- School of Biological and Health Systems Engineering, Arizona State University, Tempe, AZ, United States
Introduction: Proprioceptive error of estimated fingertip position in two-dimensional space is reduced with the addition of tactile stimulation applied at the fingertip. Tactile input does not disrupt the participants’ estimation strategy, as the individual error vector maps maintain their overall structure. This relationship suggests integration of proprioception and tactile information improves proprioceptive estimation, which can also be improved with trained mental focus and attention. Task attention and arousal are physiologically regulated by the reticular activating system (RAS), a brainstem circuit including the locus coeruleus (LC). There is direct and indirect evidence that these structures can be modulated by non-invasive trigeminal nerve stimulation (nTNS), providing an opportunity to examine nTNS effect on the integrative relationship of proprioceptive and tactile information.
Methods: Fifteen right-handed participants performed a simple right-handed proprioceptive estimation task with tactile feedback (touch) and no tactile (hover) feedback. Participants repeated the task after nTNS administration. Stimulation was delivered for 10 min, and stimulation parameters were 3,000 Hz, 50 μs pulse width, with a mean of 7 mA. Error maps across the workspace are generated using polynomial models of the participants’ target responses.
Results: Error maps did not demonstrate significant vector direction changes between conditions for any participant, indicating that nTNS does not disrupt spatial proprioception estimation strategies. A linear mixed model regression with nTNS epoch, tactile condition, and the interaction as factors demonstrated that nTNS reduced proprioceptive error under the hover condition only.
Discussion: We argue that nTNS does not disrupt spatial proprioceptive error maps but can improve proprioceptive estimation in the absence of tactile feedback. However, we observe no evidence that nTNS enhances tactile-proprioceptive integration as the touch condition does not exhibit significantly reduced error after nTNS.
1 Introduction
Non-invasive electrical neuromodulation provides an accessible, chemical free method to successfully induce systemic and effective changes in the cortex with short latency. Trigeminal and vagus nerve stimulation have seen recent success, altering brain state and helping treat anxiety (George et al., 2008; Hartley et al., 2023; Xiao et al., 2020; Burger et al., 2019; Verkuil and Burger, 2019), depression (Liu et al., 2020; Fang et al., 2016; Liu et al., 2018; Trevizol and Cordeiro, 2017), PTSD (Koek et al., 2019; Bottari et al., 2024; Trevizol et al., 2016), headache (Alt et al., 2020; Vecchio et al., 2018; Silberstein et al., 2016; Boström et al., 2019), and epilepsy (Xue et al., 2022; Hödl et al., 2020; Pop et al., 2011). Success has been seen in invasive implanted devices as well as non-invasive transcutaneous devices. The benefit of non-invasive trigeminal nerve stimulation (nTNS) and non-invasive vagus nerve stimulation (nVNS) exists in the accessibility of the method, requiring no implants nor surgery, and demonstrated effective somatosensory modulation.
The vagus and trigeminal nerves project directly to the brainstem (van Boekholdt et al., 2020; de Cicco et al., 2018; Broncel et al., 2020; Luckey et al., 2023; Schwarz and Luo, 2015; Zerari-Mailly et al., 2005), providing an avenue to affect cortical circuits regulating autonomic activity, including the reticular activating system (RAS) with the locus coeruleus (LC). The RAS and LC regulate arousal, attention, sleep, and somatosensory behaviors (Khroud et al., 2020). Multiple approaches demonstrate evidence that cranial nerve stimulation modulates these brainstem nuclei and consequentially modulates neocortex activity. Using blink reflex as an indirect model of subcortical activity, nTNS is shown to modulate brainstem circuits with long-term effects (Mercante et al., 2015; Pilurzi et al., 2016). In rodent models, cranial nerve stimulation directly drives LC firing rate (Hulsey et al., 2017), and increases cFOS expression in RAS nuclei and somatosensory cortex—indicating increased neural activity (Mercante et al., 2017). In humans, nTNS suppresses proprioceptive brainstem nuclei and nVNS activates the nucleus of the solitary tract and the LC, both integral to the RAS (Yakunina et al., 2017).
There is strong evidence of successful modulation of somatosensory perception and behavior via cranial nerve stimulation. Closed loop cranial nerve stimulation, specifically vagus nerve stimulation, has been successful in neurorehabilitation of motor function and tactile sensitivity after nerve damage or stroke (Darrow et al., 2020; Kilgard et al., 2018; Meyers et al., 2019). In these cases, neuromodulation was able to decrease pain sensitivity (Busch et al., 2013; Lerman et al., 2019), increase pain and pressure thresholds (Kilgard et al., 2018), and modulate tactile sensitivity (Darrow et al., 2020). Investigating nTNS in psychophysical tasks, it can improve motor learning (Arias and Buneo, 2022) or can decrease vigilance and attention depending on stimulation parameters (Piquet et al., 2011).
In summary, the neuroanatomical pathways linking both vagus and trigeminal nerves to relevant brainstem nuclei are similar. Benefits in anxiety, depression, and other pathologies are consistently observed with both modalities. Somatosensory plasticity and even changes in tactile-proprioceptive integration can be observed after neurostimulation as well. Reaction time tasks, measuring alertness and arousal, consistently demonstrate improvement in participants who are healthy (Lerman et al., 2022; Jongkees et al., 2018), enduring sleep deprivation (McIntire et al., 2021), or diagnoses with attention-deficit/hyperactivity disorder (McGough et al., 2019) that is linked to EEG alpha oscillations (Chen et al., 2023). We aim to further demonstrate the effect of nTNS on somatosensory and proprioceptive integration in an established task (Rincon-Gonzalez et al., 2011; Tanner et al., 2021) that requires a cognitive response evaluating perception rather than an reaction-time task relying on instinctual responses.
2 Methods
Participants. For this experiment, 17 participants were recruited to perform a right-handed proprioceptive estimation task with non-invasive trigeminal nerve stimulation. The task, parameters, and experimental protocols were reviewed and approved by the Institutional Review Board at Arizona State University.
Handedness. Handedness was self-reported by each participant, and the Edinburgh Handedness Inventory questionnaire (Oldfield, 1971) was used to confirm the handedness of each participant before the experiment. Only right-handed participants were included in the subsequent analysis as we have found differences in performance between the dominant and non-dominant hands (Rincon-Gonzalez et al., 2011), especially under novel stimulus conditions (Tanner et al., 2021). Out of the 17 participants recruited, 15 were right-handed with a mean age of 21.8 years old.
Task. Participants sat in front of a table with a 50 cm wide and 35 cm deep grid, consisting of 280 targets with alphanumeric and color assignments (Figure 1). A set of 75 random targets was chosen for each participant. Target sets were consistent for all iterations of a participant session and randomized between blocks. For each trial of the task, the participants held their hand a few centimeters above the edge of the workspace midline, close to their chest. With the participant’s eyes closed, the experimenter guided the participant’s hand to a target over the grid, held the hand in position with or without other input for 5 s, and then guided the hand back to the starting position. The participant could then open their eyes and, without moving their arm, report the estimated target by alphanumeric value and color, e.g., “A1Red.” At least one practice trial was explained and administered followed by verbal confirmation of trial comprehension. Trials were only aborted and repeated if the experimenter accidentally touched the participants hand to the table in the process of approaching the target.
