- 1Department of Physical Therapy, School of Health Professions, University of Alabama at Birmingham, Birmingham, AL, United States
- 2Department of Biomedical Engineering, Heersink School of Medicine, University of Alabama at Birmingham, Birmingham, AL, United States
- 3Department of Physical Medicine and Rehabilitation, Heersink School of Medicine, University of Alabama at Birmingham, Birmingham, AL, United States
- 4Department of Clinical and Diagnostic Studies, School of Health Professions, University of Alabama at Birmingham, Birmingham, AL, United States
Rehabilitation approaches for individuals with neurologic conditions have increasingly shifted toward promoting neuroplasticity for enhanced recovery and restoration of function. This review focuses on exercise strategies and non-invasive neuromodulation techniques that target neuroplasticity, including transcranial magnetic stimulation (TMS), vagus nerve stimulation (VNS), and peripheral nerve stimulation (PNS). We have chosen to focus on non-invasive neuromodulation techniques due to their greater potential for integration into routine clinical practice. We explore and discuss the application of these interventional strategies in four neurological conditions that are frequently encountered in rehabilitation settings: Parkinson’s Disease (PD), Traumatic Brain Injury (TBI), stroke, and Spinal Cord Injury (SCI). Additionally, we discuss the potential benefits of combining non-invasive neuromodulation with rehabilitation, which has shown promise in accelerating recovery. Our review identifies studies that demonstrate enhanced recovery through combined exercise and non-invasive neuromodulation in the selected patient populations. We primarily focus on the motor aspects of rehabilitation, but also briefly address non-motor impacts of these conditions. Additionally, we identify the gaps in current literature and barriers to implementation of combined approaches into clinical practice. We highlight areas needing further research and suggest avenues for future investigation, aiming to enhance the personalization of the unique neuroplastic responses associated with each condition. This review serves as a resource for rehabilitation professionals and researchers seeking a comprehensive understanding of neuroplastic exercise interventions and non-invasive neuromodulation techniques tailored for specific diseases and diagnoses.
Introduction
Neuroplasticity refers to the brain’s unique capacity to reorganize, modify, and adjust. It encompasses the central nervous system’s ability to forge, reinforce, and restructure neural connections in response to changes in sensory inputs or motor demands. Neuroplasticity plays a crucial role in the acquisition of new skills as well as in the recovery and rehabilitation processes following the diagnosis of various neurological conditions. This involves a complex interplay of coordinated neurotransmitter release, including acetylcholine (ACh), norepinephrine (NE), epinephrine (Epi), and dopamine (DA). The release of these neurotransmitters induces changes in both white and gray matter through neurogenesis, synaptogenesis, angiogenesis, and gliogenesis (Zatorre et al., 2012), thereby creating stronger and more efficient connections within the relevant neural pathways (Figure 1). These changes enable more efficient information translation through neural circuits and aid in successful task completion. The effectiveness of both exercise and neuromodulation can be attributed to their ability to promote neuroplastic change.
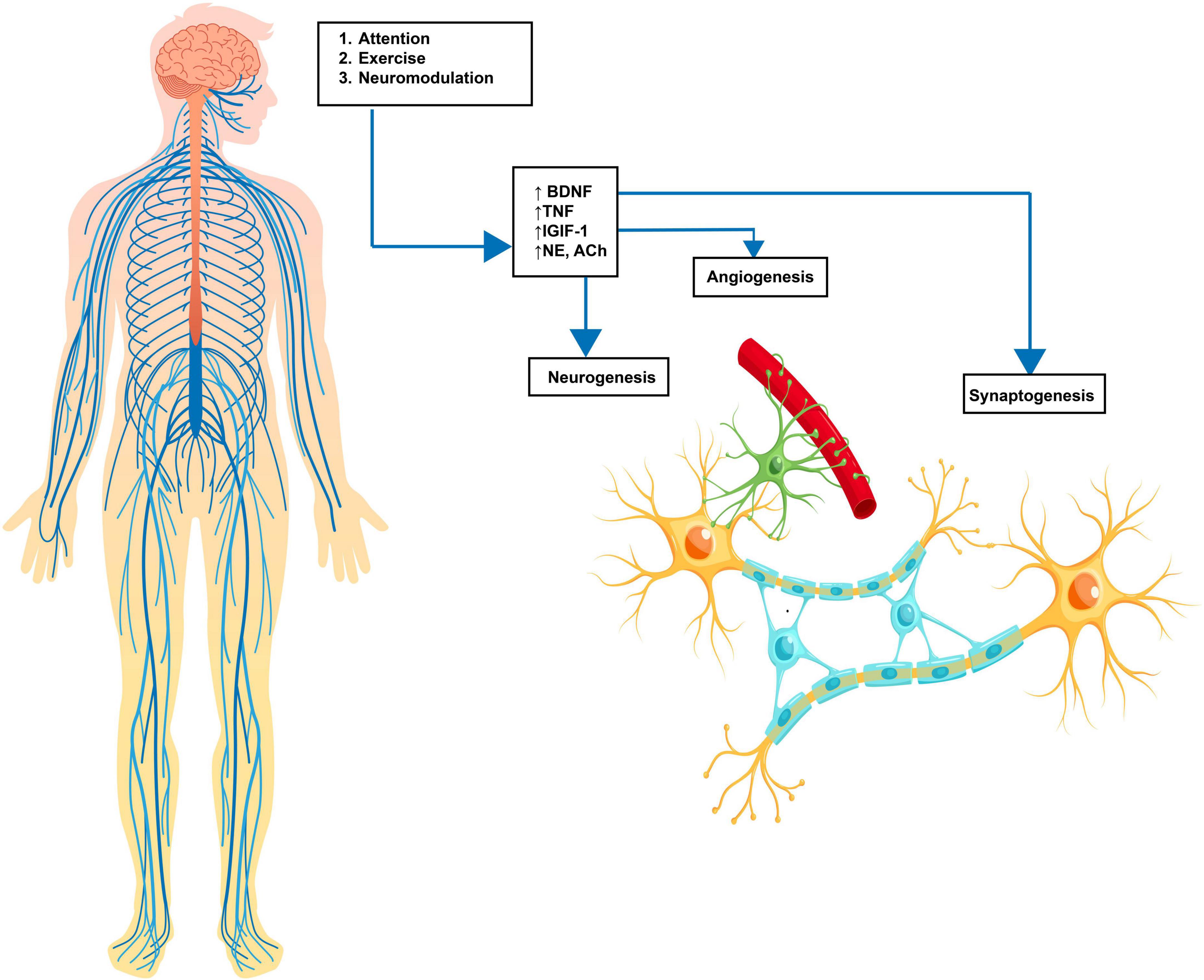
Figure 1. Neuroplasticity cascade. Enviromental input via learning, exercise or neuromodulation causes an increase in molecular substrates, including BDNF (brain-derived neurotrophic Factor), TNF (tumor necrosis factor), NE (norepinephrine), DA (dopamine), and 5-HT (serotonin). These molecular changes lead to neuroplastic cellular changes, including angiogenesis (new blood supply), neurogenesis (new neuronal growth), and synaptogenesis (increased synaptic connectivity). The result is an increased strength of neural pathways. Source: Biorender.com.
Understanding how natural and induced neuroplastic changes can occur in the brain is vital for the development of effective rehabilitative treatments involving exercise and neuromodulation. For example, neuroplasticity-based approaches may exhibit differing impacts, depending on whether the individual has experienced a neurological insult or is living with a neurodegenerative disease. Following neurological insults such as stroke, traumatic brain injury (TBI), and spinal cord injury (SCI), neuroplastic changes occur naturally to compensate for new structural and functional deficits. This compensation is achieved through the rewiring of neural pathways and significant cortical alterations in brain function (Nudo, 2007). Therefore, an individual who has experienced a neurological insult may display a heightened state of neuroplasticity following the event as the brain actively works to restore lost function. In contrast, individuals with neurodegenerative diseases experience a gradual and progressive loss of neuronal function and structure. In this case, the goal of a neuroplasticity-based approach is to slow this decline by stimulating the brain’s inherent plasticity to compensate for the loss of function over time. Ultimately, optimizing patient outcomes through a neuroplasticity-based approach hinges upon a deep understanding of the neuroplastic changes that occur in distinct pathological conditions, and how we can strategically manipulate the human nervous system through exercise and neuromodulation to enhance neuroplasticity.
In this review, we explore the intersection of neuromodulation and rehabilitation, investigating how these combined approaches shape neuroplastic changes across a range of neurologic diagnoses. Our primary focus is on the motor aspects of these conditions, as the enhancement of motor function is the primary aim of physical therapy in neurorehabilitation. However, we also acknowledge that these condition also impact non-motor aspects, and we touch on non-motor aspects throughout the manuscript. We have selected to discuss both neurodegenerative disease and neurological insults, considering their distinct mechanisms of action that could affect their response to neuroplasticity. Given the vast spectrum of research available, the constraints of space, and individual variations in treatment responses, we will concentrate on how exercise and neuromodulation promote neuroplasticity within a selection of neurological diagnoses commonly encountered in the rehabilitation setting. The conditions we have chosen to focus on include Parkinson’s Disease (PD), Traumatic Brain Injury (TBI), Stroke, and Spinal Cord Injury (SCI). Subsequently, we delve into three non-invasive neuromodulation techniques – Transcranial Magnetic Stimulation (TMS), Vagus Nerve Stimulation (VNS), and Peripheral Nerve Stimulation (PNS). These techniques have been chosen due to the available evidence highlighting these modalities’ capacity to induce neuroplastic changes. Additionally, their non-invasive nature amplifies their relevance in a clinical context, indicating a higher likelihood of integration into rehabilitation settings. We will also briefly touch upon emerging technologies such as transcranial direct current stimulation (tDCS) and focused ultrasound (FUS), acknowledging their potential and indicating areas where further research is needed for their safe and effective implementation in clinical practice.
Our primary objective is to offer a comprehensive overview of the existing literature concerning the application of combined exercise and neuromodulation in rehabilitation for selected diagnoses. To achieve this, we start by examining the role of exercise in enhancing neuroplasticity across the selected conditions. We then transition into a discussion of neuromodulation approaches, including the latest research findings within each diagnosis. Within these sections, we present studies that have effectively integrated exercise and neurostimulation for enhanced outcomes, advocating for a combined approach of neuromodulation and physical therapy. Finally, we propose specific, innovative interventions that merge exercise and neurostimulation for each diagnosis discussed, paving the way for future research in this promising field. By understanding the unique neural targets, optimal intervention timing and parameters, and the appropriate type and intensity of treatment protocols for each patient, we aim to establish a foundational framework for integrating exercise and neurostimulation approaches in the rehabilitation setting. The suggested interventions offered in this article promote potential research directions, emphasizing careful intervention selection tailored to each patient’s diagnosis, while considering each intervention’s unique neuroplastic effects. By adopting this neuroscience-guided approach, we aim to ultimately enhance patient outcomes and contribute to the advancement of the field of neurorehabilitation.
Exercise and neuroplasticity
While the positive effects of exercise on overall health have been well established (Powell and Paffenbarger, 1985; Booth et al., 2002) it is only during the last decade that researchers have delved more deeply into the effects of exercise on brain health (van Praag, 2009). Exercise, specifically aerobic exercise, offers a wealth of benefits for brain health, including enhancing neuroplasticity by creating a neural environment that is primed for change. One of the pathways through which exercise induces neuroplasticity is the production of brain-derived neurotrophic factor (BDNF) (Murer et al., 2001), a protein that promotes the growth and survival of neurons. Exercise stimulates the release of BDNF, setting off a chain of events leading to structural and functional transformations within the brain (Cotman et al., 2007; Hamilton and Rhodes, 2015). For example, increased levels of BDNF activate tropomyosinrelated receptor kinase B (TrkB), which influences neuronal dendritic spine growth (synaptogenesis) (Guo et al., 2018), ultimately increasing post-synaptic drive to the motor neuron and improving nervous system communication. This increase in BDNF with exercise has been observed in both animal (Neeper et al., 1996) and human studies (Rasmussen et al., 2009), serving as an important mechanism underlying exercise-induced neuroplasticity and cognitive enhancement (Berchtold et al., 2005; Intlekofer et al., 2013). In addition to BDNF production, exercise also elevates insulin-like growth factor-1 (IGF-1), which, like BDNF, facilitates exercise-induced growth of blood vessels (angiogenesis) (Ding et al., 2006) and the formation of new neurons (neurogenesis) (Lopez-Lopez et al., 2004). Additionally, IGF-1 stimulates BDNF upregulation (Carro et al., 2001; Ding et al., 2006), further contributing to enhanced neural function and cognitive performance. Another growth factor contributing to the beneficial effects of exercise is vascular endothelial growth factor (VEGF), which is linked to the proliferation of neurons and the growth of blood vessels (Fabel et al., 2003). Overall, these changes at a cellular and molecular level drive changes in brain structure (both white and gray matter) and function, resulting in an increased efficiency of neural activation and communication, ultimately changing cognitive and motor performance (El-Sayes et al., 2019). It is worth noting that while these systemic effects create a favorable environment for neuroplasticity, the specific skills acquired through these changes may depend on other factors and interactions.
Implications for exercise-based therapies across neurological disorders and injury
Parkinson’s disease
Parkinson’s disease is a degenerative neurological condition that gradually damages dopamineproducing neurons in two regions of the brain, namely the substantia nigra pars compacta (SNc) and ventral tegmental area (VTA), leading to dopamine deficiency. The disease presents with both motor (bradykinesia, hypokinesia, rigidity, and tremor) and non-motor (depression, cognitive impairment, apathy, visual impairments, fatigue, and insomnia) symptoms, all of which negatively impact quality of life. An expanding body of research highlights the significance of physical exercise in managing Parkinson’s Disease symptoms through enhanced neuroplasticity (Johansson et al., 2020). In a comprehensive 2020 review article, Johanssen et al. conducted a meta-analysis and synthesis of exercise studies in humans with PD that looked at neuroplastic markers. They determined that various forms of physical exercise may lead to changes in a range of markers of neuroplasticity including increases in blood/serum BDNF and BDNF-TrkB signaling. Additionally, they evaluated studies that measured changes in brain structure and function, finding increased dopamine transmission, increased corticomotor excitability, weakened overactive indirect striatal pathway DA-D2R expression, and changes in gray matter volume with exercise (Mackay et al., 2017). Of note is that exercise intensity is a crucial factor in eliciting neuroplastic changes, with most studies recommending moderate-high intensity exercise interventions. The concept proposed is not merely to achieve a cardiovascular or metabolic challenge, but to engage the brain with specific, complex and coordinated activity that demands a higher degree of cortical involvement. LSVT BIG, a research-based exercise protocol developed from Lee Silverman’s LSVT LOUD speech therapy program, is a PD specific intervention designed to improve function and slow progression of motor symptoms (Fox et al., 2012; Isaacson et al., 2018; Flood et al., 2020; Fleming Walsh et al., 2022). LSVT BIG incorporates principles aligned with the literature that identifies fundamental components of exercise that enhance neuroplasticity and promote brain reorganization, including specificity, intensity, repetition, and salience of treatment. An updated variant of this program, known as Parkinson’s Wellness Recovery (PWR), could potentially provide both learning and cardiovascular benefits. Such moderate to high-intensity exercise protocols, including LSVT BIG or PWR, should be employed when treating individuals with PD to stimulate neuroplasticity and promote optimal functional recovery. For more information, refer to the 2022 clinical practice guideline by Osborne et al. published in the Journal of Physical Therapy for a comprehensive approach (Osborne et al., 2022).
Stroke
Stroke is one of the most prevalent neurological conditions worldwide, resulting in physical impairments including hemiparesis (weakness on one side of the body) and/or hemiplegia (paralysis on one side of the body). Following stroke, patients typically experience the most significant improvements in physical function within the first three months, and then progress plateaus (Jørgensen et al., 1995a,b). Despite this initial spontaneous remodeling, these changes are often insufficient in producing functional recovery (Xing and Bai, 2020). However, patients that engage in physical rehabilitation demonstrate greater improvement in functional skills compared to those who do not (Pollock et al., 2014). Effective post-stroke rehabilitation utilizes key principles of neuroplasticity to restore motor function (Dobkin and Carmichael, 2016). Constraint Induced Movement Therapy (CIMT), a series of interventions that force patients to utilize their affected limb to perform salient and repetitive tasks, can increase dendritic projections and reestablish axonal connections between hemispheres (Nesin et al., 2019). This intervention results in brain reorganization and changes in brain function (Mark et al., 2006), including expanded representation of the affected limb in the motor cortex, improving limb use for individuals with chronic stroke hemiparesis (Taub et al., 1993). Other stroke rehabilitation interventions, including aerobic exercise and general task-specific training, have identified increased production of BDNF as a key facilitator of motor learning during neuroplastic rehabilitation (Mang et al., 2013). Overall, exercise facilitates neuroplasticity post-stroke in multiple ways, including increasing synaptic plasticity, dendritic and axonal growth and function improving interhemispheric connection, promoting neural regeneration and organization, and increasing strength of surviving brain areas (Xing and Bai, 2020). Therefore, guided by evidence-based practice (Taub et al., 2002; Chen et al., 2019), clinicians can choose from an array of exercise interventions known to induce neuroplastic changes, tailoring the selection to the specific needs and circumstances of the individual recovering from stroke.
