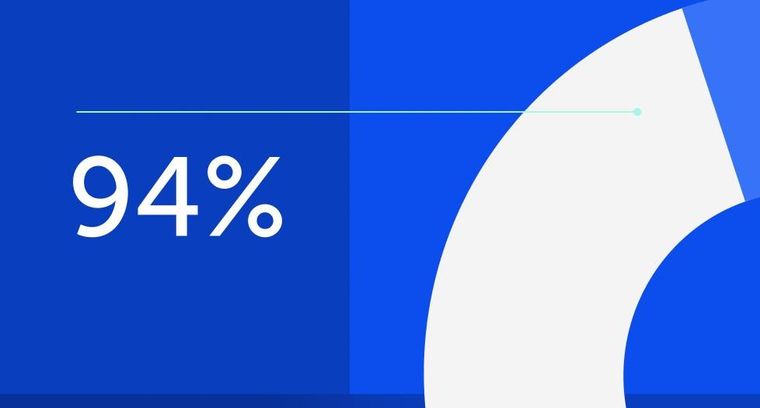
94% of researchers rate our articles as excellent or good
Learn more about the work of our research integrity team to safeguard the quality of each article we publish.
Find out more
SYSTEMATIC REVIEW article
Front. Hum. Neurosci., 03 March 2025
Sec. Brain Imaging and Stimulation
Volume 19 - 2025 | https://doi.org/10.3389/fnhum.2025.1501209
This article is part of the Research TopicMethods in Brain StimulationView all 8 articles
Introduction: Electrical stimulation (ES), including transcranial electrical stimulation (tES) and transcutaneous vagus nerve stimulation (tVNS), has shown potential for cognitive enhancement in military contexts. Various types of ES, such as transcranial direct current stimulation (tDCS) and transcranial alternating current stimulation (tACS), modulate neuronal membrane potentials and cortical excitability, potentially improving cognitive functions relevant to military training and operations.
Methods: This systematic review updates previous findings by examining studies published between 2019 and 2024 that investigated electrical stimulation effects on cognitive performance in military personnel and tasks. We focused on whether the studies addressed key questions about the generalizability of lab findings to military tasks, the frequency and intensity of adverse effects, the impact of repeated ES administration, and the ethical and regulatory considerations for its use in potentially vulnerable military populations.
Results: Eleven studies met the inclusion criteria; most demonstrated overall low to some concerns, however, two of these had overall high risk of bias. While tES and tVNS showed some promise for enhancing multitasking and visual search performance, the results were mixed, with no reliable effects on vigilance tasks.
Discussion: The reviewed studies highlight the need for a better understanding of ES mechanisms, optimal stimulation parameters, and individual differences in response to ES. They also highlight the importance of conducting high-powered research in military settings to evaluate the efficacy, safety, and ethical implications of ES. Future research should address the generalizability of lab-based results to real-world military tasks, monitor the frequency and intensity of adverse effects, and explore the long-term impacts of repeated administration. Furthermore, ethical and regulatory considerations are crucial for the responsible application of ES in military contexts, and a series of outstanding questions is posed to guide continuing research in this domain.
Electrical stimulation (ES) involves administering low intensity (0.5 m–3.0 mA) electrical current (direct or alternating) to the surface of the scalp or skin via two or more electrodes. Mechanistic models of transcranial ES (tES) suggest that the applied electrical current propagates through the skull, dura mater, arachnoid and subarachnoid space to modulate cortical neuronal membrane potentials (Galan-Gadea et al., 2023; Lefaucheur and Wendling, 2019; Molaee-Ardekani et al., 2013; Nitsche et al., 2008; Nitsche and Paulus, 2000; Reato et al., 2019). There are several different types of ES, including transcranial direct current stimulation (tDCS), transcranial alternating current stimulation (tACS), transcranial random noise stimulation (tRNS), and transcutaneous vagus nerve stimulation (tVNS). tDCS applies a current, which can be excitatory (hypopolarization) or inhibitory (hyperpolarization), influencing local and distal networks of cortical and subcortical neurons (Kunze et al., 2016; Lefaucheur and Wendling, 2019). tACS is thought to influence neuronal oscillations, thereby affecting neuronal communication within the brain (Fries, 2005; Herrmann et al., 2013), and tRNS is a type of tACS that applies a frequency spectrum of alternating current likely acting on sodium channels (Chaieb et al., 2015; Schoen and Fromherz, 2008). Electrical current applied transcutaneously, for example, with auricular or cervical tVNS can affect cortical processing likely via modulation of brainstem activity, autonomic nervous system activity, and perhaps changes in cortical excitability (Capone et al., 2015). These distributed effects on neuronal activity can produce a broad range of behavioral effects in both clinical and non-clinical participants (Bestmann et al., 2015; Brunoni et al., 2012; Sun et al., 2021), including faster reaction times and/or improved accuracy on cognitive and motor tasks, and improved spatial working memory performance. Thus, ES holds potential for improving performance in military domains including aviation, training, and operations.
In a series of comprehensive reviews on enhancement research for military applications, tES is identified as a promising method for altering cognitive function in military personnel in addition to other interventions, for example, augmented reality, mindfulness training, and sleep modification (Brunyé et al., 2020; Davis and Smith, 2019; Feltman et al., 2019; Lu et al., 2022; Peltier et al., 2019). Research using tES to target the dorsolateral prefrontal cortex (DLPFC), medial temporal lobes, fusiform gyrus, and frontopolar regions have shown beneficial effects on cognitive functions ranging from vigilance and threat detection to executive function, face memory, and creative problem solving (Brunyé et al., 2017; Koizumi et al., 2020; McKinley et al., 2012). Despite these initial promising results, overarching conclusions from these reviews and others (including meta-analyses) consistently point to equivocal results across published research and a need to better understand a multitude of outstanding questions (see Table 1) (de Berker et al., 2013; Brunoni et al., 2012; Horvath et al., 2014, 2015, 2016; Imburgio and Orr, 2018; Paulus, 2014; Prehn and Flöel, 2015). These outstanding questions generally cover topics related to underlying mechanisms, experimental methodology, task-related outcomes, short-and long-term effects, adverse effects, individual differences, ethics and regulation, and generalizability of laboratory findings to military contexts and tasks.
Table 1. Outstanding research questions to guide continuing research and development with ES, with an emphasis on eventual military applications.
A recent systematic review of transcranial direct current stimulation (tDCS) effects on performance enhancement in military contexts examined 34 articles published between 2008 and 2018 (Feltman et al., 2019). This review was restricted to randomized controlled experimental designs with military-age (18–50 years) healthy non-clinical samples. Most examined articles (26 of 34) reported some positive effects of tDCS on cognitive performance, including executive function (2), learning (6), creativity and cognitive flexibility (2), perception and attention (8), memory (3), and working memory (7). Based on the results of the review, the authors suggest promise for tES, and tDCS in particular, imparting positive effects on cognitive functions with applicability to military contexts.
The present systematic review was conducted to update the most recent review (Feltman et al., 2019). We identified articles using military personnel and/or military outcome tasks published in 2019–2024. We assessed whether the identified studies adequately addressed any of the questions posed in Table 1. We first briefly summarize the prospective application of ES in military training and operations, and some of the challenges in realizing this goal. We then discuss the questions posed in Table 1 and detail the methodology and results of our systematic review.
Cognitive performance is a critical factor responsible for successes and failures during military training and operations with cognitive decrements estimated to account for the majority (80–85%) of accidents during military training and operations (Thomas and Russo, 2007). Many core cognitive functions are therefore foundational to the successful performance of a broad range of military tasks. The cognitive tasks demanded of military personnel vary widely as a function of military occupational specialization and level of responsibility introduced by ascending rank (echelon). According to an international expert consensus panel, critical among those cognitive functions are attention and vigilance, processing speed, cognitive control (performance monitoring, response selection, inhibition, goal selection/updating/maintenance), shifting, self-knowledge, visual perception, and understanding others’ mental states (Albertella et al., 2023). Examples of tactical-level military tasks critically involving each of these cognitive functions are detailed in Table 2.
Table 2. Core cognitive functions involved in successful military task performance (in descending order of importance), and example tactical military tasks engaging those core functions.
One unique aspect of military training and operations is that they are conducted under high levels of cognitive and physical stress, energy imbalance, sleep loss, dehydration, and thermal burden (Adler et al., 2004; Brunyé et al., 2021; Campbell and Nobel, 2009). Many of these states independently and interactively produce acute impairments of cognitive function (Brunyé et al., 2021; Flood and Keegan, 2022; Lieberman et al., 2002, 2005, 2006, 2009; Orasanu and Backer, 1996; Vartanian et al., 2018). For example, the psychological stress imposed during combat-like training of elite military units is associated with impairments of attention and vigilance, memory, and reasoning (Lieberman et al., 2005). Sleep loss slows processing speed and lengthens reaction times, lowers task accuracy, and negatively influences moral decision making (Good et al., 2020; Petrofsky et al., 2022). Calorie deprivation causes decrements in executive function (Giles et al., 2019) (but also (see Lieberman et al., 2008)); dehydration impairs executive function, attention, and motor skills (Wittbrodt and Millard-Stafford, 2018); and both cold and heat stress negatively influence higher-level cognitive functions (Martin et al., 2019).
Given that ES may hold potential for improving performance in each of these cognitive functions, it is explored as a tool to remediate cognitive decrements induced by the physical and cognitive demands of military training and operations. As such, some studies using ES interventions examine effects under conditions of relative stress and adversity, complementing basic research done in relatively comfortable settings.
In Table 1, we posed a series of questions valuable for guiding continuing research examining the prospective application of ES to military contexts and tasks. We briefly summarize each question below, and then detail the methods and results of our systematic review. A more exhaustive list of outstanding questions is included in the Discussion section, broadly motivating continuing research and application.
The generalizability of human sciences, particularly in the domain of human performance, offers both opportunities and challenges when transitioning from basic research to applied military settings (Blacker et al., 2019; Goodwin et al., 2018; Hedrick et al., 1993; Shenberger-Trujillo and Kurinec, 2016). Basic research provides a foundational and mechanistic understanding of human behavior, cognition, and performance, which can inform application to military contexts such as training and operations. The perceptual, cognitive, and affective processes responsible for executing laboratory tasks are foundational, theoretically underlying the performance of any cognitive task, in any context. Basic research therefore enables the development of broadly applicable strategies for adopting new tools and technologies.
However, challenges arise in transferring discoveries made in basic research to diverse real-world scenarios. With respect to military applications, there are inherent contextual differences between controlled laboratory environments and complex military operations, inter-and intra-individual variability in human performance, operational constraints such as high-stress environments, and security concerns regarding the application of susceptible technologies to potentially vulnerable populations of military personnel (Blacker et al., 2019; Hedrick et al., 1993; Niemeyer, 2009). It is important to conduct high-powered research in military settings, with military personnel, using military tasks and relevant performance outcomes. Addressing these challenges necessitates interdisciplinary collaboration among researchers, military professionals, and policymakers to ensure that insights from basic research are effectively translated into practical applications while considering the unique complexities of military training and operations.
