- 1Department of Neurology, Beijing Tiantan Hospital, Capital Medical University, Beijing, China
- 2China National Clinical Research Center for Neurological Diseases, Beijing, China
- 3Advanced Innovation Center for Human Brain Protection, Capital Medical University, Beijing, China
- 4Collaborative Innovation Center for Brain Disorders, Beijing Institute of Brain Disorders, Capital Medical University, Beijing, China
Cortical network hyperexcitability is an inextricable feature of Alzheimer’s disease (AD) that also might accelerate its progression. Seizures are reported in 10–22% of patients with AD, and subclinical epileptiform abnormalities have been identified in 21–42% of patients with AD without seizures. Accurate identification of hyperexcitability and appropriate intervention to slow the compromise of cognitive functions of AD might open up a new approach to treatment. Based on the results of several studies, epileptiform discharges, especially those with specific features (including high frequency, robust morphology, right temporal location, and occurrence during awake or rapid eye movement states), frequent small sharp spikes (SSSs), temporal intermittent rhythmic delta activities (TIRDAs), and paroxysmal slow wave events (PSWEs) recorded in long-term scalp electroencephalogram (EEG) provide sufficient sensitivity and specificity in detecting cortical network hyperexcitability and epileptogenicity of AD. In addition, magnetoencephalogram (MEG), foramen ovale (FO) electrodes, and computational approaches help to find subclinical seizures that are invisible on scalp EEGs. We performed a comprehensive analysis of the aforementioned electrophysiological biomarkers of AD-related seizures.
Introduction
Brain rhythms are fundamental in maintaining normal cognition and behavior, and neuronal hyperexcitability has emerged as an important electrical abnormality that could not only lead to memory failure in the early stage of Alzheimer’s disease (AD) but also contribute to the progression of the disease (Noebels, 2011; Vossel et al., 2013; Busche and Konnerth, 2015, 2016; Palop and Mucke, 2016). Once neuronal hyperexcitability of the cerebral cortex is established, it can manifest as epileptic seizures or subclinical epileptiform discharges. Seizures are reported in 10–22% of patients with AD (Friedman et al., 2011; Vossel et al., 2013; Cretin et al., 2016; Sarkis et al., 2016), and subclinical epileptiform activities have been found in more than 40% of patients with AD in a recent prospective electroencephalogram (EEG) study (Vossel et al., 2016).
The presence of seizure and subclinical epileptiform activities have been shown to contribute to impaired memory and attention, especially cognitive fluctuation (Palop and Mucke, 2009; Vossel et al., 2016). A greater extent of neuronal hyperactivity tends to occur in the earliest stages of AD compared with later stages, which has been shown from both fMRI studies in humans and neuronal activity studies in mouse models of AD (Zott et al., 2018). “Antiepileptogenic” therapies in AD appear to be feasible in order to delay the progression of the disease. Although they are easy to implement, performing these treatments in all patients with AD presents a problem as the majority of patients with AD would not have any benefit, though the subset of patients with AD who have epileptiform activity might benefit greatly from early treatment with antiepileptic drugs (AEDs). Hence, effective treatment first requires the detection and suppression of seizures and subclinical epileptiform activity. In this study, we performed a comprehensive analysis of the potential electrophysiological biomarkers of AD-related seizures in humans and discuss their feasibility in clinical practice and their potential to predict the subsequent development of clinical seizures and epilepsy.
Scalp Electroencephalogram
Epileptiform Discharges
The best-known biomarker of hyperexcitability in humans is the epileptiform discharge, which is defined as a paroxysmal EEG graphoelement (spike or sharp wave) with a duration of 20–200 ms that is clearly distinct from ongoing background EEG activity followed by slow waves (Noachtar and Remi, 2009) (Figure 1A). On scalp EEGs, visible subclinical epileptiform discharges occur in 9–21% of patients with AD who had no prior history of epilepsy or seizure, a higher rate than 0–5% of healthy controls (Vossel et al., 2016; Brunetti et al., 2020; Lam et al., 2020). Long-term EEG detection has found that epileptiform discharges in patients with AD mainly occur during a sleep state and are largely lateralized to the temporal lobe, especially the left temporal lobe.
