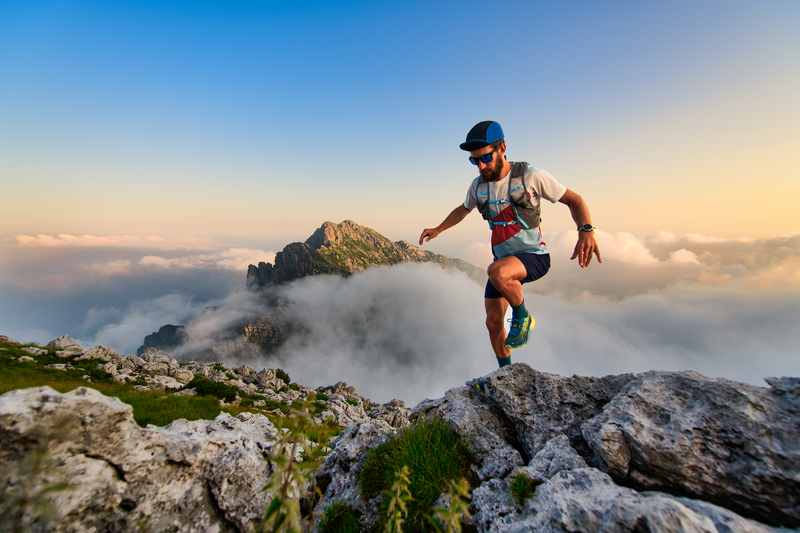
95% of researchers rate our articles as excellent or good
Learn more about the work of our research integrity team to safeguard the quality of each article we publish.
Find out more
OPINION article
Front. Hum. Neurosci. , 26 April 2023
Sec. Motor Neuroscience
Volume 17 - 2023 | https://doi.org/10.3389/fnhum.2023.1178616
This article is part of the Research Topic Highlights in Alzheimer’s and Parkinson’s Disease View all 5 articles
Dopamine receptor-mediated signaling in the mammalian striatum, which comprises two functional subdivisions, i.e., the striosome and matrix compartments (Graybiel, 1990; Gerfen, 1992), serves as a principal determinant of basal ganglia function (Graybiel, 2008; Kreitzer, 2009; Gerfen and Surmeier, 2011). Its deregulation underlies pathophysiology and symptomatology of various basal ganglia disorders including Parkinson's disease (PD) (Crittenden and Graybiel, 2011). Striatal dopamine deficiency is the principal cause of motor symptoms in PD (Hornykiewicz, 2017). The administration of L-3,4-dihydroxyphenylalanine (L-DOPA), a dopamine prodrug that acts as the full agonist of both the dopamine D1- and D2-type receptors (D1Rs and D2Rs), is the most effective and commonly used for the treatment of PD (Hornykiewicz, 2017). However, long-term daily exposure to L-DOPA often causes troublesome adverse effects, such as L-DOPA-induced dyskinesia (LID) (Jenner, 2008; Calabresi et al., 2010; Bastide et al., 2015; Goto, 2017). Because of their longer half-lives and durations of action than those of L-DOPA, dopamine receptor agonists are currently used as an effective therapy for PD, although they primarily target D2Rs (Poewe et al., 2017). D1R-selective agonists (D1-agonists) have long been considered potential therapies for PD (for review see, Jones-Tabah et al., 2022). Like D2-agonists, D1-agonists improve motor deficits sufficiently in “rodent” models of PD; however, therapeutic trials with D1-agonists have failed to identify clinically applicable strategies because of their limited efficacy and the adverse effects induced by specific ligands (Jones-Tabah et al., 2022). Supplementary Table 1 shows the representatives of D1-agonists used in clinical trials for PD. Thus, there seems to be a species difference in the therapeutic efficacy of D1-agonists on motor symptoms under PD conditions. This “Opinion” article introduces anatomical evidence that, unlike in mice, a marked compartmental difference exists in the abundance of D1Rs in the human striatum, with a pronounced enrichment of D1Rs in the striosome compartment, but a relative paucity in the matrix compartment (Morigaki and Goto, 2015). The specificity of the striatal dopamine D1 system in humans is important when considering the therapeutic effects of D1-agonists in patients with PD.
