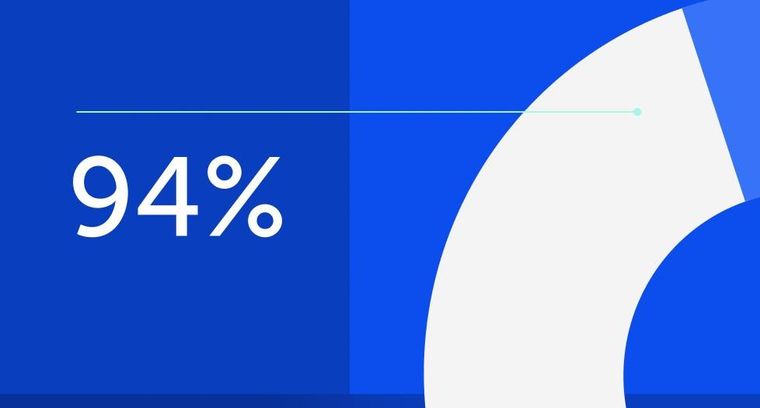
94% of researchers rate our articles as excellent or good
Learn more about the work of our research integrity team to safeguard the quality of each article we publish.
Find out more
ORIGINAL RESEARCH article
Front. Hum. Neurosci., 02 October 2023
Sec. Brain Imaging and Stimulation
Volume 17 - 2023 | https://doi.org/10.3389/fnhum.2023.1224611
This article is part of the Research TopicDeep Brain Stimulation Think Tank: Updates in Neurotechnology and Neuromodulation, Volume IVView all 17 articles
Background: Antiparkinson medication and subthalamic nucleus deep brain stimulation (STN-DBS), two common treatments of Parkinson’s disease (PD), effectively improve skeletomotor movements. However, evidence suggests that these treatments may have differential effects on eye and limb movements, although both movement types are controlled through the parallel basal ganglia loops.
Objective: Using a task that requires both eye and upper limb movements, we aimed to determine the effects of medication and STN-DBS on eye and upper limb movement performance.
Methods: Participants performed a visually-guided reaching task. We collected eye and upper limb movement data from participants with PD who were tested both OFF and ON medication (n = 34) or both OFF and ON bilateral STN-DBS while OFF medication (n = 11). We also collected data from older adult healthy controls (n = 14).
Results: We found that medication increased saccade latency, while having no effect on reach reaction time (RT). Medication significantly decreased saccade peak velocity, while increasing reach peak velocity. We also found that bilateral STN-DBS significantly decreased saccade latency while having no effect on reach RT, and increased saccade and reach peak velocity. Finally, we found that there was a positive relationship between saccade latency and reach RT, which was unaffected by either treatment.
Conclusion: These findings show that medication worsens saccade performance and benefits reaching performance, while STN-DBS benefits both saccade and reaching performance. We explore what the differential beneficial and detrimental effects on eye and limb movements suggest about the potential physiological changes occurring due to treatment.
Two common treatments for Parkinson’s disease (PD) are antiparkinson medication and subthalamic nucleus deep brain stimulation (STN-DBS). While both treatments effectively improve the motor signs of PD, the mechanisms by which treatment improves behavior may be different. An indirect way to assess these mechanisms is to determine the treatment effects on different effectors, such as the eyes and upper limbs. This will help elucidate how treatments are affecting multiple neural circuits. However, treatment effects on eye and upper limb performance have typically been assessed in separate experiments with different tasks. We aimed to assess and better understand how the different treatments of PD affect both eye and upper limb movements during the same movement task using comparable outcomes. We focused on two aspects of eye and upper limb movement: latency/reaction time (RT) and peak velocity.
The results from separate eye only and upper limb only movement tasks suggest that antiparkinson medication may have differential effects on the eyes and upper limbs. We have recently shown that medication increased saccade latency (Munoz et al., 2022), confirming the findings of previous studies (Müller et al., 1994; Michell et al., 2006; Hood et al., 2007; Dec-Ćwiek et al., 2017; Lu et al., 2019; Waldthaler et al., 2019). However, other studies have reported that medication does not have a significant effect on saccade latency (Gibson et al., 1987; Rascol et al., 1989; Temel et al., 2009; van Stockum et al., 2012; Cubizolle et al., 2014; Bakhtiari et al., 2020), which could be due to suboptimal medication doses (Munoz et al., 2022). Conversely, the medication effect on simple RT tasks evaluating upper limb movement has typically lacked statistical significance, however, there has been a consistent pattern of medication decreasing simple RT within all (Velasco and Velasco, 1973; Bloxham et al., 1987; Pullman et al., 1988, 1990; Starkstein et al., 1989; Jahanshahi et al., 1992; Fern-Pollak et al., 2004; Ingram et al., 2021) but one study (Müller and Harati, 2020). Additionally, one study found this decrease in latency with medication to be statistically significant (Montgomery and Nuessen, 1990). Overall, these findings suggest that medication significantly increases saccade latency and decreases upper limb simple RT, but not significantly.
During the visually-guided saccade task, medication typically decreased saccade peak velocity, either significantly (Munoz et al., 2022) or not significantly (Dec-Ćwiek et al., 2017; Lu et al., 2019). Conversely, studies reported that medication typically increased the peak velocity of simple upper limb movements during reach-to-grasp tasks (Castiello et al., 2000; Negrotti et al., 2005; Schettino et al., 2006), arm abduction to match a target (Baroni et al., 1984), wrist flexion (Berardelli et al., 1986; Johnson et al., 1994), elbow flexion (Robichaud et al., 2002; Vaillancourt et al., 2004), and reach- or point-to-target tasks (Kelly et al., 2002; Camarda et al., 2005). Similarly, medication has improved finger tapping speed and pronation-supination speed (Brzezicki et al., 2023). Taken together, previous literature suggests that medication will decrease saccade peak velocity but increase upper limb peak velocity. However, the effects of medication on both eye and limb movements have not been tested using a single task and cohort of participants with PD.
Unlike studies examining the effects of medication, the previous literature evaluating the effects of STN-DBS suggest that bilateral STN-DBS may have similar effects on eye and upper limb movements. Using the visually-guided saccade task, most studies have shown that STN-DBS decreased saccade latency compared to OFF stimulation, typically significantly (Fawcett et al., 2007, 2010; Sauleau et al., 2008; Temel et al., 2008, 2009; Yugeta et al., 2010; Antoniades et al., 2012a,b, 2015; Dec-Ćwiek et al., 2017; Goelz et al., 2017; Bakhtiari et al., 2020), but occasionally not significantly (Rivaud-Péchoux et al., 2000; Lohnes and Earhart, 2012; Pinkhardt et al., 2012; Nilsson et al., 2013). Similarly to saccade latency, studies found that bilateral STN-DBS decreased upper limb RT during a simple RT task, either significantly (Brown et al., 1999; Temel et al., 2006; Antoniades et al., 2012b) or trending toward significance (Kumru et al., 2004). Additionally, one study compared the effect of STN-DBS on visually-guided saccade latency and button press RT. They found that STN-DBS decreased both latency and RT (Antoniades et al., 2012b), but other aspects of movement, such as peak velocity, were not evaluated. Overall, these findings suggest that bilateral STN-DBS will decrease both saccade latency and reach RT.
Previous studies using the visually-guided saccade task reported that bilateral STN-DBS increased saccade peak velocity, either significantly (Nilsson et al., 2013) or not significantly (Pinkhardt et al., 2012; Dec-Ćwiek et al., 2017). Similarly, STN-DBS has repeatedly been found to increase peak velocity of upper limb movements, such as reach-to-grasp (Dafotakis et al., 2008), finger tapping (Dafotakis et al., 2008; Tamás et al., 2016), repetitive pointing (Dafotakis et al., 2008), hand grasping (Tamás et al., 2016), elbow flexion (Vaillancourt et al., 2004), pronation/supination (Tamás et al., 2016), and cued upper limb joint movements (Joundi et al., 2012). This would be expected as STN-DBS is a common treatment of PD because it has been proven to improve motor function (Limousin et al., 1995; Pollak et al., 1996; Krack et al., 1998; Brown et al., 1999; Thobois et al., 2002; Rodriguez-Oroz et al., 2005). Taken together, previous literature suggests that STN-DBS will increase saccade and upper limb peak velocity. However, the effects of STN-DBS on eye and upper limb movements have mostly been evaluated separately, limiting direct comparisons.