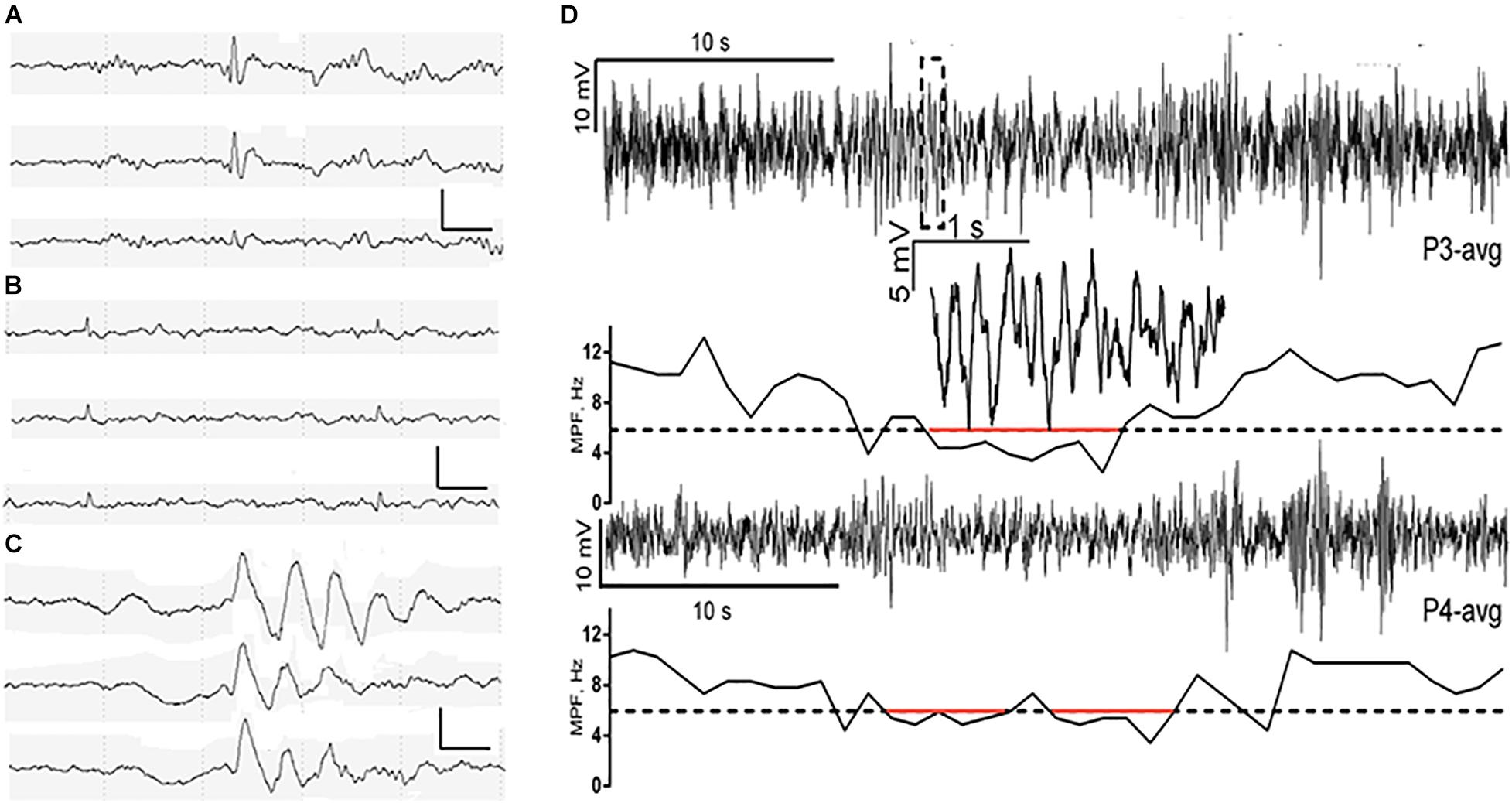
Figure 1. Characterization of spike, small sharp spike (SSS), temporal intermittent rhythmic delta activities (TIRDA), and paroxysmal slow-wave event (PSWE). (A–C) Representative examples of spike, SSS, and TIRDA from the right temporal region in three patients with AD from our own ward, respectively. Scalp electrodes were placed using the international 10–20 system, sampling at 200 Hz. EEG channels (top to bottom): F8-ave, T4-ave, and T6-ave. Calibration bars: 100 μV and 500 ms. (D) A PSWE detected in a patient with AD. Traces from electrodes P3 (upper trace) and P4 (averaged as reference) are shown. The segment within the dashed rectangle of P3 is shown magnified. The median power frequency is presented below each trace. Segments below 6 Hz (dashed line) are marked in red. From Milikovsky et al. (2019). Reprint with permission from The American Association for the Advancement of Science (AAAS).
Epileptiform discharges that had more robust morphological features including significantly larger trough voltage, peak-to-trough voltage, and slope of falling half-wave of the peak are more strongly associated with clinical seizures in AD (Lam et al., 2020). The guiding significance of robust epileptiform discharges is similar to giant spikes (simultaneous spikes in all channels, with massive voltages > ± 10 SD from the filtered baseline in at least one channel) observed in transgenic mice with APP/PS1 mutations (Gureviciene et al., 2019). The same indicative effect being strongly associated with clinical seizures has also been seen in right temporal epileptiform discharges (100% specificity and 43% sensitivity) (Lam et al., 2020).
The detection rate of epileptic discharges in patients with AD by scalp EEG is significantly correlated with recording time. Approximately, 62% of patients with AD showed epileptic discharges in EEG recordings lasting 24 h (Horvath et al., 2018) and only 3% in 20 min of eyes-closed EEG recordings (Liedorp et al., 2010). Most epileptiform discharges from patients with AD occur during sleep, requiring overnight EEG monitoring for detection. Epileptiform discharges occurred most frequently during N2 sleep, while awake and rapid eye moment (REM) states are the least permissive states for the expression of epileptiform discharges in patients with focal epilepsy (Sammaritano et al., 1991; Malow et al., 1997; Diaz-Negrillo, 2013), and the results of EEG monitoring in patients with AD are consistent with this. While epileptiform discharges detected during awake or REM states were suggestive for clinical seizures (85.7% specificity and 85.7% sensitivity) (Lam et al., 2020), the study by Lam et al. (2020) also pointed out that patients with AD who had high frequencies of epileptiform discharges were more likely to have generalized convulsions.
Lam et al. (2017a) found that spikes (sharply contoured transients, clearly distinguishable from, and usually interrupting background activities, with a duration of <70 ms) from patients with aMCI were largely lateralized to the left mesial temporal lobe (mTL), whereas they were largely lateralized to the right mTL in moderate patients with AD. Thus, they proposed that there might be left hemisphere hyperactivity predisposition and left mTL susceptibility in the early stages of AD. Data from anatomical and functional connectivity modalities also support this; the result may also be mediated in part by the E4 variant of apolipoprotein E (APOE4) allele (Thompson et al., 2003; Damoiseaux et al., 2009; Donix et al., 2013; Wessa et al., 2016; Yang et al., 2017; Liu et al., 2018; Zott et al., 2018).
Studies in animal models with AD have established a direct link between neuronal hyperactivity and propagation of amyloid and tau pathology (Cirrito et al., 2005; Busche et al., 2012; Pooler et al., 2013; Wu et al., 2016). Reyes-Marin and Nunez (2017) also found that the frequency of epileptiform-like discharges was significantly correlated with the number of amyloid-β plaques in APP/PS1 mice. The results of these animal studies illustrate that the asymmetry in temporal lobe hyperexcitability might be related to an asymmetric cascade of AD pathology.