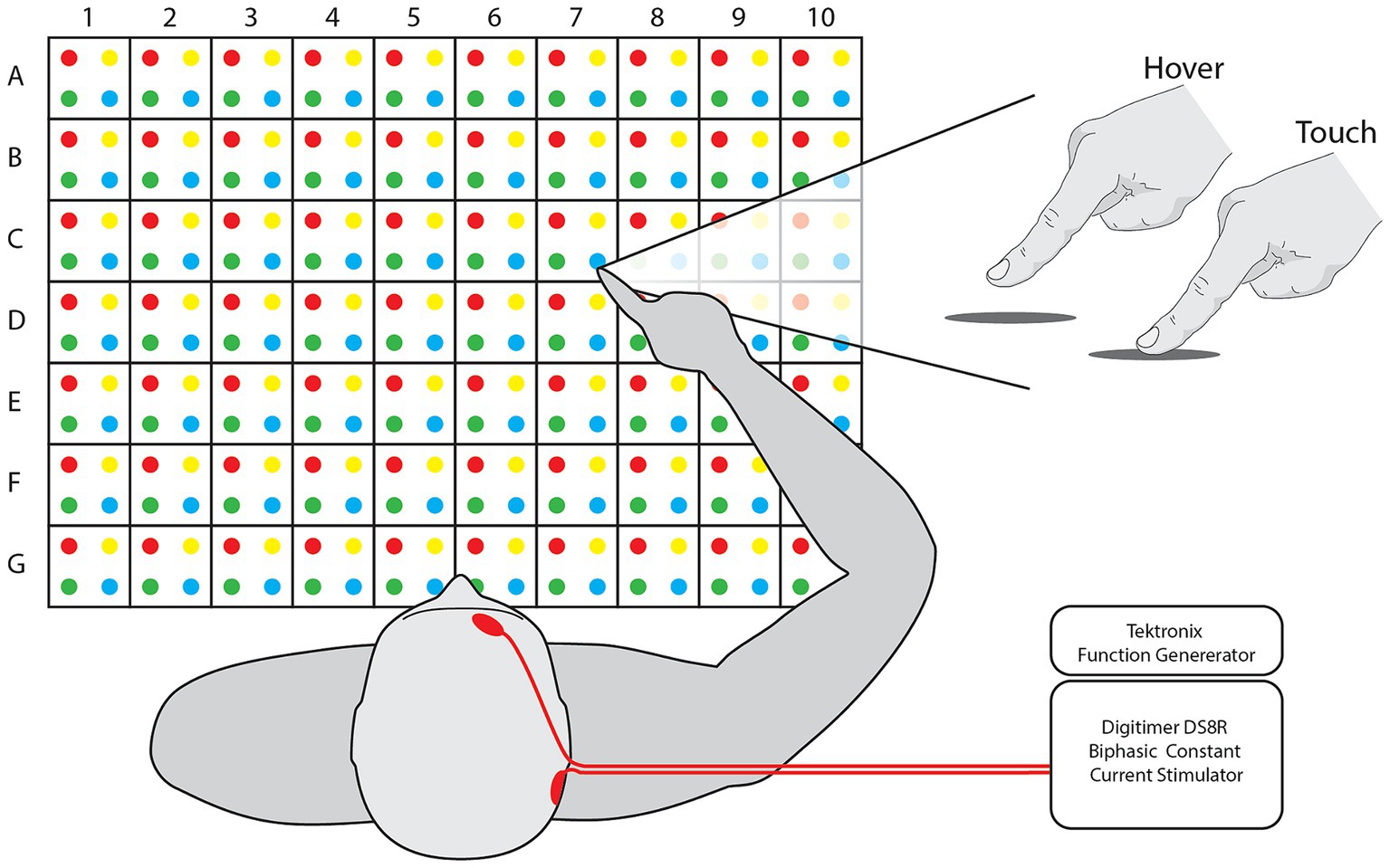
Figure 1. Workspace and feedback. [Left] Participants sit in front of a grid of dots with 2.5 cm spacing between them, creating a workspace 50 cm wide and 35 cm deep. Targets and responses are referred to via their numerical value, alphabetical letter, and color, e.g., A1Red. [Right] Participant finger position for each feedback mode. [Inset] nTNS was delivered with Tektronix and Digitimer equipment. Electrode placement is indicated on the illustrated participant’s head.
The task was performed in four separate blocks: for both hover and touch conditions before and after administration of nTNS. This resulted in four blocks of two factors with two levels: Condition [Hover and Touch] and Epoch [Baseline and nTNS]. In the Hover condition, the participant’s hand was kept above the table and no tactile input was provided before returning to the starting position. In the Touch condition, the experimenter moved the participant’s hand to the target, then vertically lowered it to the table, allowing contact for 5 s before raising the hand and returning it to the starting position.
Neuromodulatory stimulation was delivered via a Digitimer DS8R stimulator after the baseline blocks of hover and touch trials. The positive electrode was placed above the right eyebrow, near the foramen of the ophthalmic branch of the trigeminal nerve, and the ground was placed on the mastoid behind the ipsilateral ear. We used 1.5″ round Axelgaard PALS neurostimulation electrodes and adhered them in place with medical tape if necessary. The stimulation was delivered as biphasic, symmetrical 50 microsecond pulses delivered at 3,000 Hz (Arias and Buneco, 2022). Pulse amplitude was determined individually for each participant, starting at 2 mA and increasing until the participant reported the sensation as “tolerable but clear,” (4–9 mA, mean = 7 mA). The stimuli were controlled with a Tektronix function generator set to stimulate in one of two timing paradigms. The “Constant” paradigm consists of constant stimulation over a 10-min window. The “Cycling” paradigm consists of stimulation cycled on and off at 30 s intervals across a 10-min window. Participants were randomly separated into one paradigm group, resulting in 8 participants for Constant and 7 participants for Cycle. Immediately following the nTNS delivery, the participants performed the second set of hover and touch trials. Due to low sample size and to ensure statistical confidence, no comparisons between nTNS paradigms were analyzed. All 15 participants were pooled into a single group and considered equal as recipients of nTNS.
Analysis. Using a participant’s estimations of the 75 targets, we first transformed the raw error magnitude and direction across the entire sampled workspace. To obtain estimations across the entire workspace, X and Y components of the error vector were modeled with 4th order polynomial regressions. We used these models to calculate an error estimate for each of the 280 potential targets. It is necessary to this analysis that the targets are consistent across each block in order compare the results across sessions. Figure 2 illustrates this process for a single participant’s raw and calculated error from feedback conditions before stimulation. The first two columns illustrate the error maps alone, but the third column overlays the two maps to visualize comparison. Both error map shape and error magnitude need to be evaluated statistically, and all reported analyses were performed on each the calculated error generated from the polynomial models (bottom row of Figure 2).