This study investigated the effects of antiparkinson medication and bilateral STN-DBS on the oculomotor and skeletomotor systems during a task requiring both eye and upper limb movement. First, we determined the effect of medication on saccade and reach latency/RT and peak velocity. We hypothesized that medication would increase saccade latency while having no effect on or decreasing reach RT and would decrease saccade peak velocity while increasing reach peak velocity. Second, we determined the effect of bilateral STN-DBS on saccade and reach latency/RT and peak velocity. We hypothesized that STN-DBS would decrease both saccade latency and reach RT and would increase both saccade and reach peak velocity. Third, we determined the relationship between saccade latency and reach RT for a cohort of people with PD tested OFF and ON medication, a cohort of people with PD tested OFF and ON STN-DBS, and healthy controls to evaluate whether treatment has an impact on this relationship.
Northwestern University and Rush University Medical Center Institutional Review Boards approved this study, and all experiments were completed in accord with the Helsinki Declaration of 1975. We obtained informed consent from all participants. Participants with PD were recruited from the movement disorder clinics at both institutions. Participants with PD were examined by a movement disorders neurologist and met the United Kingdom PD Society brain bank clinical diagnostic criteria (Hughes et al., 1992a,b; Berardelli et al., 2013) but had no neurological comorbidities, while healthy controls had no reported history of any neurological disorders. All participants had (1) normal or corrected-to-normal visual acuity, (2) no eye movement abnormalities, such as blepharospasm, double vision, and/or eyelid opening apraxia, and (3) the ability to understand and perform the experimental task during intake. All participants were right-hand dominant, as confirmed by the Edinburgh Handedness Inventory (Oldfield, 1971).
To examine the medication effect, 34 individuals with PD (28 males, 6 females) who were treated with antiparkinson medication completed testing of the visually-guided reaching task (Table 1). To examine the STN-DBS effect, we recruited 14 individuals with PD who had high-frequency bilateral STN-DBS. Three participants were unable to complete testing OFF STN-DBS. Therefore, the STN-DBS effect analysis included 11 individuals with PD (11 males) who were treated with bilateral STN-DBS (Table 1). Individuals were tested 8 months post-surgery on average (range: 6–12 months). Finally, we also tested 17 older adult healthy controls, but 3 were excluded due to a high Movement Disorder Society-Unified Parkinson’s Disease Rating Scale (MDS-UPDRS) Part III motor score (>12), a low Montreal Cognitive Assessment (MoCA) score (<18), or fatigue preventing the participant from successfully completing the task. The final healthy control group included 14 participants (12 males, 2 females) (Table 1).
Table 1. Characteristics of participants with Parkinson’s disease in the medication and STN-DBS effect analyses and healthy controls.
To determine the medication effect, data collection took place over 3 days: 1 day for intake and 2 days for testing. During intake, participants with PD were consented, were administered the MoCA, and practiced the experimental tasks while ON medication. Testing occurred over the next 2 days: 1 day OFF medication and 1 day ON medication, with the order of medication condition randomized across participants. For OFF medication testing, participants withdrew from all antiparkinson medications for at least 12-h before the start of testing (Langston et al., 1992). For ON medication testing, participants took their medications as usual. To verify that participants were in the “off state” or “on state,” the experimenter confirmed with the participant that they felt “off” or “on” before testing began. MDS-UPDRS Part III was administered right before testing each day.
To determine the bilateral STN-DBS effect, data collection took place over 5 days: 1 day for intake and 4 days for testing. Intake was completed ON medication and ON bilateral STN-DBS and otherwise was identical to the intake day of the medication effect participants. Testing occurred over the next 4 days: 1 day OFF STN-DBS, 1 day with only the left stimulator on, 1 day with only the right stimulator on, and 1 day ON bilateral stimulation with the order of STN-DBS condition randomized across subjects. Only data from the OFF STN-DBS and ON bilateral STN-DBS conditions are presented in this manuscript. Stimulators were turned OFF at least 3-h prior to testing (Temperli et al., 2003). ON bilateral stimulation testing was completed on clinical stimulation settings (Table 2). All testing was completed OFF medication after at least 12-h overnight withdrawal (Langston et al., 1992). MDS-UPDRS Part III was administered right before testing each day.
For healthy controls, intake and testing were completed in 1 day. Testing for all participants included a series of 6 different eye and upper limb movement tasks, but only data from one, the visually-guided reaching task, is reported in this manuscript.
Participants sat upright in a height-adjustable chair with their chin placed on a chin rest to minimize head movement. We recorded binocular eye movements at 500 Hz using an infrared camera-based eye-tracking system (Eyelink II, SR Research Ltd, Ottawa, ON, Canada). We recorded head, upper limb, and robotic arm movements at 240 Hz using a three-dimensional motion capture system (Optotrak 3020, Northern Digital, Waterloo, ON, Canada). To capture head and upper limb movements, participants had infrared light-emitting diodes (iLED) attached to the eye tracking system on the head and another iLED attached to their right index finger adjacent to their fingernail. To capture the robotic arm movements, another iLED was attached to the end of the robotic arm. Eye, head, upper limb, and robotic arm movements were synchronized, after down-sampling the eye-tracking data to 240 Hz, and stored using The MotionMonitor (Innovative Sports Training, Chicago, IL, USA).
The task was presented using 3 mm green LEDs (70 mcd), the first as the central fixation LED mounted on a central fixation stand and the second as the peripheral target LED, which was attached to the tip of a robotic arm (Thermo CRS, Burlington, ON, Canada). The central fixation LED was positioned at eye level, 42 cm away from the chin rest and participant. The task was completed in the dark.
Each trial began with the participant fixating their eyes on the central LED and their right index finger resting on the central fixation stand for a time interval between 2000 and 3000 ms, after which the central LED was extinguished. After a 200 ms gap, a peripheral target LED was presented to the right along the horizontal plane. The participant was instructed to “look and touch the target LED as accurately as possible at a comfortable speed.” The target LED was presented for 2000 ms at a 10° or 15° visual angle (7.41 or 11.25 cm) from the central LED at random, but only trials with the 15° target were analyzed in this manuscript for simplicity. We chose a 15° visual angle due to equipment limitations for accurate eye-tracking and we used the 10° visual angle to introduce a choice to prevent memorization of the target location. We chose to analyze only the 15° target trials because these trials were more difficult than the 10° target trials, making them likely more sensitive to differences in behavior. Participants performed one block of 20 trials, 10 trials for each target LED location, to prevent fatigue while having enough trials to reach statistical significance according to pilot testing. Participants were given a short break after 10 trials and the lights were turned on to limit adaptation to the dark. Before the block of 20 trials, all participants completed practice trials until they could confidently perform the task correctly.
The eye and upper limb data were processed using a custom MATLAB script (The MathWorks Inc., Natick, MA, USA). Eye and upper limb position data were filtered using a 20 Hz low-pass second-order, zero-phase Butterworth filter. The filtered position data were differentiated to calculate velocity. Tangential velocity was calculated in 2 dimensions for the eye movement data and in 3 dimensions for upper limb movement data.
Using the eye and upper limb position data and the tangential velocity data, an approximate estimate of the eye and upper limb movement onset and offset was marked using visual inspection to create a region of interest in time. We computed eye and upper limb peak velocities within this region of time. From the peak velocities, we identified the first time point when velocity went below 5% of the peak velocity, which was defined as the eye or upper limb movement onset. Saccade latency and reach RT were defined as the time difference between target LED onset and the algorithmically identified movement onset of the saccade or reach. During visual inspection, if it was clear the trial was not performed correctly, it was marked invalid. For instance, if the participant moved their eyes or upper limb prior to the presentation of the target LED or if they did not complete the reach in the allotted time. All trials marked invalid were excluded.
After visual inspection, trials were further excluded based on predetermined criteria: (1) saccade latency was < 90 ms (Munoz et al., 1998) or >1000 ms, (2) reach RT was < 200 ms or >1000 ms, or (3) reach RT occurred >500 ms before saccade latency. Based on visual inspection of the data, trials were excluded as outliers if reach peak velocity was >1 m/s or reach end point error was >5 cm. Our outcome variables were saccade latency, reach RT, saccade peak velocity, and reach peak velocity.
Linear mixed-effect regression models were used to assess each of the saccade and reach outcomes. For the medication analysis, the fixed effect was medication condition (OFF and ON medication). For the STN-DBS analysis, the fixed effect was STN-DBS condition (OFF and ON bilateral STN-DBS). The random effect was participant in both analyses. To meet the distributional assumptions for mixed modeling, if the observed data was right skewed, the data was transformed using a log function. This occurred for saccade latency, reach RT, and reach peak velocity. If the observed data was left skewed, the data was transformed using a squared function. This was the case for saccade peak velocity. The statistics presented are in log or squared scales, along with the estimated difference transformed back to the original scale (Est diffBT). The relationship between saccade latency and reach RT was assessed across medication conditions, across STN-DBS conditions, and in the healthy controls using mixed-effect regression models. Mixed models were also used to assess the interaction between the treatment and saccade latency effects on reach RT. The significance of the results was identical between the original scale and the log transformation scale, so we present the data and statistics in the original scale for ease of interpretation. All statistical analyses were performed using SAS® (version 9.4, SAS Institute, Cary, NC, USA).