Finally, there is growing evidence that scalp EEG epileptiform discharges in patients with AD have a variable association with clinical seizures (Vossel et al., 2016; Lam et al., 2020). With an infrequent frequency (<10 per 24 h), these epileptiform discharges typically arise locally from the lateral temporal cortex or propagate to the surface from deep mTL foci (Bakker et al., 2012; Lam et al., 2017a). Thus, to some extent, scalp EEG epileptiform discharges alone are limited, and they may not be the optimal biomarkers for epileptogenicity in AD.
Small Sharp Spikes
Small sharp spikes (SSSs), also known as benign sporadic sleep spikes (White et al., 1977), are low amplitude (30–50 uV), short duration (<50 ms) spikes that occur during early drowsiness, and N1 and N2 sleep stages (Figure 1B). They are widely distributed in bilateral brain hemispheres typically seen independently over the bi-frontotemporal regions but can also occur unilaterally. In addition, SSSs are usually considered as a benign variant of EEG, which has no association with epilepsy. However, recent studies have indicated that SSS-like waveforms in the scalp might be related to mTL epileptiform discharges, especially unilateral SSS-like waveforms (Lam et al., 2017a; Issa et al., 2018). According to the study by Lam et al. (2020) unilateral (left or right temporal region) SSS-like waveforms with high frequency (>100 per 24 h) are associated with clinical seizure in AD. However, pinpointing SSSs as an electrophysiological biomarker of seizure in patients with AD requires further validation in more well-designed studies to distinguish pathological SSSs from benign SSSs.
Temporal Intermittent Rhythmic Delta Activity
Temporal intermittent rhythmic delta activity (TIRDA) refers to the delta activity distributed in the temporal lobe with a frequency of 2.5–3.0 Hz and a sinusoidal or serrated waveform that occurs repeatedly and intermittently and has a strong association with mesial temporal lobe epilepsy (mTLE) (Reiher et al., 1989; Gambardella et al., 1995; Normand et al., 1995; Gennaro et al., 2003) (Figure 1C). TIRDA occurs in 26% of patients with AD-related epilepsy (Lam et al., 2020). Although occurring at a lower frequency in patients with AD without epilepsy, TIRDA has been associated with higher diagnostic confidence compared to epileptiform discharges (positive predictive value for determining epileptiform, 83.3 vs. 61.5%) (Lam et al., 2020). Like epileptiform discharges, TIRDA is more likely to occur during N2 sleep and in the left temporal location, while TIRDA that does occur during awake or REM states is more strongly associated with clinical seizures in AD (Lam et al., 2020). What is more, the lateralization of TIRDA matches that of epileptiform discharges in patients with AD. However, because of its low frequency (<10 per 24 h) of occurrence in AD, the utility of TIRDA as a quantitative biomarker is somewhat limited (Lam et al., 2020).
Paroxysmal Slow Cortical Activity
Slowing of scalp EEG activity has been observed in AD by studying early quantitative EEG power changes in patients with AD (Musaeus et al., 2018), and the EEG slowing correlates with decreased cognition in patients with AD and unimpaired older adults (Stomrud et al., 2010; Musaeus et al., 2018). The EEG slowing might be a potential sign of neural network dysfunction. Milikovsky et al. (2019) analyzed the temporal characteristics of EEG slowing from patients with AD and found that cortical slowing is in part composed of transient paroxysmal slowing of the cortical network. This transient paroxysmal slowing of the cortical network is called paroxysmal slow wave events (PSWEs), which refer to “events” when the median power frequency is lower than 6 Hz for at least 5 consecutive s on the scalp EEG (Milikovsky et al., 2019) (Figure 1D). These PSWEs were also obtained in patients with epilepsy, animal models of AD, and animal models of epilepsy.
Investigating PSWE characteristics in aged mice, young 5xFAD mice, and young rats with epilepsy, Milikovsky et al. (2019) found that there was a spatial correlation between PSWEs and blood-brain barrier dysfunction (BBBd). They also observed a causal link between BBBd and PSWE in animal models by infusing albumin into the lateral ventricle of rats and then detecting the PSWEs in epidural recordings after a month of infusion. Their results indicate that PSWEs can be an indicator for the subclinical seizure-like activity that reflects microvascular pathology. Hence, PSWE detection in routine scalp EEG recordings might be an affordable and sensitive diagnostic indicator for subclinical seizures among patients with AD and as a pharmacodynamic measure for AEDs. However, we must realize that seizure and epileptiform activity mostly occur in the mTL in patients with AD and are often undetectable by scalp EEG recording. Thus, more effective approaches that can detect epileptiform discharges in the mTL are needed to improve the efficiency of scalp EEG monitoring.
Magnetoencephalography
Magnetoencephalography (MEG), which is thought to be more sensitive to discharges of tangential sulcal sources than EEG with a high temporal and spatial resolution (Oishi et al., 2002), has also been widely used as a non-invasive tool to assess mTL activity and localized epileptogenic lesions in an epilepsy non-invasive tool (Enatsu et al., 2008; Kaiboriboon et al., 2010; Wennberg et al., 2011; Wennberg and Cheyne, 2014). Although the main application field of MEG is the presurgical evaluation of drug-resistant epilepsy, MEG also provides a complementary approach to scalp EEG in detecting cortical network dysfunction in patients with AD. Vossel et al. (2016) prospectively assessed the epileptiform activities in 33 patients with AD and 19 age-matched healthy controls, by 1-h resting MEG recordings and overnight scalp EEG recordings. Vossel et al. (2016) found that visible epileptiform discharges on MEG occurred in 33.3% of patients with AD, much higher than that of 21.1% on overnight scalp EEGs. Although the specificity of MEG might not be as high as that of overnight EEG (11% of healthy controls had epileptiform discharges visible on MEG while had them on overnight EEG), patients with epileptiform activity on MEG or overnight EEG had significantly faster rates of cognitive decline than those without epileptiform activity (Vossel et al., 2016). These findings suggest that MEG is an effective electrophysiological biomarker of cortical hyperexcitability in patients with AD with much higher sensitivity than scalp EEG. Interestingly, epileptiform discharges detected by MEG were more right-sided compared with the more left-sided epileptiform discharges detected by scalp-EEG (Vossel et al., 2016). Different detection capabilities or different interpretation techniques may contribute to the discordance, but the principle behind this remains unclear.