For example, while tDCS targeting the DLPFC shows promise for improving outcomes on abstract working memory tasks performed in laboratory settings, does it improve outcomes in relatively demanding and dynamic contexts with challenging and highly applied tasks (e.g., processing and manipulating verbal and spatial information in the context of tactical communications)? The relatively small effect sizes seen on the aggregate when examining links between tES and cognitive performance (Brunoni and Vanderhasselt, 2014; Hill et al., 2016; Horvath et al., 2015) could suggest it is unlikely to affect performance on relatively variable tasks performed in noisy contexts. Similarly, the promising effects of combining tES with working memory training may or may not transfer to similar tasks performed outside of a laboratory context. It has indeed been challenging to find evidence for such transfer within the laboratory itself (Brunoni and Vanderhasselt, 2014; Melby-Lervåg et al., 2016; Pergher et al., 2022).
Acute adverse effects of ES administration include those occurring during or immediately after stimulation (Antal et al., 2017). An early systematic review of tDCS-associated adverse effects (Brunoni et al., 2011) found the most frequently reported effects compared to sham to be itching (39.3% vs. 32.9%), tingling (22.2% vs. 18.3%), a burning sensation at the electrode site(s) (8.7% vs. 10%), headache (14.8% vs. 16.2%), and discomfort (10.4% vs. 13.4%). However, the reporting of adverse events was generally inadequate and likely biased, limiting the ability to effectively assess their frequency, intensity, and presence across experimental conditions. A more recent review found that most adverse effects of tDCS are mild, not considered serious, and short-lived, but that relatively prolonged adverse effects can also occur—namely skin lesions; and mania or hypomania primarily in patients with depression. Similarly, these adverse events were inconsistently reported and the authors suggest that further investigations are needed to characterize their type, frequency, intensity, and duration (Matsumoto and Ugawa, 2017).
A systematic review of tVNS found the most common adverse effects to be local skin irritation from electrode placement (18.2%), headache (3.6%), and nasopharyngitis (1.7%), with a minority (2.6%) dropping out of the studies due to tolerability. Stimulation was not accounted for in the heterogeneity of effects from these studies as many of the studies did not report all parameters (Redgrave et al., 2018). A more recent systematic review and meta-analysis of auricular tVNS reported that half of the studies did not disclose whether adverse effects were recorded. The most frequently reported adverse effects were ear pain, headache, and tingling. Overall, there were no differences in the risk of adverse effects following auricular tVNS when compared to controls. There appears to be no causal relationship between taVNS and severe adverse events (Kim et al., 2022).
A large systematic review and meta-analysis on tolerability found that higher levels of tDCS exposure through repeated administration (typically separated by 1 day) do not increase the incidence or intensity of adverse events, and did not vary across clinical and non-clinical groups (Nikolin et al., 2018, 2019). An additional study examining five tDCS sessions within a 25-h period found no serious adverse events, but did report mild adverse effects including scalp erythema, tingling and burning sensation at the electrode site, and a transient metallic taste (Zappasodi et al., 2018).
The effect of repeated tDCS assessed with neuroimaging has yielded variable results. No metabolite changes are observed during magnetic resonance spectroscopy following five tDCS sessions within 25-h periods (Zappasodi et al., 2018). Similarly, no change in blood-based metabolic biomarkers indicative of neuronal atrophy are observed following five tDCS sessions (Kortteenniemi et al., 2020). In contrast, three sessions of prefrontal tDCS were found to increase resting cerebral profusion in the locus coeruleus that persisted across sessions of active stimulation (Sherwood et al., 2021). Another study examining three sessions of prefrontal tDCS showed highly variable effects on resting-state functional connectivity that resulted in extremely low intra-participant reliability. Interestingly, intra-participant reliability was relatively high in a sham condition, suggesting that tDCS exerts markedly different functional effects across sessions, i.e., dose dependent effects (Wörsching et al., 2017). Moreover, low intra-individual variability is observed in tDCS-induced motor evoked potentials over the course of three sessions, suggesting a general lack of habituation (Ammann et al., 2017). These studies suggest that effects of repeated tDCS administration are very difficult to predict within and across individuals.
While preliminary evidence suggests that repeated tDCS administration is safe and tolerable, this is far from exhaustive, and more research is needed to understand how higher exposures (intensity, duration, frequency) and other types of ES affect tolerability, brain, and behavior. Much remains unknown about the chronic risk profile of ES. With the proliferation of ES devices onto the open consumer market, this is a particularly important question to consider. While laboratory studies with humans might typically consider the effects of 3–5 sessions, home users of do-it-yourself consumer devices can administer ES multiple times a day for months or years, resulting in over 100 sessions of self-administered ES (Jwa, 2015; Wexler, 2016, 2018; Wexler and Reiner, 2017, 2019). In addition to skin lesions and burns reported by home users, there are potential long-term effects of repeated exposures to direct ES of the scalp, skull, meninges, and cortex. Additional insights can be gathered from clinical trials involving multi-session tDCS administration over the course of days and weeks. For example, when examining patients with bipolar depression, Sampai-Junior and colleagues demonstrated no difference in rates of adverse events between sham and active groups after 12 daily sessions over 6 weeks (at 2 mA for 30 min), but noted some evidence for increased reports of localized skin redness in the active group (Sampaio-Junior et al., 2018). Similar findings were noted in a clinical trial examining the effect of repeated tDCS (21 sessions, 2 mA for 30 min) in patients with major depressive disorder (MDD), demonstrating no differences between groups in the frequency or severity of adverse events, while noting increased rates of local skin redness and heat or burning sensations in the active group (Borrione et al., 2024). While these results are compelling, it will be important to understand not only subject experiences but also potential effects on biomarkers of neuronal integrity over the course of dozens or hundreds of sessions.
Herein we describe our search strategy, inclusion and exclusion criteria, study selection, data extraction, and risk of bias assessments. The full PRISMA diagram can be found in Figure 1.
Figure 1. PRISMA flow diagram depicting the flow of information through the three phases of our systematic review (identification, screening, inclusion).
Electronic searches of titles, abstracts, and keywords were conducted using the databases Scopus, Medeline, and Embase, using Boolean operators. The search terms are provided in the Supplementary materials. Database searches were conducted on December 14, 2023. No date restrictions were placed on the literature search at this point to capture forward and backwards citations (snowballing) within our timeframe. The reference lists of the included studies were screened (backward citation) as well as studies citing the included studies (forward citation) using the online tool Citation Chaser (Haddaway et al., 2022). Studies not indexed via Citation Chaser were screened manually. The results were imported in EndNote and Excel.
Full text journal articles were included if they used a non-invasive electrical brain stimulation technique (e.g., tDCS or peripheral nerve stimulation; see the Supplementary materials), focused on cognitive performance modulation, and included a military population or a military relevant task (see Table 2 for example military tasks). Studies were excluded if the abstract and title were in a language other than English, and if they were of a non-experimental nature, for example, reviews.
One author (OvdG) initially screened titles and abstracts. Consequently, three authors (OvdG, TB, and SR) independently screened full text reports of all identified eligible records. Articles not in English were translated. Discrepancies were resolved through discussion and consensus. The screening process was carried out using EndNote and Microsoft Excel.
A standardized form based on the Cochrane data collection form was used for data extraction of relevant study characteristics, including general information, and study eligibility screen. If the study was found to be eligible, additional data were extracted from the methods, results/outcomes, and discussion (e.g., strengths and limitations) sections.
The Cochrane risk of bias assessment tool, RoB2, was used to evaluate the methodological quality of each included study (Sterne et al., 2019). This tool included biases induced due to the randomization process, deviation from the intended intervention, missing outcome data, outcome measures used, and bias in selection of presented results. The risk bias for each study was categorized as ‘low risk, ‘some concerns’ or ‘high risk’ automatically by the algorithm, and then again by a consensus meeting between three assessors (authors TTB, MGM, ET). Table 3 reports the results of this process for both the algorithm and assessors.
A total of 15 records were included in our review, 11 of which report experimental research, and four of which review ethical challenges. For each of the 11 experimental records, Table 3 details the risk of bias, design, sample size, targeted brain area, electrode size and positioning, stimulation intensity, density, duration, timing of stimulation, outcome tasks, and a summary of outcomes. For the four ethics reviews, we include them in the Discussion section.
According to assessors’ evaluations, most studies had overall low risk (4) or some concerns (5) of bias. Two studies demonstrated overall high risk of bias.
All but three studies administered tDCS; the others administered tACS or tVNS (transauricular or cervical). The vast majority (10/11) of studies used a between-participants design with either exclusively military personnel as participants or a mixed sample (i.e., military and civilian). Overall sample sizes varied from small to large (N = 12–98), but were small to medium when divided into groups in between-participants designs (n = 8–34).
Studies using tDCS or tACS variably administered from 1 m to 2 mA intensity for 20 m–30 min, with most stimulating during (online, 5) or prior to (offline, 4) task performance, and two studies stimulating both prior to and during task performance (mixed). The cortical target sites were the left (6) or right (2) DLPFC, right posterior parietal cortex (PPC, 1), right inferior frontal gyrus (IFG, 1) and primary visual cortex (V1, 1) using highly varied electrode types (e.g., sponge versus sintered ring) and surface areas (and thus current densities).
Studies using tVNS variably administered stimulation to auricular or cervical vagus afferents, with a consistent stimulation frequency of 25 Hz but different durations of 8 and 60 min. In both cases, stimulation intensity was individualized based on each participant’s pain or discomfort threshold, but the authors did not disclose actual stimulation intensity. Note that in some cases, the latter may be due to inadequate disclosure of proprietary stimulation parameters by device manufacturers.
A wide range of outcome tasks were administered across studies. The most commonly employed task was the Mackworth Clock Test (4), generally employed as a measure of vigilance. Other tasks included the diffuse & focused attention tests, a continuous performance task (CPT; measuring vigilance and attention), a psychomotor vigilance task (PVT; measuring vigilance), object recognition, visual search, oddball task (measuring target detection), the Multi-Attribute Task Battery (measuring multitasking), Go/No-Go (measuring impulsivity), Stroop tasks (measuring executive control), an emotional working memory task, and a threat of shock paradigm (measuring startle responses). In Table 2 we detailed 10 core cognitive processes important for common military functions; in general, the identified studies targeted most (6) of these with the absence of outcome tasks related to understanding the self and others’ mental states, shifting, language production and comprehension, and declarative memory.
In addition to behavioral outcomes, three studies measured brain activity using electroencephalography (EEG), and two using magnetic resonance imaging (MRI). In two of those cases, behavioral results were either not measured or not reported. Specifically, Sherwood et al. (2021) and Kim et al. (2021) used MRI and administered the Mackworth Clock Test to participants but did not measure and/or report behavioral data from this task. Dai and colleagues (Dai et al., 2022) used EEG and administered the Mackworth Clock Test and Go/No-Go task, and did report behavioral results.
For the tDCS or tACS studies, most (5) reported no effects of stimulation on any measured behavioral outcomes, or did not report behavioral outcomes. This included studies targeting the left DLPFC (Dai et al., 2022; Kim et al., 2021; Sherwood et al., 2021), the right PPC (Blacker et al., 2020), the right IFG, and V1 (Willmot et al., 2023). These studies measured outcomes on vigilance, executive function, visual search, and object recognition tasks.
