 and CD36, and cross-present antigens to cytotoxic T lymphocytes. J. Exp. Med. 188, 1359–1368.
Aliberti, J., Schulz, O., Pennington, D. J., Tsujimura, H., Reis E Sousa, C., Ozato, K., and Sher, A. (2003). Essential role for ICSBP in the in vivo development of murine CD8alpha + dendritic cells. Blood 101, 305–310.
Allan, R. S., Smith, C. M., Belz, G. T., Van Lint, A. L., Wakim, L. M., Heath, W. R., and Carbone, F. R. (2003). Epidermal viral immunity induced by CD8alpha+ dendritic cells but not by Langerhans cells. Science 301, 1925–1928.
Allan, R. S., Waithman, J., Bedoui, S., Jones, C. M., Villadangos, J. A., Zhan, Y., Lew, A. M., Shortman, K., Heath, W. R., and Carbone, F. R. (2006). Migratory dendritic cells transfer antigen to a lymph node-resident dendritic cell population for efficient CTL priming. Immunity 25, 153–162.
Allenspach, E. J., Lemos, M. P., Porrett, P. M., Turka, L. A., and Laufer, T. M. (2008). Migratory and lymphoid-resident dendritic cells cooperate to efficiently prime naive CD4 T cells. Immunity 29, 795–806.
Allman, D., Dalod, M., Asselin-Paturel, C., Delale, T., Robbins, S. H., Trinchieri, G., Biron, C. A., Kastner, P., and Chan, S. (2006). Ikaros is required for plasmacytoid dendritic cell differentiation. Blood 108, 4025–4034.
Anderson, K. L., Perkin, H., Surh, C. D., Venturini, S., Maki, R. A., and Torbett, B. E. (2000). Transcription factor PU.1 is necessary for development of thymic and myeloid progenitor-derived dendritic cells. J. Immunol. 164, 1855–1861.
Arakura, F., Hida, S., Ichikawa, E., Yajima, C., Nakajima, S., Saida, T., and Taki, S. (2007). Genetic control directed toward spontaneous IFN-alpha/IFN-beta responses and downstream IFN-gamma expression influences the pathogenesis of a murine psoriasis-like skin disease. J. Immunol. 179, 3249–3257.
Bar-On, L., Birnberg, T., Lewis, K. L., Edelson, B. T., Bruder, D., Hildner, K., Buer, J., Murphy, K. M., Reizis, B., and Jung, S. (2010). CX3CR1+ CD8alpha+ dendritic cells are a steady-state population related to plasmacytoid dendritic cells. Proc. Natl. Acad. Sci. U.S.A. 107, 14745–14750.
Bedoui, S., Whitney, P. G., Waithman, J., Eidsmo, L., Wakim, L., Caminschi, I., Allan, R. S., Wojtasiak, M., Shortman, K., Carbone, F. R., Brooks, A. G., and Heath, W. R. (2009). Cross-presentation of viral and self antigens by skin-derived CD103+ dendritic cells. Nat. Immunol. 10, 488–495.
Belz, G. T., Behrens, G. M., Smith, C. M., Miller, J. F., Jones, C., Lejon, K., Fathman, C. G., Mueller, S. N., Shortman, K., Carbone, F. R., and Heath, W. R. (2002a). The CD8alpha(+) dendritic cell is responsible for inducing peripheral self- tolerance to tissue-associated antigens. J. Exp. Med. 196, 1099–1104.
Belz, G. T., Vremec, D., Febbraio, M., Corcoran, L., Shortman, K., Carbone, F. R., and Heath, W. R. (2002b). CD36 is differentially expressed by CD8+ splenic dendritic cells but is not required for cross-presentation in vivo. J. Immunol. 168, 6066–6070.
Belz, G. T., Shortman, K., Bevan, M. J., and Heath, W. R. (2005). CD8alpha+ dendritic cells selectively present MHC class I-restricted noncytolytic viral and intracellular bacterial antigens in vivo. J. Immunol. 175, 196–200.
Belz, G. T., Smith, C. M., Eichner, D., Shortman, K., Karupiah, G., Carbone, F. R., and Heath, W. R. (2004a). Cutting edge: conventional CD8 alpha+ dendritic cells are generally involved in priming CTL immunity to viruses. J. Immunol. 172, 1996–2000.
Belz, G. T., Smith, C. M., Kleinert, L., Reading, P., Brooks, A., Shortman, K., Carbone, F. R., and Heath, W. R. (2004b). Distinct migrating and nonmigrating dendritic cell populations are involved in MHC class I-restricted antigen presentation after lung infection with virus. Proc. Natl. Acad. Sci. U.S.A. 101, 8670–8675.