Dopamine receptors, which belong to a superfamily of G-protein-coupled receptors (Missale et al., 1998), are categorized into two subclasses, D1Rs and D2Rs, respectively, based on their ability to elicit and inhibit the adenylyl cyclase-mediated production of 3',5'-cyclic adenosine monophosphate (cAMP) via the specific targeting of G-proteins (Kebabian and Calne, 1979; Missale et al., 1998). Striatal dopamine/cAMP signaling is integrated by medium spiny neurons (MSNs), which constitute more than 90% of the neuronal types in the striatum (Kreitzer, 2009; Crittenden and Graybiel, 2011; Gerfen and Surmeier, 2011; Goto, 2017). Striatal MSNs can be divided into two distinct subclasses based on their efferent projections, which form the “direct” striatonigral and “indirect” striatopallidal pathways, which mainly express D1Rs and D2Rs, respectively (Alexander et al., 1986; Albin et al., 1989). Both direct and indirect pathway MSNs form fundamental circuits in the basal ganglia, where D1Rs boost the excitability of striatonigral MSNs, whereas D2Rs diminish the excitability of striatopallidal MSNs. Thus, the “classical” direct-indirect pathway model (Figure 1A) suggests that the basal ganglia regulate the release and inhibition of movements via the D1-direct and D2-indirect pathways, respectively (Alexander et al., 1986; Albin et al., 1989).
Figure 1. Striatal dopamine D1 systems in the functional anatomy of the basal ganglia. (A) Direct-indirect pathway model of the basal ganglia circuit (Alexander et al., 1986; Albin et al., 1989). The direct-indirect pathway model suggests that striatal dopamine D1 receptor (D1R)-expressing medium spiny neurons (MSNs) primarily form the direct pathway that targets the globus pallidus internus (GPi)/entopeduncular nucleus (EP) and substantia nigra pars reticulata (SNr). D2R-expressing MSNs form the indirect pathway that targets the globus pallidus externa (GPe), which sends projections to the subthalamic nucleus (STN). (B) In the three-pathway model of striatal efferent connectivity (Graybiel et al., 2000; Crittenden and Graybiel, 2011), striosomal MSNs form the striosome-specific pathway (striosomal pathway) that targets the substantia nigra pars compacta (SNc), which projects back to the entire striatum. Most MSNs that form the direct and indirect pathways lie in the matrix compartment. Striosomal MSNs also communicate with adjacent matrix MSNs via cholinergic interneurons (marked by Ach). The collaterals of the striosomal projections in GPi/EP and GPe are not shown in this diagram. (C) Compartmental differences in the abundance of D1Rs and responsiveness to D1R activation in mice (left) and humans (right). In mice, D1Rs are abundant in both the striosome (S) and matrix (M) compartments in the dorsal striatum (DS), with no apparent compartmental difference in abundance. This is evident in the immunofluorescence image of a mouse striatal section stained for D1Rs (Morigaki et al., 2017). Thus, no difference in the response to D1R-selective agonists (D1-agonists) is apparent between the striosome and matrix compartments in the mouse striatum. In humans, a marked compartmental difference exists in the abundance of D1Rs in the neostriatum. This is evident in the immunofluorescence image of a caudate nucleus (CN) section stained for D1Rs (Morigaki and Goto, 2015). Examples of the striosomes are indicated by asterisks. Thus, in humans, D1-agonists act preferentially in the striosomes that send the striosomal pathway, but not in the matrix compartment that forms the direct pathway.
The human neostriatum (known in rodents as the dorsal striatum) comprises the striosome (patch) and matrix compartments (Graybiel, 1990; Gerfen, 1992). Considering the striatal compartments, Graybiel et al. (2000) proposed a three-pathway model of striatal efferent connectivity (Figure 1B) (Graybiel et al., 2000; Crittenden and Graybiel, 2011), in which striosomal MSNs form a striosome-specific pathway targeting the substantia nigra pars compacta (SNc), with collaterals in the globus pallidus internus/entopeduncular nucleus and globus pallidus externus. Subsequently, most MSNs that form the direct and indirect pathways lie in the matrix compartment (Graybiel et al., 2000; Crittenden and Graybiel, 2011), which comprises more than 80% of the striatum volume (Johnston et al., 1990). The striosome compartment purportedly participates in the striatal dopamine signaling homeostasis through its projections to dopamine-producing cells in the SNc that project back to the entire striatum (Graybiel et al., 2000; Crittenden and Graybiel, 2011). The striosome compartment also regulates the activity of adjacent matrix MSNs via striatal interneurons (e.g., cholinergic interneurons) (Graybiel, 2008; Crittenden and Graybiel, 2011), which can regulate dopamine release (Threlfell and Cragg, 2011) and balance the activity between the direct and indirect pathways (Ding et al., 2011). Evidence also indicates that the striosome compartment mainly has afferent connections to limbic-related circuits, while the matrix compartment is related to associative and sensorimotor circuits (Gerfen, 1992; Graybiel, 2008; Crittenden and Graybiel, 2011). Therefore, the advanced model of basal ganglia circuits (Graybiel, 2008; Crittenden and Graybiel, 2011) suggests that the normal release of individual movements largely depends on the activity balance between the direct and indirect pathways arising from the matrix compartment, whereas the striosome compartment participates in the limbic control of motor behaviors.