Two of the tDCS/tACS studies showed mixed results, with stimulation targeting the left DLPFC enhancing performance on one but not another task. Zhu and colleagues (Zhu et al., 2023) reported faster reaction times and higher accuracy on a Stroop task in active versus sham tACS conditions, but no effect of stimulation condition on the Go/No-Go task. In general, the Stroop task focuses on selective attention and managing interference, more consistently engaging the anterior cingulate cortex (ACC) and DLPFC. The Go/No-Go task instead focuses on inhibiting prepotent motor responses, with a more consistent involvement of the right IFG and basal ganglia. Given that the authors targeted the left DLPFC with tACS, this may explain why positive effects were found with the Stroop but not Go/No-Go task. Smits et al. (2023) found no effect of active versus sham tDCS on startle response or working memory task performance; however, exploratory analyses did suggest that baseline EEG theta:beta ratio might predict whether anodal versus sham stimulation affects working memory performance.
Finally, the two remaining tDCS studies showed beneficial results of tDCS on visual search, vigilance, and multitasking tasks. First, Nelson et al. (2019) reported higher throughput on the MATB task in the active versus sham group with online anodal stimulation (2 mA) targeting the left DLPFC. This study did however employ a small sample size (n = 8 per group) which reduces the statistical power, leading to unreliable results and increasing the risk of both Type I (false positive) and Type II (false negative) errors. Studies with small sample sizes also make findings less representative of the broader population, limiting the generalizability and reproducibility of the results. Second, Fatideh et al. (2023) administered 2 mA of offline tDCS to the right DLPFC, and reported faster reaction times and higher accuracy in the anodal versus cathodal or sham conditions on both visual search and vigilance tasks. However, this study also employed a small sample size (N = 12), and demonstrated overall high risk of bias. The results of these studies should therefore be interpreted with caution.
The two studies using tVNS showed mixed results. McIntire et al. (2021) administered either active or sham cervical tVNS offline before participants completed the Mackworth Clock Test, N-back task, PVT, and MATB task. The authors reported higher throughput on the MATB task, and higher discriminability on the PVT in the active versus sham group; however, no effects were found on the Mackworth Clock Test or N-back test. Wang et al. (2022) combined inhibitory control training with simultaneous (online) active or sham auricular tVNS, and measured outcomes on a Stop-Signal task, Go/No-Go task, and Stroop task. The authors found beneficial effects of active versus sham tVNS on the Stop-Signal and Go/No-Go tasks (accelerated training and near transfer effects), but no effects on the Stroop task. The discrepancy in results in these two tVNS studies are likely due to the use of different stimulation sites (i.e., auricular versus cervical), stimulation durations (i.e., 8 versus 60 min), or other experimental factors. It may also be the case that tVNS can benefit vigilance, multitasking, Stop-Signal, and Go/No-Go task performance by enhancing arousal, attention, and response inhibition through its putative effects on the autonomic nervous system and the locus coeruleus-norepinephrine (LC-NE) system. However, it may not significantly benefit working memory or Stroop task performance because these tasks rely on specific executive functions and neural circuits (such as the DLPFC and ACC) that may not be as directly influenced by the generalized autonomic nervous system effects of tVNS. These remain open questions for continuing research.
We began this review by posing a series of three outstanding questions for researchers and practitioners interested in the potential for ES methods to enhance performance in military training or operations. The first question was whether ES effects seen with validated laboratory tasks can generalize to relatively realistic and complex military tasks. Across the included studies, most cognitive tasks were validated and commonly used laboratory tasks, including the Go/No-Go, Stroop, CPT, startle response, and working memory tasks. However, relatively applied and military relevant tasks were also used in some studies; these included the Mackworth Clock Test, the MATB, and visual search and threat detection tasks.
The studies identified in this review found no evidence that performance on the Mackworth Clock Test is modulated with tDCS or tVNS. This is a somewhat surprising finding given prior research demonstrating effects of similar tDCS parameters on this task (McIntire et al., 2014, 2017). Evidence that MATB performance can be modulated was positive, with one tDCS study suggesting performance improvement with tDCS (Nelson et al., 2019), and one study suggesting improvements with tVNS (McIntire et al., 2021). For visual search and threat detection tasks, results were mixed. For example, in a within-subjects study, Fatideh and colleagues applied 2 mA bilateral tDCS to the DLPFC (F3, F4) of Iranian aviators (N = 12) over 3 sessions separated by 48 h (Fatideh et al., 2023). Their findings suggested that visual search improved with right anodal DLPFC stimulation, relative to left DLPFC anodal or sham stimulation. In contrast, in a repeated measures (2 × 2) study featuring a larger sample (N = 74; n = 18, 19, 18, 17) of US military personnel, participants received anodal or sham tDCS to the right PPC as they underwent training in one of two visual search tasks across three identical sessions (Blacker et al., 2020). The authors found participants improved their performance in response to both training paradigms, those being object recognition and visual search strategy, but that these improvements were unaffected by right PPC stimulation. Moreover, in a between subjects study involving Australian Army soldiers (N = 74), Willmot and colleagues found that participants receiving 2 mA tDCS to the right IFG or the V1 during a militarized threat detection task did not perform significantly better than those receiving sham stimulation to the rIFG (Willmot et al., 2023), contrary to previous studies using civilian samples (Clark et al., 2012). It could be the case that right PPC or IFG stimulation are less likely to modulate applied visual search and threat detection tasks, whereas right DLPFC stimulation holds more potential. However, given the large difference in sample sizes amongst the aforementioned papers, and the variable training level of the military personnel, it is a much more parsimonious explanation to reason that effects of tDCS could in fact be lessened by both the inclusion of larger samples in the later two studies (Minarik et al., 2016). In addition, there could be an interaction between prior expertise of participants and the training task, reducing the effectiveness of tDCS (Brunyé et al., 2014; Sánchez-Kuhn et al., 2018). Returning to Table 2, continuing research should also begin to examine other important and military-relevant cognitive tasks such as understanding the self and others’ mental states, language production and comprehension, and declarative memory.
The second question posed in the introduction related to the frequency and intensity of acute and/or long-term adverse effects of ES. Most studies (8/11) unfortunately did not report the presence of cutaneous sensations or adverse effects in subjects. In the three papers that did report, results were mixed. Zhu et al. (2023) found that tACS elicited “mild skin sensations” in five of the 60 participants, and none reported visual phosphenes. Smits et al. (2023) reported that one participant had a skin lesion that healed within a week, and some participants reported other mild cutaneous sensations (burning, itching, tingling); however, there were no significant differences in adverse effects between active and sham conditions. Kim et al. (2021) measured and reported cutaneous sensations, noting that there were no significant differences in adverse effects between the active and sham condition. Based on these reports, mild cutaneous sensations appear to rarely occur (in about 10% of a sample), though there is an improbable but possible risk of more serious cutaneous injury (burns, lesions). Measuring and reporting the frequency and intensity of adverse effects is critically important given that military personnel are considered a vulnerable population, with discomfort and adverse effects detracting from occupational duties, and that acceptance and adoption of novel biotechnologies might be limited by perceived discomfort. Adverse effects and drop-out rates are often used as proxy measures of acceptability/participant perspectives (van der Groen, 2023). However, intervention acceptability by the end-user and stakeholders is implicated in intervention uptake, adherence, and overall effectiveness (Sekhon et al., 2022). Continuing research should place more emphasis on measuring and reporting acceptability, and adverse events.
The third question posed in the introduction related to the effects of repeated ES administration on tolerability, brain function and structure, and behavior. Across the included studies, stimulation durations were predominantly limited to single sessions of 20m–30 min of tES or 8m–60 min of tVNS, which do not provide new information regarding relatively sustained or repeated stimulation conditions. There was one exception, with Fatideh et al. (2023) administering 20 min of tDCS repeatedly over three sessions. While the authors did assess participants’ mood states, they did not report information regarding cutaneous sensations or adverse effects, and did not assess effects on brain function or structure.
Several additional questions are important to examine as scientists and practitioners considering adopting tES, and/or tVNS in military training or operations.
What are the mechanisms underlying ES effects at the micro, meso, and macro scales? Models of ES effects can be generally trifurcated into characterizing the microscopic, mesoscopic, and macroscropic levels of description (Bestmann et al., 2015). There are several putative mechanistic models of ES, mainly surrounding tDCS effects on cortical function. At the microscopic level, models tend to focus on neuronal membrane polarization induced by electrical currents. While many models and empirical research emphasizes sliding-scale models (i.e., anodal excitatory, cathodal inhibitory), more recent research suggests a much more complex and nonlinear series of functional activity changes with tDCS (Batsikadze et al., 2013; Bonaiuto and Bestmann, 2015; Molaee-Ardekani et al., 2013; Rawji et al., 2018; Silvanto et al., 2008; Vergallito et al., 2023). On the mesoscopic scale, models tend to focus on the effects on activity of relatively distributed populations and networks of cortical and subcortical neurons. Research has showcased tDCS effects on resting-state functional network connectivity in the motor network, sensorimotor network, default mode network, frontal–parietal network, and self-referential network (Chan et al., 2021; Fischer et al., 2017; Mencarelli et al., 2020; Peña-Gómez et al., 2012), including both intrinsic activity within a given network and extrinsic activity between networks. Interestingly, there is a pronounced gap between microscopic and mesoscopic models and what should be expected behaviorally from ES administration. This gap unfortunately makes it difficult or impossible to predict the behavioral consequences of ES administration, whether related to performance improvement or degradation (Bestmann et al., 2015; Bonaiuto and Bestmann, 2015).
How do stimulation parameters modulate ES effects, and what is their optimal combination? There is a complex parameter space surrounding the administration of ES, including the targeted brain region, the number and size of electrodes, the polarity, intensity, timing (i.e., online versus offline), duration of stimulation, and whether the stimulation is personalized to individual structural or functional brain characteristics. Each of these parameters independently influences the behavioral and functional effects of ES, which likely have yet unknown interactive effects (Ehrhardt et al., 2021; Filmer et al., 2019; Friehs and Frings, 2019; Ho et al., 2016; Jacobson et al., 2012; Kessler et al., 2013; Opitz et al., 2015; Saturnino et al., 2019; Truong et al., 2013; Živanović et al., 2021). Given the inherent flexibility of ES stimulation parameters and their variability across studies, it is difficult to predict the optimal combinations of parameters to influence cognitive function (Au et al., 2017; Khorrampanah et al., 2020; Wagner et al., 2016). This is a challenging combinatorial modeling problem, especially given that very few studies manipulate two or more parameters across multiple (i.e., >2) levels. Recent research has attempted to use hierarchical Bayesian meta-regressions to predict the magnitude of tDCS effects as a function of 10 stimulation parameters (including electrode number, polarity, intensity, timing, duration), and identify optimal parameter combinations for maximizing effects on five performance domains (motor skill acquisition, visual search, working memory, vigilance, inhibitory control) (Santander et al., 2024). The model did not successfully converge on optimal solutions to confer reliable predictions to the five behavioral outcomes, largely due to extensive variability across research studies (e.g., experimental methods, tasks, and outcome measures). A relatively domain-general outcome suggested an advantage to anodal versus cathodal stimulation, but this pattern was not found in any specific outcome domain. The authors conclude that there is an urgent need for research to parametrically manipulate and probe independent and interactive effects across relatively complex parameter spaces.