Birnberg, T., Bar-on, L., Sapoznikov, A., Caton, M. L., Cervantes-Barragan, L., Makia, D., Krauthgamer, R., Brenner, O., Ludewig, B., Brockschnieder, D., Riethmacher, D., Reizis, B., and Jung, S. (2008). Lack of conventional dendritic cells is compatible with normal development and T cell homeostasis, but causes myeloid proliferative syndrome. Immunity 29, 986–997.
Bogunovic, M., Ginhoux, F., Helft, J., Shang, L., Hashimoto, D., Greter, M., Liu, K., Jakubzick, C., Ingersoll, M. A., Leboeuf, M., Stanley, E. R., Nussenzweig, M., Lira, S. A., Randolph, G. J., and Merad, M. (2009). Origin of the lamina propria dendritic cell network. Immunity 31, 513–525.
Burgdorf, S., Lukacs-Kornek, V., and Kurts, C. (2006). The mannose receptor mediates uptake of soluble but not of cell-associated antigen for cross-presentation. J. Immunol. 176, 6770–6776.
Burkly, L., Hession, C., Ogata, L., Reilly, C., Marconi, L. A., Olson, D., Tizard, R., Cate, R., and Lo, D. (1995). Expression of relB is required for the development of thymic medulla and dendritic cells. Nature 373, 531–536.
Bursch, L. S., Wang, L., Igyarto, B., Kissenpfennig, A., Malissen, B., Kaplan, D. H., and Hogquist, K. A. (2007). Identification of a novel population of Langerin+ dendritic cells. J. Exp. Med. 204, 3147–3156.
Caminschi, I., Proietto, A. I., Ahmet, F., Kitsoulis, S., Shin Teh, J., Lo, J. C., Rizzitelli, A., Wu, L., Vremec, D., Van Dommelen, S. L., Campbell, I. K., Maraskovsky, E., Braley, H., Davey, G. M., Mottram, P., Van De Velde, N., Jensen, K., Lew, A. M., Wright, M. D., Heath, W. R., Shortman, K., and Lahoud, M. H. (2008). The dendritic cell subtype-restricted C-type lectin Clec9A is a target for vaccine enhancement. Blood 112, 3264–3273.
Carotta, S., Dakic, A., D’Amico, A., Pang, S. H., Greig, K. T., Nutt, S. L., and Wu, L. (2010). The transcription factor PU.1 controls dendritic cell development and Flt3 cytokine receptor expression in a dose-dependent manner. Immunity 32, 628–641.
Chappaz, S., and Finke, D. (2010). The IL-7 signaling pathway regulates lymph node development independent of peripheral lymphocytes. J. Immunol. 184, 3562–3569.
Cheong, C., Matos, I., Choi, J. H., Dandamudi, D. B., Shrestha, E., Longhi, M. P., Jeffrey, K. L., Anthony, R. M., Kluger, C., Nchinda, G., Koh, H., Rodriguez, A., Idoyaga, J., Pack, M., Velinzon, K., Park, C. G., and Steinman, R. M. (2010). Microbial stimulation fully differentiates monocytes to DC-SIGN/CD209(+) dendritic cells for immune T cell areas. Cell 143, 416–429.
Chorro, L., Sarde, A., Li, M., Woollard, K. J., Chambon, P., Malissen, B., Kissenpfennig, A., Barbaroux, J. B., Groves, R., and Geissmann, F. (2009). Langerhans cell (LC) proliferation mediates neonatal development, homeostasis, and inflammation-associated expansion of the epidermal LC network. J. Exp. Med. 206, 3089–3100.
Cisse, B., Caton, M. L., Lehner, M., Maeda, T., Scheu, S., Locksley, R., Holmberg, D., Zweier, C., Den Hollander, N. S., Kant, S. G., Holter, W., Rauch, A., Zhuang, Y., and Reizis, B. (2008). Transcription factor E2-2 is an essential and specific regulator of plasmacytoid dendritic cell development. Cell 135, 37–48.
Colonna, M., Trinchieri, G., and Liu, Y. J. (2004). Plasmacytoid dendritic cells in immunity. Nat. Immunol. 5, 1219–1226.
de Pooter, R. F., and Kee, B. L. (2010). E proteins and the regulation of early lymphocyte development. Immunol. Rev. 238, 93–109.
del Rio, M. L., Bernhardt, G., Rodriguez-Barbosa, J. I., and Forster, R. (2010). Development and functional specialization of CD103+ dendritic cells. Immunol. Rev. 234, 268–281.
den Haan, J. M., and Bevan, M. J. (2002). Constitutive versus activation-dependent cross-presentation of immune complexes by CD8(+) and CD8(-) dendritic cells in vivo. J. Exp. Med. 196, 817–827.
den Haan, J. M., Lehar, S. M., and Bevan, M. J. (2000). CD8(+) but not CD8(-) dendritic cells cross-prime cytotoxic T cells in vivo. J. Exp. Med. 192, 1685–1696.