Traumatic brain injury
Traumatic brain injury (TBI) is a broad category encompassing a range of injury severities, mechanisms, and presentations. It is primarily characterized by brain damage occurring after birth, independent of congenital or developmental conditions (National Institute of Neurological Disorders and Stroke, 2022). The heterogeneity of TBI can cause a wide range of impairments, and TBI classifications (mild, moderate, severe) each present unique characteristics and therapeutic needs. Broadly speaking, almost 30% of patients experience balance issues (Basford et al., 2003) and motor functional limitations including gait abnormalities (Marshall et al., 2007) after TBI. Disrupted vestibular function is also often comorbid with TBI (Mucha et al., 2018; Marcus et al., 2019), and vestibular rehabilitation is commonly prescribed to treat vestibular dysfunction. Additionally, neuroinflammation is a significant secondary consequence following traumatic brain injury (TBI), playing a substantial role in subsequent cell death (Kumar and Loane, 2012). This inflammatory response is initiated immediately following the traumatic event and can persist for an extended period, potentially up to 17 years post-TBI (Johnson et al., 2013). While this response initially aims to repair damaged cells and protect the brain from potential pathogen invasion, excessive and persistent inflammation can have harmful effects (Wofford et al., 2019). In animal models, exercise after TBI can counteract neuroinflammation and promote neuroprotection; however, only after a period of delay after injury (Piao et al., 2013). Excessive exertion with premature exercise could interrupt the natural restorative processes triggered after TBI (Griesbach, 2011), and both animal and human studies have revealed that early initiation of exercise following TBI might inhibit neuroplasticity and deteriorate outcomes (Griesbach, 2011). Accordingly, preliminary efforts have been made to develop protocols that consider these conflicting effects of exercise on neurorecovery. For example, the Buffalo Concussion Treadmill Test was developed for sports-related concussion, which is a type of mild TBI. This assessment acts as a return-to activity assessment (Leddy and Willer, 2013), which allows clinicians to record the individuals threshold of symptom exacerbation. The results of this assessment can be used to safely prescribe a progressive aerobic exercise program (Leddy et al., 2018). Overall, if initiated in the appropriate time window, postinjury exercise can enhance functional recovery, with improvements in motor performance, spatial learning and memory tasks linked to increases in exercise-induced BDNF after TBI (Griesbach et al., 2004). Additional research evaluating specific exercise protocols targeting induction of neuroplasticity is needed to fully understand the mechanisms by which exercise may promote adaptation in individuals with mild, moderate, and severe TBI. These efforts will help inform the optimal timing, intensity, and duration of training for these individuals.
Spinal cord injury
Spinal cord injury (SCI) occurs when the spinal cord is damaged by trauma, disease, or degeneration. This can cause partial or complete loss of sensory or motor function in the arms, legs, or body (WHO, 2022). Physical exercise has been shown to have positive effects at the cellular and molecular level (Fouad and Tetzlaff, 2012), leading to the restoration of both sensory and motor function (Hutchinson et al., 2004; Sandrow-Feinberg and Houlé, 2015). For example, exercise increases synaptic plasticity after SCI by increasing the production of neurotrophic factors (Vaynman et al., 2003), which can be found in higher concentrations in spinal and muscle tissue after physical activity (Gómez-Pinilla et al., 2002; Ying et al., 2005; Côté et al., 2011). Additionally, exercise has been shown to decrease inflammation around the injury site (Sandrow-Feinberg et al., 2009). These neuroplastic effects have been linked to improved global motor function (Bilchak et al., 2021), with both treadmill (Wang et al., 2015) and bike training (Ganzer et al., 2018a) shown to increase dendritic density and total neurite length compared to sedentary controls. The clinician, however, must consider the type of spinal cord injury when prescribing neuroplastic exercise interventions. In complete SCI, there is a total loss of all motor and sensory function below the level of injury (Wilson et al., 2012). In incomplete SCI, some function remains below the primary level of injury, and individuals with this type of SCI may show faster and more significant improvements due to spared neural circuits (Little et al., 1999). Additionally, some patients with high-level SCI may not be able to tolerate the intensity of exercise required to induce neuroplastic changes due to compromised diaphragmatic function (Zimmer et al., 2007). Therefore, the type and intensity of exercise prescribed for individuals with SCI should be tailored to the individual’s specific needs and abilities.
Neuromodulation and neuroplasticity
As defined by the International Neuromodulation Society, neuromodulation is an external alteration of nerve activity through delivery of a distinct stimulus, such as a magnetic field or electric current (International Neuromodulation Society, 2023). This technique is increasingly being studied regarding its ability to modulate neuroplastic changes in the brain (Danilov and Paltin, 2018). By altering the nervous system’s electrical activity, neuromodulation can lead to changes in brain structure or function. In the following sections, we discuss three specific non-invasive neuromodulation techniques including transcranial magnetic stimulation (TMS), peripheral nerve stimulation (PNS), and vagus nerve stimulation (VNS), and their applications in neurologic diagnoses including PD, Stroke, TBI, and SCI, which are the conditions we focus on in this review. This section aims to combine this information with the previously discussed disease-specific exercise considerations, enabling a comprehensive understanding of how to optimize the combination of neuromodulation and exercise. The goal is to outline a path toward the personalization of interventions, tailoring the treatments to each patient’s unique needs.
Transcranial magnetic stimulation
Overview
Transcranial Magnetic Stimulation (TMS) is a non-invasive neuromodulation method that utilizes a strategically placed wire coil over the scalp to induce a magnetic field. This approach generates an electric current penetrating the skull, provoking neuronal depolarization in the targeted cortical region. TMS is an enticing neuromodulation strategy due to its non-surgical application, ability to focus on precise brain regions, and capacity to incite motor contractions (Figure 2). TMS serves investigative and therapeutic roles, fostering the exploration of human brain function and promoting neuroplasticity non-invasively (O’Malley et al., 2006). When employed therapeutically, the objective of TMS is to modulate neural activity in the targeted cortical region (Kesikburun, 2022), often achieved via repetitive TMS pulses (rTMS). Notably, rTMS has been deemed safe with no lasting neurological, cognitive, or cardiovascular sequelae reported (George and Aston-Jones, 2010), further bolstering its appeal in neurorehabilitation.
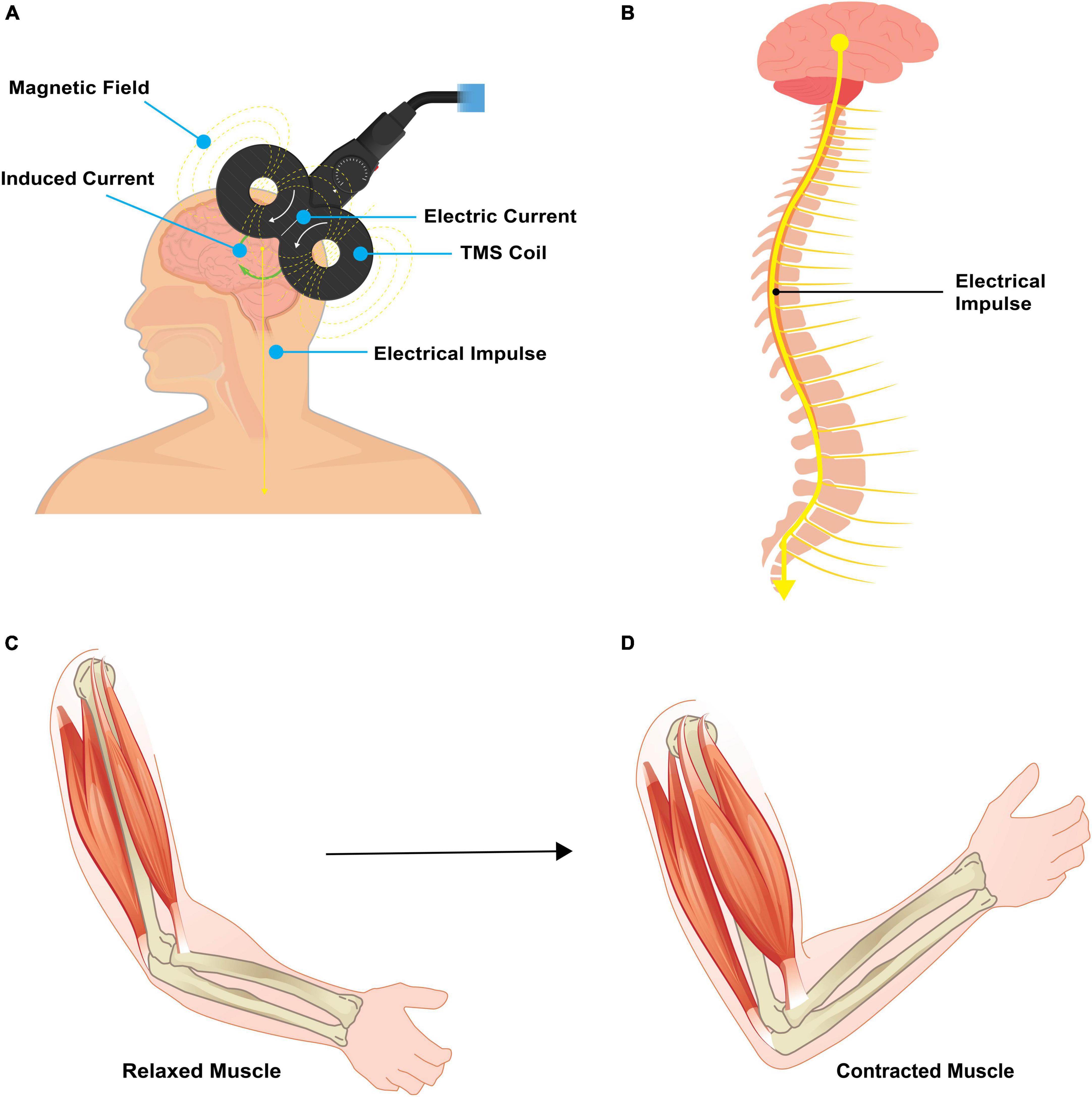
Figure 2. Pictorial representation of TMS. (A) Transcranial magnetic stimulation involves placement of a magnet coil over the scalp to induce an electric field. This electric field passes through the scalp and skull to act directly on the targeted brain area. (B) When applied to the primary motor cortex, an electrical impulse travels down the corticospinal tract and results in a targeted muscle contraction. (C,D) A relaxed muscle will contract with targeted TMS. Source: Biorender.com.
TMS affects a number of neurophysiological processes in the brain, including long term potentiation (LTP) and long term depression (LTD) (Gersner et al., 2011). LTD and LTP are long-term changes in the strength of connections between neurons, specifically at the synapse. These changes are important for learning, skill acquisition, and memory (Abraham et al., 2019). LTP is a long-term increase in the strength of a synapse, which can be triggered by high-frequency stimulation of the synapse (Purves et al., 2001), and is responsible for Hebbian learning and formation of long-term memories. LTD is a long-term decrease in the strength of a synapse, which can be triggered by repeated low-frequency stimulation of the synapse (Gonzalez et al., 2014). This decrease in synapse strength may be important for weakening or eliminating connections between neurons that are no longer needed and is therefore considered inhibitory. Evidence suggests that TMS may be able to modulate LTD and LTP in the brain (Chervyakov et al., 2015). For example, high frequency TMS can have an excitatory effect, resulting in LTP (Tian and Izumi, 2022). On the other hand, low-frequency TMS can inhibit neuronal synapses, resulting in LTD (Casula et al., 2014) (Figure 3A). Additional neuroplastic properties of rTMS include changes in cerebral blood flow (Jung et al., 2020), increased neurotransmitter levels (Strafella et al., 2001), and modification of gene expression (Hwang et al., 2022).
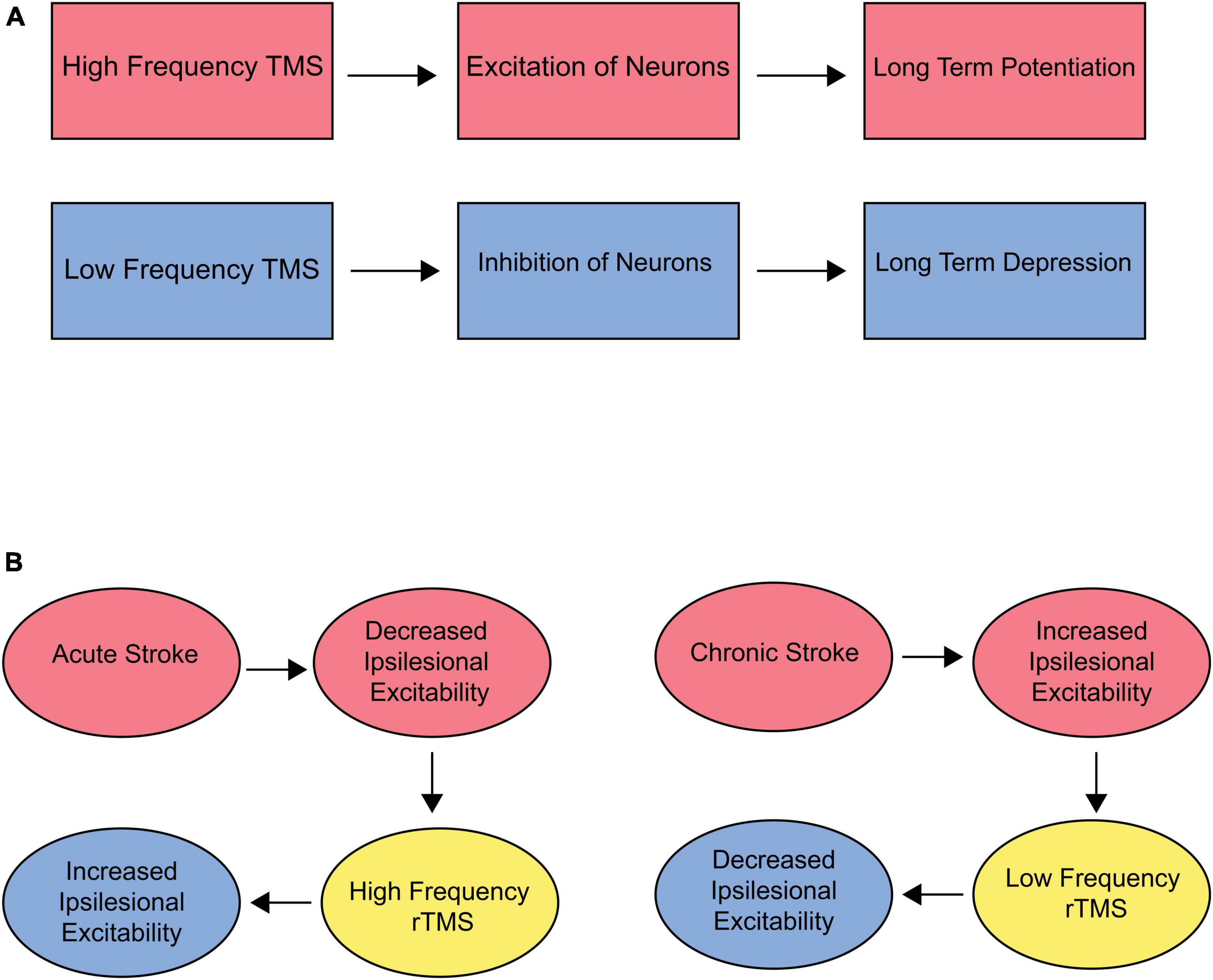
Figure 3. (A) Effect of TMS on LTP/LTD. High frequency stimulation has an overall excitatory effect on neurons, theoretically resulting in long-term potentiation (LTP). Low frequency stimulation has an inhibitory effect, resulting in long-term depression (LTD). (B) Target and frequency recommendations for acute and chronic stroke. Acute stroke is accompanied by decreased ipsilesional excitability. Therefore, high-frequency rTMS should be used over the ipsilesional M1 to optimize outcomes. Chronic stroke is associated with increased ipsilesional excitability. Therefore, low-frequency stimulation to downregulate the contralesional hemisphere could be beneficial.
TMS implications by disease
Parkinson’s disease
Transcranial Magnetic Stimulation (TMS), particularly repetitive TMS (rTMS), is increasingly seen as a potential therapeutic intervention for Parkinson’s Disease (PD). The growing body of research we highlight here mainly focuses on motor learning, to introduce promising implications for incorporating TMS into rehabilitation settings. Several studies have demonstrated how rTMS can mitigate motor symptoms in PD by targeting specific brain regions such as the M1, SMA, and dorsolateral prefrontal cortex with low or high-frequency protocols (Wagle Shukla et al., 2016; Goodwill et al., 2017; Yang et al., 2018). A seminal study from 2020 by Chung et al. showed that the benefits of treadmill training could be significantly amplified when the brain is primed with 1 and 25 Hz rTMS, resulting in sustained motor improvement up to three months post-intervention (Chung et al., 2020). This integration enhances activity-dependent plasticity primarily by stabilizing the consolidation process and adjusting cortical excitability. These findings demonstrate the value of pairing exercise and neuromodulation, specifically by priming. This research also notes the potential of rTMS to improve non-motor symptoms of PD, including cognition and depression (Sanches et al., 2020), further supporting the utility of TMS to address a variety of symptoms in a rehabilitative context.
Furthermore, a comprehensive 2018 meta-analysis by Yang et al. demonstrated the therapeutic potential of rTMS on the motor recovery in PD patients (Yang et al., 2018). They incorporated 23 studies with a total of 646 participants and found significant improvements in motor function in the short and long term. Importantly, high-frequency rTMS substantially impacted motor function improvement, while low frequency rTMS did not share the same effect. Among high-frequency rTMS interventions, multisession rTMS targeting bilateral M1 regions was found to be the most effective, showcasing the greatest effect size (Yang et al., 2018) and demonstrates the importance of personalized interventions to optimize patient outcomes.
Stroke
In a healthy brain, interhemispheric communication is vital as the two brain hemispheres constantly exchange information (Gazzaniga, 2000). This balance is disrupted by stroke, leading to a complex pattern of cortical reorganization as the brain attempts to compensate for this deficit (Casula et al., 2021). The use of repetitive Transcranial Magnetic Stimulation (rTMS) has been proven to facilitate cortical reorganization in stroke patients, both in the acute and chronic phases. Doing so helps to avert abnormal reorganization and fosters functional preservation or enhancement of motor abilities (Dionísio et al., 2018).