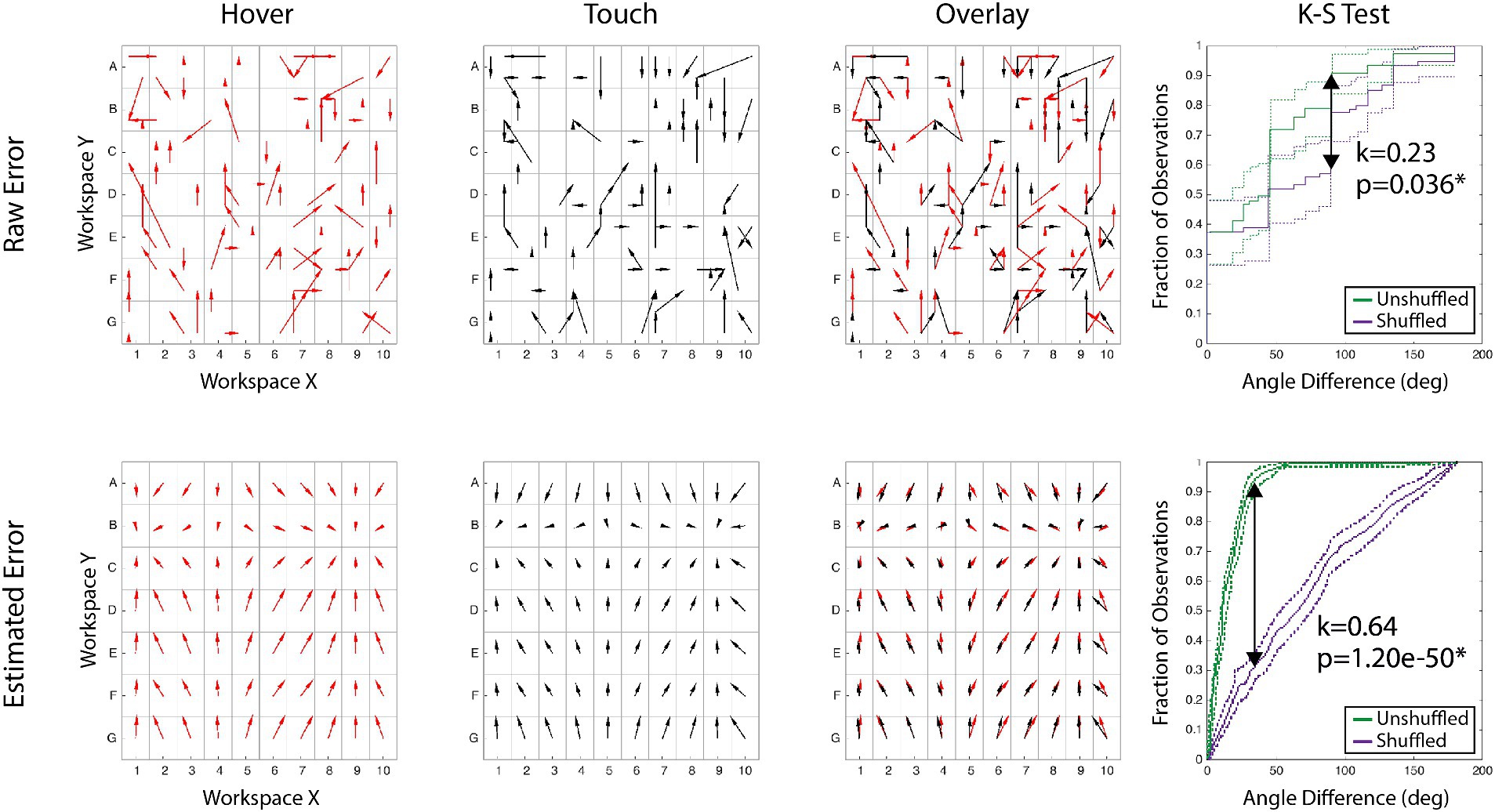
Figure 2. Data processing example. Arrows show proprioceptive error magnitude and direction for the hover and touch conditions. Each tail is the target, and each head is the response. [Top Row] Raw error of actual responses to targets for two conditions, the overlay, and the comparison of the error maps. [Bottom Row] Modeled error of the same conditions constructed for each alphanumeric grid. The right column demonstrates the K-S test statistical process: the maximum span between the shuffled and unshuffled error distributions is denoted as ‘k’ and accompanied by the p-value of the test.
To evaluate the individual difference of error magnitude between blocks, a Wilcoxon sign-ranked test was performed on the vector magnitudes. This was performed within participants for each pair of blocks, and Bonferroni corrected for multiple comparisons.
For a comprehensive analysis of the effect nTNS has on proprioceptive error magnitude, a linear mixed-effect model (Equation 1) was implemented with Epoch and Condition as factors, including the interaction effect. For the independent variable, we used proprioceptive error for each potential target. Participant ID was included as a random effect to mitigate discrepancies in baseline performance.
To determine if the shape of the error map is maintained between blocks, we employed the Kolmogorov–Smirnov (K-S) test. Thoroughly explained in Rincon-Gonzalez et al. (2011), the K-S test non-parametrically compares the distribution of two variables. Comparing error distributions directly between blocks does not adequately account for variance across the workspace. In this study, we use the K-S test to compare the difference in error distribution (angle between response vectors of two blocks) and a randomized error distribution (angle between response vector of two blocks, with the second block’s vectors shuffled across the workspace). An unshuffled distribution represents each target’s error vector’s angular difference between feedback modes. A shuffled distribution is built by finding the angular difference between one feedback mode’s actual error vector at a target and a randomly shuffled target’s error vector for the second mode. If the unshuffled error vectors are similar, the angular difference is often small, creating a steep cumulative distribution function (CDF). Both an error map with varied vector differences and a shuffled set would have CDFs that are more linear. Therefore, if the maximum difference between of the populations’ CDFs is sufficiently large, then the error map shapes are significantly similar. An example of this is presented in the far-right column of Figure 2 for both raw and calculated error, displaying the shuffled and unshuffled error distributions. As proprioceptive error maps are idiosyncratic, K-S tests were performed within participants for each pair of blocks, and Bonferroni corrected for multiple comparisons.
3 Results
To evaluate the effect of nTNS on the map of proprioceptive error, the magnitude and shape of error were tested between blocks: hover and touch before and after nTNS. These experiments provided raw error across 75 targets of the workspace (Figure 2, Top Row). Analyses were then performed on 4th order polynomial models of each participants’ X and Y error, evaluated at each of the 280 target locations (Figure 2, Bottom Row). R2 coefficients of the 4th order polynomial fits were at minimum 0.92, averaged 0.96, and are displayed in Table 1. Using the resultant vectors, a Kolmogorov–Smirnov test compared the spatial structure between modes and a two-sample t-test compared the magnitude of error.
Error maps of each block and comparisons between each block for a single participant, as well as that participant’s statistical results, are included in Figure 3. Visually, it is apparent the spatial structure is maintained across all modes and as detailed in Table 2; the significant K-S test results corroborate. Table 2 outlines the statistical results of both tests with respective k values (spatial structure) and change in mean error (ΔM). Comparing vector directions using the K-S test shows significantly similar spatial structures in 90/90 cases. Neither tactile condition nor nTNS epoch alters the spatial structure of individual proprioceptive error in this task.