Desch, A. N., Randolph, G. J., Murphy, K., Gautier, E. L., Kedl, R. M., Lahoud, M. H., Caminschi, I., Shortman, K., Henson, P. M., and Jakubzick, C. V. (2011). CD103+ pulmonary dendritic cells preferentially acquire and present apoptotic cell-associated antigen. J. Exp. Med. 208, 1789–1797.
Dorner, B. G., Dorner, M. B., Zhou, X., Opitz, C., Mora, A., Guttler, S., Hutloff, A., Mages, H. W., Ranke, K., Schaefer, M., Jack, R. S., Henn, V., and Kroczek, R. A. (2009). Selective expression of the chemokine receptor XCR1 on cross-presenting dendritic cells determines cooperation with CD8+ T cells. Immunity 31, 823–833.
Dorsey, M. J., Tae, H. J., Sollenberger, K. G., Mascarenhas, N. T., Johansen, L. M., and Taparowsky, E. J. (1995). B-ATF: a novel human bZIP protein that associates with members of the AP-1 transcription factor family. Oncogene 11, 2255–2265.
Echlin, D. R., Tae, H. J., Mitin, N., and Taparowsky, E. J. (2000). B-ATF functions as a negative regulator of AP-1 mediated transcription and blocks cellular transformation by Ras and Fos. Oncogene 19, 1752–1763.
Edelson, B. T., Bradstreet, T. R., Hildner, K., Carrero, J. A., Frederick, K. E., Kc, W., Belizaire, R., Aoshi, T., Schreiber, R. D., Miller, M. J., Murphy, T. L., Unanue, E. R., and Murphy, K. M. (2011). CD8alpha(+) dendritic cells are an obligate cellular entry point for productive infection by Listeria monocytogenes. Immunity 35, 236–248.
Edelson, B. T., Kc, W., Juang, R., Kohyama, M., Benoit, L. A., Klekotka, P. A., Moon, C., Albring, J. C., Ise, W., Michael, D. G., Bhattacharya, D., Stappenbeck, T. S., Holtzman, M. J., Sung, S. S., Murphy, T. L., Hildner, K., and Murphy, K. M. (2010). Peripheral CD103+ dendritic cells form a unified subset developmentally related to CD8alpha+ conventional dendritic cells. J. Exp. Med. 207, 823–836.
Esashi, E., Wang, Y. H., Perng, O., Qin, X. F., Liu, Y. J., and Watowich, S. S. (2008). The signal transducer STAT5 inhibits plasmacytoid dendritic cell development by suppressing transcription factor IRF8. Immunity 28, 509–520.
Fancke, B., Suter, M., Hochrein, H., and O’Keeffe, M. (2008). M-CSF: a novel plasmacytoid and conventional dendritic cell poietin. Blood 111, 150–159.
Fogg, D. K., Sibon, C., Miled, C., Jung, S., Aucouturier, P., Littman, D. R., Cumano, A., and Geissmann, F. (2006). A clonogenic bone marrow progenitor specific for macrophages and dendritic cells. Science 311, 83–87.
Gascoyne, D. M., Long, E., Veiga-Fernandes, H., De Boer, J., Williams, O., Seddon, B., Coles, M., Kioussis, D., and Brady, H. J. (2009). The basic leucine zipper transcription factor E4BP4 is essential for natural killer cell development. Nat. Immunol. 10, 1118–1124.
Ghosh, H. S., Cisse, B., Bunin, A., Lewis, K. L., and Reizis, B. (2010). Continuous expression of the transcription factor e2-2 maintains the cell fate of mature plasmacytoid dendritic cells. Immunity 33, 905–916.
Gilliet, M., Boonstra, A., Paturel, C., Antonenko, S., Xu, X. L., Trinchieri, G., O’Garra, A., and Liu, Y. J. (2002). The development of murine plasmacytoid dendritic cell precursors is differentially regulated by FLT3-ligand and granulocyte/macrophage colony-stimulating factor. J. Exp. Med. 195, 953–958.
Ginhoux, F., Collin, M. P., Bogunovic, M., Abel, M., Leboeuf, M., Helft, J., Ochando, J., Kissenpfennig, A., Malissen, B., Grisotto, M., Snoeck, H., Randolph, G., and Merad, M. (2007). Blood-derived dermal langerin+ dendritic cells survey the skin in the steady state. J. Exp. Med. 204, 3133–3146.
Ginhoux, F., Liu, K., Helft, J., Bogunovic, M., Greter, M., Hashimoto, D., Price, J., Yin, N., Bromberg, J., Lira, S. A., Stanley, E. R., Nussenzweig, M., and Merad, M. (2009). The origin and development of nonlymphoid tissue CD103+ DCs. J. Exp. Med. 206, 3115–3130.