In the subacute stroke phase, a decline in motor cortex excitability and the cortical representation of the paretic muscles occurs (Dimyan and Cohen, 2011). Consequently, TMS has been extensively explored to externally augment the excitability of the stroke-affected (ipsilateral) M1 through high-frequency stimulation to improve function. Numerous studies focused on ipsilateral M1 stimulation have documented at least one improvement in motor function after treatment (Dimyan and Cohen, 2011). In chronic phases, the unaffected (contralateral) hemisphere shows increased cortical excitability, an adaptive mechanism to compensate for functional deficits. An alternative strategy to improve motor function on the paretic side involves the downregulation of M1 excitability in the intact hemisphere. This approach would allow more normal functioning of the ipsilateral hemisphere (Figure 3B).
Adding to this, a comprehensive 2022 systematic review and meta-analysis by Gao et al. investigated the combination of intermittent theta-burst stimulation (a method of modifying cortical excitability with repetitive TMS stimulation) with exercise. They identified thirteen randomized controlled trials (RCTs) involving 334 patients. Primary endpoints were the Fugl-Meyer Assessment Scale (FMA), a stroke-specific, performance-based impairment index, and the Motor Assessment Scale (MAS), a clinical tool for measuring task performance related to daily living activities. The analysis found significant improvement in FMA scores when iTBS was combined with physical exercise. These scores reflect on coordinated and dissociative movements. However, they concluded that the positive effect of iTBS on motor function was only evident in chronic stroke patients, not in those in the subacute phase (Gao et al., 2022). These findings highlight TMS, particularly when paired with physical exercise, as a promising intervention for stroke rehabilitation. Its value lies in its potential as a standalone therapy and its complementary role when used alongside other treatments, such as physical therapy and occupational therapy, to optimize rehabilitation outcomes.
Traumatic brain injury
Although there is limited research on Transcranial Magnetic Stimulation (TMS) following traumatic brain injury (TBI), preliminary pre-clinical and human pilot studies indicate that brain stimulation holds promise as a treatment for TBI-related motor deficits (Clayton et al., 2016). In the past, safety concerns regarding seizure susceptibility made the use of cortical stimulation in individuals with TBI somewhat controversial. However, it is now understood that the incidence of seizure relates more directly to the severity of injury rather than the stimulation itself (Dhaliwal et al., 2015), indicating that individuals with more severe brain injury are at an increased risk. In light of these findings, it is important to differentiate between mild and moderate/severe TBI in the approach to TMS. Those with mild TBI may respond differently and with decreased risk to these treatments than individuals with moderate to severe TBI; therefore, stratifying by TBI severity could enhance this technique’s therapeutic potential and safety profile.
Individuals sustaining mild TBI often report a constellation of physical, cognitive, and emotional/behavioral symptoms referred to as persistent post-concussion symptoms (Ryan and Warden, 2003). These symptoms can persist from months to years following injury (Bramlett and Dietrich, 2015), and include vertigo, dizziness, imbalance, and vision changes (Mucha et al., 2018). While the exact pathophysiology of persistent post-concussion symptoms are not entirely understood, increasing evidence suggests that disruptions in large-scale brain networks, particularly those associated with cognitive control, play a significant role (Sharp et al., 2014). Given the observed correlation between network dysfunction and mTBI symptoms (Churchill et al., 2019), neuromodulation of brain areas affected by mTBI is emerging as a promising approach for addressing persistent postconcussion symptoms (Buhagiar et al., 2020). Additionally, considering the broad cortical projections of the vestibular system as well as the interconnection between cognitive and affective networks, rTMS can be a powerful tool for influencing motion perception and postural control (Paxman et al., 2018). This opens an avenue for enhancing the treatment outcomes of balance and dizziness issues post-TBI, which are frequently managed by physical therapists. Furthermore, TMS has demonstrated promising results for postconcussive depression (Rao et al., 2019) and headache (Leung et al., 2018). The reduction of these symptoms could result in increased participation in traditional rehabilitation therapies, ultimately improving the overall effectiveness of therapy and patient outcomes.
Functional recovery following moderate to severe TBI requires interventions that vary depending on the time elapsed since the injury. In the acute phase, the focus of recovery should be neuroprotective, with the aim of minimizing neurologic damage. In contrast, during the chronic stages of TBI, the rehabilitation strategy shifts to suppressing maladaptive changes and fostering behavioral improvements (Demirtas-Tatlidede et al., 2012). TMS should be applied in a manner that complements these rehabilitation strategies, tailoring the approach to each stage of brain injury for optimal therapeutic benefit. Several experimental studies in animal models have explored the use of rTMS to enhance neuroprotection and neurorecovery after TBI with promising results (Lu et al., 2015, 2017; Verdugo-Diaz et al., 2017; Shin et al., 2018), however there are surprisingly few studies that have obtained substantial evidence regarding effects of rTMS in humans with TBI. Although rTMS has the potential to be a useful treatment for common TBI symptoms, brain injury is generally considered a contraindication to the repetitive forms of TMS due to the increased overall neural excitability and risk of seizures. This has led to TBI patients being excluded from most rTMS studies, making it challenging to accurately evaluate the safety and effectiveness of rTMS as a treatment for TBI (Rossi et al., 2009).
One standout clinical trial demonstrates the potential for rTMS to be combined with neurorehabilitation to enhance patient outcomes. This study explored the effects of rTMS paired with cognitive training on cognitive impairment in patients with traumatic brain injury (TBI) using multimodal magnetic resonance imaging (MRI) (Zhou et al., 2021). It included 166 patients with cognitive impairment post-TBI, dividing participants into two groups: one received rTMS combined with cognitive training (the observation group), while the other received cognitive training only (the control group). Results indicated that the observation group, who underwent rTMS, displayed significant improvements in various measures, such as the Glasgow Coma Scale (GCS) score, metabolic ratios examined by magnetic resonance spectroscopy imaging (MRSI), cognitive impairment score and grading, as well as the modified Barthel index. These results demonstrate the potential of rTMS as a viable adjunct to traditional rehabilitation therapy, amplifying the beneficial effects on cognitive impairment in patients post-TBI. However, while these findings are promising, particularly in cognitive rehabilitation, the use of rTMS in conjunction with motor rehabilitation in human subjects still requires further investigation.
Spinal cord injury
When considering TMS for individuals with SCI, it is important to consider the differences between the use-case for complete vs. incomplete SCI. Patients with incomplete paraplegia generally have a good prognosis in regaining locomotor ability (∼76% of patients) within a year of injury (Waters et al., 1994), whereas individuals with complete spinal cord injury experience limited recovery of lower limb function, especially if their neurologic level of injury is above T9 (Waters et al., 1992). Therefore, TMS for functional motor recovery should be considered primarily for individuals with incomplete injury. On the other hand, TMS has also been investigated for its ability to reduce spasticity, a common disorder in patients with incomplete and complete SCI. Therefore, patients with complete SCI should not be excluded when considering future research clinical applications of TMS.
rTMS has demonstrated preliminary promise as an effective therapeutic modality for the recovery of motor function after spinal cord injury. In a 2004 study, Belci et al. examined TMS’s ability to promote somatomotor functional recovery in patients after incomplete SCI. The results indicated a short-term reduction in cortical inhibition with rTMS, and a temporary decrease in the activity of inhibitory neurons in the cerebral cortex. Reducing cortical inhibition can have various effects on brain function, including improving the function of certain brain circuits involved in movement. This study also reported a lasting improvement in sensory and motor function as per the ASIA scale, a tool used to assess the severity of SCI (Belci et al., 2004).
Additionally, a few studies have demonstrated the enhanced therapeutic potential of rTMS when combined with other therapeutic interventions. In a 2019 study, Krishan et al. aimed to test if rTMS promotes plasticity and rehabilitation in a rat model of acute vs. chronic SCI. The acute-TMS group demonstrated significant improvements in locomotor performance compared to chronic and no-TMS groups, indicating that rTMS therapy beginning in the acute phase after SCI promotes neuroplastic change. In a 2022 randomized control trial, Pulverenti et al. combined TMS with spinal cord stimulation during robotic-assisted locomotor training (Pulverenti et al., 2022). Their findings suggest pairing spinal cord stimulation with brain stimulation via TMS may further augment the benefits of locomotor training vs. locomotor training alone. Kumru et al. conducted two sham-controlled randomized controlled trials, combining high-frequency TMS with supervised gait training in groups of sub-acute SCI ambulators and marginal ambulators. The trials reported that rTMS has positive effects on the recovery of gait function and lower limb strength (Krogh et al., 2022). Overall, while these studies demonstrate the potential of rTMS in SCI rehabilitation, further research is needed before integrating this technique into clinical practice. Understanding the optimal dosage and timining of rTMS in combination with other physical therapy interventions will be critical to fully harnessing the benefits of this technology in the clinical setting.
Discussion: TMS-enhanced rehabilitation
TMS shows significant promise as a neurostimulation technique to enhance neuroplasticity in various neurological conditions. By strategically integrating TMS with motor learning practices, their individual therapeutic effects could be amplified, as they demonstrate similar mechanisms for driving neuroplasticity. TMS can prime neuronal networks in the cortex when delivered prior to a task, while stimulation delivered simultaneously with the task can recruit specific sets of synapses involved in performance (Villamar et al., 2012). Based on the existing evidence, rTMS could potentially play a role in improving the effectiveness of other rehabilitation treatments (Formica et al., 2021). However, to effectively utilize TMS as a complementary therapy, the specific neurological characteristics of each condition and diagnosis must be considered. For instance, high-amplitude training programs such as LSVT BIG and PWR inherently demand large movement patterns, making it challenging to conduct TMS therapy alongside this exercise intervention. In this case, it may be more beneficial to prime the nervous system with TMS prior to administering the exercise intervention.
Conversely, upper extremity CIMT for stroke rehabilitation, which can be completed in a seated position, may be more appropriately combined simultaneously with TMS. Importantly, TMS studies need to provide more precise information about stimulation location. For instance, while TMS over the motor cortex (M1) has shown promising effects on motor learning, stimulation of other regions, such as the cerebellum, might yield different results. See Table 1 for a summary of potential clinical applications and future areas for research. Further research is needed to derive ideal parameters, timing, and application of TMS to prescribe personalized therapeutic interventions.
Peripheral nerve stimulation (PNS)
Overview
Peripheral nerve stimulation (PNS) is a type of non-invasive neuromodulation that delivers electrical stimuli to targeted peripheral nerves. PNS is traditionally used in physical therapy clinics for pain management, however with varied parameters and settings, PNS serves as a potential therapeutic modality to aid in the rehabilitation of neurological conditions. PNS techniques such as neuromuscular electrical stimulation (NMES) and functional electrical stimulation (FES) facilitate voluntary muscle contractions, and can be strategically used in the rehabilitation setting for muscle re-education (Price and Pandyan, 2000; Ada and Foongchomcheay, 2002; Ragnarsson, 2008; Sujith, 2008). NMES & FES specifically target lower motor neurons (LMNs) to produce a muscle contraction. NMES involves the application of electrical stimuli to a muscle, with the goal of improving muscle strength, reducing muscle atrophy, and facilitating motor recovery (Chipchase et al., 2011). The stimulation is used to artificially generate a muscle contraction in the absence of voluntary muscle control or to augment a weak voluntary muscle contraction. On the other hand, FES is typically used to enable functional movements or tasks. The electrical stimulation in FES is often synchronized with a specific task or movement to assist with function. Refer to Figure 4A for an overview of different types of electrical stimulation used in the clinical setting.
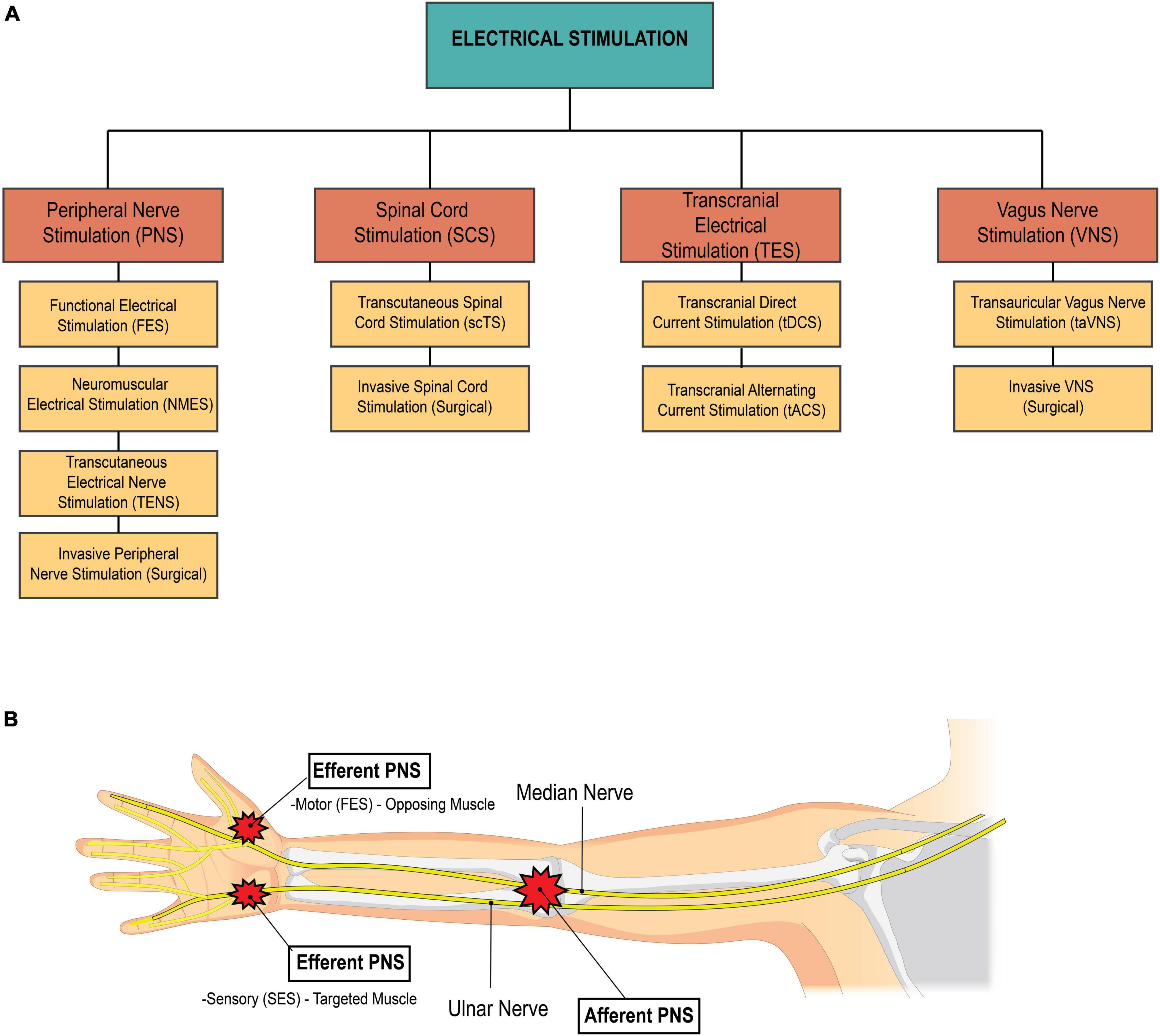
Figure 4. (A) Breakdown of types of electrical stimulation. The four main categories of electrical stimulation used in medical management of disease and injury include peripheral nerve stimulation, spinal cord stimulation, transcranial electrical stimulation, and vagus nerve stimulation. (B) Afferent vs. efferent PNS. Afferent PNS involves stimulation proximally to effect distal structures. For example, stimulation of the median and radial nerves travels down the arm to effect hand function. On the other hand, efferent PNS involves stimulation at or near the target structure. The two types of efferent PNS are sensory electrical stimulation and motor electrical stimulation. Sensory electrical stimulation (SES) is applied on the targeted muscle at intensities below the motor threshold. functional electrical stimulation (FES), a type of motor stimulation, is applied to the muscle opposing the desired action, at intensities at or above the motor threshold. Source: Biorender.com.
Importantly, these PNS techniques also promote neuroplasticity by increasing the basal level of spinal excitability, leading to improved voluntary motor function even with lower input levels (Grahn et al., 2017). Additionally, emerging research suggests that electrical stimulation techniques like NMES and FES can enhance cortical plasticity by increasing the corresponding motor and sensory cortical representation areas (Chipchase et al., 2011). Therefore, PNS can be integrated with traditional rehabilitation methods to amplify neuroplastic changes and enhance outcomes in patients with neurological conditions. Further research to explore these potentials can open new avenues for personalized interventions, enhancing patient outcomes in various neurological conditions.
PNS implication by disease
Parkinson’s disease
PD patients can exhibit a variety of motor symptoms, with resting tremors being one of the most significant. Studies have shown that 69% of patients have resting tremor at the onset of disease, and 75% develop tremor during the course of the disease (Louis and Machado, 2015). Hand tremors can significantly affect quality of life (Meng et al., 2022), and are commonly treated with oral medications or invasive surgery. However, non-invasive electrical stimulation methods have emerged as effective alternative for tremor reduction (Meng et al., 2022).
PNS techniques for tremor reduction falls into one of two categories: efferent PNS or afferent PNS. Efferent PNS involves stimulating the targeted muscle or its antagonist to reduce tremor (Figure 4B). Sensory electrical stimulation (SES), a subtype of efferent PNS, involves stimulation to the targeted muscle below motor threshold. This acts to suppress or regulate the neuronal pathway involved in tremor (Raethjen et al., 2000; Xu et al., 2016; Dideriksen et al., 2017). Conversely, functional electrical stimulation (FES), which is another form of efferent PNS, involves stimulation above the motor threshold to the antagonist, which leads to active muscle contraction to control tremor. Afferent PNS involves stimulation of the afferent nerve fibers of the radial and median nerves to inhibit muscles related to tremor (Figure 4B; Meng et al., 2022). All three of these PNS strategies have shown promise in reducing tremor and are comparable or superior to first-line pharmacotherapies. FES appears to be the most effective of these strategies (Meng et al., 2022), yielding an average rate of tremor inhibition of over 50% (Popović Maneski et al., 2011; Zhang et al., 2011; Gallego et al., 2013; Freeman et al., 2015). However, SES may be a more comfortable alternative for tremor suppression. In clinical settings, these PNS techniques could potentially be used to reduce tremor before or during exercise or functional training sessions, improving motor control and accuracy of movements and enhancing physical therapy interventions.