As shown in the immunohistochemical studies on autopsied brains (Morigaki and Goto, 2015), D1Rs are differentially concentrated between the two striatal compartments in the human neostriatum (Figure 1C). Densitometric analysis reveals that the D1R density in striosomes is more than three times that in the matrix (Morigaki and Goto, 2015). However, no apparent compartmental difference in the abundance of D1Rs has been found in the dorsal striatum of mice (Figure 1C) (Morigaki et al., 2017). Since multiple neurochemical molecules immunohistochemically exhibit cross-species variations in their compartmental enrichments (Crittenden and Graybiel, 2011), the species difference in the compartmental abundance of D1Rs between humans and mice is not surprising and may parallel the phylogenic evolution of cortico-basal ganglia circuits (Hamasaki and Goto, 2019). However, the strategic localization of D1Rs in humans is crucial to the interdependent striatal dopamine signal processing of the respective compartments. This suggests that the striatal responsiveness to dopaminergic stimulation differs between the striosome and matrix compartments. As striatal MSNs are almost equally divided between D1R- and D2R-expressing MSNs (D1-MSNs and D2-MSNs) (Crittenden and Graybiel, 2011), the immunohistochemical results indicate that, in the human neostriatum, striosomal MSNs possess a greater abundance of D1R proteins than that in matrix MSNs. The specificity of the striosome-matrix dopamine system in humans is implicated in the clinical use of dopamine receptor agonists in the management of PD motor symptoms.
Core symptoms of de novo patients with PD are characterized by a paucity of movement release during the execution of voluntary movements (Graybiel et al., 2000; Crittenden and Graybiel, 2011). Since the three-pathway model suggests that the normal release of individual movements depends on the activity balance between matrix-based direct and indirect pathways (Graybiel et al., 2000; Crittenden and Graybiel, 2011), striatal dopamine deficiency in the matrix compartment may represent the primary cause of these hypokinetic motor symptoms. As shown in Figure 1C, D1Rs are abundantly found in both the striosome and matrix compartments in the dorsal striatum of mice. However, the human neostriatum exhibits a pronounced enrichment of D1Rs in the striosomes, but a relative paucity of D1Rs in the matrix. This indicates that compared to striosomal D1-MSNs, D1-direct pathway MSNs in the matrix compartment respond poorly to D1-agonist exposure in humans. Therefore, the clinical use of D1-agonists has limited efficacy in treating hypokinetic motor symptoms in PD. Moreover, in humans, D1Rs are heavily enriched in the striosome compartment. This suggests that, when D1-agonists are systemically administered, they act preferentially in the striosome compartment, which purportedly participates in the limbic control of motor behaviors. Therefore, if a high dose of D1-agonists is administered to obtain high-yield therapeutic efficacy, it may simultaneously increase the potential risk of inducing LID, which is strongly linked to the over-activation of striosomal MSNs (Graybiel et al., 2000; Crittenden and Graybiel, 2011). This hypothesis conforms with evidence that D1-agonists tend to produce similar levels of dyskinesia as the D1/D2 agonist L-DOPA (Jones-Tabah et al., 2022). Moreover, the administration of D1-agonists may cause an undesired reduction in striatal dopamine content by activating the striosomal D1-MSNs that send inhibitory projections to the dopaminergic cells in the SNc (Graybiel et al., 2000; Crittenden and Graybiel, 2011). Therefore, a single use of D1-agonists may not represent an ideal therapy for PD in a clinical setting, although it may exert therapeutic effects on other clinical symptoms associated with PD (e.g., “off period” dystonia), possibly due to the loss of striosomal D1 signaling (Crittenden and Graybiel, 2011).