Medication significantly increased saccade latency [Est diffBT = 25.98 ms; F(1,542) = 17.50; p < 0.001; Figure 1A] but had no statistically significant effect on reach RT [Est diffBT = 13.96 ms; F(1,542) = 2.96; p = 0.086; Figure 1B] compared to OFF medication. Additionally, medication significantly decreased saccade peak velocity [Est diffBT = −0.04 m/s; F(1,542) = 4.75; p = 0.030; Figure 1C] but significantly increased reach peak velocity [Est diffBT = 0.03 m/s; F(1,542) = 23.00; p < 0.001; Figure 1D] compared to OFF medication. Observationally, with medication, the mean saccade latency and peak velocity became further from healthy control means, while mean reach peak velocity became closer to healthy control means.
Figure 1. The medication effect on saccades and reaching. The observed medication effect on (A) saccade latency, (B) reach RT, (C) saccade peak velocity, and (D) reach peak velocity. The plots show the mean medication effect and standard errors, with observed means from healthy controls presented for reference (dashed line). * Indicates that the medication effect was statistically significant (p < 0.05).
Bilateral STN-DBS significantly decreased saccade latency [Est diffBT = −38.89 ms; F(1,159) = 7.75; p = 0.006; Figure 2A] but had no statistically significant effect on reach RT [Est diffBT = −29.93 ms; F(1,159) = 2.91; p = 0.090; Figure 2B] compared to OFF STN-DBS. Additionally, bilateral STN-DBS significantly increased saccade peak velocity [Est diffBT = 0.09 m/s; F(1,159) = 4.20; p = 0.042; Figure 2C] and significantly increased reach peak velocity [Est diffBT = 0.03 m/s; F(1,159) = 6.48; p = 0.012; Figure 2D] compared to OFF STN-DBS. Observationally, all three statistically significant findings resulted in performance changes with STN-DBS that became closer to healthy control performance.
Figure 2. The bilateral STN-DBS effect on saccades and reaching. The observed STN-DBS effect on (A) saccade latency, (B) reach RT, (C) saccade peak velocity, and (D) reach peak velocity. The plots show the mean STN-DBS effect and standard errors, with observed means from healthy controls presented for reference (dashed line). Participants were OFF medication during OFF and ON bilateral STN-DBS testing. * Indicates that the bilateral STN-DBS effect was statistically significant (p < 0.05).
Since the direction of change in mean reach RT followed the change in mean saccade latency for both treatments, we wanted to determine the relationship between saccade latency and reach RT. Saccade latency had a significant positive relationship with reach RT across the OFF and ON data of the participants in the medication analysis [ß = 0.452; estimated intraclass correlation coefficient (ICC) = 30.58%; p < 0.001; Figure 3A] and of the participants in the STN-DBS analysis [ß = 0.389; ICC = 43.26%; p < 0.001; Figure 3B]. To determine if this relationship was affected by treatment, we also determined whether there was an interaction between the treatment and saccade latency effects on reach RT. There was no interaction between either medication condition and saccade latency (p = 0.171) or STN-DBS condition and saccade latency (p = 0.211). Finally, the positive relationship between saccade latency and reach RT was also seen in the healthy controls (ß = 0.484; ICC = 65.74%; p < 0.001; Figure 3C). In the vast majority of trials, saccade initiation occurred before reach initiation for the participants in the medication analysis (Figure 3D), participants in the STN-DBS analysis (Figure 3E), and healthy controls (Figure 3F).
Figure 3. Relationship between saccade latency and reach reaction time. The relationship was significant for the data analyzed for the (A) medication group, (B) STN-DBS group, and (C) healthy controls. The plots depict the model-estimated relationship (solid black line) and 95% confidence interval (gray shaded area). The observed trial-level data are overlaid onto the plots (gray filled circles). The histograms show the difference in time between the reach RT and saccade latency for the (D) medication group, (E) STN-DBS group, and (F) healthy controls. All values over zero show that saccade initiation occurs before reach initiation.
We investigated the effects of antiparkinson medication and bilateral STN-DBS on visually-guided saccades and reaching movements during a visually-guided reaching task. Notably, this is the first report of both the medication and STN-DBS effects on the eye and upper limb movements during the same task. We have three key findings. First, antiparkinson medication had an adverse effect on saccade performance and a beneficial effect on one aspect of reach performance. Antiparkinson medication increased saccade latency, decreased saccade peak velocity, and increased reach peak velocity. Second, STN-DBS had a beneficial effect on saccade performance and a beneficial effect on one aspect of reach performance. Bilateral STN-DBS decreased saccade latency, increased saccade peak velocity, and increased reach peak velocity. Third, we confirmed that there was a positive relationship between saccade latency and reach RT across treatment groups and healthy controls, in which saccade initiation preceded limb initiation. Importantly, the positive relationship remained unaffected by treatment. Finally, we discuss the possible parallel mechanisms underlying the similar effects of medication and STN-DBS on reach peak velocity and the possible unique mechanisms underlying the differential effects of medication and STN-DBS on saccade performance.
We found that antiparkinson medication adversely impacted saccade performance and benefitted one aspect of reach performance (Figure 1). Our findings confirmed our prediction that antiparkinson medication would increase saccade latency. Our recent study and other previous studies have shown that medication increases saccade latency during a saccade only task (Müller et al., 1994; Michell et al., 2006; Hood et al., 2007; Dec-Ćwiek et al., 2017; Lu et al., 2019; Waldthaler et al., 2019; Munoz et al., 2022). The effect of medication on saccade peak velocity has been less established. Three previous studies have found no statistically significant effect on saccade peak velocity (Rascol et al., 1989; Dec-Ćwiek et al., 2017; Lu et al., 2019), but, observationally, 2 of the 3 showed a slight decrease in peak velocity with medication during the visually-guided saccade task (Dec-Ćwiek et al., 2017; Lu et al., 2019). The third study did not show a slight decrease, possibly because of the small medication dosage given to the participants (Rascol et al., 1989). Our recent study found a statistically significant decrease in peak velocity with medication on the visually-guided saccade task (Munoz et al., 2022). In the present study, we extended previous saccade findings. Medication increases saccade latency and decreases saccade peak velocity not only during a saccade task, but also in a task requiring eye-hand coordination.
Additionally, our findings confirmed our hypothesis that antiparkinson medication would significantly increase reach peak velocity, while increasing reach peak velocity. The beneficial medication effects on upper limb velocity are well known, and we replicate this beneficial effect (Baroni et al., 1984; Berardelli et al., 1986; Johnson et al., 1994; Castiello et al., 2000; Kelly et al., 2002; Camarda et al., 2005; Negrotti et al., 2005; Schettino et al., 2006). This finding, in addition to a clear increase in MDS-UPDRS Part III score, made it clear that a 12-h withdrawal period was sufficient for our participants to be in an “off state,” even those on longer-acting medications, like dopamine agonists. Together, these findings suggest that medication has a differential effect on the oculomotor and skeletomotor systems.
We found that bilateral STN-DBS improved both saccade performance and one aspect of reach performance (Figure 2). Our findings confirmed our hypotheses that bilateral STN-DBS would significantly decrease saccade latency and increase saccade peak velocity compared to OFF stimulation. Previous studies have also reported that bilateral STN-DBS decreased saccade latency (Fawcett et al., 2007, 2010; Sauleau et al., 2008; Temel et al., 2008, 2009; Yugeta et al., 2010; Antoniades et al., 2012b,a, 2015; Dec-Ćwiek et al., 2017; Goelz et al., 2017; Bakhtiari et al., 2020) and increased saccade peak velocity (Nilsson et al., 2013) in a saccade only task. In addition, bilateral STN-DBS has improved saccadic eye movements during a more complex visual searching task (Tokushige et al., 2018). In the current study, we extend these findings and demonstrate that STN-DBS also has beneficial effects on saccades during a task requiring eye-hand coordination.
Additionally, we confirmed our prediction that STN-DBS would significantly increase reach peak velocity. This is similar to previously reported improvements in upper limb peak velocity (Vaillancourt et al., 2004; Dafotakis et al., 2008; Joundi et al., 2012; Tamás et al., 2016). However, it was surprising that we did not see a larger effect of STN-DBS on reach peak velocity. This was likely because our participants were instructed to move “at a comfortable speed” instead of as fast as possible. This guidance was necessary to prevent participants from hitting the robotic arm that presented the target. Another potential explanation is that the target amplitude was relatively small, only 15° or 11.25 cm away from center, meaning the reach was small. A smaller movement limits how fast a participant can move, even with the beneficial effects of stimulation. Overall, our data suggests that bilateral STN-DBS acts similarly on the oculomotor and skeletomotor systems. The effects of STN-DBS on these systems seem to be beneficial as stimulation brings performance of participants with PD closer to healthy control levels.