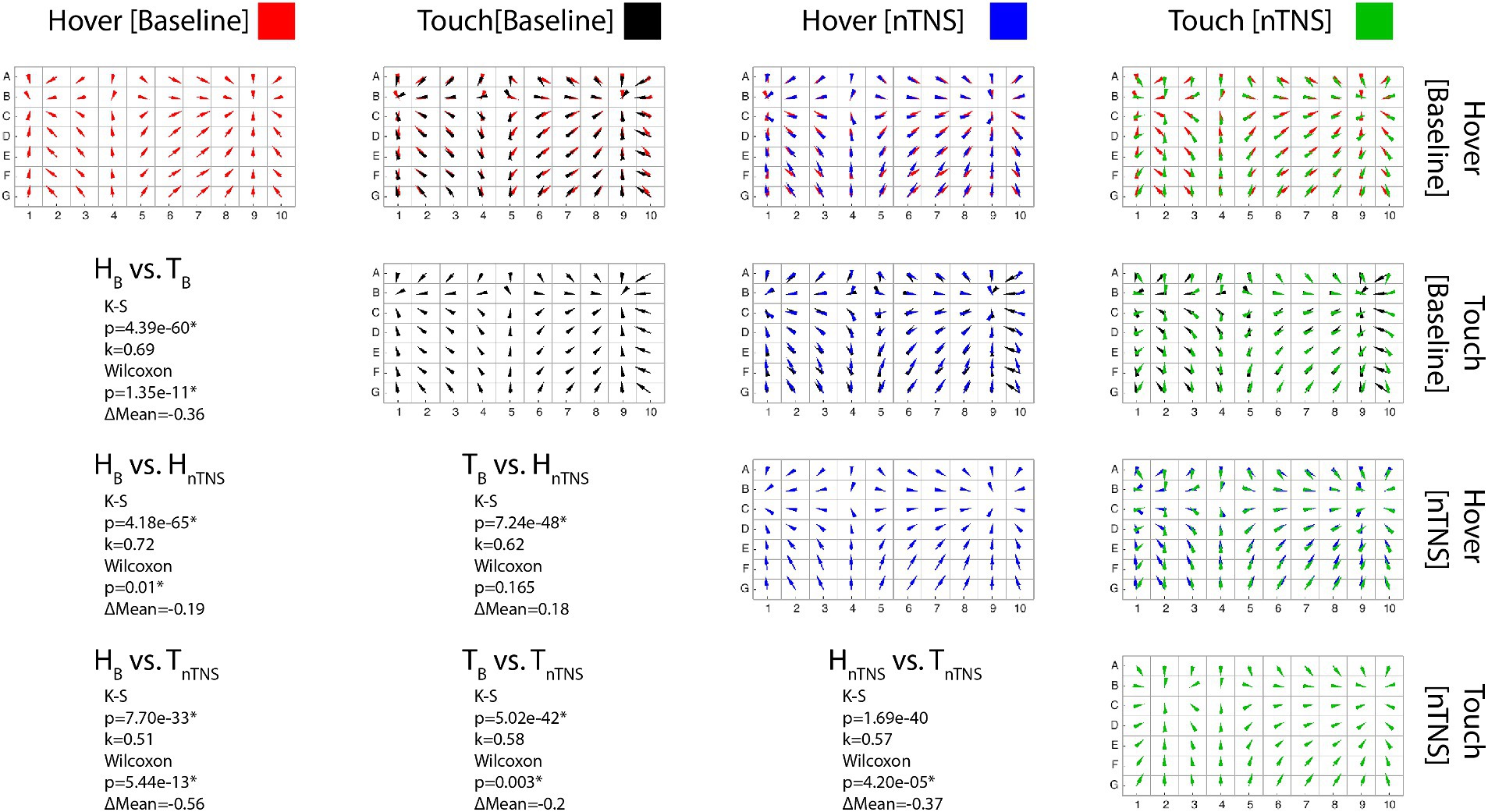
Figure 3. Error maps comparisons. [Diagonal] Each error map shows the proprioceptive error direction and magnitude across the workspace for a single participant. (Red: Hover at Baseline; Black: Touch at Baseline; Blue: Hover after Modulation; Green: Touch after Modulation) [Upper Triangle] Conditions overlayed in each row/column pair to compare maps. [Lower Triangular] Statistical results for each pair. K-S significance implies the maps possess statistically similar shapes. Wilcoxon significance implies a difference in the error means, where positive ΔM values indicate increased mean error in the latter mode.
In Table 2, we also show comparisons of error magnitude between blocks. We found significant differences in 63/90 Wilcoxon sign-ranked tests after adjusting for multiple comparisons via Bonferroni’s correction. This table also provides directionality of the error changes, and more participants showed improved hover error than improved touch error after nTNS. Before nTNS, we found the hover condition had higher error in 9 of the 11 significant cases. After nTNS, this was only true for 7 of the 12 significant cases. Fewer cases were present as there was a lower hover condition error in seven participants after nTNS. This was less pronounced in the touch condition, which showed significantly lower error after nTNS in only five participants.
To determine comprehensive results of nTNS effect on error, we implemented a linear mixed-effect model with Condition and Epoch as factors, including the interaction effect. Coefficients and p-values for this model are listed in Table 3 and illustrated in Figure 4. Tactile condition (β2 = −0.35, p < 0.001) and nTNS epoch (β1 = −0.42, p < 0.001) are significant, suggesting lower error during touch and lower error after nTNS. The interaction term was also significant (β3 = 0.323, p < 0.001). This overall model supports the conclusion that tactile information and nTNS reduce proprioceptive error, but the relationship is unclear and prompts post-hoc analysis.