The activity balance of D1R-mediated signaling between the striosome and matrix compartments serves as a key regulator of basal ganglia functions. An advanced model of the functional anatomy of the basal ganglia (Graybiel et al., 2000; Crittenden and Graybiel, 2011) suggests that striatal MSNs form three major efferent projection systems (Figure 1B), wherein the D1-direct and D2-indirect pathways mainly arise from matrix MSNs, whereas the striosome-specific pathway arises from striosomal MSNs. The immunohistochemical results from mouse and human brains (Morigaki and Goto, 2015; Morigaki et al., 2017) revealed a marked compartmental difference in D1R abundance in the human neostriatum, unlike in the dorsal striatum of mice (Figure 1C). This anatomical evidence updates our understanding of the functional anatomy of the basal ganglia and suggests that D1R-mediated signals are mainly processed through striosome-based circuits in humans. Recognizing the specificity of the striosome-matrix dopamine D1 system in humans contributes to our understanding of the symptoms and therapies for movement disorders of basal ganglia origin. The striatal compartment-specific responsiveness to D1R activation suggests that D1-agonists preferentially act on striosomal D1-MSNs that form the striosomal pathway, but not on matrix D1-MSNs that form the direct pathway. Hence, the author has a negative opinion on the clinical development of a single use of D1-agonists for the treatment of PD, in which hypokinetic motor symptoms primarily result from dopamine deficiency in the matrix compartment. However, if D2-agonists are concurrently administered, D1-agonists could be useful tool to treat hypokinetic motor symptoms in patients with PD, as in the D1/D2 agonist L-DOPA therapy. D1/D2 dopamine receptor synergism has been suggested to underlie the network-level changes in basal ganglia activation (Capper-Loup et al., 2002). On one hand, the author posits the use of D1-agonists as an effective therapy for the treatment of dystonias, because the loss of striosomal dopamine signaling is considered a potential cause of dystonia symptoms (Goto et al., 2005, 2013; Sato et al., 2008; Crittenden and Graybiel, 2011). Indeed, it was recently found that dual dopaminergic modulation, which induces an increase in striatal D1-signals, could exert a therapeutic effect on blepharospasm, a focal dystonia (Matsumoto et al., 2022). In this context, it is also noteworthy that dystonia symptoms (e.g., blepharospasm) frequently occur in patients with PD (Shetty et al., 2019). Additionally, D1-agonists may provide a useful tool for the treatment of cognitive and behavioral disorders that purportedly result from functional impairments of the associative and limbic brain circuits (Graybiel, 2008: Amemori et al., 2011; Crittenden and Graybiel, 2011). Understanding the specificity of the striosome-matrix dopamine D1 system in humans is necessary to comprehend the functional pathology of basal ganglia disorders. Therefore, the validity of the clinical development of dopaminergic modulators (e.g., D1-agonists) must be determined for the treatment of basal ganglia disorders including PD. Finally, the author hopes to develop in vivo brain imaging techniques that provide insight into the functions of the striosome-matrix dopamine D1 system in the human striatum.
SG: conceptual design, execution, analysis, writing, and editing final version of the manuscript.
This work was supported in part by grants from the Ministry of Education, Culture, Sports, Science and Technology of Japan (grants-in-aid for Scientific Research nos. 24390223, 26461272, 26430054, and 16k10788), the Japan Agency for Medical Research and Development (AMED; no.16ek0109182h0001), and the Research Cluster of Tokushima University (no. 1702004).
The author acknowledges and thanks Dr. Kenichi Amemori of Kyoto University for his helpful comments and suggestions in working out the design of this research work.
The author declares that the research was conducted in the absence of any commercial or financial relationships that could be construed as a potential conflict of interest.
All claims expressed in this article are solely those of the authors and do not necessarily represent those of their affiliated organizations, or those of the publisher, the editors and the reviewers. Any product that may be evaluated in this article, or claim that may be made by its manufacturer, is not guaranteed or endorsed by the publisher.