Ginhoux, F., Tacke, F., Angeli, V., Bogunovic, M., Loubeau, M., Dai, X. M., Stanley, E. R., Randolph, G. J., and Merad, M. (2006). Langerhans cells arise from monocytes in vivo. Nat. Immunol. 7, 265–273.
Guerriero, A., Langmuir, P. B., Spain, L. M., and Scott, E. W. (2000). PU.1 is required for myeloid-derived but not lymphoid-derived dendritic cells. Blood 95, 879–885.
Hacker, C., Kirsch, R. D., Ju, X. S., Hieronymus, T., Gust, T. C., Kuhl, C., Jorgas, T., Kurz, S. M., Rose-John, S., Yokota, Y., and Zenke, M. (2003). Transcriptional profiling identifies Id2 function in dendritic cell development. Nat. Immunol. 4, 380–386.
Hashimoto, D., Miller, J., and Merad, M. (2011). Dendritic cell and macrophage heterogeneity in vivo. Immunity 35, 323–335.
Heath, W. R., Belz, G. T., Behrens, G. M., Smith, C. M., Forehan, S. P., Parish, I. A., Davey, G. M., Wilson, N. S., Carbone, F. R., and Villadangos, J. A. (2004). Cross-presentation, dendritic cell subsets, and the generation of immunity to cellular antigens. Immunol. Rev. 199, 9–26.
Hida, S., Ogasawara, K., Sato, K., Abe, M., Takayanagi, H., Yokochi, T., Sato, T., Hirose, S., Shirai, T., Taki, S., and Taniguchi, T. (2000). CD8(+) T cell-mediated skin disease in mice lacking IRF-2, the transcriptional attenuator of interferon-alpha/beta signaling. Immunity 13, 643–655.
Hildner, K., Edelson, B. T., Purtha, W. E., Diamond, M., Matsushita, H., Kohyama, M., Calderon, B., Schraml, B. U., Unanue, E. R., Diamond, M. S., Schreiber, R. D., Murphy, T. L., and Murphy, K. M. (2008). Batf3 deficiency reveals a critical role for CD8alpha+ dendritic cells in cytotoxic T cell immunity. Science 322, 1097–1100.
Hohl, T. M., Rivera, A., Lipuma, L., Gallegos, A., Shi, C., Mack, M., and Pamer, E. G. (2009). Inflammatory monocytes facilitate adaptive CD4 T cell responses during respiratory fungal infection. Cell Host Microbe 6, 470–481.
Holtschke, T., Lohler, J., Kanno, Y., Fehr, T., Giese, N., Rosenbauer, F., Lou, J., Knobeloch, K. P., Gabriele, L., Waring, J. F., Bachmann, M. F., Zinkernagel, R. M., Morse, H. C. III, Ozato, K., and Horak, I. (1996). Immunodeficiency and chronic myelogenous leukemia-like syndrome in mice with a targeted mutation of the ICSBP gene. Cell 87, 307–317.
Honda, K., Mizutani, T., and Taniguchi, T. (2004). Negative regulation of IFN-alpha/beta signaling by IFN regulatory factor 2 for homeostatic development of dendritic cells. Proc. Natl. Acad. Sci. U.S.A. 101, 2416–2421.
Ichikawa, E., Hida, S., Omatsu, Y., Shimoyama, S., Takahara, K., Miyagawa, S., Inaba, K., and Taki, S. (2004). Defective development of splenic and epidermal CD4+ dendritic cells in mice deficient for IFN regulatory factor-2. Proc. Natl. Acad. Sci. U.S.A. 101, 3909–3914.
Ingersoll, M. A., Platt, A. M., Potteaux, S., and Randolph, G. J. (2011). Monocyte trafficking in acute and chronic inflammation. Trends Immunol. 32, 470–477.
Jackson, J. T., Hu, Y., Liu, R., Masson, F., D’Amico, A., Carotta, S., Xin, A., Camilleri, M. J., Mount, A. M., Kallies, A., Wu, L., Smyth, G. K., Nutt, S. L., and Belz, G. T. (2011). Id2 expression delineates differential checkpoints in the genetic program of CD8alpha+ and CD103+ dendritic cell lineages. EMBO J. 30, 2690–2704.
Jelinek, I., Leonard, J. N., Price, G. E., Brown, K. N., Meyer-Manlapat, A., Goldsmith, P. K., Wang, Y., Venzon, D., Epstein, S. L., and Segal, D. M. (2011). TLR3-specific double-stranded RNA oligonucleotide adjuvants induce dendritic cell cross-presentation, CTL responses, and antiviral protection. J. Immunol. 186, 2422–2429.
