- Sensory-Motor Lab (SeMoLa), Department of Ophthalmology-University of Lausanne, Jules Gonin Eye Hospital-Fondation Asile des Aveugles, Lausanne, Switzerland
Vision is the main entrance for environmental input to the human brain. Even if vision is our most used sensory modality, its importance is not limited to environmental exploration. Rather it has strong links to motor competences, further extending to cognitive and social aspects of human life. These multifaceted relationships are particularly important in developmental age and become dramatically evident in presence of complex deficits originating from visual aberrancies. The present review summarizes the available neuropsychological evidence on the development of visual competences, with a particular focus on the associated visuo-motor integration skills in health and disease. With the aim of supporting future research and interventional settings, the goal of the present review is to constitute a solid base to help the translation of neuropsychological hypotheses into straightforward empirical investigations and rehabilitation/training protocols. This approach will further increase the impact, ameliorate the acceptance, and ease the use and implementation of lab-derived intervention protocols in real-life situations.
Neural Correlates of Vision
Visual perception permeates our life, not only for merely gathering information about the environment, but also for having important influence on our motor skills. Revealing the neural mechanisms of the multifaceted relationships between vision and other domains of human life, visual neuropsychology goes beyond the traditional consideration of vision as a passive function, and rather highlights how visual competences can impact typical and atypical development at a more systemic, dynamic, and integrated level. The neurobiological machinery that brings from light to vision starts in the eyes, where the photoreceptors of the retina are able to selectively respond to the photons of light (entered through the cornea and projected to the retina) and “translate” them into neural signals. These signals are transported by the optic nerves to subcortical structures (the lateral geniculate and pulvinar nuclei of the thalamus) which relay signals mainly to the visual cortex, in the posterior part of the brain, but also to the superior colliculus in the midbrain (Shipp, 2004). The occipital lobe is further organized in several sub-regional areas, including the striate primary visual cortex (V1) and a series of interconnected, extra-striate, and progressively more specialized areas for higher-level processing of visual input (Figure 1). Thus, while V1 is sensitive to basic features of the visual input, such as line orientation, motion direction, and depth perception, the secondary visual cortex (V2) receives fibers from V1, projects to the third visual cortex (V3), and is already able to perform figure/background distinctions (Qiu and Von Der Heydt, 2005; Maruko et al., 2008), to process illusory contours (Von Der Heydt et al., 1984; Anzai et al., 2007), and to build binocular disparity (Von Der Heydt et al., 2000). V3 projects to areas out of the occipital lobe, including the posterior parietal cortex (Stepniewska et al., 2016) and the inferior temporal cortex (Ponce et al., 2017), and is sensitive to global motion (Braddick et al., 2001), covering larger portions of the visual field with respect to V1 (Lui et al., 2006). The fourth visual cortex (V4) it tightly connected to V1 and V2 (Liu et al., 2020) and projects mainly to the inferior temporal cortex (Bohon et al., 2016). It is involved in color perception, object recognition, and is sensitive to top-down attentional modulation (Roe et al., 2012). The fifth visual cortex (V5) receives input from V1, V2, V3, as well as from the thalamus (Ungerleider and Desimone, 1986; Felleman and Van Essen, 1991; Sincich et al., 2004; Warner et al., 2010) and projects to the superior temporal gyrus (Handa et al., 2017; Handa and Mikami, 2018), the frontal eye fields (Machner et al., 2010) and lateral intraparietal cortex (De Azevedo Neto and Amaro Junior, 2018). Some fibers reach V5 directly from the thalamus, bypassing V1 (Warner et al., 2012). Encoding speed and direction of visual input (Dubner and Zeki, 1971; Maunsell and Van Essen, 1983), V5 is mostly important for motion perception and smooth guidance of eye movements (Dursteler et al., 1987) as well for “building” a continuous perception of moving targets and scenes instead of a “crystallized” vision of distinct frames (Hess et al., 1989; Baker et al., 1991). The sixth visual cortex (V6) is located medially and connected to parietal and pre/post-central regions (Shipp et al., 1998; Galletti et al., 2001; Luppino et al., 2005; Smith et al., 2018; Serra et al., 2019) of the brain is responsible for “subtracting out” the visual input related to self-motion from the rest of the visual perception (Pitzalis et al., 2013), as well as for visually guiding movements (Pitzalis et al., 2015).