We found a positive relationship between saccade latency and reach RT in the medication group, the STN-DBS group, and in our healthy controls (Figure 3). This relationship was unaffected by medication and STN-DBS. The positive relationship between saccade latency and reach RT has previously been seen in reports on healthy populations (Prablanc et al., 1979; Herman et al., 1981; Biguer et al., 1982; Gielen et al., 1984; Fischer and Rogal, 1986). Specifically, the eyes typically lead the hands (Biguer et al., 1982). In the current study, the initiation of the saccade to the target preceded the initiation of the reach in over 96% of trials for the medication group and over 94% of trials for the STN-DBS group. Therefore, the previously reported positive relationship between saccade latency and reach RT in healthy controls persists in people with PD and remains unaffected by treatment.
The fact that the current study uses a task requiring eye-hand coordination may explain why we found a non-significant increase in reach RT with medication, whereas previous studies have shown a decrease. Simple RT studies have consistently reported that medication significantly (Montgomery and Nuessen, 1990) or non-significantly decreases RT (Velasco and Velasco, 1973; Bloxham et al., 1987; Pullman et al., 1988, 1990; Starkstein et al., 1989; Jahanshahi et al., 1992; Fern-Pollak et al., 2004; Ingram et al., 2021). Even in more complex reaching tasks, in which upper limb RT was measured before a reach to one of multiple targets, medication still decreased RT either significantly (Zappia et al., 1994) or not significantly (Girotti et al., 1986). However, these previous studies did not require explicit eye-hand coordination to complete the task, whereas our task required eye-hand coordination to look and reach to a visual target. As saccade latency was prolonged by medication, it would follow that reach RT would be unable to decrease.
We found that both medication and bilateral STN-DBS improved upper limb peak velocity, as would be expected. The two common pathophysiological models of PD, the rate model and the oscillation model, can both explain this benefit to upper limb peak velocity (David et al., 2020).
Without treatment, PD is characterized by slowness of movement, which is thought to be due to the loss of dopaminergic neurons in the substantia nigra pars compacta projecting to the striatum (Brooks et al., 1990). This results in decreased activity of the basal ganglia direct pathway and increased activity of the indirect pathway (Albin et al., 1989). Combined, the imbalance between the basal ganglia pathways increases the inhibitory activity of the basal ganglia output nuclei, the globus pallidus internus (GPi) and substantia nigra pars reticulata (SNr) (Albin et al., 1989). Therefore, there is excessive inhibition from the basal ganglia output nuclei to downstream targets, such as thalamus, which then results in decreased activation of cortical areas (Albin et al., 1989) and disruption of the motor network (Bologna et al., 2020). It is thought that both antiparkinson medication and STN-DBS reduce the excessive inhibition from the basal ganglia onto the thalamus and reduce the resulting decreased cortical activation, with medication restoring dopamine levels in the striatum and STN-DBS decreasing the overactivity of the indirect pathway. In support of this, positron emission tomography (PET) studies looking at the effects of medication (Jenkins et al., 1992; Rascol et al., 1992, 1994) and STN-DBS (Limousin et al., 1997; Ceballos-Baumann et al., 1999; Grafton et al., 2006) have found that both treatments increase metabolic activity in the motor cortices, especially the supplementary motor cortex. The increased activation of supplementary motor cortex has been correlated with improvement in akinesia with medication (Jenkins et al., 1992) and STN-DBS (Limousin et al., 1997; Ceballos-Baumann et al., 1999), which could explain our beneficial effect on peak velocity.
Additionally, without treatment, PD is also characterized by excessive beta band synchronization throughout the motor loop at rest, both between neurons and in local field potentials (Brown et al., 2001; Levy et al., 2002; Hammond et al., 2007). A proposed explanation of the motor dysfunction in PD is that the excessive tonic beta synchronization prevents the phasic beta suppression that is needed to execute a planned movement (Brittain and Brown, 2014). Medication has been reported to suppress this excessive beta band synchronization at rest (Brown et al., 2001; Levy et al., 2002; Priori et al., 2004; Kühn et al., 2006; Ray et al., 2008), which has been associated with improvement in bradykinesia, akinesia, and rigidity (Kühn et al., 2006; Ray et al., 2008). STN-DBS has also been reported to suppress excessive beta band synchronization at rest in the STN (Eusebio et al., 2011) and throughout the motor loop (Brown et al., 2004; Silberstein et al., 2005). STN-DBS improvement in bradykinesia and rigidity correlates with beta suppression at the cortex during STN-DBS (Silberstein et al., 2005) and following the cessation of STN-DBS (Kühn et al., 2008). The similar mechanistic effects of medication and STN-DBS associated with the beneficial motor effects could also contribute to the reported increase in upper limb peak velocity.
We found that medication worsened saccade performance by increasing latency and decreasing peak velocity, while STN-DBS improved saccade performance by decreasing latency and increasing peak velocity. In previous studies showing that STN-DBS improves visually-guided saccade performance, the proposed mechanisms are similar to those thought to underlie the improvement in the skeletomotor system after STN-DBS (Sauleau et al., 2008; Temel et al., 2009; Fawcett et al., 2010; Yugeta et al., 2010; Nilsson et al., 2013; Goelz et al., 2017). The worsened visually-guided saccade performance in PD is thought to be due to excessive inhibition on the superior colliculus (Albin et al., 1989; Terao et al., 2011) from the SNr (Hikosaka et al., 2000). STN-DBS reduces the activity of the SNr (Benazzouz et al., 1995; Maltête et al., 2007), which suggests that the superior colliculus would be released from this excessive inhibition. The benefit to saccade performance could also be explained by STN-DBS reducing the excessive beta band oscillations in the basal ganglia, returning the superior colliculus to normal activity levels, and facilitating eye movement (Yugeta et al., 2010). Previous studies have argued that the oscillation model better describes the observed changes in saccade performance in PD and with STN-DBS compared to the rate model (Yugeta et al., 2010). However, as discussed previously, it has been reported that medication also reduces the beta band synchronization in the STN (Brown et al., 2001; Levy et al., 2002; Priori et al., 2004; Kühn et al., 2006; Ray et al., 2008). Therefore, other mechanisms must be contributing to this difference in saccade performance.
It is possible that medication is also acting on other brain areas to counteract the normalization of beta oscillations. As we suggested in a prior publication (Munoz et al., 2022), medication may impair visually-guided saccadic function by overdosing dopaminergic brain regions that are not dopamine depleted in PD. One potential region is the superior colliculus, which has been found to receive dopaminergic projections from the zona incerta and have D2-expressing neurons (Bolton et al., 2015). When dopamine was washed onto the D2-expressing superior colliculus neurons, neuronal activity was suppressed (Bolton et al., 2015). In a different animal model, similar superior colliculus activity suppression resulted in inhibited behavioral responses, such as decreased orientation to stimuli (Glagow and Ewert, 1997). Relatedly, a study examining posterior subthalamic area DBS (PSA DBS) found that, unlike STN-DBS, saccade amplitude and peak velocity were decreased with stimulation (Bangash et al., 2019). It was likely that the zona incerta was being stimulated with PSA DBS (Bangash et al., 2019), which could subsequently inhibit the downstream superior colliculus potentially via an excessive release of dopamine onto the superior colliculus. Taken together, these studies suggest the possibility that the dopamine overdose of the superior colliculus, via medication or stimulation of the zona incerta, could result in worsened saccade performance. Another potential overdosed region is the prefrontal cortex, resulting in increased inhibition from the prefrontal cortex onto the superior colliculus (Hood et al., 2007). Increased dopamine levels in the prefrontal cortex have been shown to result in prolonged saccade latency (Cameron et al., 2018). Additionally, increased inhibition from the frontal cortex has been suggested to inhibit reflexive saccades to allow for sufficient processing time to make a planned saccade (Terao et al., 2013) or to detrimentally increase the focusing of attention, making attention shifting more difficult (Cameron et al., 2018). Conversely, it has been suggested that bilateral STN-DBS may improve visual attention (Tokushige et al., 2018). Dopamine overdose of both the superior colliculus or the frontal cortex could result in an increase in visually-guided saccade latency and a decrease in saccade peak velocity. The overdosing of subcortical and cortical regions is not mutually exclusive and could be occurring simultaneously.