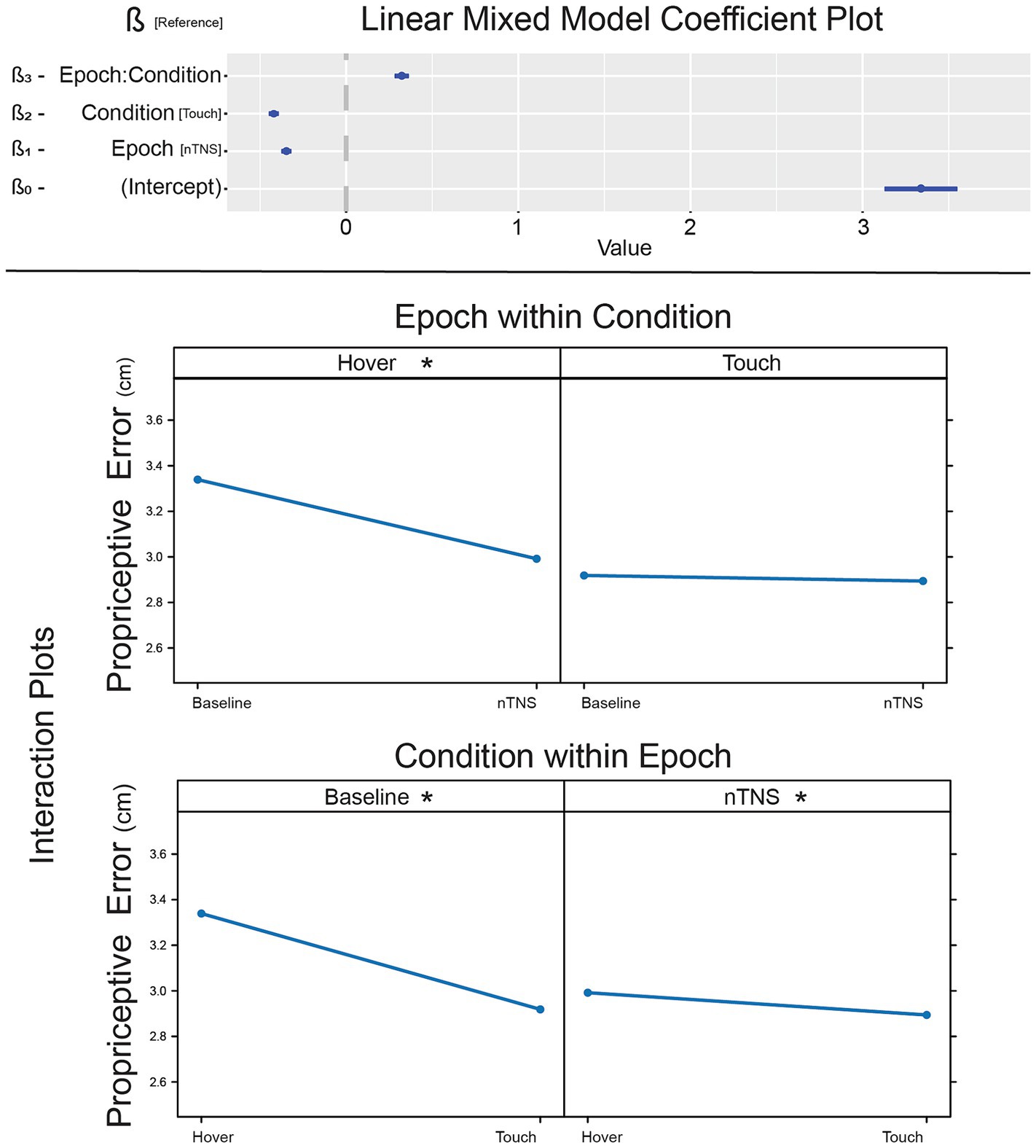
Figure 4. Effect of nTNS on proprioceptive error. [Top] Coefficients of the linear mixed model demonstrating significant factors and interaction effect. [Bottom] Interaction plots of nTNS epochs against touch conditions. *significant post-hoc pairwise comparison.
Post-hoc tests were conducted to explore pairwise differences between the two factors: Condition and Epoch (Figure 4). Pairwise comparisons are evaluated employing the Tukey adjustment for multiple comparisons, with estimates and p-values listed in Table 3. The baseline hover block exhibited more error than baseline and modulated touch (ZA = 14.44, p < 0.001; ZB = 11.925, p < 0.001), which is expected from previous literature (Rincon-Gonzalez et al., 2011; Tanner et al., 2021). After modulation, proprioception without somatosensation (hover) exhibits reduced error (ZC = 15.279, p < 0.001). Hover error is reduced to a level no different than unmodulated (ZD = −2.515, p = 0.0576) or modulated (ZE = 0.838, p = 0.8361) proprioceptive-tactile integration. While the ß-estimate comparing modulated hover against modulated touch is smaller than other significant results, hover error is still significantly higher than touch error after nTNS is administered (ZF = 3.354, p = 0.0044), which is congruent with unmodulated hover and touch in this paper literature (Rincon-Gonzalez et al., 2011; Tanner et al., 2021).
4 Conclusion
To investigate the effect of non-invasive Trigeminal Nerve Stimulation (nTNS) on tactile-proprioceptive integration, this study observed proprioceptive error in the presence and absence of tactile information before and after nTNS. Using polynomial estimation of end-point estimation error across a workspace, we statistically compared both the error magnitude and the map of proprioceptive error angles. Our primary conclusion is a reduction in pure proprioceptive error with no effect on tactile-proprioceptive integration and no disruption of proprioceptive error maps.
For each of the 15 participants, six pairwise comparisons of error shapes were completed across the four blocks of trials: hover and touch conditions before and after nTNS administration. In all 90 comparisons, K-S tests conclude there were no significant differences in vector magnitude distributions. Confirming previous literature, the proprioceptive error maps were stable across blocks (Tanner et al., 2021; Rincon-Gonzalez et al., 2012). We can contribute the novel conclusion that nTNS does not change error maps and they remain stable after modulation. As nTNS and other cranial nerve stimulation modalities are utilized in neuromuscular or motor rehabilitation (Cook et al., 2020; Fallahi et al., 2023; Gao et al., 2023), this offers assurance that neuromodulation will not acutely confound existing movement or estimation strategies.
In our linear mixed effect model, we see a general decrease in the error when tactile information is integrated, with significant pairwise comparisons between each Hover and Touch pair. Integrating tactile feedback always produces less error than pure proprioception. This relationship is present before nTNS within the baseline epoch, which confirms previous literature. After nTNS, the same tactile-proprioceptive integration reduction in error is present, but to a smaller degree. The other pairwise comparisons offer interesting insights to the nTNS effects. Pure proprioception after nTNS (nTNS Hover) is not significantly different than using tactile feedback before nTNS (baseline Touch), suggesting error estimation improved unrelated to improved tactile-proprioceptive integration. This could imply heightened attention to the task in general or a better ability to interpret the proprioceptive information alone.
The lack of significant difference between the touch condition in both epochs confirms the lack of nTNS effect on tactile-proprioceptive integration. The similarity between baseline touch and nTNS hover is striking, as it suggests modulated proprioception is comparable to default tactile-proprioceptive integration. Without defining the mechanisms, we can conclude that acute nTNS allows a single channel of proprioceptive information to be comparable to bimodal information in a task requiring a cognitive response. Sensory perception May be a function of attention which can be modulated by nTNS, while tactile-proprioceptive integration is related to previous sensory experience and sensory familiarity (Tanner et al., 2021). As tactile-proprioceptive integration is unaffected by acute neuromodulation in this study, error is likely modified by attention and not integration. It is unknown if this would be consistent in a task with accelerated responses that requires higher attentional demand, such as a race-model reaction time task with a single versus bimodal stimuli. Accelerated tasks indirectly model tactile-proprioceptive neural summation independent of cognitive task (Forster et al., 2002; Zehetleitner et al., 2015), and would efficiently probe sub-perceptual integration.