Importantly, epileptiform discharges in patients with AD mainly occur during a sleep state, while the MEG recording cannot exceed a few hours at a time. Thus, it is nearly impossible to capture natural sleep or paroxysmal events during a sleep state by MEG. Considering the high requirement of equipment maintenance and the high costs of MEG examination, it is difficult to carry out MEG as a routine examination in general patients with AD. However, for patients with high suspicion of cortical hyperexcitability without visible epileptiform discharge on scalp EEG, MEG examination can be chosen to individually guide the usage of AEDs.
Foramen Ovale Electrodes
Foramen ovale (FO) electrodes are a semi-invasive alternative to stereo-EEG electrodes. After general endotracheal anesthesia is induced, a single multi-contact FO electrode is positioned adjacent to each mTL inserted through the cheek skin to the ipsilateral natural aperture (FO) in the skull (Sheth et al., 2014). High-quality, long-term recordings can be obtained directly from the mTL using FO electrodes (Sperling et al., 1986; Fernández Torre et al., 1999). As FO electrodes will cause no skull defect, scalp EEG recording can be carried out simultaneously with FO electrodes. Furthermore, as mentioned above, since the mTL is the most affected location in patients with AD and FO electrodes that are accurate in capturing samples from deep temporal activities might be the best method for assessing subclinical discharges in patients with AD (Lam et al., 2019).
Lam et al. (2017a) used bilateral FO electrode recordings in two patients with AD (one had aMCI and another had moderate dementia; both had cerebrospinal fluid biomarker-confirmed AD) for the first time. The FO electrode recordings of both patients with AD demonstrated abundant, sleep-activated (especially non-REM sleep) spikes, and over 95% of the spikes were invisible on the simultaneous scalp EEG. In addition, three silent mTL seizures were captured on FO electrode recordings, while no visible evidence was found on scalp EEG in the patient with aMCI. They also found that mTL spikes on FO electrode recordings in the patient with aMCI occurred at up to a 10-fold rate compared with the patient with moderate dementia, supporting the concept that a greater extent of neuronal hyperactivity had developed during the earliest stages of AD rather than later stages (Zott et al., 2018). Existing studies indicate that mTL epileptiform discharges detected by FO electrodes mostly occur during a sleep state in both humans and mouse AD models (Lam et al., 2017a; Brown et al., 2018) but non-REM sleep in humans (Lam et al., 2017a; Brown et al., 2018) and REM sleep in mouse models with AD (Kam et al., 2016; Brown et al., 2018). Although FO electrodes offer high quality and long-term recordings of mTL activity, their utility as a screening tool for epileptiform discharges in patients with AD is limited because of their high cost, potential risks (for example, central nervous system infection and bleeding), and the requirement of good neurosurgical skills for electrode placement.
Computational Approaches
For patients with AD, routine scalp EEG monitoring (20–40 min) is both necessary and feasible for the purpose of observing brain rhythms, but it is less helpful in recording epileptiform activity (Liedorp et al., 2010). However, performing overnight EEG, MEG, or FO electrode recordings is not feasible for the reasons of hospital resources, willingness to monitor patients, and high costs. As such, scientists have developed non-invasive and inexpensive methods, which can be widely implemented for diagnosing epileptiform activity.
Many studies have demonstrated that though there is a lack of visible epileptiform activity on scalp EEG, non-specific subtle and quantitative changes in local or long-distance networks induced by mTL spikes or seizures (Tyvaert et al., 2009; Vulliemoz et al., 2011; Cunningham et al., 2012; Aghakhani et al., 2015; Khoo et al., 2017; Naftulin et al., 2018) can be detected indirectly using artificial intelligence approaches. In a proof-of-principle study, Lam et al. (2016) analyzed the EEG data of 25 patients with epilepsy who underwent scalp EEG recording and FO electrode recording. By dividing scalp EEG recording into epochs and extracting coherence features of each epoch, they trained a seizure detector which correctly identified 40% of scalp-negative seizures (seizures detected by FO electrode recording but invisible on the scalp EEG), with a positive predictive value of 75% (Lam et al., 2016). In another publication by the same authors (Lam et al., 2017b), they extracted scalp EEG spectral power as an input feature and trained a machine learning algorithm that correctly identified 50% of scalp-negative seizures, with a positive predictive value of 94%. Studies by other researchers (Nayak et al., 2004; Koessler et al., 2015) also pointed out that mTL spikes or seizures might be detected by quantitative EEG signatures though they are invisible on a scalp EEG. Babiloni et al. (2020) found that higher temporal delta source activities are more strongly associated with clinical seizures in AD by estimating regional EEG cortical sources using exact low-resolution brain electromagnetic tomography (eLORETA) freeware. They used the area under receiver operating characteristic (AUROC) curves to index the accuracy of eLORETA solutions in identifying seizures and found an accuracy of 69% (Babiloni et al., 2020).
Development of computational approaches that accurately identify spikes or seizures from scalp EEG or MEG is underway (Lam et al., 2016, 2017b; Spyrou et al., 2016; Pizzo et al., 2019). However, this might be difficult to validate with large clinical data sets of combined scalp EEG/MEG and intracranial (especially FO electrode) recordings. Once the computational approaches become established, patients with AD may obtain more accurate guidance on antiepileptic therapy.
Discussion
Patients with AD have an increased risk of seizures, compared to an age-matched control population, and they are over 10 times more likely to develop epilepsy, especially patients with early onset familial AD (Amatniek et al., 2006; Pandis and Scarmesa, 2012). The significance of interictal epileptiform activities in patients with AD is still controversial, and they are biomarkers of hyperexcitability, but their relation to seizures is actually unknown. Interictal epileptiform activities may compromise memory formation and consolidate themselves, even in the absence of seizures. There are also studies that contend that seizures might be a critical part of AD pathogenesis (Scarmeas et al., 2009). Numerous studies (Reyes-Marin and Nunez, 2017; Gureviciene et al., 2019) in animal models also suggest the importance of detecting these events, finding that a high number of interictal spikes increases the risk of seizures.