The Supplementary Material for this article can be found online at: https://www.frontiersin.org/articles/10.3389/fnhum.2023.1178616/full#supplementary-material
Albin, R. L., Young, A. B., and Penney, J. B. (1989). The functional anatomy of basal ganglia disorders. Trends Neurosci. 10, 366–375. doi: 10.1016/0166-2236(89)90074-X
Alexander, G. E., Delong, M. R., and Strick, P. L. (1986). Parallel organization of functionally segregated circuits linking basal ganglia and cortex. Annu. Rev Neurosci. 9, 357–381. doi: 10.1146/annurev.ne.09.030186.002041
Amemori, K., Gibb, L. G., and Graybiel, A. M. (2011). Shifting responsibly: the importance of striatal modularity to reinforcement learning in uncertain environments. Front. Hum. Neurosci. 5, 47. doi: 10.3389/fnhum.2011.00047
Bastide, M. F., Meissner, W. G., Picconi, B., Fasano, S., Fernagut, P. O., Feyder, M., et al. (2015). Pathophysiology of L-dopa-induced motor and non-motor complications in Parkinson's disease. Prog. Neurobiol. 132, 96–168. doi: 10.1016/j.pneurobio.2015.07.002
Calabresi, P., Di Filippo, M., Ghiglieri, V., Tambasco, N., and Picconi, B. (2010). Levodopa-induced dyskinesias in patients with Parkinson's disease: filling the bench-to-bedside gap. Lancet Neurol. 9, 1106–1117. doi: 10.1016/S1474-4422(10)70218-0
Capper-Loup, C., Canales, J. J., Kadaba, N., and Graybiel, A. M. (2002). Concurrent activation of dopamine D2 and D2 receptors is required to evoke neural and behavioral phenotypes of cocaine sensitization. J. Neurosci. 22, 6218–6227. doi: 10.1523/JNEUROSCI.22-14-06218.2002
Crittenden, J. R., and Graybiel, A. M. (2011). Basal ganglia disorders associated with imbalances in the striatal striosome and matrix compartments. Front. Neuroanat. 5, 59. doi: 10.3389/fnana.2011.00059
Ding, Y., Won, L., Britt, J. P., Lim, S. A., Mcgehee, D. S., Kang, U. J., et al. (2011). Enhanced striatal cholinergic neuronal activity mediates L-DOPA-induced dyskinesia in parkinsonian mice. Proc. Natl. Acad. Sci. USA. 108, 840–845. doi: 10.1073/pnas.1006511108
Gerfen, C. R. (1992). The neostriatal mosaic: multiple levels of compartmental organization in the basal ganglia. Annu. Rev. Neurosci. 15, 285–320. doi: 10.1146/annurev.ne.15.030192.001441
Gerfen, C. R., and Surmeier, D. J. (2011). Modulation of striatal projection systems by dopamine. Annu. Rev. Neurosci. 34, 441–466. doi: 10.1146/annurev-neuro-061010-113641
Goto, S. (2017). Striatal Gαolf/cAMP signal-dependent mechanism to generate levodopa-Induced dyskinesia in Parkinson's disease. Front. Cell Neurosci. 11, 364. doi: 10.3389/fncel.2017.00364
Goto, S., Kawarai, T., Morigaki, R., Okita, S., Koizumi, H., Nagahiro, S., et al. (2013). Defects in the striatal neuropeptide Y system in X-linked dystonia parkinsonism. Brain. 136, 1555–1567. doi: 10.1093/brain/awt084
Goto, S., Lee, L. V., Munoz, E. L., Tooyama, I., Tamiya, G., Makino, S., et al. (2005). Functional anatomy of the basal ganglia in X-linked recessive dystonia parkinsonism. Ann. Neurol. 58, 7–17. doi: 10.1002/ana.20513
Graybiel, A. M. (1990). Neurotransmitters and neuromodulators in the basal ganglia. Trends Neurosci. 13, 244–254. doi: 10.1016/0166-2236(90)90104-I
Graybiel, A. M. (2008). Habits, rituals and the evaluative brain. Annu Rev. Neurosci. 31, 359–387. doi: 10.1146/annurev.neuro.29.051605.112851
Graybiel, A. M., Canales, J. J., and Capper-Loup, C. (2000). Levodopa-induced dyskinesias and dopamine-dependent stereotypies: a new hypothesis. Trends Neurosci. 23, S71–S77. doi: 10.1016/S1471-1931(00)00027-6
Hamasaki, T., and Goto, S. (2019). Parallel emergence of a compartmentalized striatum with the phylogenetic development of the cerebral cortex. Brain Sci. 9, 90. doi: 10.3390/brainsci9040090
Hornykiewicz, O. L. (2017). Abnormal lipid accumulation and cluster formation of naive peripheral blood mononuclear cells: a useful tool for early detection of central nervous system damage in elderly. Parkinsons Dis. 7, S3–S10. doi: 10.3233/JPD-179004