Jung, S., Unutmaz, D., Wong, P., Sano, G., De Los Santos, K., Sparwasser, T., Wu, S., Vuthoori, S., Ko, K., Zavala, F., Pamer, E. G., Littman, D. R., and Lang, R. A. (2002). In vivo depletion of CD11c+ dendritic cells abrogates priming of CD8+ T cells by exogenous cell-associated antigens. Immunity 17, 211–220.
Kamizono, S., Duncan, G. S., Seidel, M. G., Morimoto, A., Hamada, K., Grosveld, G., Akashi, K., Lind, E. F., Haight, J. P., Ohashi, P. S., Look, A. T., and Mak, T. W. (2009). Nfil3/E4bp4 is required for the development and maturation of NK cells in vivo. J. Exp. Med. 206, 2977–2986.
Kashiwada, M., Cassel, S. L., Colgan, J. D., and Rothman, P. B. (2011). NFIL3/E4BP4 controls type 2 T helper cell cytokine expression. EMBO J. 30, 2071–2082.
Kim, T. S., and Braciale, T. J. (2009). Respiratory dendritic cell subsets differ in their capacity to support the induction of virus-specific cytotoxic CD8+ T cell responses. PLoS ONE 4, e4204. doi:10.1371/journal.pone.0004204
Kobayashi, T., Matsuoka, K., Sheikh, S. Z., Elloumi, H. Z., Kamada, N., Hisamatsu, T., Hansen, J. J., Doty, K. R., Pope, S. D., Smale, S. T., Hibi, T., Rothman, P. B., Kashiwada, M., and Plevy, S. E. (2011). NFIL3 is a regulator of IL-12 p40 in macrophages and mucosal immunity. J. Immunol. 186, 4649–4655.
Laouar, Y., Welte, T., Fu, X. Y., and Flavell, R. A. (2003). STAT3 is required for Flt3L-dependent dendritic cell differentiation. Immunity 19, 903–912.
Lemaitre, B., Nicolas, E., Michaut, L., Reichhart, J. M., and Hoffmann, J. A. (1996). The dorsoventral regulatory gene cassette spatzle/Toll/cactus controls the potent antifungal response in Drosophila adults. Cell 86, 973–983.
Lemos, M. P., Esquivel, F., Scott, P., and Laufer, T. M. (2004). MHC class II expression restricted to CD8alpha+ and CD11b+ dendritic cells is sufficient for control of Leishmania major. J. Exp. Med. 199, 725–730.
Leon, B., Martinez Del Hoyo, G., Parrillas, V., Vargas, H. H., Sanchez-Mateos, P., Longo, N., Lopez-Bravo, M., and Ardavin, C. (2004). Dendritic cell differentiation potential of mouse monocytes: monocytes represent immediate precursors of CD8- and CD8+ splenic dendritic cells. Blood 103, 2668–2676.
Lin, M. L., Zhan, Y., Proietto, A. I., Prato, S., Wu, L., Heath, W. R., Villadangos, J. A., and Lew, A. M. (2008). Selective suicide of cross-presenting CD8+ dendritic cells by cytochrome c injection shows functional heterogeneity within this subset. Proc. Natl. Acad. Sci. U.S.A. 105, 3029–3034.
Lin, Y. C., Jhunjhunwala, S., Benner, C., Heinz, S., Welinder, E., Mansson, R., Sigvardsson, M., Hagman, J., Espinoza, C. A., Dutkowski, J., Ideker, T., Glass, C. K., and Murre, C. (2010). A global network of transcription factors, involving E2A, EBF1 and Foxo1, that orchestrates B cell fate. Nat. Immunol. 11, 635–643.
Lukens, M. V., Kruijsen, D., Coenjaerts, F. E., Kimpen, J. L., and Van Bleek, G. M. (2009). Respiratory syncytial virus-induced activation and migration of respiratory dendritic cells and subsequent antigen presentation in the lung-draining lymph node. J. Virol. 83, 7235–7243.
Manfra, D. J., Chen, S. C., Jensen, K. K., Fine, J. S., Wiekowski, M. T., and Lira, S. A. (2003). Conditional expression of murine Flt3 ligand leads to expansion of multiple dendritic cell subsets in peripheral blood and tissues of transgenic mice. J. Immunol. 170, 2843–2852.
Manz, M. G., Traver, D., Miyamoto, T., Weissman, I. L., and Akashi, K. (2001). Dendritic cell potentials of early lymphoid and myeloid progenitors. Blood 97, 3333–3341.
Maraskovsky, E., Brasel, K., Teepe, M., Roux, E. R., Lyman, S. D., Shortman, K., and Mckenna, H. J. (1996). Dramatic increase in the numbers of functionally mature dendritic cells in Flt3 ligand-treated mice: multiple dendritic cell subpopulations identified. J. Exp. Med. 184, 1953–1962.