However, one thing most studies agree on is that patients with AD with seizures or subclinical epileptiform activity experience faster cognitive declines over time (Vossel et al., 2016), and patients with AD might benefit from antiepileptic therapy. Moreover, the identification of biomarkers of epileptogenesis in patients with AD is a prerequisite for designing and developing targeted therapeutic approaches.
Conclusion
To date, human studies have identified several candidate EEG biomarkers as follows: epileptiform discharges (especially those with specific features, including high frequency, robust morphology, right temporal location, and occurrence during awake or REM states), frequent SSSs, TIRDA, and PSWEs recorded by scalp EEG (Table 1). In addition, MEG, FO electrodes, and computational approaches help to find subclinical seizures that are invisible on scalp EEG electrodes in patients with AD (Table 1). However, these findings require further study in humans as well as in animals to validate them and determine which are reliable and feasible electrophysiological biomarkers of epileptogenicity in patients with AD.
Author Contributions
TY chose the search term, determined the methodology of the review, and drafted the manuscript. XL helped to choose the search term and determined the methodology of the review. JW and QW revised the manuscript and commented on previous versions of the manuscript. All authors read and approved the final manuscript.
Funding
This study was supported by the National Key R&D Program of China 2017YFC1307500 and Capital Healthy Development Research Funding 2016-1-2011 and 2020-1-2013.
Conflict of Interest
The authors declare that the research was conducted in the absence of any commercial or financial relationships that could be construed as a potential conflict of interest.
Publisher’s Note
All claims expressed in this article are solely those of the authors and do not necessarily represent those of their affiliated organizations, or those of the publisher, the editors and the reviewers. Any product that may be evaluated in this article, or claim that may be made by its manufacturer, is not guaranteed or endorsed by the publisher.
Acknowledgments
The authors thank AiMi Academic Services (www.aimieditor.com) for the English language editing and review services.
Abbreviations
5xFAD, 5x familial AD; AD, Alzheimer’s disease; AEDs, antiepileptic drugs; aMCI, amnesic mild cognitive impairment; APOE4, the E4 variant of apolipoprotein E; AUROC, area under receiver operating characteristic; BBBd, blood-brain barrier dysfunction; EEG, electroencephalogram; eLORETA, exact low-resolution brain electromagnetic tomography; FO, foramen ovale; MEG, magnetoencephalogram; mTL, mesial temporal lobe; PSWEs, paroxysmal slow wave events; REM, rapid eye moment; SSS, small sharp spikes; TIRDA, temporal inter mitten rhythmic delta activity.
References
Aghakhani, Y., Beers, C. A., Pittman, D. J., Gaxiola-Valdez, I., Goodyear, B. G., and Federico, P. (2015). Co-localization between the BOLD response and epileptiform discharges recorded by simultaneous intracranial EEG-fMRI at 3 T. Neuroimage Clin. 7, 755–763. doi: 10.1016/j.nicl.2015.03.002
Babiloni, C., Noce, G., Di Bonaventura, C., Lizio, R., Pascarelli, M. T., Tucci, F., et al. (2020). Abnormalities of cortical sources of resting state delta electroencephalographic rhythms are related to epileptiform activity in patients with amnesic mild cognitive impairment not due to Alzheimer’s disease. Front. Neurol. 11:514136. doi: 10.3389/fneur.2020.514136
Bakker, A., Krauss, G. L., Albert, M. S., Speck, C. L., Jones, L. R., Stark, C. E., et al. (2012). Reduction of hippocampal hyperactivity improves cognition in amnestic mild cognitive impairment. Neuron 74, 467–474. doi: 10.1016/j.neuron.2012.03.023
Brown, R., Lam, A. D., Gonzalez-Sulser, A., Ying, A., Jones, M., Chou, R. C., et al. (2018). Circadian and brain state modulation of network hyperexcitability in Alzheimer’s disease. eNeuro 5:ENEURO.0426-17.2018. doi: 10.1523/ENEURO.0426-17.2018
Brunetti, V., D’Atri, A., Della Marca, G., Vollono, C., Marra, C., Vita, M. G., et al. (2020). Subclinical epileptiform activity during sleep in Alzheimer’s disease and mild cognitive impairment. Clin. Neurophysiol. 131, 1011–1018. doi: 10.1016/j.clinph.2020.02.015
Busche, M. A., and Konnerth, A. (2015). Neuronal hyperactivity – a key defect in Alzheimer’s disease? BioEssays 37, 624–632. doi: 10.1002/bies.201500004
Busche, M. A., and Konnerth, A. (2016). Impairments of neural circuit function in Alzheimer’s disease. Philos. Trans. R. Soc. Lond. B Biol. Sci. 371:20150429. doi: 10.1098/rstb.2015.0429
Busche, M. A., Chen, X., Henning, H. A., Reichwald, J., Staufenbiel, M., Sakmann, B., et al. (2012). Critical role of soluble amyloid-β for early hippocampal hyperactivity in a mouse model of Alzheimer’s disease. Proc. Natl. Acad. Sci. U.S.A. 109, 8740–8745. doi: 10.1073/pnas.1206171109