How do individual differences influence ES effects? The effects of individual differences on ES-related outcomes have been examined in three primary ways. First, studies have examined how individual differences in brain structural and functional characteristics affect physiological and behavioral responses to ES. For example, individual differences in prefrontal cortical thickness influence tDCS effects on decision-making (Filmer et al., 2019). Additionally, tDCS-induced changes in resting-state functional connectivity are associated with differences in visual object-matching task performance (Pupíková et al., 2022). Secondly, studies have examined how individual differences in personality traits affect physiological and behavioral responses to tES (Brunyé et al., 2014, 2015; Krause and Cohen Kadosh, 2014). For example, tDCS affects reading speed of social sentences in readers with low scores on the behavioral approach and inhibition scales, but not those with high scores (Reyes et al., 2021); and trait anxiety modulates the effects of tDCS on creative task performance (Xiang et al., 2021). Third, studies have examined how individual differences in baseline knowledge or task proficiency affect physiological and behavioral responses to ES (Splittgerber et al., 2020). For example, tDCS increases the creativity of improvised instrument play for novices but harms expert performance (Rosen et al., 2016); and those with lower baseline reading proficiency show greater positive effects of tDCS on cross-language speech production (Bhattacharjee et al., 2020). Given the inherent heterogeneity of military personnel, the effects of relatively invariant individual characteristics is an important research topic. Individually tailored paradigms are likely needed, factoring in complex interacting variables (e.g., stimulating parameters, physiological, anatomical, and genetic differences). Personalized protocols do however raise challenges in a military setting, such as time constraints and practicalities. Recent research has further demonstrated large intra-individual variability in responses to repeat sessions of tDCS in that individuals did not respond consistently to tDCS when applied repeatedly over time (Willmot et al., 2024).
Can ES support and optimize performance, or does it truly enhance performance beyond baseline functioning? While the notion of cognitive enhancement is intriguing (Bostrom and Sandberg, 2009; Farah, 2015; Farah et al., 2014), it is challenging to truly demonstrate enhancement as opposed to performance sustainment or optimization, per se (Brunyé et al., 2020). Several philosophical positions conceptualize that enhancement must improve functioning of an individual beyond their normal range (Agar, 2013; Menuz et al., 2013). True enhancement would transcend the biological limits shaped by millennia of evolution—defining such biological limits will be critical, both within and across individuals. Demonstrating true enhancement requires quantitatively establishing baselines of an individual’s optimal biological performance potential under optimal conditions. For example, under an idealized set of hypothetical conditions, what could an individual achieve for reaction times, accuracy levels, or any other outcome of interest? Only when biotechnology-induced performance exceeds what has been established as innate optimal performance can it truly be deemed enhancement. In most cases, including when ES mitigates the performance deleterious effects of a contextual factor (e.g., sleep deprivation, stress, fatigue) (Hart-Pomerantz et al., 2024), performance is being sustained or optimized relative to a control condition that attempts to mimic some aspects of active experimental conditions (e.g., sham procedures with ES). These are important empirical comparisons, but they may not allow us to quantify the biological limits of performance or make inferences about enhancement. Moreover, military personnel might already be at their performance upper limit due to their training, limiting the effectiveness of ES. For example some tDCS studies in military samples did not observe any additional performance benefits (Blacker et al., 2020; Willmot et al., 2023). Visual search is required in many professions where an undetected threat, such as a weapon, can put the well-being of others at risk. Given the importance of detecting these threats, researchers have used various experimental techniques to improve performance in visual search tasks, albeit with varying degrees of success. Here, we explore two promising techniques to improve visual search using ecologically valid synthetic aperture radar stimuli: object recognition training and search strategy training. Search strategy training is intended to make observers search more systematically through a display, whereas object recognition training is intended to improve observers’ ability to recognize critical targets. Search strategy training was implemented by instructing participants to scan through the display in a pre-specified pattern. Object recognition training was implemented by having participants discriminate between targets and non-targets. We also manipulated whether observers received anodal or sham transcranial direct current stimulation (tDCS) during training, which has been shown to improve visual search performance and target learning. To measure the effectiveness of the training and stimulation conditions, we tested object recognition accuracy and overall visual search performance before and after three sessions of increasingly difficult training. Results indicated that object recognition training significantly improved object recognition accuracy relative to the search strategy group, whereas search strategy training was effective in improving visual search accuracy in those who adhered to the training. However, tDCS did not interact with training type, and although both training types yielded significant improvements, training-related improvements were not significantly different between the different approaches. This evidence suggests that strategy-based training could be as effective as the more prototypical object recognition training. Moreover, interactions between baseline performance and tDCS effectiveness have been demonstrated (Splittgerber et al., 2020). Whether ES can further improve performance in high performing individuals remains an open question.
Do trade-offs exist between targeted and untargeted performance domains? Using ES to enhance cognitive function raises questions about potential trade-offs between targeted and untargeted brain regions or tasks, aligning with the concept of net zero-sum dynamics within the nervous system. The net zero-sum framework posits that enhancements in one cognitive domain may be counterbalanced by detriments in another, necessitating careful consideration of cost–benefit interactions across various levels of neural processing (Brem et al., 2014; Luber, 2014). The concept underscores the need to assess potential costs alongside any enhancements. Multi-parameter considerations encompassing magnitude, duration, reversibility, and the level of impact across micro to macro scales are essential for delineating cost–benefit relationships. Within this framework, the notion of processing power emerges as pivotal, representing the brain’s capacity to allocate resources dynamically across different cognitive demands. Trade-offs, reflecting competition between sub-processes independent of top-down control, may elucidate interactions among neural elements. For instance, the speed-accuracy trade-off in decision-making tasks illustrates resource allocation dynamics, where competing demands for processing power influence task performance. While identifying the cost of ES-related enhancement remains challenging, the conceptualization of net zero-sum dynamics underscores the intricate interplay between cognitive enhancements and associated costs, necessitating nuanced framing and empirical investigation. Given the inherent complexity of military training and operations, we believe these investigations are highly warranted as military personnel are increasingly expected to perform multiple complex tasks simultaneously, recruiting diverse cognitive processes. If we are able to facilitate a few of those processes, what might happen to the other untargeted processes?
How will the use of ES devices integrate into existing military training and potentially be integrated into existing battle ensembles worn by military personnel? Schedules within military training establishments are increasingly facing time constraints and the introduction of an additional training requirement would add stress to an already overburdened system. It might be that the best solution is to identify critical periods of training where insertion of ES alongside existing training can yield performance improvement, mitigate performance decrements, or facilitate accelerated recovery. Another option could be to introduce effective interventions into the ongoing physical and wellbeing activities undertaken by military personnel during normal operation periods, thereby increasing the possibility of effective acceptance and implementation. Integrating devices into military personnel’s existing individual ensemble would involve a systematic process from research and development to acquisition and integration. Initially, the readiness and feasibility of ES technology would be assessed using the technology readiness level scale, considering factors such as safety, effectiveness, and compatibility with existing equipment (Small Business Association, 2024). Research and development efforts would focus on optimizing ES devices for military use, involving the development of prototype devices for testing and evaluation. This phase would also encompass human factors engineering to ensure the usability and ergonomic design of the devices, maximizing ease of use for warfighters. Interoperability with other equipment and systems, cybersecurity precautions, as well as considerations for sustainment and logistics, would be critical during the integration process. Insights from previous attempts to integrate EEG into warfighters’ helmets can inform this endeavor (Ko et al., 2019; Von Rosenberg et al., 2016; Zaman et al., 2022). While EEG integration faced challenges related to signal quality, comfort, and practicality, lessons learned from these efforts can guide the development and integration of other neurotechnology devices. Particularly, the importance of user-centered design, robust testing and evaluation, and ongoing lifecycle management to ensure the successful integration and utilization of neuroenhancement technologies in military operations is emphasized.
What are the ethical and regulatory considerations for ES application in military contexts? The use of ES in military contexts for enhancing cognitive functions and performance raises several ethical, legal, safety, and regulatory considerations. These include informed consent, autonomy, maintaining warfighter dignity and morality, potential risks to soldiers’ health and well-being, societal implications, and compliance with existing medical device regulations (Cinel et al., 2019; Davis and Smith, 2019; Puscas, 2020). Military personnel are a potentially vulnerable group who may face pressure to accept neuroenhancement due to authority relationships, hierarchical command structures, and the potential rights they forego when they enlist (Latheef and Henschke, 2020).
Regular and prolonged use of ES may carry yet unknown long-term effects for brain function, mental health, and neurological integrity. While acute effects are often reported as mild (e.g., tingling, itching), the consequences of repeated or long-term use remain largely unknown, raising questions about cumulative risks, dependency, and reversibility. If ES induces lasting neural plasticity or cognitive changes, what are the implications for warfighters once they leave military service? This issue extends to the ethical responsibility of the military and medical oversight bodies in ensuring that enhancement technologies do not compromise the long-term well-being of personnel, during and beyond their service. Another ethical issue is dual-use concerns, specifically the possibility that neuroenhancing technologies developed for military applications could be repurposed for punitive, coercive, or otherwise unauthorized civilian and non-civilian uses. This weaponization of neurotechnology, including potential application in interrogation, must be carefully considered and addressed through policy frameworks and regulatory oversight. An additional concern arises when the enhancement of one function coincides with diminished activity in other brain regions (Cinel et al., 2019). In future multi-domain operations that demand high levels of adaptability and multitasking, this could prove problematic.
For ES to be valuable and justifiable in military contexts, the positive effects must be superior, additive, proliferative, or associated with fewer adverse events than existing enhancement methods such as cognitive training (Davis and Smith, 2019). The use of stimulation to not only restore and maintain, but also aim to enhance human capabilities beyond natural limits raises ethical questions around fairness, merit, and the redefinition of military virtues such as courage, loyalty, and sacrifice (Puscas, 2020). Fairness and equity must also be considered, particularly regarding access to systems and potential performance disparities that can result. If ES confers advantages in certain domains, it could create disparities between enhanced versus non-enhanced personnel, and potentially lead to new stratification within military ranks where some are prioritized for promotions or special roles, whereas others are disadvantaged. Finally, it is possible that ES could create unrealistic expectations of warfighters, or cause overreliance or overestimation of its potential; indeed, if military personnel believe it provides a strong advantage for mitigating stress, fatigue, or other suboptimal states, they may take more risks and jeopardize the safety of themselves and others (Wilde, 1982).
Proactive engagement with stakeholders, including military personnel, ethicists, policymakers, and the broader scientific community, is essential to address these ethical and regulatory challenges, and establish guidelines that balance the potential benefits of ES with ethical and safety considerations in military training and operations. To this aim, a number of frameworks and regulatory guidelines on neuroenhancement are published by various government organizations and academia (Emanuel et al., 2019; Maslen et al., 2014). Furthermore, the Hybrid Minds project seeks to establish a foundational framework for ethically and legally evaluating neurostimulation systems embedded in intelligent neuroprostheses to ensure safety and ethics (Jotterand and Ienca, 2023). This framework is uniquely shaped by insights from user experiences and perspectives, along with input from scientists and engineers, helping to motivate international declarations regarding neurotechnologies and their relevance for human rights (Bublitz, 2024).
This systematic review highlights the complex effects of ES, including tDCS, tACS, and tVNS, on military-relevant cognitive performance outcomes. While some studies demonstrate potential benefits of ES on tasks like multitasking and visual search, the overall evidence remains mixed, with no significant effects observed on measures of vigilance and inconsistent outcomes across other cognitive domains. Despite the insights provided by the reviewed studies, the generalizability of these findings remains limited due to the relatively small number of studies identified and reviewed. The variability in methodologies, sample sizes, and cognitive tasks assessed further constrains the ability to draw definitive conclusions about the efficacy and safety of ES in military contexts. The scarcity of high-powered, ecologically valid studies highlights the need for more robust investigations that systematically explore ES effects across diverse military tasks and operational conditions. Future research should prioritize larger sample sizes, improved standardization of stimulation parameters, examination of individual differences, and longitudinal studies to better assess the long-term effects and practical viability of ES for performance enhancement in real-world military applications.