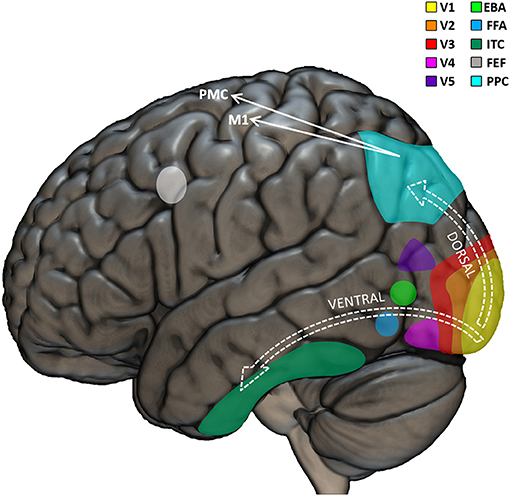
Figure 1. Visual neuropsychological model. Graphical representation of the main cortical regions involved in visual perception and visuo-motor coordination. The visual input is first processed by the primary visual cortex (V1). Further processing is performed by the extrastriate visual regions (V2, V3, V4, and V5) which triggers the recruitment of the dorsal or ventral stream as a function of whether or not the visual input needs to be used to perceive or move in the environment, respectively. EBA, extrastriate body area; FFA, fusiform face area; ITC, inferior temporal cortex; FEF, frontal eye field; PPC, posterior parietal cortex; M1, primary motor cortex; PMC, premotor cortex.
Traditional neuropsychological models of visual perception indicate that the several interconnections among the visual regions of the brain can be broadly classified according to two functionally different main streams: the well-known ventral and dorsal streams (Tong, 2003). The “what” ventral stream would pass signals from V1, V2, V3, V4, up to the inferior temporal cortex and would be implied mainly in object recognition Conversely, the “where” dorsal stream would comprise connections between V1, V2, V3, superior/medial temporal sulcus, and parietal cortex and would be particularly important for neurally encoding the visuo-spatial and motion-related aspects of visual input (Hickok and Poeppel, 2004; Almeida et al., 2010; De Haan and Cowey, 2011; Goodale, 2013). Lesions in the ventral stream produce recognition deficits such as prosopagnosia (the impossibility to recognize faces) (Mayer and Rossion, 2007). Lesions in the dorsal stream determine visuo-motor deficits, such as optic ataxia (impaired visuo-motor coordination, e.g., impossibility to reach objects despite preserved visual and motor skills separately) (Himmelbach et al., 2009). This sharp dichotomy between ventral and dorsal streams has been progressively smoothened (Rossetti et al., 2017), including the identification of bidirectional interactions between the streams (Greulich et al., 2020), especially in the context of adaptive behavior (Goodale et al., 2005) and visuo-motor skills (Van Polanen and Davare, 2015). Interestingly, different visuo-motor sub-pathways have been identified in the dorsal stream: the dorso-dorsal stream would be recruited for online action control; the ventro-dorsal stream would have be involved in higher-level cognitive functions including action understanding (Rizzolatti and Matelli, 2003). Altogether, it seems clear that the fine precision of our visual skills and their importance in action-related mechanisms are reflected in the high complexity of the involved neural architecture.
Neuro-Behavioral Developments of Visual Skills
Taking into consideration the temporal aspects of the development of the visual streams, it appears that the pace at which the ventral and the dorsal streams grow would be different already in pre-born age, indicating that the ventral stream would mature more quickly than the dorsal stream (Tadros et al., 2015). Indeed, already at birth the ability of newborns to notice that a visual event occurs, even if the classification of “what” that even is still need further cortical maturation, has been considered an example of the importance of subcortico-cortical visual functions (Bronson, 1974). In addition, the fact that newborns are particularly attracted by face-like visual stimuli (Simion et al., 2011) and, especially, by familiar faces (Bushneil et al., 1989), suggests the functioning of thalamo-V5 connections which would bypass V1. Then, during the first 6 months, more specific functions associated with neural activity in V1 progressively emerge in an ordered sequence. The first functions are sensitivity to orientation, followed by the ability to perceive directional motion, and finally binocular interactions, e.g., for depth perception (Braddick and Atkinson, 2011).