Using a visually-guided reaching task requiring eye and upper limb movements, we demonstrate that antiparkinson medication adversely impacts saccade performance, while it improves reaching performance. Additionally, we demonstrate that bilateral STN-DBS improves both saccade and reaching performance. Our findings highlight the importance of assessing multiple effectors simultaneously to evaluate how the parkinsonian brain may be affected by treatment. Crucially, the similar and differential effects of antiparkinson medication and STN-DBS on the oculomotor and skeletomotor systems suggest parallel and unique mechanisms of action of antiparkinson medication and STN-DBS. While medication and STN-DBS may impact the basal ganglia circuitry similarly, medication may also overdose other dopaminergic areas resulting in worsened saccade performance.
The raw data supporting the conclusions of this article will be made available by the authors, without undue reservation.
The studies involving humans were approved by the Northwestern University Institutional Review Board and the Rush University Medical Center Institutional Review Board. The studies were conducted in accordance with the local legislation and institutional requirements. The participants provided their written informed consent to participate in this study.
MM, LG, DC, and FD contributed to conceptualization and/or methodology of the study. MM, YR, LG, and FD contributed to project administration. GP, LV, SS, and JR provided resources needed for the study. MM, RA, YR, QD, LG, and FD assisted with data collection and/or data processing. MM performed the formal analysis and wrote the original draft of the manuscript. DC and FD supervised the study and the formal analysis. All authors reviewed, edited, and approved the submitted version of the article.
This research was completed with financial support from the National Institutes of Health (R01 NS09295001 [DC], T32 HD07418 [MM], and F31 NS12069501 [MM]).
We would like to thank all the individuals who participated in this study.
GP received grant support from National Institute of Neurological Disorders and Stroke, and consulting fees from Guidepoint, Kyowa Kirin. LV receives research support from NIH; receives research support, is on the advisory board of, and consults for Abbott, AbbVie, and Avion; receives research support from and consults for Boston Scientific; receives research support from and is on the advisory board for Biogen; and receives research support from Medtronic, Neuroderm, and Prilenia Therapeutics.
The remaining authors declare that the research was conducted in the absence of any commercial or financial relationships that could be construed as a potential conflict of interest.
All claims expressed in this article are solely those of the authors and do not necessarily represent those of their affiliated organizations, or those of the publisher, the editors and the reviewers. Any product that may be evaluated in this article, or claim that may be made by its manufacturer, is not guaranteed or endorsed by the publisher.
Albin, R. L., Young, A. B., and Penney, J. B. (1989). The functional anatomy of basal ganglia disorders. Trends Neurosci. 12, 366–375. doi: 10.1016/0166-2236(89)90074-X
Antoniades, C. A., Buttery, P., FitzGerald, J. J., Barker, R. A., Carpenter, R. H. S., and Watts, C. (2012a). Deep brain stimulation: Eye movements reveal anomalous effects of electrode placement and stimulation. PLoS One 7:e32830. doi: 10.1371/journal.pone.0032830
Antoniades, C. A., Carpenter, R. H. S., and Temel, Y. (2012b). Deep brain stimulation of the subthalamic nucleus in Parkinson’s disease: Similar improvements in saccadic and manual responses. Neuroreport 23, 179–183. doi: 10.1097/WNR.0b013e32834f6daa
Antoniades, C. A., Rebelo, P., Kennard, C., Aziz, T. Z., Green, A. L., and FitzGerald, J. J. (2015). Pallidal deep brain stimulation improves higher control of the oculomotor system in Parkinson’s disease. J. Neurosci. 35, 13043–13052. doi: 10.1523/JNEUROSCI.2317-15.2015
Bakhtiari, S., Altinkaya, A., Pack, C. C., and Sadikot, A. F. (2020). The role of the subthalamic nucleus in inhibitory control of oculomotor behavior in Parkinson’s disease. Sci. Rep. 10:5429. doi: 10.1038/s41598-020-61572-4
Bangash, O. K., Dissanayake, A. S., Knight, S., Murray, J., Thorburn, M., Thani, N., et al. (2019). Modulation of saccades in humans by electrical stimulation of the posterior subthalamic area. J. Neurosurg. 132, 1218–1226. doi: 10.3171/2018.12.JNS18502
Baroni, A., Benvenuti, F., Fantini, L., Pantaleo, T., and Urbani, F. (1984). Human ballistic arm abduction movements: Effects of L-dopa treatment in Parkinson’s disease. Neurology 34, 868–876. doi: 10.1212/wnl.34.7.868
Benazzouz, A., Piallat, B., Pollak, P., and Benabid, A. L. (1995). Responses of substantia nigra pars reticulata and globus pallidus complex to high frequency stimulation of the subthalamic nucleus in rats: Electrophysiological data. Neurosci. Lett. 189, 77–80. doi: 10.1016/0304-3940(95)11455-6
Berardelli, A., Dick, J. P., Rothwell, J. C., Day, B. L., and Marsden, C. D. (1986). Scaling of the size of the first agonist EMG burst during rapid wrist movements in patients with Parkinson’s disease. J. Neurol. Neurosur. Psychiatry 49, 1273–1279. doi: 10.1136/jnnp.49.11.1273
Berardelli, A., Wenning, G. K., Antonini, A., Berg, D., Bloem, B. R., Bonifati, V., et al. (2013). EFNS/MDS-ES/ENS [corrected] recommendations for the diagnosis of Parkinson’s disease. Eur. J. Neurol. 20, 16–34. doi: 10.1111/ene.12022
Biguer, B., Jeannerod, M., and Prablanc, C. (1982). The coordination of eye, head, and arm movements during reaching at a single visual target. Exp. Brain Res. 46, 301–304. doi: 10.1007/BF00237188
Bloxham, C. A., Dick, D. J., and Moore, M. (1987). Reaction times and attention in Parkinson’s disease. J. Neurol. Neurosurg. Psychiatry 50, 1178–1183. doi: 10.1136/jnnp.50.9.1178
Bologna, M., Paparella, G., Fasano, A., Hallett, M., and Berardelli, A. (2020). Evolving concepts on bradykinesia. Brain 143, 727–750. doi: 10.1093/brain/awz344
Bolton, A. D., Murata, Y., Kirchner, R., Kim, S.-Y., Young, A., Dang, T., et al. (2015). A diencephalic dopamine source provides input to the superior colliculus, where D1 and D2 receptors segregate to distinct functional zones. Cell Rep. 13, 1003–1015. doi: 10.1016/j.celrep.2015.09.046
Brittain, J. S., and Brown, P. (2014). Oscillations and the basal ganglia: Motor control and beyond. Neuroimage 85, 637–647. doi: 10.1016/j.neuroimage.2013.05.084
Brooks, D. J., Ibanez, V., Sawle, G. V., Quinn, N., Lees, A. J., Mathias, C. J., et al. (1990). Differing patterns of striatal 18F-dopa uptake in Parkinson’s disease, multiple system atrophy, and progressive supranuclear palsy. Ann. Neurol. 28, 547–555. doi: 10.1002/ana.410280412