Stroke
PNS can be paired with functional training in individuals with stroke, enhancing neuroplasticity and improving motor function outcomes, highlighting its applicability in a rehabilitation setting (Kim et al., 2020). For example, in 2012, Ikuno et al. investigated PNS combined with task-oriented training in 22 stroke patients by combining PNS of median and ulnar nerves with task-oriented training for one week. Combined PNS and task training significantly improved hand function as compared to the group with task-only training (Ikuno et al., 2012). Fleming et al. (2015) found that an experimental group that underwent both task-oriented training and peripheral nerve stimulation (PNS) to median, ulnar, and radial nerves showed a positive effect on upper extremity (UE) function compared to a control group that received task-oriented training alone (Fleming et al., 2015). In a 2020 study, Kim et al.’s included 29 patients with hemiplegia, with 14 subjects receiving PNS + task-oriented training for 4 weeks, while 15 control group subjects underwent only task-oriented training. After 4 weeks, muscle activity of extensor carpi radialis, flexor carpi radialis, grip strength, and Action Research Arm Test were significantly higher in the experimental group (Kim et al., 2020). Collectively, these studies validate the approach of combined electrical stimulation of peripheral nerves timed with functional movements after stroke to enhance outcomes. However, an additional review by Quandt and Hummel (2014), proposes that while there are studies indicating the potential benefit of FES in stroke rehabilitation, more homonogenous randomized controlled trials are needed to generate stronger evidence supporting an advantage of FES over traditional rehabilitation approaches (Quandt and Hummel, 2014).
However, the application of PNS improving lower extremity function may be more challenging. This is reflected in a 2021 systematic review, where Cunha et al. evaluated the effectiveness of FES applied to the paretic peroneal nerve in individuals with foot drop secondary to stroke, including fourteen studies with data from 1115 participants. Their analysis revealed low-quality evidence of positive effects of FES on gait speed when combined with physical therapy (Jaqueline da Cunha et al., 2021). Therefore, further investigation and standardization of PNS protocols combined with gait training is warranted.
Traumatic brain injury
Following a traumatic brain injury, the excitability of the motor cortex near the injury site may be substantially decreased, causing a reduction in cortical map representations of the muscles that are affected (Traversa et al., 1997; Bütefisch et al., 2006). While FES and NMES have been studied extensively in stroke, less work has been done for individuals with TBI. In a 2021 case report, Milosevic et al. delivered functional electrical stimulation therapy (FEST) combined with task-specific and repeated training to an individual with chronic TBI with the assistance of a therapist. The results suggested that task-specific and repetitive FES can effectively increase cortical recruitment (Milosevic et al., 2021). In a 2010 study, 30 participants in an inpatient setting with either stroke or TBI resulting in hemiparesis received either real FES cycling or sham-FES cycling as a treatment intervention. Electrodes were applied to bilateral quadriceps, hamstrings, glutes, and tibialis anterior, 5x/week for 4 weeks. Results demonstrated improved LE strength and gait speed after FES treatment (Ambrosini et al., 2011). However, this clinical trial did not differentiate between stroke and TBI diagnosis, and results should be considered cautiously. Although FES and TENS are recommended as adjunct treatments in published clinical practice guidelines (CPGs) for treatment of TBI, further studies are warranted to determine the efficacy of PNS as a treatment (Lee et al., 2019).
PNS has also been explored as a potential treatment for muscle wasting in critically ill TBI patients during their ICU stay. In this context, NMES has been identified as a promising approach to alleviate the functional and clinical effects of muscle wasting. Silva et al. (2019) conducted a study to evaluate the effectiveness and duration of an NMES protocol on muscle architecture, neuromuscular electrophysiological disorder (NED), and muscle strength. Their findings indicate that NMES administered for fourteen consecutive days was effective in reducing muscle atrophy, decreasing the incidence of NED, and mitigating muscle weakness in critically ill TBI patients (Silva et al., 2019). While this study did not pair NMES with traditional physical or occupational therapy, it hints at the potential benefits of a more integrated approach. Specifically, in patients with acute and severe TBI, traditional therapy could be enhanced by pairing NMES with functional, low level tasks such as bed mobility and transfer training. However, integrating PNS with traditional therapeutic approaches during the acute phase of severe TBI presents several challenges. The medical instability of patients immediately after traumatic incident often precludes additional interventions such as PNS. Additionally, cognitive deficits and communication difficulties characteristic of severe TBI may hinder patients’ understanding of or cooperation with the therapy. Future research could illuminate the possible synergies between NMES and task-oriented therapy, and address the barriers of implementing this approach in this population.
Spinal cord injury
SCI causes damage to both the pathways of efferent and afferent neurons, which include the descending motor fibers from the brain to the spinal motor neurons and the ascending somatosensory fibers from the PNS through the spinal cord and back to the brain (Hamid and Hayek, 2008). Electrical stimulation in individuals with SCI is believed to work by inducing neuroplastic changes at synapses within the spinal cord (Karamian et al., 2022), and it plays a prominent and important role in rehabilitation following SCI. Studies have demonstrated that FES can enhance muscle power output and resistance (Gorgey et al., 2018), with various research supporting its effectiveness in the recovery of upper extremity function after SCI (Popovic et al., 2011). FES shows promise for both acute and chronic SCI, as indicated by an RCT in individuals with cervical incomplete SCI (Kapadia et al., 2011). FES was applied 5 days per week for 8 weeks and compared to conventional occupational therapy targeting improvement of voluntary upper limb function. Participants receiving FES therapy showed greater improvements in hand function at discharge, as well as 6-month follow-up, compared to the control group (Kapadia et al., 2011). FES cycling can improve lower extremity function as well, indicated by a 2022 review where Scheer et al. identified ninety-two FES cycling exercise intervention studies, including 999 individuals with SCI. They concluded that FES cycling substantially improved lower body muscle health in adults with SCI (van der Scheer et al., 2021).
The flexible nature of FES protocols for SCI can be adopted to either in-patient our outpatient settings, expanding its reach and application. However, barriers do exist to implementing electrical stimulation in individuals with SCI, and should be considered. Autonomic dysreflexia (AD), a potentially lifethreatening condition characterized by an exaggerated response of the autonomic nervous system that results in a sudden and significant increase in blood pressure, can be instigated by various stimuli below the level of injury. For example, AD can be triggered by bowel or bladder distension, pressure sores, or other forms of irritation such as the application of electrical stimulation. Therefore, close monitoring of the patient’s cardiovascular response during therapy is crucial to ensuring patient safety by detecting and managing any instances of AD. Despite these concerns, the potential benefits of electrical stimulation in individuals with SCI propose an avenue for further exploration and clinical application.
Additionally, transcutaneous spinal cord stimulation (tSCS) has emerged as another approach to neuromodulation to treat patients with SCI, which is distinct from peripheral nerve stimulation (PNS), but shares its non-invasive delivery method and focuses on a specific anatomical target. The technique involves the application of electrical stimulation to the skin overlying the spinal cord, allowing for indirect modulation of spinal cord activity (Field-Fote, 2004). In the case of SCI, tSCS may provide more significant functional recovery than rehabilitation alone (Tefertiller et al., 2022; Tharu et al., 2023), especially in cases of complete SCI, where the signal is obstructed from descending below the level of the injury. While some circuits are spared in patients with complete SCI, these circuits are frequently insufficient to create a satisfactory level of excitability for stimulating motor neurons below the level of injury. Electrical stimulation at the level of the spinal cord could help strengthen spared neural circuitry in facilitation of adequate stimulation of motor neurons for muscle contraction (Harkema et al., 2011). Pairing this innovative approach with traditional rehabilitation techniques could provide enhanced outcomes for patients with SCI. The continuous exploration and refinement of these methodologies are important in realizing the full potential of SCI recovery.
Discussion: PNS-enhanced rehabilitation
In summary, PNS can be an effective adjunct intervention to enhance neuroplasticity in individuals with neurologic conditions. Unlike many other neurostimulation devices, handheld and portable peripheral nerve stimulation devices are readily available in physical therapy clinics, offering an accessible and safe tool for improving patient care (Kaye et al., 2021). While most therapists are familiar with using passive TENS for pain relief after orthopedic injuries, an expanded understanding of various applications of PNS is warranted. Clinicians should consider disease-specific implications, types of electrical stimulation, timing of stimulation, and stimulation parameters to utilize PNS to enhance neuroplasticity and improve motor outcomes in individuals with neurologic diagnoses. See Table 2 for a summary of potential clinical applications and future areas for research. Despite the substantial body of evidence supporting the use of PNS in rehabilitation, further examination of PNS and its role in neurologic recovery is needed, with specific focus on the timing, underlying mechanisms, and neural targets to enhance patient outcomes. By integrating PNS with traditional rehabilitation methods, we can capitalize on new avenues for enhancing patient recovery and outcomes in various neurological diagnoses.
Vagus nerve stimulation (VNS)
Overview
Vagus Nerve Stimulation (VNS) is a neuromodulation technique that involves applying electrical stimulation to the vagus nerve, or 10th cranial nerve. Traditional VNS is delivered via a surgically implanted device, and has now been FDA approved for clinician use in treatment of migraine, cluster headache, and depression. VNS is thought to affect various brain regions through direct effect on nucleus tractus solitarius (NTS) and locus coeruleus (LC). This activation leads to rapid activation of cholinergic and noradrenergic systems, resulting in enhanced neuroplasticity associated with coincident events (Engineer et al., 2019). VNS also increases levels of brain-derived neurotropic factor (BDNF) which is a key regulator of neuroplasticity (Follesa et al., 2007). Given the anatomical distribution of the vagus nerve and its close location to the skin surface, non-invasive methods have recently been developed, with delivery sites at the neck or via stimulation of superficial projections of the vagus nerve in the outer ear. Transauricular Vagus Nerve Stimulation (taVNS) involves stimulation of the auricular branch of the vagus nerve (Wang et al., 2021) via the cymba conche (see Figure 5).
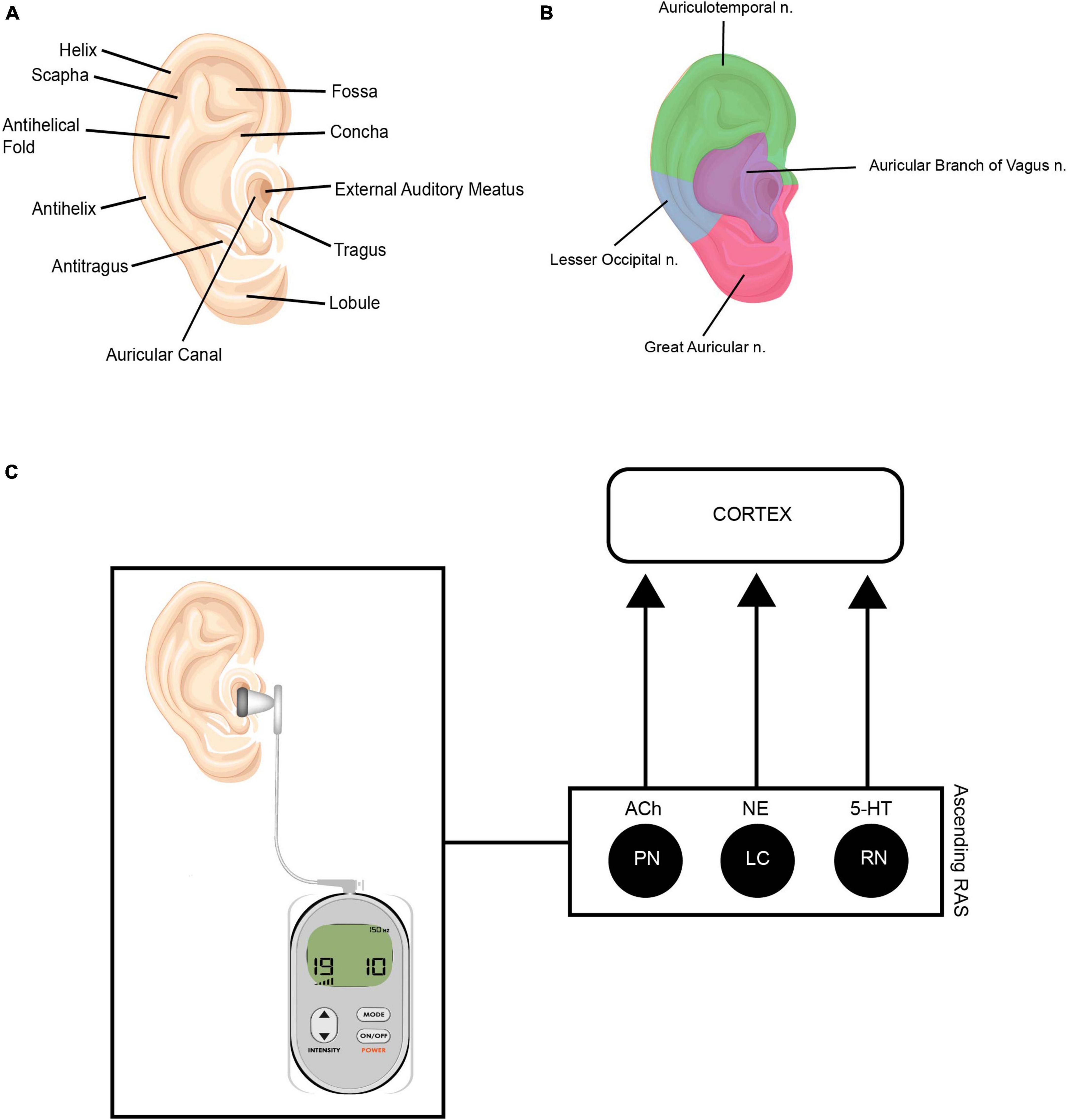
Figure 5. (A) Anatomy of the outer ear. (B) Innervation of outer ear. Illustrations depicting the auricular branch of the vagus nerve (ABVN—represented in purple) innervates the external meatus, which is the area targeted during taVNS treatment. (C) This modulates the ascending reticular activating system (RAS). The ascending RAS includes the pedunculopontine nucleus (PPN), the locus coeruleus (LC) and raphe nuclei (RN), and modulates acetylcholine (Ach), norepinephrine (NE) and serotonin (5-HT) to higher-order brain structures to modulate attention and regulate awareness, arousal and sleep. Source: Adobe Stock.
Compared to traditional VNS, non-invasive VNS is preferred to minimize the risk of infection, postoperative complications, device rejection, and patient discomfort. Additionally, taVNS is inexpensive, portable, and safe, making it an appealing device for rapid translation of VNS research into clinical practice. Considering the wide range of effects that VNS has on neural circuits, this non-invasive method is an excellent adjunct to traditional rehabilitation. By modulating the patient’s neural pathways, VNS could augment the efficacy of conventional physical and occupational therapy protocols, offering improved therapeutic outcomes.
Engineer et al. conducted an initial study to determine the effectiveness of VNS in enhancing cortical plasticity (Engineer et al., 2011). They aimed to investigate whether the presentation of tones paired with VNS could induce reorganization of the tonotopic map in the primary auditory cortex of mice. The results indicated that repeated presentation of tones without VNS did not lead to map reorganization. However, presenting the same number of tones coupled with VNS considerably increased the number of neurons that reacted to corresponding tone frequencies. This suggests that combining brief bursts of VNS with sensory or motor events can lead to potent and event-specific plasticity in neural circuits (Hays, 2016), and has formed the theoretical background for integrating VNS into personalized therapy regimens. These findings highlight the potential for VNS, when combined with specific sensory and motor stimuli during rehabilitation, to drive targeted neuroplastic changes and enhance therapeutic outcomes. As the field of personalized medicine continues to evolve, more research is needed to determine the precise timing and application of VNS to optimize the benefits of this approach.
VNS implication by disease
Parkinson’s disease
VNS has been investigated for use in the treatment of motor and non-motor symptoms of Parkinson’s Disease. While PD has traditionally been associated with the loss of dopaminergic cells in the substantia nigra, recent studies have suggested that the Locus Coeruleus Norepinephrine (LC-NE) system may also play a significant role in the pathophysiology of this condition (Rommelfanger and Weinshenker, 2007). The locus coeruleus (LC) is a brainstem structure that produces much of the brain’s norepinephrine (NE), and degradation of the neurons of the LC occurs with Parkinson’s disease (German et al., 1992; Hoogendijk et al., 1995; Marien et al., 2004). VNS stimulates the neurons of the LC, suggesting that stimulating LC output with VNS has the potential to counteract effects of LC degradation in PD (Farrand et al., 2020).
In a 2020 animal study, Farrand et al. determined that VNS results in reduced inflammation, attenuated LC and SN phenotypic neuronal loss, and enhanced motor function (Farrand et al., 2020), suggesting that stimulating LC output with VNS has the potential to modify disease progression of PD. Jiang et al. also evaluated the effects of VNS on SN-DA neurodegeneration and associated neuroinflammation & immune responses in a rat PD model, concluding that taVNS exerts neuroprotective effects against dopaminergic damage possibly by suppressing evolution of inflammation (Jiang et al., 2018). Kin et al. confirmed this again in a 2021 study, demonstrating that VNS w/0.25–0.5 mA intensity preserves dopamine neurons, reduces inflammatory glial cells, and increases noradrenergic neurons (Kin et al., 2021).