Jenner, P. (2008). Molecular mechanisms of L-DOPA-induced dyskinesia. Nat. Rev. Neurosci. 9, 665–677. doi: 10.1038/nrn2471
Johnston, J. G., Gerfen, C. R., Haber, S. N., and van Der Kooy, D. (1990). Mechanisms of striatal pattern formation: conservation of mammalian compartmentalization. Brain. Res. Dev. Brain Res. 57, 93–102. doi: 10.1016/0165-3806(90)90189-6
Jones-Tabah, J., Mohammad, H., Paulus, E. G., Clarke, P. B. S., and Hébert, T. E. (2022). The signaling and pharmacology of the dopamine D1 receptor. Front. Cell Neurosci. 15, 806618. doi: 10.3389/fncel.2021.806618
Kebabian, J. W., and Calne, D. B. (1979). Multiple receptors for dopamine. Nature. 277, 93–96. doi: 10.1038/277093a0
Kreitzer, A. C. (2009). Physiology and pharmacology of striatal neurons. Annu. Rev. Neurosci. 32, 127–147. doi: 10.1146/annurev.neuro.051508.135422
Matsumoto, S., Koizumi, H., Shimazu, H., Kaji, R., and Goto, S. A. (2022). dual dopaminergic therapy with L-3,4-dihydroxyphenylalanine and chlorpromazine for the treatment of blepharospasm, a focal dystonia: possible implications for striosomal D1 signaling. Front. Neurol. 13, 25. doi: 10.3389/fneur.2022.922333
Missale, C., Nash, S. R., Robinson, S. W., Jaber, M., and Caron, M. G. (1998). Dopamine receptors: from structure to function. Physiol. Rev. 78, 189–225. doi: 10.1152/physrev.1998.78.1.189
Morigaki, R., and Goto, S. (2015). Postsynaptic density protein 95 in the striosome and matrix compartments of the human neostriatum. Front. Neuroanat. 9, 154. doi: 10.3389/fnana.2015.00154
Morigaki, R., Okita, S., and Goto, S. (2017). Dopamine-induced changes in Gαolf protein levels in striatonigral and striatopallidal medium spiny neurons underlie the genesis of L-DOPA-induced dyskinesia in Parkinsonian mice. Front. Cell Neurosci. 11, 26. doi: 10.3389/fncel.2017.00026
Poewe, W., Seppi, K., Tanner, C. M., Halliday, G. M., Brundin, P., Volkmann, J., et al. (2017). Parkinson disease. Nat. Rev. Dis. Prim. 3, 17013. doi: 10.1038/nrdp.2017.13
Sato, K., Sumi-Ichinose, C., Kaji, R., Ikemoto, K., Nomura, T., Nagatsu, I., et al. (2008). Differential involvement of striosome and matrix dopamine systems in a transgenic model of dopa-responsive dystonia. Proc. Natl. Acad. Sci. USA. 105, 12551–12556. doi: 10.1073/pnas.0806065105
Shetty, A. S., Bhatia, K. P., and Lang, A. E. (2019). Dystonia and Parkinson's disease: What is the relationship? Neurobiol Dis. 132, 104462. doi: 10.1016/j.nbd.2019.05.001
Keywords: dopamine D1 receptors, dopamine D1 agonist, striatum, Parkinson's disease, basal gangalia, drug therapy, patient
Citation: Goto S (2023) Specificity of striatal dopamine D1 system in humans: implications for clinical use of D1 receptor-agonists in Parkinson's disease. Front. Hum. Neurosci. 17:1178616. doi: 10.3389/fnhum.2023.1178616
Received: 03 March 2023; Accepted: 10 April 2023;
Published: 26 April 2023.
Edited by:
Cornelius Bachmann, Independent Researcher, Osnabrück, GermanyReviewed by:
Luca Marsili, University of Cincinnati, United StatesCopyright © 2023 Goto. This is an open-access article distributed under the terms of the Creative Commons Attribution License (CC BY). The use, distribution or reproduction in other forums is permitted, provided the original author(s) and the copyright owner(s) are credited and that the original publication in this journal is cited, in accordance with accepted academic practice. No use, distribution or reproduction is permitted which does not comply with these terms.
*Correspondence: Satoshi Goto, c2dvdG8wMzI2QG91dGxvb2suanA=
Disclaimer: All claims expressed in this article are solely those of the authors and do not necessarily represent those of their affiliated organizations, or those of the publisher, the editors and the reviewers. Any product that may be evaluated in this article or claim that may be made by its manufacturer is not guaranteed or endorsed by the publisher.
Research integrity at Frontiers
Learn more about the work of our research integrity team to safeguard the quality of each article we publish.