Brown, P., Mazzone, P., Oliviero, A., Altibrandi, M. G., Pilato, F., Tonali, P. A., et al. (2004). Effects of stimulation of the subthalamic area on oscillatory pallidal activity in Parkinson’s disease. Exp. Neurol. 188, 480–490. doi: 10.1016/j.expneurol.2004.05.009
Brown, P., Oliviero, A., Mazzone, P., Insola, A., Tonali, P., and Di Lazzaro, V. (2001). Dopamine dependency of oscillations between subthalamic nucleus and pallidum in Parkinson’s disease. J. Neurosci. 21, 1033–1038. doi: 10.1523/JNEUROSCI.21-03-01033.2001
Brown, R. G., Dowsey, P. L., Brown, P., Jahanshahi, M., Pollak, P., Benabid, A. L., et al. (1999). Impact of deep brain stimulation on upper limb akinesia in Parkinson’s disease. Ann. Neurol. 45, 473–488. doi: 10.1002/1531-8249(199904)45:4<473:aid-ana9<3.0.co;2-v
Brzezicki, M. A., Conway, N., Sotirakis, C., FitzGerald, J. J., and Antoniades, C. A. (2023). Antiparkinsonian medication masks motor signal progression in de novo patients. Heliyon 9:16415. doi: 10.1016/j.heliyon.2023.e16415
Camarda, R., Camarda, C., Grimaldi, S., Camarda, L. K. C., Monastero, R., and Gangitano, M. (2005). Effects of levodopa oral bolus on the kinematics of the pointing movements in Parkinson’s disease patients. J. Neurol. 252, 1074–1081. doi: 10.1007/s00415-005-0818-8
Cameron, I. G. M., Wallace, D. L., Al-Zughoul, A., Kayser, A. S., and D’Esposito, M. (2018). Effects of tolcapone and bromocriptine on cognitive stability and flexibility. Psychopharmacology 235, 1295–1305. doi: 10.1007/s00213-018-4845-4
Castiello, U., Bennett, K. M., Bonfiglioli, C., and Peppard, R. F. (2000). The reach-to-grasp movement in Parkinson’s disease before and after dopaminergic medication. Neuropsychologia 38, 46–59. doi: 10.1016/s0028-3932(99)00049-4
Ceballos-Baumann, A. O., Boecker, H., Bartenstein, P., von Falkenhayn, I., Riescher, H., Conrad, B., et al. (1999). A positron emission tomographic study of subthalamic nucleus stimulation in Parkinson disease: Enhanced movement-related activity of motor-association cortex and decreased motor cortex resting activity. Arch. Neurol. 56, 997–1003. doi: 10.1001/archneur.56.8.997
Cubizolle, S., Damon-Perrière, N., Dupouy, S., Foubert-Samier, A., and Tison, F. (2014). Parkinson’s disease, L-Dopa and “express” saccades: Superior colliculus dyskinesias? Clin. Neurophysiol. 125, 647–648. doi: 10.1016/j.clinph.2013.06.188
Dafotakis, M., Fink, G. R., Allert, N., and Nowak, D. A. (2008). The impact of subthalamic deep brain stimulation on bradykinesia of proximal and distal upper limb muscles in Parkinson’s disease. J. Neurol. 255, 429–437. doi: 10.1007/s00415-008-0701-5
David, F. J., Munoz, M. J., and Corcos, D. M. (2020). The effect of STN DBS on modulating brain oscillations: Consequences for motor and cognitive behavior. Exp. Brain Res. 238, 1659–1676. doi: 10.1007/s00221-020-05834-7
Dec-Ćwiek, M., Tutaj, M., Gracies, J.-M., Volkmann, J., Rudzińska, M., Słowik, A., et al. (2017). Opposite effects of l-dopa and DBS-STN on saccadic eye movements in advanced Parkinson’s disease. Neurol. Neurochir. Pol. 51, 354–360. doi: 10.1016/j.pjnns.2017.06.002
Eusebio, A., Thevathasan, W., Doyle Gaynor, L., Pogosyan, A., Bye, E., Foltynie, T., et al. (2011). Deep brain stimulation can suppress pathological synchronisation in parkinsonian patients. J. Neurol. Neurosurg. Psychiatry 82, 569–573. doi: 10.1136/jnnp.2010.217489
Fawcett, A. P., Cunic, D., Hamani, C., Hodaie, M., Lozano, A. M., Chen, R., et al. (2007). Saccade-related potentials recorded from human subthalamic nucleus. Clin. Neurophysiol. 118, 155–163. doi: 10.1016/j.clinph.2006.09.016
Fawcett, A. P., González, E. G., Moro, E., Steinbach, M. J., Lozano, A. M., and Hutchison, W. D. (2010). Subthalamic nucleus deep brain stimulation improves saccades in Parkinson’s disease. Neuromodulation 13, 17–25. doi: 10.1111/j.1525-1403.2009.00246.x
Fern-Pollak, L., Whone, A. L., Brooks, D. J., and Mehta, M. A. (2004). Cognitive and motor effects of dopaminergic medication withdrawal in Parkinson’s disease. Neuropsychologia 42, 1917–1926. doi: 10.1016/j.neuropsychologia.2004.05.004
Fischer, B., and Rogal, L. (1986). Eye-hand-coordination in man: A reaction time study. Biol. Cybern. 55, 253–261. doi: 10.1007/BF00355600
Gibson, J. M., Pimlott, R., and Kennard, C. (1987). Ocular motor and manual tracking in Parkinson’s disease and the effect of treatment. J. Neurol. Neurosur. Psychiatry. 50, 853–860. doi: 10.1136/jnnp.50.7.853
Gielen, C. C., van den Heuvel, P. J., and van Gisbergen, J. A. (1984). Coordination of fast eye and arm movements in a tracking task. Exp. Brain Res. 56, 154–161. doi: 10.1007/BF00237452
Girotti, F., Carella, F., Grassi, M. P., Soliveri, P., Marano, R., and Caraceni, T. (1986). Motor and cognitive performances of parkinsonian patients in the on and off phases of the disease. J. Neurol. Neurosurg. Psychiatry 49, 657–660. doi: 10.1136/jnnp.49.6.657
Glagow, M., and Ewert, J. P. (1997). Dopaminergic modulation of visual responses in toads. I. Apomorphine-induced effects on visually directed appetitive and consummatory prey-catching behavior. J. Comp. Physiol. A 180, 1–9. doi: 10.1007/s003590050021
Goelz, L. C., David, F. J., Sweeney, J. A., Vaillancourt, D. E., Poizner, H., Metman, L. V., et al. (2017). The effects of unilateral versus bilateral subthalamic nucleus deep brain stimulation on prosaccades and antisaccades in Parkinson’s disease. Exp. Brain Res. 235, 615–626. doi: 10.1007/s00221-016-4830-2
Grafton, S. T., Turner, R. S., Desmurget, M., Bakay, R., Delong, M., Vitek, J., et al. (2006). Normalizing motor-related brain activity: Subthalamic nucleus stimulation in Parkinson disease. Neurology 66, 1192–1199. doi: 10.1212/01.wnl.0000214237.58321.c3
Hammond, C., Bergman, H., and Brown, P. (2007). Pathological synchronization in Parkinson’s disease: Networks, models and treatments. Trends Neurosci. 30, 357–364. doi: 10.1016/j.tins.2007.05.004
Herman, R., Herman, R., and Maulucci, R. (1981). Visually triggered eye-arm movements in man. Exp. Brain Res. 42, 392–398. doi: 10.1007/BF00237504
Hikosaka, O., Takikawa, Y., and Kawagoe, R. (2000). Role of the basal ganglia in the control of purposive saccadic eye movements. Physiol. Rev. 80, 953–978. doi: 10.1152/physrev.2000.80.3.953
Hood, A. J., Amador, S. C., Cain, A. E., Briand, K. A., Al-Refai, A. H., Schiess, M. C., et al. (2007). Levodopa slows prosaccades and improves antisaccades: An eye movement study in Parkinson’s disease. J. Neurol. Neurosur. Psychiatry 78, 565–570. doi: 10.1136/jnnp.2006.099754
Hughes, A. J., Ben-Shlomo, Y., Daniel, S. E., and Lees, A. J. (1992a). What features improve the accuracy of clinical diagnosis in Parkinson’s disease: A clinicopathologic study. Neurology 42, 1142–1146. doi: 10.1212/wnl.42.6.1142
Hughes, A. J., Daniel, S. E., Kilford, L., and Lees, A. J. (1992b). Accuracy of clinical diagnosis of idiopathic Parkinson’s disease: A clinico-pathological study of 100 cases. J. Neurol. Neurosur. Psychiatry 55, 181–184. doi: 10.1136/jnnp.55.3.181