More recently, scientists have begun investigating using taVNS in a clinical setting to enhance PD rehabilitation. In 2022, Marano et al. published results from a pilot-controlled study with a double-blind randomized crossover design, where they investigated the effects of taVNS on the gait of 12 patients with idiopathic Parkinson’s Disease. Participants were randomly assigned into two groups: active taVNS and control. The results showed statistically significant changes in stride length, swing amplitude, gait speed, and gait time after taVNS (Marano et al., 2022). Further studies are warranted to determine efficacy of combining taVNS with rehabilitation to improve motor and non-motor symptoms of Parkinson’s Disease in humans.
Stroke
After stroke, the brain begins to reorganize, undergoing adaptive neuroplastic changes to compensate for the loss of previously functional neural pathways. The goal of motor rehabilitation after stroke is to facilitate positive adaptive functional changes, reinforcing the connection between the impaired brain region and corresponding extremity. Additionally, rehabilitation aims to prevent maladaptive neuroplasticity, which can lead to overreliance on the unaffected limb and learned non-use of the affected limb. Given VNS’s ability to enhance neuroplasticity, VNS has been investigated as a therapeutic approach to enhance positive neuroplasticity during stroke recovery. Khodaparest et al. explored this theory by utilizing a rat model of ischemic stroke (Khodaparast et al., 2013). The rats were initially trained on the bradykinesia assessment task, which is a quantitative measurement of forelimb movement speed, before receiving a cortical ischemic lesion (Hays et al., 2013). They were then divided into two groups for rehabilitative training, with one group receiving VNS on successful trials over 5 weeks and the other group without VNS. They found that VNS combined with rehabilitative training fully restored forelimb performance by the second week of training and significantly enhanced recovery compared to rehab training without VNS. In a follow up study, this same group found that VNS paired with rehabilitative training resulted in greater recovery of forelimb strength as compared to training without VNS. These results also suggested long-term improvement in forelimb strength, indicating persisting results after cessation of VNS therapy (Hays et al., 2014b). Hays et al. also investigated VNS paired with rehabilitative training after hemorrhagic stroke (Hays et al., 2014a), as plasticity within spared circuitry is believed to support recovery after intracerebral hemorrhage (ICH) (Auriat et al., 2010; Santos et al., 2013). They found that VNS paired with rehabilitative training resulted in significantly improved forelimb function when compared to pre lesion levels, however unlike the complete recovery observed in ischemic stroke, VNS therapy failed to fully restore forelimb function.
Based on these preliminary animal studies, Dawson et al. evaluated VNS paired with physical rehabilitation in a pilot study in stroke patients (Dawson et al., 2016). Over 6 weeks, they combined VNS with standard rehabilitative tasks. Patients who underwent this combination showed a notable improvement in the change of UE Fugl-Meyer score as compared to patients who received the same rehabilitation but without VNS. Furthermore, in 2021, this same group conducted a pivotal multi-site randomized controlled trial (VNS-REHAB), where they paired rehabilitation with active or sham vagus nerve stimulation in individuals with moderate to severe arm weakness (Dawson et al., 2021). The individuals receiving active VNS demonstrated a mean FMA-UE score improvement of 5 points, compared to 2 points in the control group. This study demonstrated that participants with moderate to severe arm impairment after ischemic stroke showed clinically meaningful improvements in motor impairment and function with paired VNS compared to rehabilitation alone. In a 2017 study, Capone et al. (2017) explored whether non-invasive VNS combined with VNS can enhance upper limb functionality in chronic ischemic and hemorrhagic stroke by delivering taVNS for 10 working days. They found that the treatment was safe and tolerable, and FMA scores were significantly better in the real group compared to sham. Wu et al. (2020) evaluated 21 subacute ischemic stroke patients, who were assigned to rehab + taVNS or rehab + sham for 15 consecutive days, finding that scores were significantly higher than before treatment in all groups, and there was a significantly greater improvement of those measures in the taVNS group compared to the sham taVNS group. More recently, Li et al. (2022) showed that taVNS combined with conventional rehab was effective in treating stroke for up to 1 year after intervention, suggesting long term retention of benefits. In a 2021 review, Morris et al. found that VNS was most effective when paired coincident with or immediately after movements during rehabilitation, with the most ideal stimulation parameters being 0.8 mA, 30 Hz, and 100 μs, providing guidelines for future taVNS + rehabilitation studies.
These studies provide the basis for a compelling argument to incorporate VNS into clinical practice to enhance standard rehabilitation. However, several barriers need to be addressed. For example, optimal stimulation parameters have not yet been determined, and there is a paucity of evidence comparing various stimulation parameters when combined with rehabilitation. Further exploration and refinement of VNS parameters are needed to maximize functional outcomes. Additionally, patient-specific variables including the nature and severity of stroke, individual neuroplastic potential, and comorbid conditions can influence the efficacy of VNS. This highlights the need for a personalized approach in the application of VNS in stroke rehabilitation.
Traumatic brain injury
Recovery of motor function after moderate to severe TBI, similar to stroke, is linked to plasticity in surviving motor circuits (Nishibe et al., 2010). Therefore, VNS therapy combined with rehabilitation has the potential to improve plasticity and aid in recovery. To investigate this hypothesis, Pruitt et al. evaluated whether VNS therapy could improve recovery of motor function in a controlled cortical impact model of severe TBI (Pruitt et al., 2016). VNS was paired with rehabilitative therapy over a period of 5 weeks, with results showing VNS paired with rehabilitative training significantly improved recovery of volitional forelimb strength compared with rehabilitative training without VNS after TBI. In addition to enhancing plasticity, Tang et al. concluded that VNS significantly ameliorated tissue damage, neurological deficits, and cerebral edema compared with a sham VNS group in animal TBI models (Tang et al., 2020).
Human studies are sparse, as patients with TBI are commonly excluded from VNS studies due to seizure risk. However, in a 2012 case control study, Englemont et al. retrospectively compared seizure outcomes after VNS therapy in patients with post-traumatic epilepsy (PTE) vs. those with no PTE. They found that after VNS therapy, patients with PTE demonstrated greater reduction in seizure frequency than patients without PTE, concluding that VNS can help reduce seizure frequency (Englot et al., 2012). Hakon et al. evaluated the feasibility of taVNS in 5 patients presenting with diffuse axonal injury one month after severe TBI, demonstrating that taVNS is a feasible and safe VNS strategy for patients following severe TBI (Hakon et al., 2020). Similarly, Noe et al. enrolled chronic adult patients with disorders of consciousness (DOC) after severe TBI, providing forty 30 min transauricular VNS sessions 2x/day, 5x/week. They concluded that taVNS could be a safe and effective tool to facilitate consciousness recovery in severely brain-injured patents (Noé et al., 2020).
These findings indicate that VNS, particularly taVNS, can be a safe and potentially effective strategy for patients following TBI. However, the body of evidence supporting the integration of this intervention into clinical practice is sparse and preliminary. More robust studies are needed to determine the most optimal way to integrate VNS with rehabilitation interventions for individuals with TBI. Potential barriers to integration include the complexity of TBI pathology and individual variability among patient presentation, making it challenging to establish a universal treatment protocol. Further research is essential to navigate barriers and identify the best strategies to combine VNS with rehabilitation for individuals with TBI.
Spinal cord injury
As previously discussed, damaged synaptic connectivity results in impaired motor function after SCI, particularly in cases of motor complete injury (Varma et al., 2013). This means that there is a disruption of neural pathways responsible for transmitting motor commands from the brain to the relevant muscles. Therefore, therapies such as VNS that enhance plasticity could potentially improve functional outcomes after SCI by reinforcing these neural pathways. Ganzer et al. (2018b) investigated this hypothesis by training rats on a reach-and-grasp task and then inducing SCI. The rats were divided into three groups who all went through physical rehabilitation. Two of the three groups also received VNS, with one group receiving stimulation after their best trials and one group receiving stimulation after their worst trials. This type of activity-dependent stimulation is called closed-loop vagus stimulation (CLV). Their findings showed that an improvement in recovery was noticeable only when the stimulation was combined with best-effort trials that were close to the intended outcome. This highlights the importance of appropriately timing the stimulation to shape the behavioral outcomes and optimize recovery. Darrow et al. also investigated closed loop VNS combined with rehabilitation training after SCI, demonstrating VNS + rehab training significantly improved recovery of volitional forelimb strength compared to equivalent rehabilitative training without VNS (Darrow et al., 2020). Surprisingly, VNS dependent enhancement of recovery was also able to be generalized to two similar (untrained) forearm tasks, which has significant implications for future clinical trials.
These findings highlight the potential of VNS as a powerful adjunctive treatment for SCI rehabilitation. They attest to the effectiveness of VNS for enhancing functional recovery, but also emphasize the need for precise timing and strategic application of this stimulation. However, further investigations are needed to refine these approaches.
Discussion: VNS enhanced rehabilitation
In summary, mounting evidence from animal models and pilot clinical trials indicates that VNS paired with rehabilitative training can enhance the benefits of neuroplastic rehabilitative interventions. More specifically, succinctly pairing VNS with successful motor trials can optimally enhance plasticity, positively shaping behavior and improving rehabilitative outcomes. See Table 3 for a summary of potential clinical applications and future areas for research.
A significant barrier to the incorporation of non-invasive VNS into clinical practice lies in the engineering challenge of synchronizing stimulation with specific patient movements. Current devices typically utilize an ear-clip, with variable stimulation sites. They lack a precise trigger mechanism for activating stimulation at the point of successful patient movement, which as discussed is a critical parameter for optimal neuroplastic enhancement. As such, we advocate for advancements in the engineering of taVNS devices, prioritizing the creation of responsive triggers that could be activated by therapists or potentially automated through the detection of successful movement parameters.
Additionally, there is a pressing need to optimize the parameters for non-invasive VNS, as current guidelines largely extrapolate from invasive VNS studies. However, it is critical to acknowledge that non-invasive and invasive VNS parameters may significantly differ due to variances in delivery methods, and should be explored independently. A comparative study between non-invasive and invasive VNS procedures would also provide valuable insights, facilitating an informed choice of technique based on efficacy, safety, patient comfort, and feasibility. An in-depth understanding of the underlying mechanisms of VNS will further highlight its clinical utility. While non-invasive VNS, particularly taVNS, holds significant potential for wider acceptance and translation into clinical practice, it is evident that substantial work lies ahead. From engineering advancements in devices to refined parameter settings and comprehensive studies into mechanisms of action, these steps are crucial to fully unlock the promise of VNS in neurorehabilitation.
Future directions in neuromodulation
In addition to the neuromodulatory approaches explored in this review, it is also worth noting other emerging technologies that show promise in rehabilitation. Transcranial Direct Current Stimulation (tDCS) and Focused Ultrasound (FUS) are among such techniques that warrant further exploration.
Transcranial direct current stimulation
Transcranial Direct Current Stimulation (tDCS) is a non-invasive brain stimulation technique that involves the delivery of a low-amplitude current between electrodes placed on the scalp. The current generates a weak electric field across the cortex, modulating neuronal activity by altering the resting membrane potentials (Datta et al., 2009; Salvador et al., 2010). This technique is portable, cost-effective, and relatively easy to administer, making it an attractive option for clinicians. tDCS has been shown to influence various cognitive processes and motor functions (Coffman et al., 2012; Parazzini et al., 2014), potentially offering a complementary approach in rehabilitation therapies.
Evidence demonstrating the clinical applicability of tDCS predominantly comes from stroke studies. In a systematic review, Marquez et al. analyzed 15 studies on the use of tDCS for stroke recovery. The methodological quality of the included studies was consistently high, and most studies reported positive effects of tDCS on motor function and impairment immediately after the intervention. However, there were limited long-term follow-up data available. They concluded that while tDCS shows promise as a therapeutic treatment for improving motor function in adults with residual motor impairments due to stroke, further research is needed to determine its long-term effectiveness (Marquez et al., 2015). Additionally, Kang et al. performed a systematic review and meta-analysis that found that tDCS can significantly improve motor learning post-stroke, measured by various motor function tests (Kang et al., 2016). However, they also acknowledge that further research is needed to determine optimal stimulation protocols and long-term effects.
The effectiveness of tDCS in enhancing motor function in Parkinson’s Disease is a matter of ongoing debate, with one review indicating beneficial outcomes (Pol et al., 2021), and another asserting that the available evidence remains inconclusive (Liu et al., 2021). Both, however, articulate the importance of further research to determine optimal tDCS parameters for functional recovery. Additionally, Kim et al. suggest that tDCS may be beneficial to patients with TBI for neuroprotection or functional recovery. However, they state that the implementation of more robust clinical trials are needed to confirm the efficacy of tDCS in this patient population and determine the most effective stimulation patterns (Kim et al., 2019). Overall, the precise mechanisms of action of tDCS are complex and multifaceted, warranting a more thorough understanding for its safe and effective implementation in clinical settings.
Focused ultrasound
FUS is another non-invasive brain stimulation method that employs the propagation of acoustic waves to modulate neuronal activity (Kim et al., 2014; Tyler et al., 2018). It is similar to traditional medical imaging and diagnostic ultrasound techniques, however it utilizes acoustic waves that are concentrated to a specific target within the brain. Because of this similarity, FUS holds the potential for easy integration into existing physical therapy methods. Low-intensity pulsed ultrasound stimulation (LIPUS) has demonstrated a neuroprotective effect after brain injury (Bretsztajn and Gedroyc, 2018). Additionally, it has been observed to increase BDNF and VEGF expression in astrocytes while inhibiting cell apoptosis (Yang et al., 2015; Su et al., 2017). This evidence points to the potential use of FUS as a novel approach for clinical stroke treatment. Preliminary preclinical evidence indicates that FUS is safe and has beneficial neuromodulatory effects on motor behavior in Parkinson’s Disease (Lee et al., 2022). However, given the safety concerns associated with the use of focused ultrasound in humans, such as potential tissue heating and damage (Pérez-Neri et al., 2022), it requires rigorous research to establish its efficacy and safety profiles.
While these technologies were outside the scope of the present review, they nonetheless present promising possibilities for the future of physical therapy. As our understanding of these techniques continues to grow, and as further research elucidates their potential benefits and risks, it is likely that they will play a more central role in the repertoire of therapeutic neuromodulation approaches available to clinicians and researchers.
Summary
In light of the evidence presented, exercise and physical activity clearly influence neuroplastic changes in individuals with various neurologic diagnoses including PD, Stroke, TBI and SCI. The potential to amplify these neuroplastic effects by combining exercise with neuromodulation is an exciting frontier in personalized rehabilitation. Until recently, the majority of studies have concentrated solely on the effects of neuromodulation, without integrating it with any specific behavioral, physical, or occupational therapy. Nevertheless, more research is emerging to unite these two therapeutic strategies to create a synergistic effect that could enhance patient outcomes (Bolognini et al., 2009). This emerging approach is founded on the hypothesis that motor learning through exercise and neuromodulation may have complementary mechanisms of action that lead to neuroplasticity in the human cortex. Concurrent employment of these adjuvant therapies could be more advantageous than using them independently (Bolognini et al., 2009).
After a comprehensive review, we have provided suggestions for future investigation of combined neuromodulation and physical rehabilitation to optimize outcomes based on the principles of neuroplasticity and the physiologic effects of various neuromodulatory mechanisms including TMS, PNS, and VNS. Additionally, we have provided a brief overview of additional neuromodulatory methods including tDCS and focused ultrasound. The overarching aim of this review is to serve as a resource for clinicians striving to combine neuromodulation with rehabilitation, as well as to encourage researchers to optimize these approaches for broad clinical application. We hope to propel advancements in personalized physical therapy, establishing initial guidelines for diagnosis-specific protocols, ultimately amplifying the effectiveness of rehabilitation outcomes. See Table 4 for a summary of potential research applications.
Author contributions
AE and WT contributed to conception and design of the review manuscript. AE wrote the first draft of the manuscript. WT and KM completed article review and provided suggested edits. All authors approved the submitted version.
Acknowledgments
We thank the following individuals for their expertise and assistance in writing the manuscript. WT, KM, Wendy Reed, and Dave Morris.
Conflict of interest
The authors declare that the research was conducted in the absence of any commercial or financial relationships that could be construed as a potential conflict of interest.
Publisher’s note
All claims expressed in this article are solely those of the authors and do not necessarily represent those of their affiliated organizations, or those of the publisher, the editors and the reviewers. Any product that may be evaluated in this article, or claim that may be made by its manufacturer, is not guaranteed or endorsed by the publisher.
References
Abraham, W., Jones, O., and Glanzman, D. (2019). Is plasticity of synapses the mechanism of long-term memory storage? NPJ Sci. Learn. 4, 1–10.
Ada, L., and Foongchomcheay, A. (2002). Efficacy of electrical stimulation in preventing or reducing subluxation of the shoulder after stroke: a meta-analysis. Aust. J. Physiother. 48, 257–267.
Ambrosini, E., Ferrante, S., Pedrocchi, A., Ferrigno, G., and Molteni, F. (2011). Cycling induced by electrical stimulation improves motor recovery in postacute hemiparetic patients: a randomized controlled trial. Stroke 42, 1068–1073.
Auriat, A., Wowk, S., and Colbourne, F. (2010). Rehabilitation after intracerebral hemorrhage in rats improves recovery with enhanced dendritic complexity but no effect on cell proliferation. Behav. Brain Res. 214, 42–47. doi: 10.1016/j.bbr.2010.04.025
Basford, J., Chou, L., Kaufman, K., Brey, R., Walker, A., Malec, J., et al. (2003). An assessment of gait and balance deficits after traumatic brain injury. Arch. Phys. Med. Rehabil. 84, 343–349.
Belci, M., Catley, M., Husain, M., Frankel, H., and Davey, N. (2004). Magnetic brain stimulation can improve clinical outcome in incomplete spinal cord injured patients. Spinal Cord 42, 417–419.