Furthermore, the review identified several critical gaps and outstanding questions that must be addressed to advance the application of ES in military settings. These include understanding the generalizability of lab-based findings to real-world military tasks, the frequency and intensity of acute and long-term adverse effects, the impact of repeated ES administration, and the ethical and regulatory considerations surrounding the use of these technologies. The ethical challenges of ES, particularly in military contexts, extend beyond individual safety to broader concerns about informed consent, autonomy, fairness, and the potential for coercion and inequities in hierarchical structures. Additionally, the long-term consequences of ES on neurological integrity and cognitive function remain unclear, necessitating careful oversight to prevent unintended harm to a potentially vulnerable population of end users. Addressing these questions through interdisciplinary collaboration and rigorous research is essential to fully realize the potential of ES for enhancing cognitive performance and operational effectiveness in military personnel while ensuring its responsible and ethical implementation.
All extracted data are provided in Table 3.
OG: Conceptualization, Methodology, Writing – original draft, Writing – review & editing. SR: Conceptualization, Methodology, Writing – original draft, Writing – review & editing. NW: Conceptualization, Methodology, Writing – original draft, Writing – review & editing, Investigation. MM: Data curation, Investigation, Methodology, Writing – review & editing. ET: Conceptualization, Data curation, Investigation, Methodology, Writing – review & editing. TB: Conceptualization, Methodology, Writing – original draft, Writing – review & editing, Funding acquisition, Supervision.
The author(s) declare financial support was received for the research, authorship, and/or publication of this article. Personnel time to support OG, SR, NW, and TB was funded by the authors’ respective institutions. MM and ET were funded by the U. S. Army DEVCOM Soldier Center via grant W911QY-19–2–0003 awarded to Tufts University.
The authors declare that the research was conducted in the absence of any commercial or financial relationships that could be construed as a potential conflict of interest.
The author(s) declared that they were an editorial board member of Frontiers, at the time of submission. This had no impact on the peer review process and the final decision.
All claims expressed in this article are solely those of the authors and do not necessarily represent those of their affiliated organizations, or those of the publisher, the editors and the reviewers. Any product that may be evaluated in this article, or claim that may be made by its manufacturer, is not guaranteed or endorsed by the publisher.
The views expressed in this article are solely those of the authors and do not reflect the official policies or positions of the Department of Army, the Department of Defense, or any other department or agency of the U.S. government. The contents include material subject to © Crown copyright (2025), Dstl. This information is licensed under the Open Government Licence v3.0. To view this licence, visit https://www.nationalarchives.gov.uk/doc/open-government-licence/. Where we have identified any third party copyright information you will need to obtain permission from the copyright holders concerned. Any enquiries regarding this publication should be sent to: Y2VudHJhbGVucXVpcmllc0Bkc3RsLmdvdi51aw==.
The Supplementary material for this article can be found online at: https://www.frontiersin.org/articles/10.3389/fnhum.2025.1501209/full#supplementary-material
Adler, A. B., McGurk, D., Stetz, M. C., and Bliese, P. D. (2004). Military occupational stressors in garrison, training, and deployed environments (DTIC technical report ADA425834). Walter Reed Army Institute of Research. Available online at: https://apps.dtic.mil/sti/citations/ADA425834 (Accessed January 15, 2024).
Agar, N. (2013). Truly human enhancement: A philosophical Defense of limits. Cambridge, MA: MIT Press.
Albertella, L., Kirkham, R., Adler, A. B., Crampton, J., Drummond, S. P. A., Fogarty, G. J., et al. (2023). Building a transdisciplinary expert consensus on the cognitive drivers of performance under pressure: an international multi-panel Delphi study. Front. Psychol. 13:1017675. doi: 10.3389/fpsyg.2022.1017675
Ammann, C., Lindquist, M. A., and Celnik, P. A. (2017). Response variability of different anodal transcranial direct current stimulation intensities across multiple sessions. Brain Stimul. 10, 757–763. doi: 10.1016/j.brs.2017.04.003
Antal, A., Alekseichuk, I., Bikson, M., Brockmöller, J., Brunoni, A. R., Chen, R., et al. (2017). Low intensity transcranial electric stimulation: safety, ethical, legal regulatory and application guidelines. Clin. Neurophysiol. 128, 1774–1809. doi: 10.1016/j.clinph.2017.06.001
Au, J., Karsten, C., Buschkuehl, M., and Jaeggi, S. M. (2017). Optimizing transcranial direct current stimulation protocols to promote long-term learning. J. Cogn. Enhanc. 1, 65–72. doi: 10.1007/s41465-017-0007-6
Batsikadze, G., Moliadze, V., Paulus, W., Kuo, M.-F. M.-F., and Nitsche, M. A. (2013). Partially non-linear stimulation intensity-dependent effects of direct current stimulation on motor cortex excitability in humans. J. Physiol. 591, 1987–2000. doi: 10.1113/jphysiol.2012.249730
Bestmann, S., de Berker, A. O., and Bonaiuto, J. (2015). Understanding the behavioural consequences of noninvasive brain stimulation. Trends Cogn. Sci. 19, 13–20. doi: 10.1016/j.tics.2014.10.003
Bhattacharjee, S., Kashyap, R., O’Brien, B. A., McCloskey, M., Oishi, K., Desmond, J. E., et al. (2020). Reading proficiency influences the effects of transcranial direct current stimulation: evidence from selective modulation of dorsal and ventral pathways of reading in bilinguals. Brain Lang. 210:104850. doi: 10.1016/j.bandl.2020.104850
Blacker, K. J., Hamilton, J., Roush, G., Pettijohn, K. A., and Biggs, A. T. (2019). Cognitive training for military application: a review of the literature and practical guide. J. Cogn. Enhanc. 3, 30–51. doi: 10.1007/s41465-018-0076-1
Blacker, K. J., Peltier, C., McKinley, R. A., and Biggs, A. T. (2020). What versus how in visual search: effects of object recognition training, strategy training, and non-invasive brain stimulation on satellite image search. J. Cogn. Enhanc. 4, 131–144. doi: 10.1007/s41465-020-00165-5
Bonaiuto, J. J., and Bestmann, S. (2015). Understanding the nonlinear physiological and behavioral effects of tDCS through computational neurostimulation. Prog. Brain Res. 222, 75–103. doi: 10.1016/bs.pbr.2015.06.013
Borrione, L., Cavendish, B. A., Aparicio, L. V. M., Luethi, M. S., Goerigk, S., Ramos, M. R. F., et al. (2024). Home-use transcranial direct current stimulation for the treatment of a major depressive episode: a randomized clinical trial. JAMA Psychiatry 81, 329–337. doi: 10.1001/jamapsychiatry.2023.4948
Bostrom, N., and Sandberg, A. (2009). Cognitive enhancement: methods, ethics, regulatory challenges. Sci. Eng. Ethics 15, 311–341. doi: 10.1007/s11948-009-9142-5
Brem, A.-K., Fried, P. J., Horvath, J. C., Robertson, E. M., and Pascual-Leone, A. (2014). Is neuroenhancement by noninvasive brain stimulation a net zero-sum proposition? NeuroImage 85, 1058–1068. doi: 10.1016/j.neuroimage.2013.07.038
Brunoni, A. R., Amadera, J., Berbel, B., Volz, M. S., Rizzerio, B. G., and Fregni, F. (2011). A systematic review on reporting and assessment of adverse effects associated with transcranial direct current stimulation. Int. J. Psychophysiol. 14, 1133–1145. doi: 10.1017/S1461145710001690
Brunoni, A. R., Nitsche, M. A., Bolognini, N., Bikson, M., Wagner, T., Merabet, L., et al. (2012). Clinical research with transcranial direct current stimulation (tDCS): challenges and future directions. Brain Stimul. 5, 175–195. doi: 10.1016/j.brs.2011.03.002
Brunoni, A. R., and Vanderhasselt, M.-A. (2014). Working memory improvement with non-invasive brain stimulation of the dorsolateral prefrontal cortex: a systematic review and meta-analysis. Brain Cogn. 86, 1–9. doi: 10.1016/j.bandc.2014.01.008
Brunyé, T. T., Brou, R., Doty, T. J., Gregory, F. D., Hussey, E. K., Lieberman, H. R., et al. (2020). A review of US Army research contributing to cognitive enhancement in military contexts. J. Cogn. Enhanc. 4, 453–468. doi: 10.1007/s41465-020-00167-3
Brunyé, T. T., Holmes, A., Cantelon, J., Eddy, M. D., Gardony, A. L., Mahoney, C. R., et al. (2014). Direct current brain stimulation enhances navigation efficiency in individuals with low spatial sense of direction. Neuro Report 25, 1175–1179. doi: 10.1097/WNR.0000000000000214
Brunyé, T. T., Moran, J. M., Cantelon, J., Holmes, A., Eddy, M. D., Mahoney, C. R., et al. (2015). Increasing breadth of semantic associations with left frontopolar direct current brain stimulation: a role for individual differences. Neuro Report 26, 296–301. doi: 10.1097/WNR.0000000000000348
Brunyé, T. T., Moran, J. M., Holmes, A., Mahoney, C. R., and Taylor, H. A. (2017). Non-invasive brain stimulation targeting the right fusiform gyrus selectively increases working memory for faces. Brain Cogn. 113, 32–39. doi: 10.1016/j.bandc.2017.01.006
Brunyé, T. T., Yau, K., Okano, K., Elliott, G., Olenich, S., Giles, G. E., et al. (2021). Toward predicting human performance outcomes from wearable technologies: a computational Modeling approach. Front. Physiol. 12:738973. doi: 10.3389/fphys.2021.738973
Bublitz, J. C. (2024). What an international declaration on Neurotechnologies and human rights could look like: ideas, suggestions, Desiderata. AJOB Neuroscience 15, 96–112. doi: 10.1080/21507740.2023.2270512
Campbell, D. J., and Nobel, O. B.-Y. (2009). Occupational stressors in military service: a review and framework. Mil. Psychol. 21, S47–S67. doi: 10.1080/08995600903249149
Capone, F., Assenza, G., Di Pino, G., Musumeci, G., Ranieri, F., Florio, L., et al. (2015). The effect of transcutaneous vagus nerve stimulation on cortical excitability. J. Neural Transm. 122, 679–685. doi: 10.1007/s00702-014-1299-7
Chaieb, L., Antal, A., and Paulus, W. (2015). Transcranial random noise stimulation-induced plasticity is NMDA-receptor independent but sodium-channel blocker and benzodiazepines sensitive. Front. Neurosci. 9:125. doi: 10.3389/fnins.2015.00125
Chan, M. M. Y., Yau, S. S. Y., and Han, Y. M. Y. (2021). The neurobiology of prefrontal transcranial direct current stimulation (tDCS) in promoting brain plasticity: a systematic review and meta-analyses of human and rodent studies. Neurosci. Biobehav. Rev. 125, 392–416. doi: 10.1016/j.neubiorev.2021.02.035
Cinel, C., Valeriani, D., and Poli, R. (2019). Neurotechnologies for human cognitive augmentation: current state of the art and future prospects. Front. Hum. Neurosci. 13:13. doi: 10.3389/fnhum.2019.00013
Clark, V. P., Coffman, B. A., Mayer, A. R., Weisend, M. P., Lane, T. D. R., Calhoun, V. D., et al. (2012). TDCS guided using fMRI significantly accelerates learning to identify concealed objects. NeuroImage 59, 117–128. doi: 10.1016/j.neuroimage.2010.11.036
Dai, J., Wang, H., Yang, L., Wang, C., Cheng, S., Zhang, T., et al. (2022). The neuroelectrophysiological and behavioral effects of transcranial direct current stimulation on executive vigilance under a continuous monotonous condition. Front. Neurosci. 16:910457. doi: 10.3389/fnins.2022.910457
Davis, S. E., and Smith, G. A. (2019). Transcranial direct current stimulation use in warfighting: benefits, risks, and future prospects. Front. Hum. Neurosci. 13:114. doi: 10.3389/fnhum.2019.00114
de Berker, A. O., Bikson, M., and Bestmann, S. (2013). Predicting the behavioral impact of transcranial direct current stimulation: issues and limitations. Front. Hum. Neurosci. 7:613. doi: 10.3389/fnhum.2013.00613
Ehrhardt, S. E., Filmer, H. L., Wards, Y., Mattingley, J. B., and Dux, P. E. (2021). The influence of tDCS intensity on decision-making training and transfer outcomes. J. Neurophysiol. 125, 385–397. doi: 10.1152/jn.00423.2020
Emanuel, P., Walper, S., DiEuliis, D., Klein, N., Petro, J. B., and Giordano, J. (2019). Cyborg soldier 2050: Human/machine fusion and the implications for the future of the DoD (technical report CCDC CBC-TR-1599; pp. 1–42). U.S. Army combat capabilities development command chemical biological Center. Available online at: https://apps.dtic.mil/sti/pdfs/AD1083010.pdf?ref=truth11.com (Accessed January 15, 2024).