Ingram, L. A., Carroll, V. K., Butler, A. A., Brodie, M. A., Gandevia, S. C., and Lord, S. R. (2021). Quantifying upper limb motor impairment in people with Parkinson’s disease: A physiological profiling approach. PeerJ 9:e10735. doi: 10.7717/peerj.10735
Jahanshahi, M., Brown, R. G., and Marsden, C. D. (1992). The effect of withdrawal of dopaminergic medication on simple and choice reaction time and the use of advance information in Parkinson’s disease. J. Neurol. Neurosurg. Psychiatry 55, 1168–1176. doi: 10.1136/jnnp.55.12.1168
Jenkins, I. H., Fernandez, W., Playford, E. D., Lees, A. J., Frackowiak, R. S., Passingham, R. E., et al. (1992). Impaired activation of the supplementary motor area in Parkinson’s disease is reversed when akinesia is treated with apomorphine. Ann. Neurol. 32, 749–757. doi: 10.1002/ana.410320608
Johnson, M. T. V., Mendez, A., Kipnis, A. N., Silverstein, P., Zwiebel, F., and Ebner, T. J. (1994). Acute effects of levodopa on wrist movement in Parkinson’s disease: Kinematics, volitional EMG modulation and reflex amplitude modulation. Brain 117, 1409–1422. doi: 10.1093/brain/117.6.1409
Joundi, R. A., Brittain, J. S., Punt, T. D., Green, A. L., Jenkinson, N., and Aziz, T. Z. (2012). Stimulation of the subthalamic nucleus improves velocity of ballistic movements in Parkinson’s disease. Neuroreport 23, 390–394. doi: 10.1097/WNR.0b013e3283525b39
Kelly, V. E., Hyngstrom, A. S., Rundle, M. M., and Bastian, A. J. (2002). Interaction of levodopa and cues on voluntary reaching in Parkinson’s disease. Mov. Disord. 17, 38–44. doi: 10.1002/mds.10000
Krack, P., Pollak, P., Limousin, P., Hoffmann, D., Xie, J., Benazzouz, A., et al. (1998). Subthalamic nucleus or internal pallidal stimulation in young onset Parkinson’s disease. Brain 121, 451–457. doi: 10.1093/brain/121.3.451
Kühn, A. A., Kempf, F., Brücke, C., Doyle, L. G., Martinez-Torres, I., Pogosyan, A., et al. (2008). High-frequency stimulation of the subthalamic nucleus suppresses oscillatory β activity in patients with Parkinson’s disease in parallel with improvement in motor performance. J. Neurosci. 28, 6165–6173. doi: 10.1523/JNEUROSCI.0282-08.2008
Kühn, A. A., Kupsch, A., Schneider, G.-H., and Brown, P. (2006). Reduction in subthalamic 8-35 Hz oscillatory activity correlates with clinical improvement in Parkinson’s disease. Eur. J. Neurosci. 23, 1956–1960. doi: 10.1111/j.1460-9568.2006.04717.x
Kumru, H., Summerfield, C., Valldeoriola, F., and Valls-Solé, J. (2004). Effects of subthalamic nucleus stimulation on characteristics of EMG activity underlying reaction time in Parkinson’s disease. Mov. Disord. 19, 94–100. doi: 10.1002/mds.10638
Langston, J. W., Widner, H., Goetz, C. G., Brooks, D., Fahn, S., Freeman, T., et al. (1992). Core assessment program for intracerebral transplantations (CAPIT). Mov. Disord. 7, 2–13. doi: 10.1002/mds.870070103
Levy, R., Ashby, P., Hutchison, W. D., Lang, A. E., Lozano, A. M., and Dostrovsky, J. O. (2002). Dependence of subthalamic nucleus oscillations on movement and dopamine in Parkinson’s disease. Brain 125, 1196–1209. doi: 10.1093/brain/awf128
Limousin, P., Greene, J., Pollak, P., Rothwell, J., Benabid, A. L., and Frackowiak, R. (1997). Changes in cerebral activity pattern due to subthalamic nucleus or internal pallidum stimulation in Parkinson’s disease. Ann. Neurol. 42, 283–291. doi: 10.1002/ana.410420303
Limousin, P., Pollak, P., Benazzouz, A., Hoffmann, D., Broussolle, E., Perret, J. E., et al. (1995). Bilateral subthalamic nucleus stimulation for severe Parkinson’s disease. Mov. Disord. 10, 672–674. doi: 10.1002/mds.870100523
Lohnes, C. A., and Earhart, G. M. (2012). Effect of subthalamic deep brain stimulation on turning kinematics and related saccadic eye movements in Parkinson disease. Exp. Neurol. 236, 389–394. doi: 10.1016/j.expneurol.2012.05.001
Lu, Z., Buchanan, T., Kennard, C., FitzGerald, J. J., and Antoniades, C. A. (2019). The effect of levodopa on saccades - Oxford Quantification in Parkinsonism study. Parkinsonism Relat. Disord. 68, 49–56. doi: 10.1016/j.parkreldis.2019.09.029
Maltête, D., Jodoin, N., Karachi, C., Houeto, J. L., Navarro, S., Cornu, P., et al. (2007). Subthalamic stimulation and neuronal activity in the substantia nigra in Parkinson’s disease. J. Neurophysiol. 97, 4017–4022. doi: 10.1152/jn.01104.2006
Michell, A. W., Xu, Z., Fritz, D., Lewis, S. J. G., Foltynie, T., Williams-Gray, C. H., et al. (2006). Saccadic latency distributions in Parkinson’s disease and the effects of l-dopa. Exp. Brain Res. 174, 7–18. doi: 10.1007/s00221-006-0412-z
Montgomery, E. B., and Nuessen, J. (1990). The movement speed/accuracy operator in Parkinson’s disease. Neurology 40, 269–269. doi: 10.1212/WNL.40.2.269
Müller, C., Wenger, S., Fertl, L., and Auff, E. (1994). Initiation of visual-guided random saccades and remembered saccades in parkinsonian patients with severe motor-fluctuations. J. Neural Transm. Gen. Sect. 7, 101–108. doi: 10.1007/BF02260964
Müller, T., and Harati, A. (2020). Different response to instrumental tests in relation to cognitive demand after dopaminergic stimulation in previously treated patients with Parkinson’s disease. J. Neural Transm. 127, 265–272. doi: 10.1007/s00702-020-02148-4
Munoz, D. P., Broughton, J. R., Goldring, J. E., and Armstrong, I. T. (1998). Age-related performance of human subjects on saccadic eye movement tasks. Exp. Brain Res. 121, 391–400. doi: 10.1007/s002210050473
Munoz, M. J., Reilly, J. L., Pal, G. D., Verhagen Metman, L., Rivera, Y. M., Drane, Q. H., et al. (2022). Medication adversely impacts visually-guided eye movements in Parkinson’s disease. Clin. Neurophysiol. 143, 145–153. doi: 10.1016/j.clinph.2022.07.505
Negrotti, A., Secchi, C., and Gentilucci, M. (2005). Effects of disease progression and L-dopa therapy on the control of reaching-grasping in Parkinson’s disease. Neuropsychologia 43, 450–459. doi: 10.1016/j.neuropsychologia.2004.06.009
Nilsson, M. H., Patel, M., Rehncrona, S., Magnusson, M., and Fransson, P.-A. (2013). Subthalamic deep brain stimulation improves smooth pursuit and saccade performance in patients with Parkinson’s disease. J. Neuroeng. Rehabil. 10:33. doi: 10.1186/1743-0003-10-33
Oldfield, R. C. (1971). The assessment and analysis of handedness: The Edinburgh inventory. Neuropsychologia 9, 97–113. doi: 10.1016/0028-3932(71)90067-4
Pinkhardt, E. H., Jürgens, R., Lulé, D., Heimrath, J., Ludolph, A. C., Becker, W., et al. (2012). Eye movement impairments in Parkinson’s disease: Possible role of extradopaminergic mechanisms. BMC Neurol. 12:5. doi: 10.1186/1471-2377-12-5
Pollak, P., Benabid, A. L., Limousin, P., Benazzouz, A., Hoffmann, D., Le Bas, J. F., et al. (1996). Subthalamic nucleus stimulation alleviates akinesia and rigidity in parkinsonian patients. Adv. Neurol. 69, 591–594.