Berchtold, N., Chinn, G., Chou, M., Kesslak, J., and Cotman, C. (2005). Exercise primes a molecular memory for brain-derived neurotrophic factor protein induction in the rat hippocampus. Neuroscience 133, 853–861. doi: 10.1016/j.neuroscience.2005.03.026
Bilchak, J., Caron, G., and Côté, M. (2021). Exercise-induced plasticity in signaling pathways involved in motor recovery after spinal cord Injury. Int. J. Mol. Sci. 22:4858. doi: 10.3390/ijms22094858
Bolognini, N., Pascual-Leone, A., and Fregni, F. (2009). Using non-invasive brain stimulation to augment motor training-induced plasticity. J. Neuroeng. Rehabil. 6:8.
Booth, F., Chakravarthy, M., Gordon, S., and Spangenburg, E. (2002). Waging war on physical inactivity: using modern molecular ammunition against an ancient enemy. J. Appl. Physiol. 93, 3–30. doi: 10.1152/japplphysiol.00073.2002
Bramlett, H., and Dietrich, W. (2015). Long-Term Consequences of Traumatic Brain Injury: Current Status of Potential Mechanisms of Injury and Neurological Outcomes. J. Neurotrauma 32, 1834–1848. doi: 10.1089/neu.2014.3352
Bretsztajn, L., and Gedroyc, W. (2018). Brain-focussed ultrasound: what’s the “FUS” all about? A review of current and emerging neurological applications. Br. J. Radiol. 91:20170481. doi: 10.1259/bjr.20170481
Buhagiar, F., Fitzgerald, M., Bell, J., Allanson, F., and Pestell, C. (2020). Neuromodulation for mild traumatic brain injury rehabilitation: A Systematic Review. Front. Hum. Neurosci. 14:598208. doi: 10.3389/fnhum.2020.598208
Bütefisch, C., Kleiser, R., and Seitz, R. (2006). Post-lesional cerebral reorganisation: evidence from functional neuroimaging and transcranial magnetic stimulation. J. Physiol. 99, 437–454.
Canning, C., Shepherd, R., Carr, J., Alison, J., Wade, L., and White, A. (2003). A randomized controlled trial of the effects of intensive sit-to-stand training after recent traumatic brain injury on sit-tostand performance. Clin. Rehabil. 17, 355–362. doi: 10.1191/0269215503cr620oa
Capone, F., Miccinilli, S., Pellegrino, G., Zollo, L., Simonetti, D., Bressi, F., et al. (2017). Transcutaneous Vagus Nerve Stimulation Combined with Robotic Rehabilitation Improves Upper Limb Function after Stroke. Neural Plast. 2017:7876507.
Carro, E., Trejo, J., Busiguina, S., and Torres-Aleman, I. (2001). Circulating Insulin-Like Growth Factor I Mediates the Protective Effects of Physical Exercise against Brain Insults of Different Etiology and Anatomy. J. Neurosci. 21, 5678–5684. doi: 10.1523/JNEUROSCI.21-15-05678.2001
Casula, E., Pellicciari, M., Bonnì, S., Spanò, B., Ponzo, V., Salsano, I., et al. (2021). Evidence for interhemispheric imbalance in stroke patients as revealed by combining transcranial magnetic stimulation and electroencephalography. Hum. Brain Mapp. 42, 1343–1358. doi: 10.1002/hbm.25297
Casula, E., Tarantino, V., Basso, D., Arcara, G., Marino, G., Toffolo, G., et al. (2014). Low-frequency rTMS inhibitory effects in the primary motor cortex: Insights from TMS-evoked potentials. NeuroImage 98, 225–232. doi: 10.1016/j.neuroimage.2014.04.065
Chen, K., Zheng, Y., Wei, J., Ouyang, H., Huang, X., and Zhang, F. (2019). Exercise training improves motor skill learning via selective activation of mTOR. Sci. Adv. 5, eaaw1888. doi: 10.1126/sciadv.aaw1888
Chervyakov, A., Chernyavsky, A., Sinitsyn, D., and Piradov, M. (2015). Possible Mechanisms Underlying the Therapeutic Effects of Transcranial Magnetic Stimulation. Front. Hum. Neurosci. 9:303. doi: 10.3389/fnhum.2015.00303
Chipchase, L., Schabrun, S., and Hodges, P. (2011). Peripheral electrical stimulation to induce cortical plasticity: A systematic review of stimulus parameters. Clin. Neurophysiol. 122, 456–463. doi: 10.1016/j.clinph.2010.07.025
Chung, C., Mak, M., and Hallett, M. (2020). Transcranial Magnetic Stimulation Promotes Gait Training in Parkinson Disease. Ann. Neurol. 88, 933–945. doi: 10.1002/ana.25881
Churchill, N., Hutchison, M., Graham, S., and Schweizer, T. (2019). Mapping brain recovery after concussion: From acute injury to 1 year after medical clearance. Neurology 93, e1980–e1992.
Clayton, E., Kinley-Cooper, S., Weber, R., and Adkins, D. (2016). Brain stimulation: Neuromodulation as a potential treatment for motor recovery following traumatic brain injury. Brain Res. 1640, 130–138.
Coffman, B., Trumbo, M., Flores, R., Garcia, C., van der Merwe, A., Wassermann, E., et al. (2012). Impact of tDCS on performance and learning of target detection: interaction with stimulus characteristics and experimental design. Neuropsychologia 50, 1594–1602. doi: 10.1016/j.neuropsychologia.2012.03.012
Côté, M., Azzam, G., Lemay, M., Zhukareva, V., and Houlé, J. (2011). Activity-Dependent Increase in Neurotrophic Factors Is Associated with an Enhanced Modulation of Spinal Reflexes after Spinal Cord Injury. J. Neurotrauma 28, 299–309. doi: 10.1089/neu.2010.1594
Cotman, C., Berchtold, N., and Christie, L. (2007). Exercise builds brain health: key roles of growth factor cascades and inflammation. Trends Neurosci. 30, 464–472. doi: 10.1016/j.tins.2007.06.011
Danilov, Y., and Paltin, D. (2018). Translingual Neurostimulation (TLNS): Perspective on a Novel Approach to Neurorehabilitation after Brain Injury. Neuromethods 2018, 307–327.
Darrow, M., Torres, M., Sosa, M., Danaphongse, T., Haider, Z., Rennaker, R., et al. (2020). Vagus Nerve Stimulation Paired With Rehabilitative Training Enhances Motor Recovery After Bilateral Spinal Cord Injury to Cervical Forelimb Motor Pools. Neurorehabil. Neural. Repair. 34, 200–209. doi: 10.1177/1545968319895480
Datta, A., Bansal, V., Diaz, J., Patel, J., Reato, D., and Bikson, M. (2009). Gyri-precise head model of transcranial direct current stimulation: improved spatial focality using a ring electrode versus conventional rectangular pad. Brain Stimulat. 2, 201–207.e1. doi: 10.1016/j.brs.2009.03.005
Dawson, J., Liu, C., Francisco, G., Cramer, S., Wolf, S., Dixit, A., et al. (2021). Vagus nerve stimulation paired with rehabilitation for upper limb motor function after ischaemic stroke (VNS-REHAB): a randomised, blinded, pivotal, device trial. Lancet 397, 1545–1553.
Dawson, J., Pierce, D., Dixit, A., Kimberley, T., Robertson, M., Tarver, B., et al. (2016). Safety, Feasibility, and Efficacy of Vagus Nerve Stimulation Paired With Upper-Limb Rehabilitation After Ischemic Stroke. Stroke 47, 143–150.
Demirtas-Tatlidede, A., Vahabzadeh-Hagh, A., Bernabeu, M., Tormos, J., and Pascual-Leone, A. (2012). Noninvasive brain stimulation in traumatic brain injury. J. Head Trauma Rehabil. 27, 274–292.
Dhaliwal, S., Meek, B., and Modirrousta, M. (2015). Non-Invasive Brain Stimulation for the Treatment of Symptoms Following Traumatic Brain Injury. Front. Psychiatry 6:119. doi: 10.3389/fpsyt.2015.00119
Dideriksen, J., Laine, C., Dosen, S., Muceli, S., Rocon, E., Pons, J., et al. (2017). Electrical Stimulation of Afferent Pathways for the Suppression of Pathological Tremor. Front. Neurosci. 11:178. doi: 10.3389/fnins.2017.00178
Dimyan, M., and Cohen, L. (2011). Neuroplasticity in the context of motor rehabilitation after stroke. Nat. Rev. Neurol. 7, 76–85.
Ding, Q., Vaynman, S., Akhavan, M., Ying, Z., and Gomez-Pinilla, F. (2006). Insulin-like growth factor I interfaces with brain-derived neurotrophic factor-mediated synaptic plasticity to modulate aspects of exercise-induced cognitive function. Neuroscience 140, 823–833. doi: 10.1016/j.neuroscience.2006.02.084
Dionísio, A., Duarte, I., Patrício, M., and Castelo-Branco, M. (2018). The Use of Repetitive Transcranial Magnetic Stimulation for Stroke Rehabilitation: A Systematic Review. J. Stroke Cerebrovasc. Dis. 27, 1–31.
Dobkin, B., and Carmichael, S. (2016). The Specific Requirements of Neural Repair Trials for Stroke. Neurorehabil. Neural Repair. 30, 470–478.
El-Sayes, J., Harasym, D., Turco, C., Locke, M., and Nelson, A. (2019). Exercise-Induced Neuroplasticity: A Mechanistic Model and Prospects for Promoting Plasticity. Neuroscientist 25, 65–85. doi: 10.1177/1073858418771538
Engineer, N., Kimberley, T., Prudente, C., Dawson, J., Tarver, W., and Hays, S. (2019). Targeted Vagus Nerve Stimulation for Rehabilitation After Stroke. Front. Neurosci. 13:280. doi: 10.3389/fnins.2019.00280
Engineer, N., Riley, J., Seale, J., Vrana, W., Shetake, J., Sudanagunta, S., et al. (2011). Reversing pathological neural activity using targeted plasticity. Nature 470, 101–104.
Englot, D., Rolston, J., Wang, D., Hassnain, K., Gordon, C., and Chang, E. (2012). Efficacy of vagus nerve stimulation in posttraumatic versus nontraumatic epilepsy. J. Neurosurg. 117, 970–977. doi: 10.3171/2012.8.JNS122
Fabel, K., Fabel, K., Tam, B., Kaufer, D., Baiker, A., Simmons, N., et al. (2003). VEGF is necessary for exercise-induced adult hippocampal neurogenesis. Eur. J. Neurosci. 18, 2803–2812.
Farrand, A., Verner, R., McGuire, R., Helke, K., Hinson, V., and Boger, H. (2020). Differential effects of vagus nerve stimulation paradigms guide clinical development for Parkinson’s disease. Brain Stimulat. 13, 1323–1332. doi: 10.1016/j.brs.2020.06.078
Field-Fote, E. (2004). Electrical Stimulation Modifies Spinal and Cortical Neural Circuitry. Exerc. Sport Sci. Rev. 32:155.
Fleming, M., Sorinola, I., Roberts-Lewis, S., Wolfe, C., Wellwood, I., and Newham, D. (2015). The effect of combined somatosensory stimulation and task-specific training on upper limb function in chronic stroke: a double-blind randomized controlled trial. Neurorehabil. Neural Repair. 29, 143–152. doi: 10.1177/1545968314533613
Fleming Walsh, S., Balster, C., Chandler, A., Brown, J., Boehler, M., and O’Rear, S. (2022). LSVT BIG® and long-term retention of functional gains in individuals with Parkinson’s disease. Physiother. Theory Pract. 38, 629–636. doi: 10.1080/09593985.2020.1780655
Flood, M., O’Callaghan, B., Diamond, P., Liegey, J., Hughes, G., and Lowery, M. (2020). Quantitative clinical assessment of motor function during and following LSVT-BIG® therapy. J. Neuroeng. Rehabil. 17:92. doi: 10.1186/s12984-020-00729-8
Follesa, P., Biggio, F., Gorini, G., Caria, S., Talani, G., Dazzi, L., et al. (2007). Vagus nerve stimulation increases norepinephrine concentration and the gene expression of BDNF and bFGF in the rat brain. Brain Res. 1179, 28–34. doi: 10.1016/j.brainres.2007.08.045
Formica, C., De Salvo, S., Corallo, F., Alagna, A., Logiudice, A., Todaro, A., et al. (2021). Role of neurorehabilitative treatment using transcranial magnetic stimulation in disorders of consciousness. J. Int. Med. Res. 49:300060520976472.
Fouad, K., and Tetzlaff, W. (2012). Rehabilitative training and plasticity following spinal cord injury. Exp. Neurol. 235, 91–99.
Fox, C., Ebersbach, G., Ramig, L., and Sapir, S. (2012). LSVT LOUD and LSVT B: Behavioral Treatment Programs for Speech and Body Movement in Parkinson Disease. Park Dis. 2012:391946. doi: 10.1155/2012/391946
Freeman, C., Sampson, P., Burridge, J., and Hughes, A. (2015). Repetitive control of functional electrical stimulation for induced tremor suppression. Mechatronics 32, 79–87.
Gallego, J., Rocon, E., Belda-Lois, J., and Pons, J. (2013). A neuroprosthesis for tremor management through the control of muscle co-contraction. J. Neuroeng. Rehabil. 10:36. doi: 10.1186/1743-0003-10-36
Ganzer, P., Beringer, C., Shumsky, J., Nwaobasi, C., and Moxon, K. (2018a). Serotonin receptor and dendritic plasticity in the spinal cord mediated by chronic serotonergic pharmacotherapy combined with exercise following complete SCI in the adult rat. Exp. Neurol. 304, 132–142. doi: 10.1016/j.expneurol.2018.03.006
Ganzer, P., Darrow, M., Meyers, E., Solorzano, B., Ruiz, A., Robertson, N., et al. (2018b). Closedloop neuromodulation restores network connectivity and motor control after spinal cord injury. eLife 7, e32058.
Gao, B., Wang, Y., Zhang, D., Wang, Z., and Wang, Z. (2022). Intermittent theta-burst stimulation with physical exercise improves poststroke motor function: A systemic review and meta-analysis. Front. Neurol. 13:964627. doi: 10.3389/fneur.2022.964627
Gazzaniga, M. (2000). Cerebral specialization and interhemispheric communication: Does the corpus callosum enable the human condition? Brain 123, 1293–1326. doi: 10.1093/brain/123.7.1293
George, M., and Aston-Jones, G. (2010). Noninvasive techniques for probing neurocircuitry and treating illness: vagus nerve stimulation (VNS), transcranial magnetic stimulation (TMS) and transcranial direct current stimulation (tDCS). Neuropsychopharmacology 35, 301–316. doi: 10.1038/npp.2009.87
German, D., Manaye, K., White, C., Woodward, D., McIntire, D., Smith, W., et al. (1992). Diseasespecific patterns of locus coeruleus cell loss. Ann. Neurol. 32, 667–676.
Gersner, R., Kravetz, E., Feil, J., Pell, G., and Zangen, A. (2011). Long-Term Effects of Repetitive Transcranial Magnetic Stimulation on Markers for Neuroplasticity: Differential Outcomes in Anesthetized and Awake Animals. J. Neurosci. 31, 7521–7526. doi: 10.1523/JNEUROSCI.6751-10.2011
Gómez-Pinilla, F., Ying, Z., Roy, R., Molteni, R., and Edgerton, V. (2002). Voluntary Exercise Induces a BDNF-Mediated Mechanism That Promotes Neuroplasticity. J. Neurophysiol. 88, 2187–2195. doi: 10.1152/jn.00152.2002
Gonzalez, J., Morales, I., Villarreal, D., and Derrick, B. (2014). Low-frequency stimulation induces longterm depression and slow onset long-term potentiation at perforant path-dentate gyrus synapses in vivo. J. Neurophysiol. 111, 1259–1273. doi: 10.1152/jn.00941.2012
Goodwill, A., Lum, J., Hendy, A., Muthalib, M., Johnson, L., Albein-Urios, N., et al. (2017). Using non-invasive transcranial stimulation to improve motor and cognitive function in Parkinson’s disease: a systematic review and meta-analysis. Sci. Rep. 7:14840.
Gorgey, A., Khalil, R., Lester, R., Dudley, G., and Gater, D. (2018). Paradigms of Lower Extremity Electrical Stimulation Training After Spinal Cord Injury. J. Vis. Exp. 132:57000.
Grahn, P., Lavrov, I., Sayenko, D., Van Straaten, M., Gill, M., Strommen, J., et al. (2017). Enabling Task-Specific Volitional Motor Functions via Spinal Cord Neuromodulation in a Human With Paraplegia. Mayo Clin. Proc. 92, 544–554. doi: 10.1016/j.mayocp.2017.02.014
Griesbach, G. (2011). Exercise After Traumatic Brain Injury: Is it a Double-Edged Sword? PM&R 3, S64–S72. doi: 10.1016/j.pmrj.2011.02.008
Griesbach, G., Hovda, D., Molteni, R., Wu, A., and Gomez-Pinilla, F. (2004). Voluntary exercise following traumatic brain injury: brain-derived neurotrophic factor upregulation and recovery of function. Neuroscience 125, 129–139. doi: 10.1016/j.neuroscience.2004.01.030
Guo, W., Nagappan, G., and Lu, B. (2018). Differential effects of transient and sustained activation of BDNFTrkB signaling. Dev. Neurobiol. 78, 647–659.
Hakon, J., Moghiseh, M., Poulsen, I., Øland, C., Hansen, C., and Sabers, A. (2020). Transcutaneous Vagus Nerve Stimulation in Patients With Severe Traumatic Brain Injury: A Feasibility Trial. Neuromodul. J. Int. Neuromodul. Soc. 23, 859–864. doi: 10.1111/ner.13148
Hamid, S., and Hayek, R. (2008). Role of electrical stimulation for rehabilitation and regeneration after spinal cord injury: an overview. Eur. Spine J. 17, 1256–1269.