Basic Functions
Visual Acuity
Visual acuity refers to our ability to perceive fine visual details. To reach adult levels, visual acuity rapidly develops during the first months of life and keeps improving up to 3 or 4 years (Norcia and Tyler, 1985; Banks and Dannemiller, 1987). Together with visual acuity, at birth also contrast sensitivity (the ability to discriminate light and dark) is below adult levels and progressively improves during the first months (Banks and Salapatek, 1978). From an ecological perspective, newborns don't need to perceive small details of far objects, but rather they need to recognize persons relevant for them (e.g., parents). Thus, newborns' relatively low visual acuity anyways allows them to efficiently interact with the environment relevant at their scale, even if their visual performance would be rated as “blindness” according to adult scales. Their low visual acuity might derive from the immaturity of foveal photoreceptors, retina, and eye-brain pathways, which quickly develop in early life, including denser concentration and better sensitivity of photoreceptors (Yuodelis and Hendrickson, 1986) and larger neural sprouting between the eyes and the brain (Braddick and Atkinson, 2011).
Vernier Acuity
The simple visual acuity provides the means to perceive small details, but it does not take into account the spatial relationship between such details. This capacity is defined “vernier acuity” and refers to the ability to perceive spatial incongruence, e.g., misalignment, with a resolution even higher than simple visual acuity. The behavioral aspects of vernier acuity have been long known, since the seminal work by Ewald Hering at the end of the nineteenth century (Strasburger et al., 2018). As it goes beyond the physical features of the eye, the vernier acuity is one of the examples of the importance of cortical dynamics in supporting visual skills (Manny, 1988; Skoczenski and Norcia, 1999). Indeed it progressively improves faster than the simple visual acuity (Zanker et al., 1992; Brown, 1997), in parallel with the maturation of the cortico-subcortical networks responsible for the integration of spatial relationships between different objects and their parts, and it reaches maturity much later than simple acuity (Skoczenski and Norcia, 2002). Interestingly, Braille reading triggers a progressive improvement of vernier acuity on tactile perception (Loomis, 1979), suggesting the influence of neuroplastic changes driven by specific habits.
Accommodation
Both visual acuity and vernier acuity depend on accommodation, the possibility to adapt the focus to the distance of the object by contracting or relaxing the muscles of the eye lens. Accommodation at birth is limited to objects located within a range of 40–50 cm (Horwood and Riddell, 2008), with a possibly parallel ongoing attentional limitation (Downey et al., 2017). At the neural level, accommodation is associated with brain activity in the visual cortex (Mirzajani et al., 2017) and relies on an extended cortico-cerebellar network, including links of the visual cortex with cerebellar hemispheres/vermis and temporal cortex (Richter et al., 2000), as well as with precentral and frontal regions (Lv et al., 2020).
Color
All visual functions would not reflect the real world if they would not comprise information about colors. Color perception is strongly based on the early activity of cone receptors in the eyes from the first months of life (Brown, 1990). It has roots in the development of cortical functions starting from V2 and V3 (Ting Siok et al., 2009) to allow the proper use of color information including, for example, high-level functions such as emotion (Yoto et al., 2007) or aesthetics (Maglione et al., 2017).
Integrative Functions
Contours and Motion
One the most important information about the environment refers to the contours of an object: where an object ends and another one or the background start. This type of information is not present in the raw visual input, but it is rather built by neural responses in V1 (Hubel and Wiesel, 1977). Newborns start to discriminate orientation and therefore contours within the first weeks (Slater et al., 1988), even before visual event related potentials (VERPs; the stereotyped brain response to a standardized basic visual input) associated with contours can be recorded from their brains, between 3 and 8 weeks from birth (Braddick et al., 1986). Considering the importance of sensitivity to contours in development (Candy et al., 2001), VERPs can be fundamental measures for early detection of at-risk populations (Atkinson et al., 2008). Another fundamental information to efficiently understand the environment is the ability to distinguish static and moving objects. Like contours, motion sensitivity is the result of a cortical construction based on the neural responses in the visual cortices. Usually, newborns start to perceive motion a bit earlier than 10 weeks (Braddick et al., 2003), when their VERPs start to be detectable (Wattam-Bell, 1991). The anatomical maturation necessary to discriminate contours and motion occurs in the first months of life, depending on neural sprouting and synaptic establishment (Huttenlocher et al., 1982). The functional development of sensitivity to orientation and motion (plus binocularity) occurs in sequential order and based on neurally distinct pathways. For example, even if newborns show some degrees of motion sensitivity in relatively early stages, this ability relies mostly on sub-cortical structures (Morrone et al., 1999).