Prablanc, C., Echallier, J. F., Komilis, E., and Jeannerod, M. (1979). Optimal response of eye and hand motor systems in pointing at a visual target. I. Spatio-temporal characteristics of eye and hand movements and their relationships when varying the amount of visual information. Biol. Cybern. 35, 113–124. doi: 10.1007/BF00337436
Priori, A., Foffani, G., Pesenti, A., Tamma, F., Bianchi, A. M., Pellegrini, M., et al. (2004). Rhythm-specific pharmacological modulation of subthalamic activity in Parkinson’s disease. Exp. Neurol. 189, 369–379. doi: 10.1016/j.expneurol.2004.06.001
Pullman, S. L., Watts, R. L., Juncos, J. L., Chase, T. N., and Sanes, J. N. (1988). Dopaminergic effects on simple and choice reaction time performance in Parkinson’s disease. Neurology 38, 249–254. doi: 10.1212/wnl.38.2.249
Pullman, S. L., Watts, R. L., Juncos, J. L., and Sanes, J. N. (1990). Movement amplitude choice reaction time performance in Parkinson’s disease may be independent of dopaminergic status. J. Neurol., Neurosurg. Psychiatry 53, 279–283. doi: 10.1136/jnnp.53.4.279
Rascol, O., Clanet, M., Montastruc, J. L., Simonetta, M., Soulier-Esteve, M. J., Doyon, B., et al. (1989). Abnormal ocular movements in Parkinson’s disease. Evidence for involvement of dopaminergic systems. Brain 112, 1193–1214. doi: 10.1093/brain/112.5.1193
Rascol, O., Sabatini, U., Chollet, F., Celsis, P., Montastruc, J. L., Marc-Vergnes, J. P., et al. (1992). Supplementary and primary sensory motor area activity in Parkinson’s disease. Regional cerebral blood flow changes during finger movements and effects of apomorphine. Arch. Neurol. 49, 144–148. doi: 10.1001/archneur.1992.00530260044017
Rascol, O., Sabatini, U., Chollet, F., Fabre, N., Senard, J. M., Montastruc, J. L., et al. (1994). Normal activation of the supplementary motor area in patients with Parkinson’s disease undergoing long-term treatment with levodopa. J. Neurol. Neurosurg. Psychiatry 57, 567–571. doi: 10.1136/jnnp.57.5.567
Ray, N. J., Jenkinson, N., Wang, S., Holland, P., Brittain, J. S., Joint, C., et al. (2008). Local field potential beta activity in the subthalamic nucleus of patients with Parkinson’s disease is associated with improvements in bradykinesia after dopamine and deep brain stimulation. Exp. Neurol. 213, 108–113. doi: 10.1016/j.expneurol.2008.05.008
Rivaud-Péchoux, S., Vermersch, A. I., Gaymard, B., Ploner, C. J., Bejjani, B. P., Damier, P., et al. (2000). Improvement of memory guided saccades in parkinsonian patients by high frequency subthalamic nucleus stimulation. J. Neurol. Neurosur. Psychiatry 68, 381–384. doi: 10.1136/jnnp.68.3.381
Robichaud, J. A., Pfann, K. D., Comella, C. L., and Corcos, D. M. (2002). Effect of medication on EMG patterns in individuals with Parkinson’s disease. Mov. Disord. 17, 950–960. doi: 10.1002/mds.10218
Rodriguez-Oroz, M. C., Obeso, J. A., Lang, A. E., Houeto, J.-L., Pollak, P., Rehncrona, S., et al. (2005). Bilateral deep brain stimulation in Parkinson’s disease: A multicentre study with 4 years follow-up. Brain 128, 2240–2249. doi: 10.1093/brain/awh571
Sauleau, P., Pollak, P., Krack, P., Courjon, J.-H., Vighetto, A., Benabid, A.-L., et al. (2008). Subthalamic stimulation improves orienting gaze movements in Parkinson’s disease. Clin. Neurophysiol. 119, 1857–1863. doi: 10.1016/j.clinph.2008.04.013
Schettino, L. F., Adamovich, S. V., Hening, W., Tunik, E., Sage, J., and Poizner, H. (2006). Hand preshaping in Parkinson’s disease: Effects of visual feedback and medication state. Exp. Brain Res. 168, 186–202. doi: 10.1007/s00221-005-0080-4
Silberstein, P., Pogosyan, A., Kühn, A. A., Hotton, G., Tisch, S., Kupsch, A., et al. (2005). Cortico-cortical coupling in Parkinson’s disease and its modulation by therapy. Brain 128, 1277–1291. doi: 10.1093/brain/awh480
Starkstein, S. E., Esteguy, M., Berthier, M. L., Garcia, H., and Leiguarda, R. (1989). Evoked potentials, reaction time and cognitive performance in on and off phases of Parkinson’s disease. J. Neurol. Neurosurg. Psychiatry 52, 338–340. doi: 10.1136/jnnp.52.3.338
Tamás, G., Kelemen, A., Radics, P., Valálik, I., Heldman, D., Klivényi, P., et al. (2016). Effect of subthalamic stimulation on distal and proximal upper limb movements in Parkinson’s disease. Brain Res. 1648, 438–444. doi: 10.1016/j.brainres.2016.08.019
Temel, Y., Blokland, A., Ackermans, L., Boon, P., van Kranen-Mastenbroek, V. H., Beuls, E. A., et al. (2006). Differential effects of subthalamic nucleus stimulation in advanced Parkinson disease on reaction time performance. Exp. Brain Res. 169, 389–399. doi: 10.1007/s00221-005-0151-6
Temel, Y., Visser-Vandewalle, V., and Carpenter, R. H. S. (2008). Saccadic latency during electrical stimulation of the human subthalamic nucleus. Curr. Biol. 18, R412–R414. doi: 10.1016/j.cub.2008.03.008
Temel, Y., Visser-Vandewalle, V., and Carpenter, R. H. S. (2009). Saccadometry: A novel clinical tool for quantification of the motor effects of subthalamic nucleus stimulation in Parkinson’s disease. Exp. Neurol. 216, 481–489. doi: 10.1016/j.expneurol.2009.01.007
Temperli, P., Ghika, J., Villemure, J. G., Burkhard, P. R., Bogousslavsky, J., and Vingerhoets, F. J. G. (2003). How do parkinsonian signs return after discontinuation of subthalamic DBS? Neurology 60, 78–81. doi: 10.1212/wnl.60.1.78
Terao, Y., Fukuda, H., Ugawa, Y., and Hikosaka, O. (2013). New perspectives on the pathophysiology of Parkinson’s disease as assessed by saccade performance: A clinical review. Clin. Neurophysiol. 124, 1491–1506. doi: 10.1016/j.clinph.2013.01.021
Terao, Y., Fukuda, H., Yugeta, A., Hikosaka, O., Nomura, Y., Segawa, M., et al. (2011). Initiation and inhibitory control of saccades with the progression of Parkinson’s disease - changes in three major drives converging on the superior colliculus. Neuropsychologia 49, 1794–1806. doi: 10.1016/j.neuropsychologia.2011.03.002
Thobois, S., Mertens, P., Guenot, M., Hermier, M., Mollion, H., Bouvard, M., et al. (2002). Subthalamic nucleus stimulation in Parkinson’s disease: Clinical evaluation of 18 patients. J. Neurol. 249, 529–534. doi: 10.1007/s004150200059
Tokushige, S.-I., Matsuda, S.-I., Oyama, G., Shimo, Y., Umemura, A., Sasaki, T., et al. (2018). Effect of subthalamic nucleus deep brain stimulation on visual scanning. Clin. Neurophysiol. 129, 2421–2432. doi: 10.1016/j.clinph.2018.08.003
Vaillancourt, D. E., Prodoehl, J., Verhagen Metman, L., Bakay, R. A., and Corcos, D. M. (2004). Effects of deep brain stimulation and medication on bradykinesia and muscle activation in Parkinson’s disease. Brain 127, 491–504. doi: 10.1093/brain/awh057
van Stockum, S., MacAskill, M. R., and Anderson, T. J. (2012). Impairment of voluntary saccades and facilitation of reflexive saccades do not co-occur in Parkinson’s disease. J. Clin. Neurosci. 19, 1119–1124. doi: 10.1016/j.jocn.2011.10.014
Velasco, F., and Velasco, M. (1973). A quantitative evaluation of the effects of l-DOPA on Parkinson’s disease. Neuropharmacology 12, 89–99. doi: 10.1016/0028-3908(73)90079-8
Waldthaler, J., Tsitsi, P., and Svenningsson, P. (2019). Vertical saccades and antisaccades: Complementary markers for motor and cognitive impairment in Parkinson’s disease. NPJ Parkinsons Dis. 5:11. doi: 10.1038/s41531-019-0083-7
Yugeta, A., Terao, Y., Fukuda, H., Hikosaka, O., Yokochi, F., Okiyama, R., et al. (2010). Effects of STN stimulation on the initiation and inhibition of saccade in Parkinson disease. Neurology 74, 743–748. doi: 10.1212/WNL.0b013e3181d31e0b
Keywords: Parkinson’s disease, antiparkinson medication, levodopa, subthalamic nucleus deep brain stimulation, saccade, reaching
Citation: Munoz MJ, Arora R, Rivera YM, Drane QH, Pal GD, Verhagen Metman L, Sani SB, Rosenow JM, Goelz LC, Corcos DM and David FJ (2023) Medication only improves limb movements while deep brain stimulation improves eye and limb movements during visually-guided reaching in Parkinson’s disease. Front. Hum. Neurosci. 17:1224611. doi: 10.3389/fnhum.2023.1224611
Received: 17 May 2023; Accepted: 18 September 2023;
Published: 02 October 2023.
Edited by:
Adolfo Ramirez-Zamora, University of Florida, United StatesReviewed by:
Yasuo Terao, Kyorin University, JapanCopyright © 2023 Munoz, Arora, Rivera, Drane, Pal, Verhagen Metman, Sani, Rosenow, Goelz, Corcos and David. This is an open-access article distributed under the terms of the Creative Commons Attribution License (CC BY). The use, distribution or reproduction in other forums is permitted, provided the original author(s) and the copyright owner(s) are credited and that the original publication in this journal is cited, in accordance with accepted academic practice. No use, distribution or reproduction is permitted which does not comply with these terms.
*Correspondence: Miranda J. Munoz, bWlyYW5kYW11bm96MjAyMkB1Lm5vcnRod2VzdGVybi5lZHU=
Disclaimer: All claims expressed in this article are solely those of the authors and do not necessarily represent those of their affiliated organizations, or those of the publisher, the editors and the reviewers. Any product that may be evaluated in this article or claim that may be made by its manufacturer is not guaranteed or endorsed by the publisher.
Research integrity at Frontiers
Learn more about the work of our research integrity team to safeguard the quality of each article we publish.