Hamilton, G., and Rhodes, J. (2015). Exercise Regulation of Cognitive Function and Neuroplasticity in the Healthy and Diseased Brain. Prog. Mol. Biol. Transl. Sci. 135, 381–406.
Harkema, S., Gerasimenko, Y., Hodes, J., Burdick, J., Angeli, C., Chen, Y., et al. (2011). Effect of epidural stimulation of the lumbosacral spinal cord on voluntary movement, standing, and assisted stepping after motor complete paraplegia: a case study. Lancet 377, 1938–1947. doi: 10.1016/S0140-6736(11)60547-3
Hays, S. (2016). Enhancing Rehabilitative Therapies with Vagus Nerve Stimulation. Neurother. J. Am. Soc. Exp. Neurother. 13, 382–394.
Hays, S., Khodaparast, N., Ruiz, A., Sloan, A., Hulsey, D., Rennaker, R., et al. (2014b). The timing and amount of vagus nerve stimulation during rehabilitative training affect poststroke recovery of forelimb strength. Neuroreport 25, 676–682. doi: 10.1097/WNR.0000000000000154
Hays, S., Khodaparast, N., Hulsey, D., Ruiz, A., Sloan, A., Rennaker, R., et al. (2014a). Vagus nerve stimulation during rehabilitative training improves functional recovery after intracerebral hemorrhage. Stroke 45, 3097–3100.
Hays, S., Khodaparast, N., Sloan, A., Fayyaz, T., Hulsey, D., Ruiz, A., et al. (2013). The bradykinesia assessment task: An automated method to measure forelimb speed in rodents. J. Neurosci. Methods 214, 52–61. doi: 10.1016/j.jneumeth.2012.12.022
Hoogendijk, W., Pool, C., Troost, D., van Zwieten, E., and Swaab, D. (1995). Image analyser-assisted morphometry of the locus coeruleus in Alzheimer’s disease. Parkinson’s disease and amyotrophic lateral sclerosis. Brain J. Neurol. 118, 131–143. doi: 10.1093/brain/118.1.131
Hutchinson, K., Gómez-Pinilla, F., Crowe, M., Ying, Z., and Basso, D. (2004). Three exercise paradigms differentially improve sensory recovery after spinal cord contusion in rats. Brain 127, 1403–1414. doi: 10.1093/brain/awh160
Hwang, W., Choi, J., Bang, M., Park, W., and Oh, B. (2022). Gene Expression Profile Changes in the Stimulated Rat Brain Cortex After Repetitive Transcranial Magnetic Stimulation. Brain Neurorehabil. 15, e27. doi: 10.12786/bn.2022.15.e27
Ikuno, K., Kawaguchi, S., Kitabeppu, S., Kitaura, M., Tokuhisa, K., Morimoto, S., et al. (2012). Effects of peripheral sensory nerve stimulation plus task-oriented training on upper extremity function in patients with subacute stroke: a pilot randomized crossover trial. Clin. Rehabil. 26, 999–1009. doi: 10.1177/0269215512441476
International Neuromodulation Society (2023). About Neuromodulation. San Francisco, CA: International Neuromodulation Society.
Intlekofer, K., Berchtold, N., Malvaez, M., Carlos, A., McQuown, S., Cunningham, M., et al. (2013). Exercise and sodium butyrate transform a subthreshold learning event into long-term memory via a brain-derived neurotrophic factor-dependent mechanism. Neuropsychopharmacology 38, 2027–2034. doi: 10.1038/npp.2013.104
Isaacson, S., O’Brien, A., Lazaro, J., Ray, A., and Fluet, G. (2018). The JFK BIG study: the impact of LSVT BIG® on dual task walking and mobility in persons with Parkinson’s disease. J. Phys. Ther. Sci. 30, 636–641. doi: 10.1589/jpts.30.636
Jaqueline da Cunha, M., Rech, K., Salazar, A., and Pagnussat, A. (2021). Functional electrical stimulation of the peroneal nerve improves post-stroke gait speed when combined with physiotherapy. A systematic review and meta-analysis. Ann. Phys. Rehabil. Med. 64:101388. doi: 10.1016/j.rehab.2020.03.012
Jiang, Y., Cao, Z., Ma, H., Wang, G., Wang, X., Wang, Z., et al. (2018). Auricular Vagus Nerve Stimulation Exerts Antiinflammatory Effects and Immune Regulatory Function in a 6-OHDA Model of Parkinson’s Disease. Neurochem. Res. 43, 2155–2164. doi: 10.1007/s11064-018-2639-z
Johansson, H., Hagströmer, M., Grooten, W., and Franzén, E. (2020). Exercise-Induced Neuroplasticity in Parkinson’s Disease: A Metasynthesis of the Literature. Neural. Plast. 2020, 1–15. doi: 10.1155/2020/8961493
Johnson, V., Stewart, J., Begbie, F., Trojanowski, J., Smith, D., and Stewart, W. (2013). Inflammation and white matter degeneration persist for years after a single traumatic brain injury. Brain J. Neurol. 136, 28–42. doi: 10.1093/brain/aws322
Jørgensen, H., Nakayama, H., Raaschou, H., Vive-Larsen, J., Støier, M., and Olsen, T. (1995a). Outcome and time course of recovery in stroke. Part I: Outcome. The Copenhagen Stroke Study. Arch. Phys. Med. Rehabil. 76, 399–405.
Jørgensen, H., Nakayama, H., Raaschou, H., Vive-Larsen, J., Støier, M., and Olsen, T. (1995b). Outcome and time course of recovery in stroke. Part II: Time course of recovery. The Copenhagen Stroke Study. Arch. Phys. Med. Rehabil. 76, 406–412. doi: 10.1016/s0003-9993(95)80568-0
Jung, J., Bungert, A., Bowtell, R., and Jackson, S. (2020). Modulating Brain Networks With Transcranial Magnetic Stimulation Over the Primary Motor Cortex: A Concurrent TMS/fMRI Study. Front. Hum. Neurosci. 14:31. doi: 10.3389/fnhum.2020.00031
Kang, N., Summers, J., and Cauraugh, J. (2016). Transcranial direct current stimulation facilitates motor learning post-stroke: a systematic review and meta-analysis. J. Neurol. Neurosurg. Psychiatry 87, 345–355. doi: 10.1136/jnnp-2015-311242
Kapadia, N., Bagher, S., and Popovic, M. (2014). Influence of different rehabilitation therapy models on patient outcomes: hand function therapy in individuals with incomplete SCI. J. Spinal Cord. Med. 37, 734–743. doi: 10.1179/2045772314Y.0000000203
Kapadia, N., Zivanovic, V., Furlan, J., Craven, B., McGillivray, C., and Popovic, M. (2011). Functional Electrical Stimulation Therapy for Grasping in Traumatic Incomplete Spinal Cord Injury: Randomized Control Trial. Artif. Organs. 35, 212–216. doi: 10.1111/j.1525-1594.2011.01216.x
Karamian, B., Siegel, N., Nourie, B., Serruya, M., Heary, R., Harrop, J., et al. (2022). The role of electrical stimulation for rehabilitation and regeneration after spinal cord injury. J. Orthop. Traumatol. 23:2.
Kaye, A., Ridgell, S., Alpaugh, E., Mouhaffel, A., Kaye, A., Cornett, E., et al. (2021). Peripheral Nerve Stimulation: A Review of Techniques and Clinical Efficacy. Pain Ther. 10, 961–972.
Kesikburun, S. (2022). Non-invasive brain stimulation in rehabilitation. Turk. J. Phys. Med. Rehabil. 68, 1–8.
Khodaparast, N., Hays, S., Sloan, A., Hulsey, D., Ruiz, A., Pantoja, M., et al. (2013). Vagus nerve stimulation during rehabilitative training improves forelimb strength following ischemic stroke. Neurobiol. Dis. 60, 80–88.
Kim, H., Chiu, A., Lee, S., Fischer, K., and Yoo, S. (2014). Focused Ultrasound-mediated Non-invasive Brain Stimulation: Examination of Sonication Parameters. Brain Stimulat. 7, 748–756. doi: 10.1016/j.brs.2014.06.011
Kim, S., Won, K., and Jung, E. (2020). The Effects of Simultaneous Application of Peripheral Nerve Sensory Stimulation and Task-Oriented Training to Improve Upper Extremity Motor Function After Stroke: Single Blinded Randomized Controlled Trial. Ther. Sci. Rehabil. 9, 7–20.
Kim, W., Lee, K., Kim, S., Cho, S., and Paik, N. (2019). Transcranial direct current stimulation for the treatment of motor impairment following traumatic brain injury. J. Neuroeng. Rehabil. 16:14.
Kin, I., Sasaki, T., Yasuhara, T., Kameda, M., Agari, T., Okazaki, M., et al. (2021). Vagus Nerve Stimulation with Mild Stimulation Intensity Exerts Anti-Inflammatory and Neuroprotective Effects in Parkinson’s Disease Model Rats. Biomedicines 9:789. doi: 10.3390/biomedicines9070789
Krogh, S., Aagaard, P., Jønsson, A., Figlewski, K., and Kasch, H. (2022). Effects of repetitive transcranial magnetic stimulation on recovery in lower limb muscle strength and gait function following spinal cord injury: a randomized controlled trial. Spinal Cord 60, 135–141. doi: 10.1038/s41393-021-00703-8
Kumar, A., and Loane, D. (2012). Neuroinflammation after traumatic brain injury: opportunities for therapeutic intervention. Brain Behav. Immun. 26, 1191–1201.
Leddy, J., Hinds, A., Miecznikowski, J., Darling, S., Matuszak, J., Baker, J., et al. (2018). Safety and Prognostic Utility of Provocative Exercise Testing in Acutely Concussed Adolescents: A Randomized Trial. Clin. J. Sport Med. 28:13. doi: 10.1097/JSM.0000000000000431
Leddy, J., and Willer, B. (2013). Use of Graded Exercise Testing in Concussion and Return-to-Activity Management. Curr. Sports Med. Rep. 12:370.
Lee, K., Clennell, B., Steward, T., Gialeli, A., Cordero-Llana, O., and Whitcomb, D. (2022). Focused Ultrasound Stimulation as a Neuromodulatory Tool for Parkinson’s Disease: A Scoping Review. Brain Sci. 12:289. doi: 10.3390/brainsci12020289
Lee, S., Amatya, B., Judson, R., Truesdale, M., Reinhardt, J., Uddin, T., et al. (2019). Clinical practice guidelines for rehabilitation in traumatic brain injury: a critical appraisal. Brain INJ 33, 1263–1271.
Leung, A., Metzger-Smith, V., He, Y., Cordero, J., Ehlert, B., Song, D., et al. (2018). Left Dorsolateral Prefrontal Cortex rTMS in Alleviating MTBI Related Headaches and Depressive Symptoms. Neuromodul. J. Int. Neuromodul. Soc. 21, 390–401.
Li, J., Xie, C., Li, C., Zhang, G., Tang, H., Jin, C., et al. (2022). Efficacy and safety of transcutaneous auricular vagus nerve stimulation combined with conventional rehabilitation training in acute stroke patients: a randomized controlled trial conducted for 1 year involving 60 patients. Neural Regen. Res. 17, 1809–1813. doi: 10.4103/1673-5374.332155
Little, J., Ditunno, J., Stiens, S., and Harris, R. (1999). Incomplete spinal cord injury: Neuronal mechanisms of motor recovery and hyperreflexia. Arch. Phys. Med. Rehabil. 80, 587–599.
Liu, X., Liu, H., Liu, Z., Rao, J., Wang, J., Wang, P., et al. (2021). Transcranial Direct Current Stimulation for Parkinson’s Disease: A Systematic Review and Meta-Analysis. Front. Aging Neurosci. 13:746797. doi: 10.3389/fnagi.2021.746797
Lopez-Lopez, C., LeRoith, D., and Torres-Aleman, I. (2004). Insulin-like growth factor I is required for vessel modeling in the adult brain. Proc. Natl. Acad. Sci. U. S. A. 101, 9833–9838.
Louis, E. D., and Machado, D. (2015). Tremor-related quality of life: A comparison of essential tremor vs. Parkinson’s disease patients. Parkinson. Relat. Disord. 21, 729–735. doi: 10.1016/j.parkreldis.2015.04.019
Lu, H., Kobilo, T., Robertson, C., Tong, S., Celnik, P., and Pelled, G. (2015). Transcranial magnetic stimulation facilitates neurorehabilitation after pediatric traumatic brain injury. Sci. Rep. 5:14769. doi: 10.1038/srep14769
Lu, X., Bao, X., Li, J., Zhang, G., Guan, J., Gao, Y., et al. (2017). High-frequency repetitive transcranial magnetic stimulation for treating moderate traumatic brain injury in rats: A pilot study. Exp. Ther. Med. 13, 2247–2254. doi: 10.3892/etm.2017.4283
Mackay, C., Kuys, S., and Brauer, S. (2017). The Effect of Aerobic Exercise on Brain-Derived Neurotrophic Factor in People with Neurological Disorders: A Systematic Review and MetaAnalysis. Neural Plast. 2017:4716197.
Mang, C., Campbell, K., Ross, C., and Boyd, L. (2013). Promoting Neuroplasticity for Motor Rehabilitation After Stroke: Considering the Effects of Aerobic Exercise and Genetic Variation on Brain-Derived Neurotrophic Factor. Phys. Ther. 93, 1707–1716. doi: 10.2522/ptj.20130053
Mangold, S., Keller, T., Curt, A., and Dietz, V. (2005). Transcutaneous functional electrical stimulation for grasping in subjects with cervical spinal cord injury. Spinal Cord 43, 1–13.
Marano, M., Anzini, G., Musumeci, G., Magliozzi, A., Pozzilli, V., Capone, F., et al. (2022). Transcutaneous Auricular Vagus Stimulation Improves Gait and Reaction Time in Parkinson’s Disease. Mov. Disord. 37, 2163–2164. doi: 10.1002/mds.29166
Marcus, H., Paine, H., Sargeant, M., Wolstenholme, S., Collins, K., Marroney, N., et al. (2019). Vestibular dysfunction in acute traumatic brain injury. J. Neurol. 266, 2430–2433.
Marien, M., Colpaert, F., and Rosenquist, A. (2004). Noradrenergic mechanisms in neurodegenerative diseases: a theory. Brain Res. Brain Res. Rev. 45, 38–78.
Mark, V., Taub, E., and Morris, D. (2006). Neuroplasticity and constraint-induced movement therapy. Eur. Medicophys. 42, 269–284.
Marquez, J., van Vliet, P., McElduff, P., Lagopoulos, J., and Parsons, M. (2015). Transcranial direct current stimulation (tDCS): does it have merit in stroke rehabilitation? A systematic review. Int. J. Stroke 10, 306–316. doi: 10.1111/ijs.12169
Marshall, S., Teasell, R., Bayona, N., Lippert, C., Chundamala, J., Villamere, J., et al. (2007). Motor impairment rehabilitation post acquired brain injury. Brain Inj. 21, 133–160.
Meng, L., Jin, M., Zhu, X., and Ming, D. (2022). Peripherical Electrical Stimulation for Parkinsonian Tremor: A Systematic Review. Front. Aging Neurosci. 14:795454. doi: 10.3389/fnagi.2022.795454
Milosevic, M., Nakanishi, T., Sasaki, A., Yamaguchi, A., Nomura, T., Popovic, M., et al. (2021). Cortical Reorganization After Traumatic Brain Injury Elicited Using Functional Electrical Stimulation Therapy: A Case Report. Front. Neurosci. 15:693861. doi: 10.3389/fnins.2021.693861
Mucha, A., Fedor, S., and DeMarco, D. (2018). Vestibular dysfunction and concussion. Handb. Clin. Neurol. 158, 135–144.
Murer, M., Yan, Q., and Raisman-Vozari, R. (2001). Brain-derived neurotrophic factor in the control human brain, and in Alzheimer’s disease and Parkinson’s disease. Prog. Neurobiol. 63, 71–124.
National Institute of Neurological Disorders and Stroke (2022). Traumatic Brain Injury: Hope Through Research. Bethesda, MA: National Institute of Neurological Disorders and Stroke.
Neeper, S., Gómez-Pinilla, F., Choi, J., and Cotman, C. (1996). Physical activity increases mRNA for brainderived neurotrophic factor and nerve growth factor in rat brain. Brain Res. 726, 49–56.
Nesin, S., Sabitha, K., Gupta, A., and Laxmi, T. (2019). Constraint Induced Movement Therapy as a Rehabilitative Strategy for Ischemic Stroke—Linking Neural Plasticity with Restoration of Skilled Movements. J. Stroke Cerebrovasc. Dis. 28, 1640–1653. doi: 10.1016/j.jstrokecerebrovasdis.2019.02.028
Nishibe, M., Barbay, S., Guggenmos, D., and Nudo, R. (2010). Reorganization of Motor Cortex after Controlled Cortical Impact in Rats and Implications for Functional Recovery. J. Neurotrauma 27, 2221–2232. doi: 10.1089/neu.2010.1456
Noé, E., Ferri, J., Colomer, C., Moliner, B., O’Valle, M., Ugart, P., et al. (2020). Feasibility, safety and efficacy of transauricular vagus nerve stimulation in a cohort of patients with disorders of consciousness. Brain Stimulat. 13, 427–429. doi: 10.1016/j.brs.2019.12.005
O’Malley, M., Ro, T., and Levin, H. (2006). Assessing and inducing neuroplasticity with transcranial magnetic stimulation and robotics for motor function. Arch. Phys. Med. Rehabil. 87, S59–S66.
Osborne, J., Botkin, R., Colon-Semenza, C., DeAngelis, T., Gallardo, O., Kosakowski, H., et al. (2022). Physical Therapist Management of Parkinson Disease: A Clinical Practice Guideline From the American Physical Therapy Association. Phys. Ther. 102, zab302.