Global Motion
The perception of motion alone does not account for the complexity of the environment, where different objects can move at a different pace and/or according to different spatio-temporal patterns. The capacity to perceive such a complexity seems to appear already between the first 2–6 months of life (Kellman and Spelke, 1983; Arterberry and Yonas, 2000; Johnson and Mason, 2002), with a specific ability for the recognition of human action-like motion, or biological motion (Booth et al., 2002). Such a relatively late development together with the need to convey information from larger areas of the visual field suggest that global motion skills rely on a neural architecture starting in V1 (Robertson et al., 2014) and further extending to a broader brain network (Koyama et al., 2005), likely encompassing V2 and V3 (Furlan and Smith, 2016), as well as V5 (Giaschi et al., 2007) and possibly also more frontal motor regions (Saygin et al., 2004; Wuerger et al., 2012). Already in about 5-year-old children, the perception of global motion is independent from simple visual acuity (Chakraborty et al., 2015) and is strictly related to visuo-motor skills (Chakraborty et al., 2017). In addition, sensitivity to global motion is neurally dissociable from global form perception (Vachon et al., 2009), possibly being linked to interregional neural connections (Pavlova et al., 2005). A direct way to assess global motion skills is the so-called “motion coherence sensitivity” test (Newsome and Pare, 1988), which evaluates the ability to recognize target motion patterns within a background of differently moving dots. During the first months of life, motion coherence sensitivity increases progressively (Mason et al., 2003), the associated VERPs show specificity for global motion (Wattam-Bell et al., 2010), and V5 is selectively activated by global motion in connection with other motion-related areas (Biagi et al., 2015).
Static Forms vs. Global Motion
There seem to be a clear segregation between sensitivity to global motion and static forms, as shown by the earlier readiness of VERPs associated to global motion than to static forms (Wattam-Bell et al., 2010), the larger variability of thresholds for global motion than for static forms (Braddick et al., 2016), and qualitative difference between global motion and static forms skills ranging up to adolescence (Meier and Giaschi, 2014). At the neural level, while sensitivity to global motion seems to recruit mainly the dorsal stream, the perception of static forms is mainly bound to the ventral stream. Accordingly, only the development of global motion perception (not static forms) correlates with anatomo-functional growth of neural connections between the parietal and frontal lobes, beyond the solely visual cortex (Braddick et al., 2016, 2017). This suggests that global motion perception is a higher-order function recruiting also sensorimotor integration mechanisms. Such a conclusion is further supported by the observation that the performance in global motion (not static forms) correlates with visuo-motor skills already in developmental age (Braddick et al., 2016) and that aberrancies in its parieto-frontal neural architecture might be at the origin of the so-called “dorsal stream vulnerability” in developmental deficits (Spencer et al., 2000).
3D and Depth Perception
The ability to merge and coordinate information from the two perspectives of each eye provides one of the means to perceive three-dimensionality and is a peculiar cortical function, not happening in earlier levels of the visual input's processing. In children, binocularity emerges at about 3 months (Thorn et al., 1994), depending on ocular convergence (Downey et al., 2017), cortical maturation (Elberger and Smith, 1985), neural plasticity (Chalupa, 2004), specific neurotransmitters (Kameyama et al., 2010; Krahe and Medina, 2010), and cortico-cortical interactions, both in humans (Jurcoane et al., 2007) and other mammals (Dehmel and Lowel, 2014). The failure of one or more of these cortical mechanisms can contribute to the creation of the conditions for developing binocularity-related functional deficits, such as strabismus (Berman and Murphy, 1981; Freeman et al., 1982; Di Stefano and Gargini, 2002). Together with binocularity, depth perception relies on a number of visual abilities, including shape/shade segregation, sensitivity to differential texture density, interposition of near/far surfaces, all of which start to be present between 4 and 7 months (Yonas et al., 2002), and it keeps progressing in parallel with the development of fine visuo-motor skills both in health (Braddick and Atkinson, 2013) and disease (Grant et al., 2014). A specific function tightly linked to depth perception is the ability to identify an object with respect to its background. Such a figure-ground discrimination can be based, for example, on the sensitivity to different textures between the object and the background which starts to emerge in the first month of child's life (Brooks and Clair, 1971; Wattam-Bell, 1992), keeps evolving up to adultness (Anderson et al., 2016), and is sensitive to age-related ocular diseases like macular degeneration (Tran et al., 2011). A related effect refers to the perception of the so-called “illusory contours,” proper visual illusions inducing the illusory perception of edges without physical borders, as shown by the pioneering work by Gaetano Kanisza and his famous illusory triangle (Kanisza, 1955). Children start to be sensitive to the Kanisza triangle in the first 3–5 months of life (Kavšsek, 2002; Otsuka and Yamaguchi, 2003), with increasing sensitivity up to adolescence (Bondarenko et al., 2010), possibly in parallel with improved cortico-cortical interactions (Ffytche and Zeki, 1996), increased intracortical dynamics in V2 and in V2-V5 exchanges (Grossberg, 2014), and exchanges between the different compartments of the visual cortex (Weigelt, 2007). Altogether, bidirectional interactions seem to be in place between children's improvements in perceiving basic visual features and their developments in higher-level functions beyond the mere visual perception. Such interactions typically start in the first month of life (Granrud, 2006) and keep evolving up to about 10 years (Nardini et al., 2010; Dekker et al., 2015).