Cirrito, J. R., Yamada, K. A., Finn, M. B., Sloviter, R. S., Bales, K. R., May, P. C., et al. (2005). Synaptic activity regulates interstitial fluid amyloid-beta levels in vivo. Neuron 46, 913–922. doi: 10.1016/j.neuron.2005.10.028
Amatniek, J. C., Hauser, W. A., DelCastillo-Castaneda, C., Jacobs, D. M., Marder, K., Bell, K., et al. (2006). Incidence and predictors of seiures in patients with Alzheimer’s disease. Epilepsia 47, 867–872. doi: 10.1111/j.1528-1167.2006.00554.x
Cretin, B., Sellal, F., Philippi, N., Bousiges, O., Di Bitonto, L., Martin-Hunyadi, C., et al. (2016). Epileptic prodromal Alzheimer’s disease, a retrospective study of 13 new cases: expanding the spectrum of Alzheimer’s disease to an epileptic variant? J. Alzheimers Dis. 52, 1125–1133. doi: 10.3233/JAD-150096
Cunningham, C. B., Goodyear, B. G., Badawy, R., Zaamout, F., Pittman, D. J., Beers, C. A., et al. (2012). Intracranial EEG-fMRI analysis of focal epileptiform discharges in humans. Epilepsia 53, 1636–1648. doi: 10.1111/j.1528-1167.2012.03601.x
Damoiseaux, J. S., Smith, S. M., Witter, M. P., Sanz-Arigita, E. J., Barkhof, F., Scheltens, P., et al. (2009). White matter tract integrity in aging and Alzheimer’s disease. Hum. Brain Mapp. 30, 1051–1059. doi: 10.1002/hbm.20563
Diaz-Negrillo, A. (2013). Influence of sleep and sleep deprivation on ictal and interictal epileptiform activity. Epilepsy Res. Treat. 2013:492524. doi: 10.1155/2013/492524
Donix, M., Burggren, A. C., Scharf, M., Marschner, K., Suthana, N. A., Siddarth, P., et al. (2013). APOE associated hemispheric asymmetry of entorhinal cortical thickness in aging and Alzheimer’s disease. Psychiatry Res. 214, 212–220. doi: 10.1016/j.pscychresns.2013.09.006
Enatsu, R., Mikuni, N., Usui, K., Matsubayashi, J., Taki, J., Begum, T., et al. (2008). Usefulness of MEG magnetometer for spike detection in patients with mesial temporal epileptic focus. Neuroimage 41, 1206–1219. doi: 10.1016/j.neuroimage.2008.03.038
Fernández Torre, J. L., Alarcón, G., Binnie, C. D., and Polkey, C. E. (1999). Comparison of sphenoidal, foramen ovale and anterior temporal placements for detecting interctal epileptiform discharges in presutgical assessment for temporal lobe epilepsy. Clin. Neurophysiol. 110, 895–904. doi: 10.1016/s1388-2457(99)00039-5
Friedman, D., Honig, L. S., and Scarmeas, N. (2011). Seizures and epilepsy in Alzheimer’s disease. CNS Neurosci. Ther. 18, 285–294. doi: 10.1111/j.1755-5949.2011.00251.x
Gambardella, A., Gotman, J., Cendes, F., and Andermann, F. (1995). Focal intermittent delta activity in patient with mesiotemporal atrophy: a reliable marker of the epileptogenic focus. Epilepsia 36, 122–129. doi: 10.1111/j.1528-1157.1995.tb00970.x
Gennaro, G. D., Quarato, P. P., Onorati, P., Colazza, G. B., Mari, F., Grammaldo, L. G., et al. (2003). Localizing significance of temporal intermittent rhythmic delta activity (TIRDA) in drug-resistant focal epilepsy. Clin. Neurophysiol. 114, 70–78. doi: 10.1016/s1388-2457(02)00332-2
Gureviciene, I., Ishchenko, I., Ziyatdinova, S., Jin, N., Lipponen, A., Gurevicius, K., et al. (2019). Characterization of epileptic spiking associated with brain amyloidosis in APP/PS1 mice. Front. Neurol. 12:1151. doi: 10.3389/fneur.2019.01151
Horvath, A., Szucs, A., Csukly, G., Sakovics, A., Stefanics, G., and Kamondi, A. (2018). EEG and ERP biomarkers of Alzheimer’s disease: a critical review. Front. Biosci. 23, 183–220. doi: 10.2741/4587
Issa, N. P., Wu, S., Rose, S., Towle, V. L., Warnke, P. C., and Tao, J. X. (2018). Small sharp spikes as EEG markers of mesiotemporal lobe epilepsy. Clin. Neurophysiol. 129, 1796–1803. doi: 10.1016/j.clinph.2018.06.011
Kaiboriboon, K., Nagarajan, S., Mantle, M., and Kirsch, H. E. (2010). Interictal MEG/MSI in intractable mesial temporal lobe epilepsy: spike yield and characterization. Clin. Neurophysiol. 121, 325–331. doi: 10.1016/j.clinph.2009.12.001
Kam, K., Duffy, A. M., Moretto, J., LaFrancois, J. J., and Scharfman, H. E. (2016). Interictal spikes during sleep are an early defect in the Tg2576 mouse model of β-amyloid neuropathology. Sci. Rep. 9:20119. doi: 10.1038/srep20119
Khoo, H. M., von Ellenrieder, N., Zazubovits, N., Dubeau, F., and Gotman, J. (2017). Epileptic networks in action: synchrony between distant hemodynamic responses. Ann. Neurol. 82, 57–66. doi: 10.1002/ana.24973
Koessler, L., Cecchin, T., Colnat-Coulbois, S., Vignal, J. P., Jonas, J., Vespignani, H., et al. (2015). Catching the invisible: mesial temporal source contribution to simultaneous EEG and SEEG recordings. Brain Topogr. 28, 5–20. doi: 10.1007/s10548-014-0417-z
Lam, A. D., Cole, A. J., and Cash, S. S. (2019). New approaches to studying silent mesial temporal lobe seizures in Alzheimer’s disease. Front. Neurol. 10:959. doi: 10.3389/fneur.2019.00959
Lam, A. D., Deck, G., Goldman, A., Eskandar, E. N., Noebels, J., and Cole, A. J. (2017a). Silent hippocampal seizures and spikes identified by foramen ovale electrodes in Alzheimer’s disease. Nat. Med. 23, 678–680. doi: 10.1038/nm.4330