Parazzini, M., Rossi, E., Ferrucci, R., Liorni, I., Priori, A., and Ravazzani, P. (2014). Modelling the electric field and the current density generated by cerebellar transcranial DC stimulation in humans. Clin. Neurophysiol. 125, 577–584. doi: 10.1016/j.clinph.2013.09.039
Paxman, E., Stilling, J., Mercier, L., and Debert, C. (2018). Repetitive transcranial magnetic stimulation (rTMS) as a treatment for chronic dizziness following mild traumatic brain injury. BMJ Case Rep. 2018:226698. doi: 10.1136/bcr-2018-226698
Pérez-Neri, I., González-Aguilar, A., Sandoval, H., Pineda, C., and Ríos, C. (2022). Potential Goals, Challenges, and Safety of Focused Ultrasound Application for Central Nervous System Disorders. Curr. Neuropharmacol. 20, 1807–1810. doi: 10.2174/1570159X20666220201092908
Piao, C., Stoica, B., Wu, J., Sabirzhanov, B., Zhao, Z., Cabatbat, R., et al. (2013). Late exercise reduces neuroinflammation and cognitive dysfunction after traumatic brain injury. Neurobiol. Dis. 54, 252–263. doi: 10.1016/j.nbd.2012.12.017
Pol, F., Salehinejad, M., Baharlouei, H., and Nitsche, M. (2021). The effects of transcranial direct current stimulation on gait in patients with Parkinson’s disease: a systematic review. Transl. Neurodegener. 10:22.
Pollock, A., Baer, G., Campbell, P., Choo, P., Forster, A., Morris, J., et al. (2014). Physical rehabilitation approaches for the recovery of function and mobility following stroke. Cochrane Database Syst. Rev. 2014, CD001920.
Popa, L., and Taylor, P. (2015). Functional electrical stimulation may reduce bradykinesia in Parkinson’s disease: A feasibility study. J. Rehabil. Assist. Technol. Eng. 2:2055668315607836. doi: 10.1177/2055668315607836
Popovic, M., Kapadia, N., Zivanovic, V., Furlan, J., Craven, B., and McGillivray, C. (2011). Functional electrical stimulation therapy of voluntary grasping versus only conventional rehabilitation for patients with subacute incomplete tetraplegia: a randomized clinical trial. Neurorehabil. Neural Repair. 25, 433–442. doi: 10.1177/1545968310392924
Popović Maneski, L., Jorgovanović, N., Ilić, V., Došen, S., Keller, T., Popović, M., et al. (2011). Electrical stimulation for the suppression of pathological tremor. Med. Biol. Eng. Comput. 49, 1187–1193.
Powell, K., and Paffenbarger, R. (1985). Workshop on Epidemiologic and Public Health Aspects of Physical Activity and Exercise: a summary. Public Health Rep. 100, 118–126.
Price, C., and Pandyan, A. (2000). Electrical stimulation for preventing and treating post-stroke shoulder pain. Cochrane Database Syst. Rev. 2000, CD001698.
Pruitt, D., Schmid, A., Kim, L., Abe, C., Trieu, J., Choua, C., et al. (2016). Vagus Nerve Stimulation Delivered with Motor Training Enhances Recovery of Function after Traumatic Brain Injury. J. Neurotrauma 33, 871–879. doi: 10.1089/neu.2015.3972
Pulverenti, T., Zaaya, M., and Knikou, M. (2022). Brain and spinal cord paired stimulation coupled with locomotor training affects polysynaptic flexion reflex circuits in human spinal cord injury. Exp. Brain Res. 240, 1687–1699. doi: 10.1007/s00221-022-06375-x
Purves, D., Augustine, G., Fitzpatrick, D., Katz, L., LaMantia, A., McNamara, J., et al. (2001). LongTerm Synaptic Potentiation. Bethesda, MD: National Library of Medicine.
Quandt, F., and Hummel, F. (2014). The influence of functional electrical stimulation on hand motor recovery in stroke patients: a review. Exp. Transl. Stroke Med. 6:9.
Raethjen, J., Lindemann, M., Schmaljohann, H., Wenzelburger, R., Pfister, G., and Deuschl, G. (2000). Multiple oscillators are causing parkinsonian and essential tremor. Mov. Disord. 15, 84–94.
Ragnarsson, K. (2008). Functional electrical stimulation after spinal cord injury: current use, therapeutic effects and future directions. Spinal Cord 46, 255–274.
Rao, V., Bechtold, K., McCann, U., Roy, D., Peters, M., Vaishnavi, S., et al. (2019). Low-Frequency Right Repetitive Transcranial Magnetic Stimulation for the Treatment of Depression After Traumatic Brain Injury: A Randomized Sham-Controlled Pilot Study. J. Neuropsychiatry Clin. Neurosci. 31, 306–318. doi: 10.1176/appi.neuropsych.17110338
Rasmussen, P., Brassard, P., Adser, H., Pedersen, M., Leick, L., Hart, E., et al. (2009). Evidence for a release of brain-derived neurotrophic factor from the brain during exercise. Exp. Physiol. 94, 1062–1069.
Rommelfanger, K., and Weinshenker, D. (2007). Norepinephrine: The redheaded stepchild of Parkinson’s disease. Biochem. Pharmacol. 74, 177–190. doi: 10.1016/j.bcp.2007.01.036
Rossi, S., Hallett, M., Rossini, P., Pascual-Leone, A., and Safety of Tms Consensus Group (2009). Safety, ethical considerations, and application guidelines for the use of transcranial magnetic stimulation in clinical practice and research. Clin. Neurophysiol. 120, 2008–2039.
Salvador, R., Mekonnen, A., Ruffini, G., and Miranda, P. (2010). Modeling the electric field induced in a high resolution realistic head model during transcranial current stimulation. Annu. Int. Conf. IEEE Eng. Med. Biol. Soc. IEEE Eng. Med. Biol. Soc. 2010, 2073–2076. doi: 10.1109/IEMBS.2010.5626315
Sanches, C., Stengel, C., Godard, J., Mertz, J., Teichmann, M., Migliaccio, R., et al. (2020). Past, Present, and Future of Non-invasive Brain Stimulation Approaches to Treat Cognitive Impairment in Neurodegenerative Diseases: Time for a Comprehensive Critical Review. Front. Aging Neurosci. 12:578339. doi: 10.3389/fnagi.2020.578339
Sandrow-Feinberg, H., and Houlé, J. (2015). Exercise after spinal cord injury as an agent for neuroprotection, regeneration and rehabilitation. Brain Res. 1619, 12–21.
Sandrow-Feinberg, H., Izzi, J., Shumsky, J., Zhukareva, V., and Houle, J. (2009). Forced Exercise as a Rehabilitation Strategy after Unilateral Cervical Spinal Cord Contusion Injury. J. Neurotrauma 26, 721–731.
Santos, M., Pagnussat, A., Mestriner, R., and Netto, C. (2013). Motor Skill Training Promotes Sensorimotor Recovery and Increases Microtubule-Associated Protein-2 (MAP-2) Immunoreactivity in the Motor Cortex after Intracerebral Hemorrhage in the Rat. ISRN Neurol. 2013:159184. doi: 10.1155/2013/159184
Schlemmer, E., and Nicholson, N. (2022). Vestibular Rehabilitation Effectiveness for Adults With Mild Traumatic Brain Injury/Concussion: A Mini-Systematic Review. Am. J. Audiol. 31, 228–242. doi: 10.1044/2021_AJA-21-00165
Sharp, D., Scott, G., and Leech, R. (2014). Network dysfunction after traumatic brain injury. Nat. Rev. Neurol. 10, 156–166.
Shin, S., Krishnan, V., Stokes, W., Robertson, C., Celnik, P., Chen, Y., et al. (2018). Transcranial magnetic stimulation and environmental enrichment enhances cortical excitability and functional outcomes after traumatic brain injury. Brain Stimulat. 11, 1306–1313. doi: 10.1016/j.brs.2018.07.050
Silva, P., de Cássia Marqueti, R., Livino-de-Carvalho, K., de Araujo, A., Castro, J., da Silva, V., et al. (2019). Neuromuscular electrical stimulation in critically ill traumatic brain injury patients attenuates muscle atrophy, neurophysiological disorders, and weakness: a randomized controlled trial. J. Intensive Care 7:59. doi: 10.1186/s40560-019-0417-x
Strafella, A., Paus, T., Barrett, J., and Dagher, A. (2001). Repetitive Transcranial Magnetic Stimulation of the Human Prefrontal Cortex Induces Dopamine Release in the Caudate Nucleus. J. Neurosci. 21, RC157–RC157.
Su, W., Wu, C., Chen, S., and Yang, F. (2017). Transcranial ultrasound stimulation promotes brainderived neurotrophic factor and reduces apoptosis in a mouse model of traumatic brain injury. Brain Stimulat. 10, 1032–1041. doi: 10.1016/j.brs.2017.09.003
Sujith, O. (2008). Functional electrical stimulation in neurological disorders. Eur. J. Neurol. 15, 437–444.
Tang, Y., Dong, X., Chen, G., Ye, W., Kang, J., Tang, Y., et al. (2020). Vagus Nerve Stimulation Attenuates Early Traumatic Brain Injury by Regulating the NF-κB/NLRP3 Signaling Pathway. Neurorehabil. Neural Repair. 34, 831–843.
Taub, E., Miller, N., Novack, T., Cook, E., Fleming, W., Nepomuceno, C., et al. (1993). Technique to improve chronic motor deficit after stroke. Arch. Phys. Med. Rehabil. 74, 347–354.
Taub, E., Uswatte, G., and Elbert, T. (2002). New treatments in neurorehabilitation founded on basic research. Nat. Rev. Neurosci. 3, 228–236.
Tefertiller, C., Rozwod, M., VandeGriend, E., Bartelt, P., Sevigny, M., and Smith, A. (2022). Transcutaneous Electrical Spinal Cord Stimulation to Promote Recovery in Chronic Spinal Cord Injury. Front. Rehabil. Sci. 2:740307. doi: 10.3389/fresc.2021.740307
Tharu, N., Alam, M., Ling, Y., Wong, A., and Zheng, Y. (2023). Combined Transcutaneous Electrical Spinal Cord Stimulation and Task-Specific Rehabilitation Improves Trunk and Sitting Functions in People with Chronic Tetraplegia. Biomedicines 11:34. doi: 10.3390/biomedicines11010034
Thibaut, A., Schiff, N., Giacino, J., Laureys, S., and Gosseries, O. (2019). Therapeutic interventions in patients with prolonged disorders of consciousness. Lancet Neurol. 18, 600–614.
Tian, D., and Izumi, S. (2022). Interhemispheric Facilitatory Effect of High-Frequency rTMS: Perspective from Intracortical Facilitation and Inhibition. Brain Sci. 12:970. doi: 10.3390/brainsci12080970
Traversa, R., Cicinelli, P., Bassi, A., Rossini, P., and Bernardi, G. (1997). Mapping of motor cortical reorganization after stroke. A brain stimulation study with focal magnetic pulses. Stroke 28, 110–117. doi: 10.1161/01.str.28.1.110
Tyler, W., Lani, S., and Hwang, G. (2018). Ultrasonic modulation of neural circuit activity. Curr. Opin. Neurobiol. 50, 222–231.
van der Scheer, J., Goosey-Tolfrey, V., Valentino, S., Davis, G., and Ho, C. (2021). Functional electrical stimulation cycling exercise after spinal cord injury: a systematic review of health and fitnessrelated outcomes. J. Neuroeng. Rehabil. 18:99. doi: 10.1186/s12984-021-00882-8
van Praag, H. (2009). Exercise and the brain: something to chew on. Trends Neurosci. 32, 283–290. doi: 10.1016/j.tins.2008.12.007
Varma, A., Das, A., Wallace, G., Barry, J., Vertegel, A., Ray, S., et al. (2013). Spinal cord injury: A review of current therapy, future treatments, and basic science frontiers. Neurochem Res. 38, 895–905.
Vaynman, S., Ying, Z., and Gomez-Pinilla, F. (2003). Interplay between brain-derived neurotrophic factor and signal transduction modulators in the regulation of the effects of exercise on synaptic-plasticity. Neuroscience 122, 647–657. doi: 10.1016/j.neuroscience.2003.08.001
Verdugo-Diaz, L., Estrada-Rojo, F., Garcia-Espinoza, A., Hernandez-Lopez, E., Hernandez-Chavez, A., Guzman-Uribe, C., et al. (2017). Effect of intermediate-frequency repetitive transcranial magnetic stimulation on recovery following traumatic brain injury in rats. BioMed Res. Int. 2017:4540291. doi: 10.1155/2017/4540291
Villamar, M., Santos Portilla, A., Fregni, F., and Zafonte, R. (2012). Noninvasive Brain Stimulation to Modulate Neuroplasticity in Traumatic Brain Injury. Neuromodul. Technol. Neural Interf. 15, 326–338.
Wagle Shukla, A., Shuster, J., Chung, J., Vaillancourt, D., Patten, C., Ostrem, J., et al. (2016). Repetitive Transcranial Magnetic Stimulation (rTMS) Therapy in Parkinson Disease: A Meta-Analysis. PM R. 8, 356–366.
Wang, H., Liu, N., Zhang, Y., Deng, L., Lu, Q., Shields, C., et al. (2015). Treadmill training induced lumbar motoneuron dendritic plasticity and behavior recovery in adult rats after a thoracic contusive spinal cord injury. Exp. Neurol. 271, 368–378. doi: 10.1016/j.expneurol.2015.07.004
Wang, Y., Li, S., Wang, D., Wu, M., He, J., Zhang, J., et al. (2021). Transcutaneous Auricular Vagus Nerve Stimulation: From Concept to Application. Neurosci. Bull. 37, 853–862. doi: 10.1007/s12264-020-00619-y
Waters, R., Adkins, R., Yakura, J., and Sie, I. (1994). Motor and sensory recovery following incomplete tetraplegia. Arch. Phys. Med. Rehabil. 75, 306–311.
Waters, R., Yakura, J., Adkins, R., and Sie, I. (1992). Recovery following complete paraplegia. Arch. Phys. Med. Rehabil. 73, 784–789.
Wilson, J., Cadotte, D., and Fehlings, M. (2012). Clinical predictors of neurological outcome, functional status, and survival after traumatic spinal cord injury: a systematic review. J. Neurosurg. Spine 17(1 Suppl), 11–26. doi: 10.3171/2012.4.AOSPINE1245
Wofford, K., Loane, D., and Cullen, D. (2019). Acute drivers of neuroinflammation in traumatic brain injury. Neural Regen. Res. 14, 1481–1489.
Wu, D., Ma, J., Zhang, L., Wang, S., Tan, B., and Jia, G. (2020). Effect and safety of transcutaneous auricular vagus nerve stimulation on recovery of upper limb motor function in subacute ischemic stroke patients: A randomized pilot study. Neural Plast. 2020:8841752. doi: 10.1155/2020/8841752
Xing, Y., and Bai, Y. A. (2020). Review of exercise-induced neuroplasticity in ischemic stroke: pathology and mechanisms. Mol. Neurobiol. 57, 4218–4231. doi: 10.1007/s12035-020-02021-1
Xu, F., Hao, M., Xu, S., Hu, Z., Xiao, Q., and Lan, N. (2016). “Development of a closed-loop system for tremor suppression in patients with Parkinson’s disease,” in Proceedings of the 2016 38th Annual International Conference of the IEEE Engineering in Medicine and Biology Society (EMBC), Piscataway, NJ.
Yang, C., Guo, Z., Peng, H., Xing, G., Chen, H., McClure, M., et al. (2018). Repetitive transcranial magnetic stimulation therapy for motor recovery in Parkinson’s disease: A Meta-analysis. Brain Behav. 8, e01132.
Yang, F., Lu, W., Lin, W., Chang, C., and Huang, S. (2015). Enhancement of neurotrophic factors in astrocyte for neuroprotective effects in brain disorders using low-intensity pulsed ultrasound stimulation. Brain Stimulat. 8, 465–473. doi: 10.1016/j.brs.2014.11.017
Ying, Z., Roy, R., Edgerton, V., and Gómez-Pinilla, F. (2005). Exercise restores levels of neurotrophins and synaptic plasticity following spinal cord injury. Exp. Neurol. 193, 411–419.
Zatorre, R., Fields, R., and Johansen-Berg, H. (2012). Plasticity in Gray and White. Nat. Neurosci. 15, 528–536.
Zhang, D., Poignet, P., Widjaja, F., and Tech Ang, W. (2011). Neural oscillator based control for pathological tremor suppression via functional electrical stimulation. Control Eng. Pract. 19, 74–88.
Zhou, L., Huang, X., Li, H., Guo, R., Wang, J., Zhang, Y., et al. (2021). Rehabilitation effect of rTMS combined with cognitive training on cognitive impairment after traumatic brain injury. Am. J. Transl. Res. 13, 11711–11717.
Keywords: neuromodulation, neuroplasticity, rehabilitation, vagus nerve stimulation, transcranial magnetic stimulation, peripheral nerve stimulation
Citation: Evancho A, Tyler WJ and McGregor K (2023) A review of combined neuromodulation and physical therapy interventions for enhanced neurorehabilitation. Front. Hum. Neurosci. 17:1151218. doi: 10.3389/fnhum.2023.1151218
Received: 25 January 2023; Accepted: 30 June 2023;
Published: 21 July 2023.
Edited by:
Cathra Halabi, University of California, San Francisco, United StatesReviewed by:
Erica Pitsch, University of California, San Francisco, United StatesLeighton B. Hinkley, University of California, San Francisco, United States
Copyright © 2023 Evancho, Tyler and McGregor. This is an open-access article distributed under the terms of the Creative Commons Attribution License (CC BY). The use, distribution or reproduction in other forums is permitted, provided the original author(s) and the copyright owner(s) are credited and that the original publication in this journal is cited, in accordance with accepted academic practice. No use, distribution or reproduction is permitted which does not comply with these terms.
*Correspondence: Alexandra Evancho, YW1lbGdpbkB1YWIuZWR1