Mashayekhi, M., Sandau, M. M., Dunay, I. R., Frickel, E. M., Khan, A., Goldszmid, R. S., Sher, A., Ploegh, H. L., Murphy, T. L., Sibley, L. D., and Murphy, K. M. (2011). CD8alpha(+) dendritic cells are the critical source of interleukin-12 that controls acute infection by Toxoplasma gondii tachyzoites. Immunity 35, 249–259.
McKenna, H. J. (2001). Role of hematopoietic growth factors/flt3 ligand in expansion and regulation of dendritic cells. Curr. Opin. Hematol. 8, 149–154.
Merad, M., Ginhoux, F., and Collin, M. (2008). Origin, homeostasis and function of Langerhans cells and other langerin-expressing dendritic cells. Nat. Rev. Immunol. 8, 935–947.
Mount, A. M., Smith, C. M., Kupresanin, F., Stoermer, K., Heath, W. R., and Belz, G. T. (2008). Multiple dendritic cell populations activate CD4+ T cells after viral stimulation. PLoS ONE 3, e1691. doi:10.1371/journal.pone.0001691
Nagao, K., Ginhoux, F., Leitner, W. W., Motegi, S., Bennett, C. L., Clausen, B. E., Merad, M., and Udey, M. C. (2009). Murine epidermal Langerhans cells and langerin-expressing dermal dendritic cells are unrelated and exhibit distinct functions. Proc. Natl. Acad. Sci. U.S.A. 106, 3312–3317.
Nagasawa, M., Schmidlin, H., Hazekamp, M. G., Schotte, R., and Blom, B. (2008). Development of human plasmacytoid dendritic cells depends on the combined action of the basic helix-loop-helix factor E2-2 and the Ets factor Spi-B. Eur. J. Immunol. 38, 2389–2400.
Naik, S. H., Metcalf, D., Van Nieuwenhuijze, A., Wicks, I., Wu, L., O’Keeffe, M., and Shortman, K. (2006). Intrasplenic steady-state dendritic cell precursors that are distinct from monocytes. Nat. Immunol. 7, 663–671.
Naik, S. H., Sathe, P., Park, H. Y., Metcalf, D., Proietto, A. I., Dakic, A., Carotta, S., O’Keeffe, M., Bahlo, M., Papenfuss, A., Kwak, J. Y., Wu, L., and Shortman, K. (2007). Development of plasmacytoid and conventional dendritic cell subtypes from single precursor cells derived in vitro and in vivo. Nat. Immunol. 8, 1217–1226.
Nakano, H., Lin, K. L., Yanagita, M., Charbonneau, C., Cook, D. N., Kakiuchi, T., and Gunn, M. D. (2009). Blood-derived inflammatory dendritic cells in lymph nodes stimulate acute T helper type 1 immune responses. Nat. Immunol. 10, 394–402.
Neuenhahn, M., Kerksiek, K. M., Nauerth, M., Suhre, M. H., Schiemann, M., Gebhardt, F. E., Stemberger, C., Panthel, K., Schroder, S., Chakraborty, T., Jung, S., Hochrein, H., Russmann, H., Brocker, T., and Busch, D. H. (2006). CD8alpha+ dendritic cells are required for efficient entry of Listeria monocytogenes into the spleen. Immunity 25, 619–630.
Ohnmacht, C., Pullner, A., King, S. B., Drexler, I., Meier, S., Brocker, T., and Voehringer, D. (2009). Constitutive ablation of dendritic cells breaks self-tolerance of CD4 T cells and results in spontaneous fatal autoimmunity. J. Exp. Med. 206, 549–559.
Onai, N., Obata-Onai, A., Schmid, M. A., Ohteki, T., Jarrossay, D., and Manz, M. G. (2007). Identification of clonogenic common Flt3+M-CSFR+ plasmacytoid and conventional dendritic cell progenitors in mouse bone marrow. Nat. Immunol. 8, 1207–1216.
Onai, N., Obata-Onai, A., Tussiwand, R., Lanzavecchia, A., and Manz, M. G. (2006). Activation of the Flt3 signal transduction cascade rescues and enhances type I interferon-producing and dendritic cell development. J. Exp. Med. 203, 227–238.
Poltorak, A., He, X., Smirnova, I., Liu, M. Y., Van Huffel, C., Du, X., Birdwell, D., Alejos, E., Silva, M., Galanos, C., Freudenberg, M., Ricciardi-Castagnoli, P., Layton, B., and Beutler, B. (1998). Defective LPS signaling in C3H/HeJ and C57BL/10ScCr mice: mutations in Tlr4 gene. Science 282, 2085–2088.