Lam, A. D., Maus, D., Zafar, S. F., Cole, A. J., and Cash, S. S. (2017b). SCOPE-mTL: a noninvasive tool for identifying and lateralizing mesial temporal lobe seizures prior to scalp EEG ictal onset. Clin. Neurophysiol. 128, 1647–1655. doi: 10.1016/j.clinph.2017.06.040
Lam, A. D., Sarkis, R. A., Pellerin, K. R., Jing, J., Dworetzky, B. A., Hoch, D. B., et al. (2020). Association of epileptiform abnormalities and seizures in Alzheimer disease. Neurology 20, e2259–e2270. doi: 10.1212/WNL.0000000000010612
Lam, A. D., Zepeda, R., Cole, A. J., and Cash, S. S. (2016). Widespread changes in network activity allow noninvasive detection of mesial temporal lobe seizures. Brain 139, 2679–2693. doi: 10.1093/brain/aww198
Liedorp, M., Stam, C. J., van der Flier, W. M., Pijnenburg, Y. A. L., and Scheltens, P. (2010). Prevalence and clinical significance of epileptiform EEG discharges in a large memory clinic cohort. Dement. Geriatr. Cogn. Disord. 29, 432–437. doi: 10.1159/000278620
Liu, H., Zhang, L., Xi, Q., Zhao, X., Wang, F., Wang, X., et al. (2018). Changes in brain lateralization in patients with mild cognitive impairment and Alzheimer’s disease: a resting-state functional magnetic resonance study from Alzheimer’s disease neuroimaging initiative. Front. Neurol. 9:3. doi: 10.3389/fneur.2018.00003
Malow, B. A., Kushwaha, R., Lin, X., Morton, K. J., and Aldrich, M. S. (1997). Relationship of interictal epileptiform discharges to sleep depth in partial epilepsy. Electroencephalogr. Clin. Neurophysiol. 102, 20–26. doi: 10.1016/s0013-4694(96)96028-9
Milikovsky, D. Z., Ofer, J., Senatorov, V. V. Jr., Friedman, A. R., Prager, O., Sheintuch, L., et al. (2019). Paroxysmal slow cortical activity in Alzheimer’s disease and epilepsy is associated with blood-brain barrier dysfunction. Sci. Transl. Med. 11:eaaw8954. doi: 10.1126/scitranslmed.aaw8954
Musaeus, C. S., Engedal, K., Hogh, P., Jelic, V., Morup, M., Naik, M., et al. (2018). EEG theta power is an early marker of cognitive decline in dementia due to Alzheimer’s disease. J. Alzheimers Dis. 64, 1359–1371. doi: 10.3233/JAD-180300
Naftulin, J. S., Ahmed, O. J., Piantoni, G., Eichenlaub, J. B., Martinet, L. E., Kramer, M. A., et al. (2018). Ictal and preictal power changes outside of the seizure focus correlate with seizure generalization. Epilepsia 59, 1398–1409. doi: 10.1111/epi.14449
Nayak, D., Valentin, A., Alarcon, G., Garcia Seoane, J. J., Brunnhuber, F., Juler, J., et al. (2004). Characteristics of scalp electrical fields associated with deep medial temporal epileptiform discharges. Clin. Neurophysiol. 115, 1423–1435. doi: 10.1016/j.clinph.2004.01.009
Noachtar, S., and Remi, J. (2009). The role of EEG in epilepsy: a critical review. Epilepsy Behav. 15, 22–33. doi: 10.1016/j.yebeh.2009.02.035
Noebels, J. (2011). A perfect storm: converging paths of epilepsy and Alzheimer’s dementia intersect in the hippocampal formation. Epilepsia 52(Suppl. 1), 39–46. doi: 10.1111/j.1528-1167.2010.02909.x
Normand, M. M., Wszolek, Z. K., and Klass, D. W. (1995). Temporal intermittent rhythmic delta activity in electroencephalograms. J. Clin. Neurophysiol. 12, 280–284. doi: 10.1097/00004691-199505010-00005
Oishi, M., Otsubo, H., Kameyama, S., Morota, N., Masuda, H., Kitayama, M., et al. (2002). Epileptic spikes: magnetoencephay versus simultaneous electrocorticography. Epilepsia 43, 1390–1395. doi: 10.1046/j.1528-1157.2002.10702.x
Palop, J. J., and Mucke, L. (2009). Epilepsy and cognitive impairments in Alzheimer disease. Arch. Neurol. 66, 435–440. doi: 10.1001/archneurol.2009.15
Palop, J. J., and Mucke, L. (2016). Network abnormalities and interneuron dysfunction in Alzheimer disease. Nat. Rev. Neurosci. 17, 777–792. doi: 10.1038/nrn.2016.141
Pandis, D., and Scarmesa, N. (2012). Seizures in Alzheimer disease: clinical and epidemiological data. Epilepsy Curr. 12, 184–187. doi: 10.5698/1535-7511-12.5.184
Pizzo, F., Roehri, N., Medina Villalon, S., Trebuchon, A., Chen, S., Lagarde, S., et al. (2019). Deep brain activities can be detected with magnetoencephalography. Nat. Commun. 10:971. doi: 10.1038/s41467-019-08665-5
Pooler, A. M., Phillips, E. C., Lau, D. H., Noble, W., and Hanger, D. P. (2013). Physiological release of endogenous tau is stimulated by neuronal activity. EMBO Rep. 14, 389–394. doi: 10.1038/embor.2013.15
Reiher, J., Beaudry, M., and Leduc, C. P. (1989). Temporal intermittent rhythmic delta activity (TIRDA) in the diagnosis of complex partial epilepsy: sensitivity, specificity and predictive value. Can. J. Neurol. Sci. 16, 398–401. doi: 10.1017/s0317167100029450
Reyes-Marin, K. E., and Nunez, A. (2017). Seizure susceptibility in the APP/PS1 mouse model of Alzheimer’s disease and relationship with amyloid β plaques. Brain Res. 15, 93–100. doi: 10.1016/j.brainres.2017.09.026
Sammaritano, M., Gigli, G. L., and Gotman, J. (1991). Interictal spiking during wakefulness and sleep and the localization of foci in temporal lobe epilepsy. Neurology 41, 290–297. doi: 10.1212/wnl.41.2_part_1.290