Farah, M. J. (2015). The unknowns of cognitive enhancement. Science 350, 379–380. doi: 10.1126/science.aad5893
Farah, M. J., Smith, M. E., Ilieva, I., and Hamilton, R. H. (2014). Cognitive enhancement. WIREs. Cogn. Sci. 5, 95–103. doi: 10.1002/wcs.1250
Fatideh, S. P., Hosseini, S. M., Amini, A., Vaezmousavi, M., and Shirvani, H. (2023). Effect of dorsolateral prefrontal cortex (DLPFC) transcranial direct current stimulation (tDCS) on visual search and attention functions of aeronaut. J. Military Med. 25, 1774–1786. doi: 10.30491/JMM.2023.1006296.0
Feltman, K. A., Hayes, A. M., Bernhardt, K. A., Nwala, E., and Kelley, A. M. (2019). Viability of tDCS in military environments for performance enhancement: a systematic review. Mil. Med. 185, e53–e60. doi: 10.1093/milmed/usz189
Filmer, H. L., Ehrhardt, S. E., Shaw, T. B., Mattingley, J. B., and Dux, P. E. (2019). The efficacy of transcranial direct current stimulation to prefrontal areas is related to underlying cortical morphology. NeuroImage 196, 41–48. doi: 10.1016/j.neuroimage.2019.04.026
Fischer, D. B., Fried, P. J., Ruffini, G., Ripolles, O., Salvador, R., Banus, J., et al. (2017). Multifocal tDCS targeting the resting state motor network increases cortical excitability beyond traditional tDCS targeting unilateral motor cortex. NeuroImage 157, 34–44. doi: 10.1016/j.neuroimage.2017.05.060
Flood, A., and Keegan, R. J. (2022). Cognitive resilience to psychological stress in military personnel. Front. Psychol. 13:809003. doi: 10.3389/fpsyg.2022.809003
Friehs, M. A., and Frings, C. (2019). Offline beats online: transcranial direct current stimulation timing influences on working memory. NeuroReport 30, 795–799. doi: 10.1097/WNR.0000000000001272
Fries, P. (2005). A mechanism for cognitive dynamics: neuronal communication through neuronal coherence. Trends Cogn. Sci. 9, 474–480. doi: 10.1016/j.tics.2005.08.011
Galan-Gadea, A., Salvador, R., Bartolomei, F., Wendling, F., and Ruffini, G. (2023). Spherical harmonics representation of the steady-state membrane potential shift induced by tDCS in realistic neuron models. J. Neural Eng. 20:026004. doi: 10.1088/1741-2552/acbabd
Giles, G. E., Mahoney, C. R., Caruso, C., Bukhari, A. S., Smith, T. J., Pasiakos, S. M., et al. (2019). Two days of calorie deprivation impairs high level cognitive processes, mood, and self-reported exertion during aerobic exercise: a randomized double-blind, placebo-controlled study. Brain Cogn. 132, 33–40. doi: 10.1016/j.bandc.2019.02.003
Good, C. H., Brager, A. J., Capaldi, V. F., and Mysliwiec, V. (2020). Sleep in the United States military. Neuropsychopharmacology 45, 176–191. doi: 10.1038/s41386-019-0431-7
Goodwin, G. F., Blacksmith, N., and Coats, M. R. (2018). The science of teams in the military: contributions from over 60 years of research. Am. Psychol. 73, 322–333. doi: 10.1037/amp0000259
Haddaway, N. R., Grainger, M. J., and Gray, C. T. (2022). Citationchaser: a tool for transparent and efficient forward and backward citation chasing in systematic searching. Res. Synth. Methods 13, 533–545. doi: 10.1002/jrsm.1563
Hart-Pomerantz, H., Roe, E., and Brunyé, T. T. (2024). Effects of transcranial electrical stimulation on physiological responses to acute stress: a systematic review. J. Cogn. Enhanc. doi: 10.1007/s41465-024-00315-z
Hedrick, T. E., Bickman, L., and Rog, D. J. (1993). Applied research design: A practical guide. Newbury Park, CA: SAGE Publications.
Herrmann, C. S., Rach, S., Neuling, T., and Strüber, D. (2013). Transcranial alternating current stimulation: a review of the underlying mechanisms and modulation of cognitive processes. Front. Hum. Neurosci. 7:279. doi: 10.3389/fnhum.2013.00279
Hill, A. T., Fitzgerald, P. B., and Hoy, K. E. (2016). Effects of anodal transcranial direct current stimulation on working memory: a systematic review and meta-analysis of findings from healthy and neuropsychiatric populations. Brain Stimul. 9, 197–208. doi: 10.1016/j.brs.2015.10.006
Ho, K.-A., Taylor, J. L., Chew, T., Gálvez, V., Alonzo, A., Bai, S., et al. (2016). The effect of transcranial direct current stimulation (tDCS) electrode size and current intensity on motor cortical excitability: evidence from single and repeated sessions. Brain Stimul. 9, 1–7. doi: 10.1016/j.brs.2015.08.003
Horvath, J. C., Carter, O., and Forte, J. D. (2014). Transcranial direct current stimulation: five important issues we aren’t discussing (but probably should be). Front. Syst. Neurosci. 8:2. doi: 10.3389/fnsys.2014.00002
Horvath, J. C., Carter, O., and Forte, J. D. (2016). No significant effect of transcranial direct current stimulation (tDCS) found on simple motor reaction time comparing 15 different simulation protocols. Neuropsychologia 91, 544–552. doi: 10.1016/j.neuropsychologia.2016.09.017
Horvath, J. C., Forte, J. D., and Carter, O. (2015). Quantitative review finds no evidence of cognitive effects in healthy populations from single-session transcranial direct current stimulation (tDCS). Brain Stimul. 8, 535–550. doi: 10.1016/j.brs.2015.01.400
Imburgio, M. J., and Orr, J. M. (2018). Effects of prefrontal tDCS on executive function: methodological considerations revealed by meta-analysis. Neuropsychologia 117, 156–166. doi: 10.1016/j.neuropsychologia.2018.04.022
Jacobson, L., Koslowsky, M., and Lavidor, M. (2012). TDCS polarity effects in motor and cognitive domains: a meta-analytical review. Exp. Brain Res. 216, 1–10. doi: 10.1007/s00221-011-2891-9
Jotterand, F., and Ienca, M. (2023). The Routledge handbook of the ethics of human enhancement. 1st Edn. New York, NY: Routledge.
Jwa, A. (2015). Early adopters of the magical thinking cap: a study on do-it-yourself (DIY) transcranial direct current stimulation (tDCS) user community. J. Law Biosci. 2, 292–335. doi: 10.1093/jlb/lsv017
Kessler, S. K., Minhas, P., Woods, A. J., Rosen, A., Gorman, C., and Bikson, M. (2013). Dosage considerations for transcranial direct current stimulation in children: a computational Modeling study. PLoS One 8:e76112. doi: 10.1371/journal.pone.0076112
Khorrampanah, M., Seyedarabi, H., Daneshvar, S., and Farhoudi, M. (2020). Optimization of montages and electric currents in tDCS. Comput. Biol. Med. 125:103998. doi: 10.1016/j.compbiomed.2020.103998
Kim, A. Y., Marduy, A., de Melo, P. S., Gianlorenco, A. C., Kim, C. K., Choi, H., et al. (2022). Safety of transcutaneous auricular vagus nerve stimulation (taVNS): a systematic review and meta-analysis. Sci. Rep. 12:22055. doi: 10.1038/s41598-022-25864-1
Kim, K., Sherwood, M. S., McIntire, L. K., McKinley, R. A., and Ranganath, C. (2021). Transcranial direct current stimulation modulates connectivity of left dorsolateral prefrontal cortex with distributed cortical networks. J. Cogn. Neurosci. 33, 1381–1395. doi: 10.1162/jocn_a_01725
Ko, L.-W., Chang, Y., Wu, P.-L., Tzou, H.-A., Chen, S.-F., Tang, S.-C., et al. (2019). Development of a smart helmet for strategical BCI applications. Sensors 19:1867. doi: 10.3390/s19081867
Koizumi, K., Ueda, K., Li, Z., and Nakao, M. (2020). Effects of transcranial direct current stimulation on brain networks related to creative thinking. Front. Hum. Neurosci. 14:541052. doi: 10.3389/fnhum.2020.541052
Kortteenniemi, A., Ortega-Alonso, A., Javadi, A.-H., Tolmunen, T., Ali-Sisto, T., Kotilainen, T., et al. (2020). Anodal tDCS over the left prefrontal cortex does not cause clinically significant changes in circulating metabolites. Front. Psych. 11:403. doi: 10.3389/fpsyt.2020.00403
Krause, B., and Cohen Kadosh, R. (2014). Not all brains are created equal: the relevance of individual differences in responsiveness to transcranial electrical stimulation. Front. Syst. Neurosci. 8:25. doi: 10.3389/fnsys.2014.00025
Kunze, T., Hunold, A., Haueisen, J., Jirsa, V., and Spiegler, A. (2016). Transcranial direct current stimulation changes resting state functional connectivity: a large-scale brain network modeling study. NeuroImage 140, 174–187. doi: 10.1016/j.neuroimage.2016.02.015
Latheef, S., and Henschke, A. (2020). Can a soldier say no to an enhancing intervention? Philosophies 5:13. doi: 10.3390/philosophies5030013
Lefaucheur, J.-P., and Wendling, F. (2019). Mechanisms of action of tDCS: a brief and practical overview. Neurophysiol. Clin. 49, 269–275. doi: 10.1016/j.neucli.2019.07.013
Lieberman, H. R., Bathalon, G. P., Falco, C. M., Morgan, C. A., Niro, P. J., and Tharion, W. J. (2005). The fog of war: decrements in cognitive performance and mood associated with combat-like stress. Aviat. Space Environ. Med. 76, C7–C14.