Alternatively, the results suggest the possibility that the difference between hover and touch May reflect a process separate from tactile-proprioceptive integration: somatosensory LC activation. Our working hypothesis of the nTNS effect is that it activates attention and arousal via synaptic input from the trigeminal nucleus to the LC through direct synaptic inputs from the nucleus of the solitary tract (NTS; Schwarz and Luo, 2015; Zerari-Mailly et al., 2005; Contreras et al., 1982), but also transit through the nucleus paragigantocellularis, prepositus hypoglossi, and the reticular formation (de Cicco et al., 2018). Tactile input activates LC at short latencies, exhibiting enhanced firing rates like arousal states (Foote et al., 1980). This raises the possibility that lower error in baseline touch conditions is due to increased arousal. Without nTNS, this would be due to heightened LC activity from natural tactile input (Rincon-Gonzalez et al., 2011). In our nTNS epoch conditions, we suggest nTNS induces LC arousal and both hover and touch conditions demonstrate decreased, but comparable, error. In short, nTNS induces an arousal state that tactile inputs normally provide, causing hover trials to exhibit similar error to touch trials.
While the comprehensive analysis demonstrates compelling results, Table 2 illustrates some variability in the individual response to nTNS. Specifically, participants JA and NB demonstrate a poor response to the nTNS, either with significantly higher error or no significant difference in epochs. However, both participants also demonstrate significantly higher error in the baseline epoch touch condition versus the hover condition. This is contradictory with typical tactile-proprioceptive integration and could indicate poor task comprehension or poor default tactile-proprioceptive integration. Regardless of these cases, it is clear not all subjects demonstrate a reduction in proprioceptive error after receiving nTNS. From literature, we know there is variability in individual response to neuromodulation, specifically for motor rehabilitation. This variability has been linked to specific biomarkers such as genetic polymorphisms (Cheeran et al., 2008; Antal et al., 2010; Fritsch et al., 2010). This study is not powered to evaluate genetics, nor is it in the scope of the resources. However, such sources of variability are necessary to consider in effective larger studies.
Data availability statement
The original contributions presented in the study are publicly available. This data can be found here: https://osf.io/2mzng/?view_only=3cbc95a17a144de8b47b2d801c73ce4e.
Ethics statement
The studies involving humans were approved by Arizona State University Institutional Review Board. The studies were conducted in accordance with the local legislation and institutional requirements. The participants provided their written informed consent to participate in this study.
Author contributions
JT: Conceptualization, Data curation, Formal analysis, Investigation, Methodology, Project administration, Supervision, Visualization, Writing – original draft, Writing – review & editing. GO: Conceptualization, Data curation, Formal analysis, Investigation, Methodology, Project administration, Supervision, Writing – original draft, Writing – review & editing. SH: Conceptualization, Investigation, Methodology, Project administration, Resources, Supervision, Writing – review & editing.
Funding
The author(s) declare that no financial support was received for the research, authorship, and/or publication of this article.
Conflict of interest
The authors declare that the research was conducted in the absence of any commercial or financial relationships that could be construed as a potential conflict of interest.
Publisher’s note
All claims expressed in this article are solely those of the authors and do not necessarily represent those of their affiliated organizations, or those of the publisher, the editors and the reviewers. Any product that may be evaluated in this article, or claim that may be made by its manufacturer, is not guaranteed or endorsed by the publisher.
References
Alt, L. K., Wach, K., Liebler, E. J., Straube, A., and Ruscheweyh, R. (2020). A randomized sham-controlled cross-over study on the short-term effect of non-invasive cervical Vagus nerve stimulation on spinal and Supraspinal nociception in healthy subjects. Headache 60, 1616–1631. doi: 10.1111/head.13891
Antal, A., Chaieb, L., Moliadze, V., Monte-Silva, K., Poreisz, C., Thirugnanasambandam, N., et al. (2010). Brain-derived neurotrophic factor (BDNF) gene polymorphisms shape cortical plasticity in humans. Brain Stimul. 3, 230–237. doi: 10.1016/J.BRS.2009.12.003
Arias, D. E., and Buneo, C. A. (2022). Effects of kilohertz electrical stimulation of the trigeminal nerve on motor learning. Proc Annu Int Conf IEEE Eng Med Biol Soc EMBS 2022, 5103–5106. doi: 10.1109/EMBC48229.2022.9871095
Boström, A., Scheele, D., Stoffel-Wagner, B., Hönig, F., Chaudhry, S. R., Muhammad, S., et al. (2019). Saliva molecular inflammatory profiling in female migraine patients responsive to adjunctive cervical non-invasive vagus nerve stimulation: the MOXY study. J. Transl. Med. 17:53. doi: 10.1186/s12967-019-1801-y
Bottari, S. A., Lamb, D. G., Porges, E. C., Murphy, A. J., Tran, A. B., Ferri, R., et al. (2024). Preliminary evidence of transcutaneous vagus nerve stimulation effects on sleep in veterans with post-traumatic stress disorder. J. Sleep Res. 33:e13891. doi: 10.1111/JSR.13891
Broncel, A., Bocian, R., Kłos-Wojtczak, P., Kulbat-Warycha, K., and Konopacki, J. (2020). Vagal nerve stimulation as a promising tool in the improvement of cognitive disorders. Brain Res. Bull. 155, 37–47. doi: 10.1016/J.BRAINRESBULL.2019.11.011
Burger, A. M., van Diest, I., van der Does, W., Korbee, J. N., Waziri, N., Brosschot, J. F., et al. (2019). The effect of transcutaneous vagus nerve stimulation on fear generalization and subsequent fear extinction. Neurobiol. Learn. Mem. 161, 192–201. doi: 10.1016/J.NLM.2019.04.006
Busch, V., Zeman, F., Heckel, A., Menne, F., Ellrich, J., and Eichhammer, P. (2013). The effect of transcutaneous vagus nerve stimulation on pain perception – an experimental study. Brain Stimul. 6, 202–209. doi: 10.1016/j.brs.2012.04.006