Sarkis, R. A., Dickerson, B. C., Cole, A. J., and Chemali, Z. N. (2016). Clinical and neurophysiologic characteristics of unprovoked seizures in patients diagnosed with dementia. J. Neuropsychiatry Clin. Neurosci. 28, 56–61. doi: 10.1176/appi.neuropsych.15060143
Scarmeas, N., Honig, L. S., Choi, H., Cantero, J., Brandt, J., Blacker, D., et al. (2009). Seizures in Alzheimer disease: who, when, and how common? Arch. Neurol. 66, 992–997. doi: 10.1001/archneurol.2009.130
Sheth, S. A., Aronson, J. P., Shafi, M. M., Phillips, H. W., Velez-Ruiz, N., Walcott, B. P., et al. (2014). Utility of foramen ovale electrodes in mesial temporal lobe epilepsy. Epilepsia 55, 713–724. doi: 10.1111/epi.12571
Sperling, M. R., Mendius, J. R., and Engel, J. Jr. (1986). Mesial temporal spikes: a simultaneous comparison of sphenidal, nasopharyngeal, and ear electrodes. Epilepsia 27, 81–86. doi: 10.1111/j.1528-1157.1986.tb03505.x
Spyrou, L., Martin-Lopez, D., Valentin, A., Alarcon, G., and Sanei, S. (2016). Detection of intracranial signatures of interictal epileptiform discharges from concurrent scalp EEG. Int. J. Neural Syst. 26:1650016. doi: 10.1142/S0129065716500167
Stomrud, E., Hansson, O., Minthon, L., Blennow, K., Rosen, I., and Londos, E. (2010). Slowing of EEG correlates with CSF biomarkers and reduced cognitive speed in elderly with normal cognition over 4 years. Neurobiol. Aging 31, 215–223. doi: 10.1016/j.neurobiolaging.2008.03.025
Thompson, P. M., Hayashi, K. M., Zubicaray, G. D., Janke, A. L., Rose, S. E., Semple, J., et al. (2003). Dynamics of gray matter loss in Alzheimer’s disease. J. Neurosci. 23, 994–1005. doi: 10.1523/JNEUROSCI.23-03-00994.2003
Tyvaert, L., LeVan, P., Dubeau, F., and Gotman, J. (2009). Noninvasive dynamic imaging of seizures in epileptic patients. Hum. Brain Mapp. 30, 3993–4011. doi: 10.1002/hbm.20824
Vossel, K. A., Beagle, A. J., Rabinovici, G. D., Shu, H., Lee, S. E., Naasan, G., et al. (2013). Seizures and epileptiform activity in the early stages of Alzheimer disease. JAMA Neurol. 70, 1158–1166. doi: 10.1001/jamaneurol.2013.136
Vossel, K. A., Ranasinghe, K. G., Beagle, A. J., Mizuiri, D., Honma, S. M., Dowling, A. F., et al. (2016). Incidence and impact of subclinical epileptiform activity in Alzheimer’s disease. Ann. Neurol. 80, 858–870. doi: 10.1002/ana.24794
Vulliemoz, S., Carmichael, D. W., Rosenkranz, K., Diehl, B., Rodionov, R., Walker, M. C., et al. (2011). Simultaneous intracranial EEG and fMRI of interictal epileptic discharges in humans. Neuroimage 54, 182–190. doi: 10.1016/j.neuroimage.2010.08.004
Wennberg, R., and Cheyne, D. (2014). Reliability of MEG source imaging of anterior temporal spikes: analysis of an intracranially characterized spike focus. Clin. Neurophysiol. 125, 903–918. doi: 10.1016/j.clinph.2013.08.032
Wennberg, R., Valiante, T., and Cheyne, D. (2011). EEG and MEG in mesial temporal lobe epilepsy: where do the spikes really come from? Clin. Neurophysiol. 122, 1295–1313. doi: 10.1016/j.clinph.2010.11.019
Wessa, M., King, A. V., Meyer, P., Frolich, L., Flor, H., Poupon, C., et al. (2016). Impaired and preserved aspects of feedback learning in aMCI: contributions of structural connectivity. Brain Struct. Funct. 221, 2831–2846. doi: 10.1007/s00429-015-1075-y
White, J. C., Langston, J. W., and Pedley, T. A. (1977). Benign epileptiform transients of sleep. Clarification of the small sharp spike controversy. Neurology 27, 1061–1068. doi: 10.1212/wnl.27.11.1061
Wu, J. W., Hussaini, S. A., Bastille, I. M., Rodriguez, G. A., Mrejeru, A., Rilett, K., et al. (2016). Neuronal activity enhances tau propagation and tau pathology in vivo. Nat. Neurosci. 19, 1085–1092. doi: 10.1038/nn.4328
Yang, C., Zhong, S., Zhou, X., Wei, L., Wang, L., and Nie, S. (2017). The abnormality of topological asymmetry between hemispheric brain white matter networks in Alzheimer’s disease and mild cognitive impairment. Front. Aging Neurosci. 9:261. doi: 10.3389/fnagi.2017.00261
Keywords: Alzheimer’s disease, epileptogenesis, seizure, electrophysiology, biomarkers
Citation: Yu T, Liu X, Wu J and Wang Q (2021) Electrophysiological Biomarkers of Epileptogenicity in Alzheimer’s Disease. Front. Hum. Neurosci. 15:747077. doi: 10.3389/fnhum.2021.747077
Received: 25 July 2021; Accepted: 01 November 2021;
Published: 30 November 2021.
Edited by:
Henning Müller, University of Applied Sciences and Arts of Western Switzerland, SwitzerlandReviewed by:
Anna Szucs, Semmelweis University, HungaryAngel Nunez, Autonomous University of Madrid, Spain
Qing Yun Wang, Beihang University, China
James Tao, University of Chicago, United States
Copyright © 2021 Yu, Liu, Wu and Wang. This is an open-access article distributed under the terms of the Creative Commons Attribution License (CC BY). The use, distribution or reproduction in other forums is permitted, provided the original author(s) and the copyright owner(s) are credited and that the original publication in this journal is cited, in accordance with accepted academic practice. No use, distribution or reproduction is permitted which does not comply with these terms.
*Correspondence: Qun Wang, d2FuZ3FAY2NtdS5lZHUuY24=