Lieberman, H. R., Caruso, C. M., Niro, P. J., Adam, G. E., Kellogg, M. D., Nindl, B. C., et al. (2008). A double-blind, placebo-controlled test of 2 d of calorie deprivation: effects on cognition, activity, sleep, and interstitial glucose concentrations 12. Am. J. Clin. Nutr. 88, 667–676. doi: 10.1093/ajcn/88.3.667
Lieberman, H. R., Castellani, J. W., and Young, A. J. (2009). Cognitive function and mood during acute cold stress after extended military training and recovery. Aviat. Space Environ. Med. 80, 629–636. doi: 10.3357/ASEM.2431.2009
Lieberman, H. R., Niro, P., Tharion, W. J., Nindl, B. C., Castellani, J. W., and Montain, S. J. (2006). Cognition during sustained operations: comparison of a laboratory simulation to field studies. Aviat. Space Environ. Med. 77, 929–935
Lieberman, H. R., Tharion, W. J., Shukitt-Hale, B., Speckman, K. L., and Tulley, R. (2002). Effects of caffeine, sleep loss, and stress on cognitive performance and mood during U.S. navy SEAL training. Psychopharmacology 164, 250–261. doi: 10.1007/s00213-002-1217-9
Lu, H., Zhang, Y., Huang, P., Zhang, Y., Cheng, S., and Zhu, X. (2022). Transcranial electrical stimulation offers the possibility of improving teamwork among military pilots: a review. Front. Neurosci. 16:931265. doi: 10.3389/fnins.2022.931265
Luber, B. (2014). Neuroenhancement by noninvasive brain stimulation is not a net zero-sum proposition. Front. Syst. Neurosci. 8:127. doi: 10.3389/fnsys.2014.00127
Martin, K., McLeod, E., Periard, J., Rattray, B., Keegan, R., and Pyne, D. B. (2019). The impact of environmental stress on cognitive performance: A systematic review. 61, 1205–1246
Maslen, H., Douglas, T., Cohen Kadosh, R., Levy, N., and Savulescu, J. (2014). The regulation of cognitive enhancement devices: extending the medical model. J. Law Biosci. 1, 68–93. doi: 10.1093/jlb/lst003
Matsumoto, H., and Ugawa, Y. (2017). Adverse events of tDCS and tACS: a review. Clin. Neurophysiol. Pract. 2, 19–25. doi: 10.1016/j.cnp.2016.12.003
McIntire, L. K., McKinley, R. A., Goodyear, C., McIntire, J. P., and Brown, R. D. (2021). Cervical transcutaneous vagal nerve stimulation (ctVNS) improves human cognitive performance under sleep deprivation stress. Commun. Biol. 4, 634–639. doi: 10.1038/s42003-021-02145-7
McIntire, L. K., McKinley, R. A., Goodyear, C., and Nelson, J. M. (2014). A comparison of the effects of transcranial direct current stimulation and caffeine on vigilance and cognitive performance during extended wakefulness. Brain Stimul. 7, 499–507. doi: 10.1016/j.brs.2014.04.008
McIntire, L. K., McKinley, R. A., Nelson, J. M., and Goodyear, C. (2017). Transcranial direct current stimulation versus caffeine as a fatigue countermeasure. Brain Stimul. 10, 1070–1078. doi: 10.1016/j.brs.2017.08.005
McKinley, R. A., Bridges, N., Walters, C. M., and Nelson, J. (2012). Modulating the brain at work using noninvasive transcranial stimulation. NeuroImage 59, 129–137. doi: 10.1016/j.neuroimage.2011.07.075
Melby-Lervåg, M., Redick, T. S., and Hulme, C. (2016). Working memory training does not improve performance on measures of intelligence or other measures of “far transfer”: evidence from a meta-analytic review. Perspect. Psychol. Sci. 11, 512–534. doi: 10.1177/1745691616635612
Mencarelli, L., Menardi, A., Neri, F., Monti, L., Ruffini, G., Salvador, R., et al. (2020). Impact of network-targeted multichannel transcranial direct current stimulation on intrinsic and network-to-network functional connectivity. J. Neurosci. Res. 98, 1843–1856. doi: 10.1002/jnr.24690
Menuz, V., Hurlimann, T., and Godard, B. (2013). Is human enhancement also a personal matter? Sci. Eng. Ethics 19, 161–177. doi: 10.1007/s11948-011-9294-y
Minarik, T., Berger, B., Althaus, L., Bader, V., Biebl, B., Brotzeller, F., et al. (2016). The importance of sample size for reproducibility of tDCS effects. Front. Hum. Neurosci. 10:453. doi: 10.3389/fnhum.2016.00453
Molaee-Ardekani, B., Márquez-Ruiz, J., Merlet, I., Leal-Campanario, R., Gruart, A., Sánchez-Campusano, R., et al. (2013). Effects of transcranial direct current stimulation (tDCS) on cortical activity: a computational modeling study. Brain Stimul. 6, 25–39. doi: 10.1016/j.brs.2011.12.006
Nelson, J. M., Phillips, C. A., McKinley, R. A., McIntire, L. K., Goodyear, C., and Monforton, L. (2019). The effects of transcranial direct current stimulation (tDCS) on multitasking performance and oculometrics. Mil. Psychol. 31, 212–226. doi: 10.1080/08995605.2019.1598217
Niemeyer, K. (2009). Transformation: military and science. Inform. Secur. 23, 245–258. doi: 10.11610/isij.2319
Nikolin, S., Huggins, C., Martin, D., Alonzo, A., and Loo, C. K. (2018). Safety of repeated sessions of transcranial direct current stimulation: a systematic review. Brain Stimul. 11, 278–288. doi: 10.1016/j.brs.2017.10.020
Nikolin, S., Huggins, C., Martin, D., Alonzo, A., and Loo, C. (2019). Adverse events associated with repeated sessions of tDCS: a systematic review and meta-analysis. Brain Stimul. 12:483. doi: 10.1016/j.brs.2018.12.577
Nitsche, M. A., Cohen, L. G., Wassermann, E. M., Priori, A., Lang, N., Antal, A., et al. (2008). Transcranial direct current stimulation: state of the art 2008. Brain Stimul. 1, 206–223. doi: 10.1016/j.brs.2008.06.004
Nitsche, M. A., and Paulus, W. (2000). Excitability changes induced in the human motor cortex by weak transcranial direct current stimulation. J. Physiol. 527, 633–639. doi: 10.1111/j.1469-7793.2000.t01-1-00633.x
Opitz, A., Paulus, W., Will, S., Antunes, A., and Thielscher, A. (2015). Determinants of the electric field during transcranial direct current stimulation. NeuroImage 109, 140–150. doi: 10.1016/j.neuroimage.2015.01.033
Orasanu, J. M., and Backer, P. (1996). “Stress and military performance” in Stress and human performance. (Mahwah, NJ: Lawrence Erlbaum Associates, Inc), 89–125.
Paulus, W. (2014). Transcranial brain stimulation: potential and limitations. e-Neuroforum 5, 29–36. doi: 10.1007/s13295-014-0056-6
Peltier, C., Pettijohn, K., and Blacker, K. (2019). Developing the third offset: transcranial direct current stimulation can improve the human operator. Mil. Med. 184, 11–13. doi: 10.1093/milmed/usy197
Peña-Gómez, C., Sala-Lonch, R., Junqué, C., Clemente, I. C., Vidal, D., Bargalló, N., et al. (2012). Modulation of large-scale brain networks by transcranial direct current stimulation evidenced by resting-state functional MRI. Brain Stimul. 5, 252–263. doi: 10.1016/j.brs.2011.08.006
Pergher, V., Au, J., Alizadeh Shalchy, M., Santarnecchi, E., Seitz, A., Jaeggi, S. M., et al. (2022). The benefits of simultaneous tDCS and working memory training on transfer outcomes: a systematic review and meta-analysis. Brain Stimul. 15, 1541–1551. doi: 10.1016/j.brs.2022.11.008
Petrofsky, L. A., Heffernan, C. M., Gregg, B. T., and Smith-Forbes, E. V. (2022). Effects of sleep deprivation in military service members on cognitive performance: a systematic review. Mil. Behav. Health 10, 202–220. doi: 10.1080/21635781.2021.1982088
Prehn, K., and Flöel, A. (2015). Potentials and limits to enhance cognitive functions in healthy and pathological aging by tDCS. Front. Cell. Neurosci. 9:355. doi: 10.3389/fncel.2015.00355
Pupíková, M., Šimko, P., Lamoš, M., Gajdoš, M., and Rektorová, I. (2022). Inter-individual differences in baseline dynamic functional connectivity are linked to cognitive aftereffects of tDCS. Sci. Rep. 12:20754. doi: 10.1038/s41598-022-25016-5
Puscas, I. M. (2020). “Military enhancement: technologies, ethics, and operational issues” in Ethics of medical innovation, experimentation, and enhancement in military and humanitarian contexts (Springer, Cham, Switzerland: Springer), 127–146.
Rawji, V., Ciocca, M., Zacharia, A., Soares, D., Truong, D., Bikson, M., et al. (2018). tDCS changes in motor excitability are specific to orientation of current flow. Brain Stimul. 11, 289–298. doi: 10.1016/j.brs.2017.11.001
Reato, D., Salvador, R., Bikson, M., Opitz, A., Dmochowski, J., and Miranda, P. C. (2019). “Principles of transcranial direct current stimulation (tDCS): introduction to the biophysics of tDCS” in Practical guide to transcranial direct current stimulation: Principles, procedures and applications. eds. H. Knotkova, M. A. Nitsche, M. Bikson, and A. J. Woods (Springer, Cham, Switzerland: Springer International Publishing), 45–80.