Cheeran, B., Talelli, P., Mori, F., Koch, G., Suppa, A., Edwards, M., et al. (2008). A common polymorphism in the brain-derived neurotrophic factor gene (BDNF) modulates human cortical plasticity and the response to rTMS. J. Physiol. 586, 5717–5725. doi: 10.1113/jphysiol.2008.159905
Chen, Y., Lu, X., and Hu, L. (2023). Transcutaneous auricular Vagus nerve stimulation facilitates cortical arousal and alertness. Int. J. Environ. Res. Public Health 20:1402. doi: 10.3390/ijerph20021402
Contreras, R. J., Beckstead, R. M., and Norgren, R. (1982). The central projections of the trigeminal, facial, glossopharyngeal and vagus nerves: an autoradiographic study in the rat. J. Auton. Nerv. Syst. 6, 303–322. doi: 10.1016/0165-1838(82)90003-0
Cook, D. N., Thompson, S., Stomberg-Firestein, S., Bikson, M., George, M. S., Jenkins, D. D., et al. (2020). Design and validation of a closed-loop, motor-activated auricular vagus nerve stimulation (MAAVNS) system for neurorehabilitation. Brain Stimul. 13, 800–803. doi: 10.1016/J.BRS.2020.02.028
Darrow, M. J., Mian, T. M., Torres, M., Haider, Z., Danaphongse, T., Rennaker, R. L. Jr., et al. (2020). Restoration of somatosensory function by pairing Vagus nerve stimulation with tactile rehabilitation. Ann. Neurol. 87, 194–205. doi: 10.1002/ana.25664
de Cicco, V., Tramonti Fantozzi, M. P., Cataldo, E., Barresi, M., Bruschini, L., Faraguna, U., et al. (2018). Trigeminal, visceral and vestibular inputs may improve cognitive functions by acting through the locus coeruleus and the ascending reticular activating system: a new hypothesis. Front. Neuroanat. 11:130. doi: 10.3389/fnana.2017.00130
Fallahi, M. S., Azadnajafabad, S., Maroufi, S. F., Pour-Rashidi, A., Khorasanizadeh, M. H., Sattari, S. A., et al. (2023). Application of Vagus nerve stimulation in spinal cord injury rehabilitation. World Neurosurg. 174, 11–24. doi: 10.1016/j.wneu.2023.02.101
Fang, J., Rong, P., Hong, Y., Fan, Y., Liu, J., Wang, H., et al. (2016). Transcutaneous vagus nerve stimulation modulates default mode network in major depressive disorder. Biol. Psychiatry 79, 266–273. doi: 10.1016/J.BIOPSYCH.2015.03.025
Foote, S. L., Aston-Jones, G., and Bloom, F. E. (1980). Impulse activity of locus coeruleus neurons in awake rats and monkeys is a function of sensory stimulation and arousal. Proc. Natl. Acad. Sci. USA 77, 3033–3037. doi: 10.1073/pnas.77.5.3033
Forster, B., Cavina-Pratesi, C., Aglioti, S. M., and Berlucchi, G. (2002). Redundant target effect and intersensory facilitation from visual-tactile interactions in simple reaction time. Exp. Brain Res. 143, 480–487. doi: 10.1007/s00221-002-1017-9
Fritsch, B., Reis, J., Martinowich, K., Schambra, H. M., Ji, Y., Cohen, L. G., et al. (2010). Direct current stimulation promotes BDNF-dependent synaptic plasticity: potential implications for motor learning. Neuron 66, 198–204. doi: 10.1016/j.neuron.2010.03.035
Gao, Y., Zhu, Y., Lu, X., Wang, N., Zhu, S., Gong, J., et al. (2023). Vagus nerve stimulation paired with rehabilitation for motor function, mental health and activities of daily living after stroke: a systematic review and meta-analysis. J. Neurol. Neurosurg. Psychiatry 94, 257–266. doi: 10.1136/JNNP-2022-329275
George, M. S., Ward, H. E., Ninan, P. T., Pollack, M., Nahas, Z., Anderson, B., et al. (2008). A pilot study of vagus nerve stimulation (VNS) for treatment-resistant anxiety disorders. Brain Stimul. 1, 112–121. doi: 10.1016/j.brs.2008.02.001
Hartley, S., Bao, G., Zagdoun, M., Chevallier, S., Lofaso, F., Leotard, A., et al. (2023). Noninvasive Vagus nerve stimulation: a new therapeutic approach for Pharmacoresistant restless legs syndrome. Neuromodulation 26, 629–637. doi: 10.1016/j.neurom.2022.10.046
Hödl, S., Carrette, S., Meurs, A., Carrette, E., Mertens, A., Gadeyne, S., et al. (2020). Neurophysiological investigations of drug resistant epilepsy patients treated with vagus nerve stimulation to differentiate responders from non-responders. Eur. J. Neurol. 27, 1178–1189. doi: 10.1111/ENE.14270
Hulsey, D. R., Riley, J. R., Loerwald, K. W., Rennaker, R. L., Kilgard, M. P., and Hays, S. A. (2017). Parametric characterization of neural activity in the locus coeruleus in response to vagus nerve stimulation. Exp. Neurol. 289, 21–30. doi: 10.1016/j.expneurol.2016.12.005
Jongkees, B. J., Immink, M. A., Finisguerra, A., and Colzato, L. S. (2018). Transcutaneous vagus nerve stimulation (tVNS) enhances response selection during sequential action. Front. Psychol. 9:347635. doi: 10.3389/FPSYG.2018.01159/BIBTEX
Khroud, N. K., Reddy, V., and Saadabadi, A. (2020). Neuroanatomy, Locus Ceruleus. US: Stat Pearls Publishing.
Kilgard, M. P., Rennaker, R. L., Alexander, J., and Dawson, J. (2018). Vagus nerve stimulation paired with tactile training improved sensory function in a chronic stroke patient. NeuroRehabilitation 42, 159–165. doi: 10.3233/NRE-172273
Koek, R. J., Roach, J., Athanasiou, N., van 't Wout-Frank, M., and Philip, N. S. (2019). Neuromodulatory treatments for post-traumatic stress disorder (PTSD). Prog. Neuro-Psychopharmacol. Biol. Psychiatry 92, 148–160. doi: 10.1016/J.PNPBP.2019.01.004
Lerman, I., Davis, B., Huang, M., Huang, C., Sorkin, L., Proudfoot, J., et al. (2019). Noninvasive vagus nerve stimulation alters neural response and physiological autonomic tone to noxious thermal challenge. PLoS One 14:e0201212. doi: 10.1371/JOURNAL.PONE.0201212
Lerman, I., Klaming, R., Spadoni, A., Baker, D. G., and Simmons, A. N. (2022). Non-invasive cervical vagus nerve stimulation effects on reaction time and valence image anticipation response. Brain Stimul. 15, 946–956. doi: 10.1016/J.BRS.2022.06.006
Liu, A., Rong, P., Gong, L., Song, L., Wang, X., Li, L., et al. (2018). Efficacy and safety of treatment with transcutaneous vagus nerve stimulation in 17 patients with refractory epilepsy evaluated by electroencephalogram, seizure frequency, and quality of life. Med. Sci. Monit. 24, 8439–8448. doi: 10.12659/MSM.910689
Liu, C. H., Yang, M. H., Zhang, G. Z., Wang, X. X., Li, B., Li, M., et al. (2020). Neural networks and the anti-inflammatory effect of transcutaneous auricular vagus nerve stimulation in depression. J. Neuroinflammation 17, 1–11. doi: 10.1186/s12974-020-01732-5
Luckey, A. M., Adcock, K., and Vanneste, S. (2023). Peripheral nerve stimulation: a neuromodulation-based approach. Neurosci. Biobehav. Rev. 149:105180. doi: 10.1016/J.NEUBIOREV.2023.105180