Poulin, L. F., Henri, S., De Bovis, B., Devilard, E., Kissenpfennig, A., and Malissen, B. (2007). The dermis contains langerin+ dendritic cells that develop and function independently of epidermal Langerhans cells. J. Exp. Med. 204, 3119–3131.
Randolph, G. J., Ochando, J., and Partida-Sanchez, S. (2008). Migration of dendritic cell subsets and their precursors. Annu. Rev. Immunol. 26, 293–316.
Rathinam, C., Geffers, R., Yucel, R., Buer, J., Welte, K., Moroy, T., and Klein, C. (2005). The transcriptional repressor Gfi1 controls STAT3-dependent dendritic cell development and function. Immunity 22, 717–728.
Reizis, B., Bunin, A., Ghosh, H. S., Lewis, K. L., and Sisirak, V. (2011). Plasmacytoid dendritic cells: recent progress and open questions. Annu. Rev. Immunol. 29, 163–183.
Sancho, D., Joffre, O. P., Keller, A. M., Rogers, N. C., Martinez, D., Hernanz-Falcon, P., Rosewell, I., and Reis E Sousa, C. (2009). Identification of a dendritic cell receptor that couples sensing of necrosis to immunity. Nature 458, 899–903.
Sapoznikov, A., and Jung, S. (2008). Probing in vivo dendritic cell functions by conditional cell ablation. Immunol. Cell Biol. 86, 409–415.
Satpathy, A. T., Murphy, K. M., and Kc, W. (2011). Transcription factor networks in dendritic cell development. Semin. Immunol. 23, 388–397.
Schiavoni, G., Mattei, F., Borghi, P., Sestili, P., Venditti, M., Morse, H. C. III, Belardelli, F., and Gabriele, L. (2004). ICSBP is critically involved in the normal development and trafficking of Langerhans cells and dermal dendritic cells. Blood 103, 2221–2228.
Schiavoni, G., Mattei, F., Sestili, P., Borghi, P., Venditti, M., Morse, H. C. III, Belardelli, F., and Gabriele, L. (2002). ICSBP is essential for the development of mouse type I interferon-producing cells and for the generation and activation of CD8alpha(+) dendritic cells. J. Exp. Med. 196, 1415–1425.
Schmutz, S., Bosco, N., Chappaz, S., Boyman, O., Acha-Orbea, H., Ceredig, R., Rolink, A. G., and Finke, D. (2009). Cutting edge: IL-7 regulates the peripheral pool of adult ROR gamma+ lymphoid tissue inducer cells. J. Immunol. 183, 2217–2221.
Schnorrer, P., Behrens, G. M., Wilson, N. S., Pooley, J. L., Smith, C. M., El-Sukkari, D., Davey, G., Kupresanin, F., Li, M., Maraskovsky, E., Belz, G. T., Carbone, F. R., Shortman, K., Heath, W. R., and Villadangos, J. A. (2006). The dominant role of CD8+ dendritic cells in cross-presentation is not dictated by antigen capture. Proc. Natl. Acad. Sci. U.S.A. 103, 10729–10734.
Schotte, R., Nagasawa, M., Weijer, K., Spits, H., and Blom, B. (2004). The ETS transcription factor Spi-B is required for human plasmacytoid dendritic cell development. J. Exp. Med. 200, 1503–1509.
Schulz, O., Diebold, S. S., Chen, M., Naslund, T. I., Nolte, M. A., Alexopoulou, L., Azuma, Y. T., Flavell, R. A., Liljestrom, P., and Reis E Sousa, C. (2005). Toll-like receptor 3 promotes cross-priming to virus-infected cells. Nature 433, 887–892.
Shklovskaya, E., O’Sullivan, B. J., Ng, L. G., Roediger, B., Thomas, R., Weninger, W., and Fazekas De St Groth, B. (2011). Langerhans cells are precommitted to immune tolerance induction. Proc. Natl. Acad. Sci. U.S.A. 108, 18049–18054.
Siddiqui, K. R., Laffont, S., and Powrie, F. (2010). E-cadherin marks a subset of inflammatory dendritic cells that promote T cell-mediated colitis. Immunity 32, 557–567.
Spits, H., Couwenberg, F., Bakker, A. Q., Weijer, K., and Uittenbogaart, C. H. (2000). Id2 and Id3 inhibit development of CD34(+) stem cells into predendritic cell (pre-DC)2 but not into pre-DC1. Evidence for a lymphoid origin of pre-DC2. J. Exp. Med. 192, 1775–1784.
Spooner, C. J., Cheng, J. X., Pujadas, E., Laslo, P., and Singh, H. (2009). A recurrent network involving the transcription factors PU.1 and Gfi1 orchestrates innate and adaptive immune cell fates. Immunity 31, 576–586.
Steinman, R. M. (2010). Some active areas of DC research and their medical potential. Eur. J. Immunol. 40, 2085–2088.