Redgrave, J., Day, D., Leung, H., Laud, P. J., Ali, A., Lindert, R., et al. (2018). Safety and tolerability of transcutaneous Vagus nerve stimulation in humans; a systematic review. Brain Stimul. 11, 1225–1238. doi: 10.1016/j.brs.2018.08.010
Reyes, C., Padrón, I., Nila Yagual, S., and Marrero, H. (2021). Personality traits modulate the effect of tDCS on Reading speed of social sentences. Brain Sci. 11:1464. doi: 10.3390/brainsci11111464
Rosen, D. S., Erickson, B., Kim, Y. E., Mirman, D., Hamilton, R. H., and Kounios, J. (2016). Anodal tDCS to right dorsolateral prefrontal cortex facilitates performance for novice jazz improvisers but hinders experts. Front. Hum. Neurosci. 10:579. doi: 10.3389/fnhum.2016.00579
Sampaio-Junior, B., Tortella, G., Borrione, L., Moffa, A. H., Machado-Vieira, R., Cretaz, E., et al. (2018). Efficacy and safety of transcranial direct current stimulation as an add-on treatment for bipolar depression: a randomized clinical trial. JAMA Psychiatry 75, 158–166. doi: 10.1001/jamapsychiatry.2017.4040
Sánchez-Kuhn, A., Pérez-Fernández, C., Moreno, M., Flores, P., and Sánchez-Santed, F. (2018). Differential effects of transcranial direct current stimulation (tDCS) depending on previous musical training. Front. Psychol. 9:1465. doi: 10.3389/fpsyg.2018.01465
Santander, T., Leslie, S., Li, L. J., Skinner, H. E., Simonson, J. M., Sweeney, P., et al. (2024). Towards optimized methodological parameters for maximizing the behavioral effects of transcranial direct current stimulation. Front. Hum. Neurosci. 18:1305446. doi: 10.3389/fnhum.2024.1305446
Saturnino, G. B., Siebner, H. R., Thielscher, A., and Madsen, K. H. (2019). Accessibility of cortical regions to focal TES: dependence on spatial position, safety, and practical constraints. NeuroImage 203:116183. doi: 10.1016/j.neuroimage.2019.116183
Schoen, I., and Fromherz, P. (2008). Extracellular stimulation of mammalian neurons through repetitive activation of Na+ channels by weak capacitive currents on a silicon Chip. J. Neurophysiol. 100, 346–357. doi: 10.1152/jn.90287.2008
Sekhon, M., Cartwright, M., and Francis, J. J. (2022). Development of a theory-informed questionnaire to assess the acceptability of healthcare interventions. BMC Health Serv. Res. 22:279. doi: 10.1186/s12913-022-07577-3
Shenberger-Trujillo, J. M., and Kurinec, C. A. (2016). Bridging the research to application divide: recommendations for community-based participatory research in a military setting. Mil. Behav. Health 4, 316–324. doi: 10.1080/21635781.2016.1181580
Sherwood, M. S., McIntire, L., Madaris, A. T., Kim, K., Ranganath, C., and McKinley, R. A. (2021). Intensity-dependent changes in quantified resting cerebral perfusion with multiple sessions of transcranial DC stimulation. Front. Hum. Neurosci. 15:679977. doi: 10.3389/fnhum.2021.679977
Silvanto, J., Muggleton, N., and Walsh, V. (2008). State-dependency in brain stimulation studies of perception and cognition. Trends Cogn. Sci. 12, 447–454. doi: 10.1016/j.tics.2008.09.004
Small Business Association. (2024). Tutorial 1: An overview of DoD acquisition process. Available online at: SBIR.gov and https://www.sbir.gov/tutorials/acquisition-basics/tutorial-1
Smits, F. M., Geuze, E., De Kort, G. J., Kouwer, K., Geerlings, L., Van Honk, J., et al. (2023). Effects of multisession transcranial direct current stimulation on stress regulation and emotional working memory: a randomized controlled trial in healthy military personnel. Neuromodulation Technol. Neural Interface 26, 817–828. doi: 10.1016/j.neurom.2022.05.002
Splittgerber, M., Salvador, R., Brauer, H., Breitling-Ziegler, C., Prehn-Kristensen, A., Krauel, K., et al. (2020). Individual baseline performance and electrode montage impact on the effects of anodal tDCS over the left dorsolateral prefrontal cortex. Front. Hum. Neurosci. 14:349. doi: 10.3389/fnhum.2020.00349
Sterne, J. A. C., Savović, J., Page, M. J., Elbers, R. G., Blencowe, N. S., Boutron, I., et al. (2019). RoB 2: a revised tool for assessing risk of bias in randomised trials. BMJ. 366:l4898. doi: 10.1136/bmj.l4898
Sun, J.-B., Cheng, C., Tian, Q.-Q., Yuan, H., Yang, X.-J., Deng, H., et al. (2021). Transcutaneous auricular Vagus nerve stimulation improves spatial working memory in healthy Young adults. Front. Neurosci. 15:790793. doi: 10.3389/fnins.2021.790793
Thomas, M. L., and Russo, M. B. (2007). Neurocognitive monitors: toward the prevention of cognitive performance decrements and catastrophic failures in the operational environment. Aviat. Space Environ. Med. 78, B144–B152.
Truong, D. Q., Magerowski, G., Blackburn, G. L., Bikson, M., and Alonso-Alonso, M. (2013). Computational modeling of transcranial direct current stimulation (tDCS) in obesity: impact of head fat and dose guidelines. NeuroImage 2, 759–766. doi: 10.1016/j.nicl.2013.05.011
van der Groen, O. (2023). Focusing on the treatment acceptability of noninvasive brain stimulation. Neuromodulation 26, 705–706. doi: 10.1016/j.neurom.2023.01.004
Vartanian, O., Fraser, B., Saunders, D., Suurd Ralph, C., Lieberman, H. R., Morgan, C. A., et al. (2018). Changes in mood, fatigue, sleep, cognitive performance and stress hormones among instructors conducting stressful military captivity survival training. Physiol. Behav. 194, 137–143. doi: 10.1016/j.physbeh.2018.05.008
Vergallito, A., Varoli, E., Pisoni, A., Mattavelli, G., Del Mauro, L., Feroldi, S., et al. (2023). State-dependent effectiveness of cathodal transcranial direct current stimulation on cortical excitability. NeuroImage 277:120242. doi: 10.1016/j.neuroimage.2023.120242
Von Rosenberg, W., Chanwimalueang, T., Goverdovsky, V., Looney, D., Sharp, D., and Mandic, D. P. (2016). Smart helmet: wearable multichannel ECG and EEG. IEEE J. Transl. Engin. Health Med. 4, 1–11. doi: 10.1109/JTEHM.2016.2609927
Wagner, S., Burger, M., and Wolters, C. H. (2016). An optimization approach for well-targeted transcranial direct current stimulation. SIAM J. Appl. Math. 76, 2154–2174. doi: 10.1137/15M1026481
Wang, C., Cao, X., Gao, Z., Liu, Y., and Wen, Z. (2022). Training and transfer effects of combining inhibitory control training with transcutaneous Vagus nerve stimulation in healthy adults. Front. Psychol. 13:858938. doi: 10.3389/fpsyg.2022.858938
Wexler, A. (2016). A pragmatic analysis of the regulation of consumer transcranial direct current stimulation (TDCS) devices in the United States. J. Law Biosci. 2, 669–696. doi: 10.1093/jlb/lsv039
Wexler, A. (2018). Who uses direct-to-consumer brain stimulation products, and why? A study of home users of tDCS devices. J. Cogn. Enhanc. 2, 114–134. doi: 10.1007/s41465-017-0062-z
Wexler, A., and Reiner, P. B. (2017). “Home use of tDCS: from “do-it-yourself” to “direct-to-consumer.”” in The Routledge handbook of Neuroethics (Routledge).
Wexler, A., and Reiner, P. B. (2019). Oversight of direct-to-consumer neurotechnologies. Science 363, 234–235. doi: 10.1126/science.aav0223
Wilde, G. J. S. (1982). The theory of risk homeostasis: implications for safety and health. Risk Anal. 2, 209–225. doi: 10.1111/j.1539-6924.1982.tb01384.x
Willmot, N. S., Leow, L.-A., Filmer, H. L., and Dux, P. E. (2023). Failure of tDCS to impact militarised threat-detection in a military cohort. Imaging Neuroscience 1, 1–11. doi: 10.1162/imag_a_00004
Willmot, N. S., Leow, L.-A., Filmer, H. L., and Dux, P. E. (2024). Exploring the intra-individual reliability of tDCS: a registered report. Cortex 173, 61–79. doi: 10.1016/j.cortex.2023.12.015
Wittbrodt, M. T., and Millard-Stafford, M. (2018). Dehydration impairs cognitive performance: a meta-analysis. Med. Sci. Sports Exerc. 50, 2360–2368. doi: 10.1249/MSS.0000000000001682
Wörsching, J., Padberg, F., Helbich, K., Hasan, A., Koch, L., Goerigk, S., et al. (2017). Test-retest reliability of prefrontal transcranial direct current stimulation (tDCS) effects on functional MRI connectivity in healthy subjects. NeuroImage 155, 187–201. doi: 10.1016/j.neuroimage.2017.04.052
Xiang, S., Qi, S., Li, Y., Wang, L., Dai, D. Y., and Hu, W. (2021). Trait anxiety moderates the effects of tDCS over the dorsolateral prefrontal cortex (DLPFC) on creativity. Personal. Individ. Differ. 177:110804. doi: 10.1016/j.paid.2021.110804
Zaman, A., Khan, R. T., Karim, N., Nazrul Islam, M., Uddin, M. S., and Hasan, M. M. (2022). “Intelli-helmet: an early prototype of a stress monitoring system for military operations” in Information systems and management science. eds. L. Garg, N. Kesswani, J. G. Vella, P. A. Xuereb, M. F. Lo, and R. Diaz, et al. (Berlin, Germany: Springer International Publishing), 22–32.
Zappasodi, F., Musumeci, G., Navarra, R., Di Lazzaro, V., Caulo, M., and Uncini, A. (2018). Safety and effects on motor cortex excitability of five cathodal transcranial direct current stimulation sessions in 25 hours. Neurophysiol. Clin. 48, 77–87. doi: 10.1016/j.neucli.2017.09.002
Zhu, Y., Wu, D., Sun, K., Chen, X., Wang, Y., He, Y., et al. (2023). Alpha and theta oscillations are causally linked to interference inhibition: evidence from high-definition transcranial alternating current stimulation. Brain Sci. 13:1026. doi: 10.3390/brainsci13071026
Živanović, M., Paunović, D., Konstantinović, U., Vulić, K., Bjekić, J., and Filipović, S. R. (2021). The effects of offline and online prefrontal vs parietal transcranial direct current stimulation (tDCS) on verbal and spatial working memory. Neurobiol. Learn. Mem. 179:107398. doi: 10.1016/j.nlm.2021.107398
Keywords: human performance, transcranial electrical stimulation, transcranial direct current stimulation, transcranial alternating current stimulation, military, peripheral nerve stimulation, vagus nerve stimulation
Citation: van der Groen O, Rafique SA, Willmot N, Murphy MG, Tisnovsky E and Brunyé TT (2025) Transcutaneous and transcranial electrical stimulation for enhancing military performance: an update and systematic review. Front. Hum. Neurosci. 19:1501209. doi: 10.3389/fnhum.2025.1501209
Received: 24 September 2024; Accepted: 12 February 2025;
Published: 03 March 2025.
Edited by:
Jorge Leite, Portucalense University, PortugalReviewed by:
Alexander Hunold, Ilmenau University of Technology, GermanyCopyright © 2025 van der Groen, Rafique, Willmot, Murphy, Tisnovsky and Brunyé. This is an open-access article distributed under the terms of the Creative Commons Attribution License (CC BY). The use, distribution or reproduction in other forums is permitted, provided the original author(s) and the copyright owner(s) are credited and that the original publication in this journal is cited, in accordance with accepted academic practice. No use, distribution or reproduction is permitted which does not comply with these terms.
*Correspondence: Tad T. Brunyé, VGhhZGRldXMudC5CcnVueWUuY2l2QGFybXkubWls
Disclaimer: All claims expressed in this article are solely those of the authors and do not necessarily represent those of their affiliated organizations, or those of the publisher, the editors and the reviewers. Any product that may be evaluated in this article or claim that may be made by its manufacturer is not guaranteed or endorsed by the publisher.
Research integrity at Frontiers
Learn more about the work of our research integrity team to safeguard the quality of each article we publish.