McGough, J. J., Sturm, A., Cowen, J., Tung, K., Salgari, G. C., Leuchter, A. F., et al. (2019). Double-blind, sham-controlled, pilot study of trigeminal nerve stimulation for attention-deficit/hyperactivity disorder. J. Am. Acad. Child Adolesc. Psychiatry 58, 403–411.e3. doi: 10.1016/J.JAAC.2018.11.013
McIntire, L. K., McKinley, R. A., Goodyear, C., McIntire, J. P., and Brown, R. D. (2021). Cervical transcutaneous vagal nerve stimulation (ctVNS) improves human cognitive performance under sleep deprivation stress. Commun Biol 4, 634–639. doi: 10.1038/s42003-021-02145-7
Mercante, B., Enrico, P., Floris, G., Quartu, M., Boi, M., Serra, M. P., et al. (2017). Trigeminal nerve stimulation induces Fos immunoreactivity in selected brain regions, increases hippocampal cell proliferation and reduces seizure severity in rats. Neuroscience 361, 69–80. doi: 10.1016/j.neuroscience.2017.08.012
Mercante, B., Pilurzi, G., Ginatempo, F., Manca, A., Follesa, P., Tolu, E., et al. (2015). Trigeminal nerve stimulation modulates brainstem more than cortical excitability in healthy humans. Exp. Brain Res. 233, 3301–3311. doi: 10.1007/s00221-015-4398-2
Meyers, E., Kasliwal, N., Solorzano, B., Lai, E., Bendale, G., Berry, A., et al. (2019). Enhancing plasticity in central networks improves motor and sensory recovery after nerve damage. Nat. Commun. 10, 5782–5714. doi: 10.1038/s41467-019-13695-0
Oldfield, R. C. (1971). The assessment and analysis of handedness: the Edinburgh inventory. Neuropsychologia 9, 97–113. doi: 10.1016/0028-3932(71)90067-4
Pilurzi, G., Mercante, B., Ginatempo, F., Follesa, P., Tolu, E., and Deriu, F. (2016). Transcutaneous trigeminal nerve stimulation induces a long-term depression-like plasticity of the human blink reflex. Exp. Brain Res. 234, 453–461. doi: 10.1007/s00221-015-4477-4
Piquet, M., Balestra, C., Sava, S. L., and Schoenen, J. E. (2011). Supraorbital transcutaneous neurostimulation has sedative effects in healthy subjects. BMC Neurol. 11:135. doi: 10.1186/1471-2377-11-135
Pop, J., Murray, D., Markovic, D., and DeGiorgio, C. M. (2011). Acute and long-term safety of external trigeminal nerve stimulation for drug-resistant epilepsy. Epilepsy Behav E&B. 22, 574–576. doi: 10.1016/j.yebeh.2011.06.024
Rincon-Gonzalez, L., Buneo, C. A., and Tillery, S. I. H. (2011). The proprioceptive map of the arm is systematic and stable, but idiosyncratic. PLoS One 6:e25214. doi: 10.1371/journal.pone.0025214
Rincon-Gonzalez, L., Naufel, S., Santos, V., and Tillery, S. (2012). Interactions between tactile and proprioceptive representations in haptics. J. Mot. Behav. 44, 391–401. doi: 10.1080/00222895.2012.746281
Schwarz, L. A., and Luo, L. (2015). Organization of the Locus Coeruleus-Norepinephrine System. Curr. Biol. 25, R1051–R1056. doi: 10.1016/J.CUB.2015.09.039
Silberstein, S. D., Calhoun, A. H., Lipton, R. B., Grosberg, B. M., Cady, R. K., Dorlas, S., et al. (2016). Chronic migraine headache prevention with noninvasive vagus nerve stimulation: the EVENT study. Neurology 87, 529–538. doi: 10.1212/WNL.0000000000002918
Tanner, J., Orthlieb, G., Shumate, D., and Helms, T. S. (2021). Effect of tactile sensory substitution on the proprioceptive error map of the arm. Front. Neurosci. 15:15. doi: 10.3389/fnins.2021.586740
Trevizol, A. P., and Cordeiro, Q. (2017). Trigeminal nerve stimulation for major depressive disorder: an updated systematic review. Arch. Neurosci. 4:39263. doi: 10.5812/ARCHNEUROSCI.39263
Trevizol, A. P., Sato, I. A., Cook, I. A., Shiozawa, P., Lowenthal, R., and Cordeiro, Q. (2016). Trigeminal nerve stimulation (TNS) for posttraumatic stress disorder and major depressive disorder: an open-label proof-of-concept trial. Epilepsy Behav. 60, 240–241. doi: 10.1016/j.yebeh.2016.04.014
van Boekholdt, L., Kerstens, S., Khatoun, A., Asamoah, B., and Mc, L. M. (2020). tDCS peripheral nerve stimulation: a neglected mode of action? Mol Psychiatry 2020 262 26, 456–461. doi: 10.1038/s41380-020-00962-6
Vecchio, E., Bassez, I., Ricci, K., Tassorelli, C., Liebler, E., and de Tommaso, M. (2018). Effect of non-invasive vagus nerve stimulation on resting-state electroencephalography and laser-evoked potentials in migraine patients: mechanistic insights. Front. Hum. Neurosci. 12:398984. doi: 10.3389/FNHUM.2018.00366/BIBTEX
Verkuil, B., and Burger, A. M. (2019). Transcutaneous vagus nerve stimulation does not affect attention to fearful faces in high worriers. Behav. Res. Ther. 113, 25–31. doi: 10.1016/J.BRAT.2018.12.009
Xiao, X., Hou, X., Zhang, Z., Li, Y., Yu, X., Wang, Y., et al. (2020). Efficacy and brain mechanism of transcutaneous auricular vagus nerve stimulation for adolescents with mild to moderate depression: study protocol for a randomized controlled trial. Pediatr. Investig. 4, 109–117. doi: 10.1002/PED4.12198
Xue, T., Chen, S., Bai, Y., Han, C., Yang, A., and Zhang, J. (2022). Neuromodulation in drug-resistant epilepsy: a review of current knowledge. Acta Neurol. Scand. 146, 786–797. doi: 10.1111/ane.13696
Yakunina, N., Kim, S. S., and Nam, E. C. (2017). Optimization of transcutaneous Vagus nerve stimulation using functional MRI. Neuromodulation Technol. Neural Interface 20, 290–300. doi: 10.1111/ner.12541
Zehetleitner, M., Ratko-Dehnert, E., and Müller, H. J. (2015). Modeling violations of the race model inequality in bimodal paradigms: co-activation from decision and non-decision components. Front. Hum. Neurosci. 9:93369. doi: 10.3389/FNHUM.2015.00119/BIBTEX
Keywords: proprioception, trigeminal nerve stimulation, cranial nerve stimulation, tactile-proprioceptive integration, neuromodulation
Citation: Tanner J, Orthlieb G and Helms Tillery S (2024) Effect of touch on proprioception: non-invasive trigeminal nerve stimulation suggests general arousal rather than tactile-proprioceptive integration. Front. Hum. Neurosci. 18:1429843. doi: 10.3389/fnhum.2024.1429843
Edited by:
Carlotta Fossataro, University of Turin, ItalyReviewed by:
Diego Manzoni, University of Pisa, ItalyMichael C. Wiest, Wellesley College, United States
Copyright © 2024 Tanner, Orthlieb and Helms Tillery. This is an open-access article distributed under the terms of the Creative Commons Attribution License (CC BY). The use, distribution or reproduction in other forums is permitted, provided the original author(s) and the copyright owner(s) are credited and that the original publication in this journal is cited, in accordance with accepted academic practice. No use, distribution or reproduction is permitted which does not comply with these terms.
*Correspondence: Justin Tanner, amN0YW5uZXJAYXN1LmVkdQ==