Steinman, R. M., and Cohn, Z. A. (1973). Identification of a novel cell type in peripheral lymphoid organs of mice. I. Morphology, quantitation, tissue distribution. J. Exp. Med. 137, 1142–1162.
Steinman, R. M., and Witmer, M. D. (1978). Lymphoid dendritic cells are potent stimulators of the primary mixed leukocyte reaction in mice. Proc. Natl. Acad. Sci. U.S.A. 75, 5132–5136.
Sung, S. S., Fu, S. M., Rose, C. E. Jr., Gaskin, F., Ju, S. T., and Beaty, S. R. (2006). A major lung CD103 (alphaE)-beta7 integrin-positive epithelial dendritic cell population expressing Langerin and tight junction proteins. J. Immunol. 176, 2161–2172.
Suzuki, S., Honma, K., Matsuyama, T., Suzuki, K., Toriyama, K., Akitoyo, I., Yamamoto, K., Suematsu, T., Nakamura, M., Yui, K., and Kumatori, A. (2004). Critical roles of interferon regulatory factor 4 in CD11bhighCD8alpha- dendritic cell development. Proc. Natl. Acad. Sci. U.S.A. 101, 8981–8986.
Tailor, P., Tamura, T., Morse, H. C. III, and Ozato, K. (2008). The BXH2 mutation in IRF8 differentially impairs dendritic cell subset development in the mouse. Blood 111, 1942–1945.
Tamura, T., Tailor, P., Yamaoka, K., Kong, H. J., Tsujimura, H., O’Shea, J. J., Singh, H., and Ozato, K. (2005). IFN regulatory factor-4 and -8 govern dendritic cell subset development and their functional diversity. J. Immunol. 174, 2573–2581.
Tsujimura, H., Tamura, T., and Ozato, K. (2003). Cutting edge: IFN consensus sequence binding protein/IFN regulatory factor 8 drives the development of type I IFN-producing plasmacytoid dendritic cells. J. Immunol. 170, 1131–1135.
Waskow, C., Liu, K., Darrasse-Jeze, G., Guermonprez, P., Ginhoux, F., Merad, M., Shengelia, T., Yao, K., and Nussenzweig, M. (2008). The receptor tyrosine kinase Flt3 is required for dendritic cell development in peripheral lymphoid tissues. Nat. Immunol. 9, 676–683.
Wilson, N. K., Foster, S. D., Wang, X., Knezevic, K., Schutte, J., Kaimakis, P., Chilarska, P. M., Kinston, S., Ouwehand, W. H., Dzierzak, E., Pimanda, J. E., De Bruijn, M. F., and Gottgens, B. (2010). Combinatorial transcriptional control in blood stem/progenitor cells: genome-wide analysis of ten major transcriptional regulators. Cell Stem Cell 7, 532–544.
Wu, L., D’Amico, A., Winkel, K. D., Suter, M., Lo, D., and Shortman, K. (1998). RelB is essential for the development of myeloid-related CD8alpha- dendritic cells but not of lymphoid-related CD8alpha+ dendritic cells. Immunity 9, 839–847.
Wu, L., Nichogiannopoulou, A., Shortman, K., and Georgopoulos, K. (1997). Cell-autonomous defects in dendritic cell populations of Ikaros mutant mice point to a developmental relationship with the lymphoid lineage. Immunity 7, 483–492.
Keywords: dendritic cells, transcription factors, differentiation, immunity
Citation: Chopin M, Allan RS and Belz GT (2012) Transcriptional regulation of dendritic cell diversity. Front. Immun. 3:26. doi: 10.3389/fimmu.2012.00026
Received: 05 December 2011;
Accepted: 08 February 2012;
Published online: 27 February 2012.
Edited by:
Christian Kurts, Friedrich Wilhelms-Universität Bonn, GermanyReviewed by:
Oliver Pabst, Hannover Medical School, GermanyCopyright: © 2012 Chopin, Allan and Belz. This is an open-access article distributed under the terms of the Creative Commons Attribution Non Commercial License, which permits non-commercial use, distribution, and reproduction in other forums, provided the original authors and source are credited.
*Correspondence: Gabrielle T. Belz, Division of Molecular Immunology, Walter and Eliza Hall Institute of Medical Research, 1G Royal Parade, Parkville, Melbourne, VIC 3052, Australia. e-mail:YmVsekB3ZWhpLmVkdS5hdQ==
Disclaimer: All claims expressed in this article are solely those of the authors and do not necessarily represent those of their affiliated organizations, or those of the publisher, the editors and the reviewers. Any product that may be evaluated in this article or claim that may be made by its manufacturer is not guaranteed or endorsed by the publisher.
Research integrity at Frontiers
Learn more about the work of our research integrity team to safeguard the quality of each article we publish.