Face Perception
The human face is probably the most salient visual stimulus in our life. The evolutionary and adaptive importance of the recognizing, understanding, and interpreting human faces is demonstrated by the existence of a region in the extrastriate cortex of the human brain, specifically dedicated to processing face-related visual input: the fusiform face area or FFA (Kanwisher et al., 1997). Behavioral evidence shows that sensitivity to faces is one of the first visual abilities in newborns (Goren et al., 1975; Ferrari et al., 1986), is based on contrast polarity (Rosa Salva et al., 2012), can be functional even with a relatively low resolution at short distances (Von Hofsten et al., 2014), and can be shaped by life experience (Cobbett and Snelgrove-Clarke, 2016). At the neural level, the early appearance of the strong bias toward faces suggests that it is based on subcortical mechanisms aiding the newborn to fixate a face which in turn would favor a frequent exposure to faces and the associated development of selective cortical processing. This idea is supported by the supposed presence of visual pathways which would allow face perception by directly connecting the thalamus and the amygdala to FFA, bypassing V1 in both the human (Morris et al., 2001) and the primate brain (Bourne and Morrone, 2017). Despite cortical electrophysiology suggests that the FFA is sensitive to observation of faces already at 4 months (De Heering and Rossion, 2015), face-related neural response (De Haan et al., 2003) and cortical specialization (Peelen et al., 2009; Deen et al., 2017) seem less pronounced in children than adults.
Body Perception
Together with face perception, also the visual perception of the human body plays a crucial role in daily life. Similarly to the fusiform face area, and in obvious anatomical closeness, the extrastriate body area (EBA) is functionally-defined brain regions, specifically sensitive to the observation of the human body (Downing et al., 2001) and part of the lateral occipitotemporal cortex possibly overlapping with V5 (Ferri et al., 2013). The inhibition (Urgesi et al., 2007; Candidi et al., 2008) or lesion (Peelen and Downing, 2007) of EBA support its causal implication in selectively respond to the observation of human bodies. Not only is EBA important for body-related visual processing, but also it is involved in higher-level visual cognition related to the human body, including identity attribution (Myers and Sowden, 2008), emotional resonance (Ionta et al., 2020), and mental imagery (Arzy et al., 2006; Costantini et al., 2011; Perruchoud et al., 2016). Even if the development of EBA in the life span remains largely unexplored, recent evidence suggests that the development of the body-specific responsiveness of EBA can take several years. Indeed neuro-functional differences of EBA can be noticeable between 6 and 8 vs. 9–12 year-old children (Walbrin et al., 2020), and the development of EBA can be affected by neurological disorders in early age (Okamoto et al., 2017).
Visuo-Motor Interactions
Moving is one of the most direct and evolutionary relevant reason to have vision. Thanks to movements we can preserve our body, and therefore our life, for example by escaping dangers and reaching targets. Even if these functions can be technically possible also without vision, in typical conditions human beings are historically hardwired to vision. Therefore, it is not surprising that a large part of visual functions is subsequently used to control movements, as suggested by tight visuo-motor resonance already in early age (Lepage and Théoret, 2007). The neural architecture for such visuo-motor couplings would be present already at birth (Meltzoff and Moore, 1977) and it would be promoted by the repeated exposure to sensorimotor events (Cook et al., 2014), being its development further depending on experience (Simpson et al., 2014).
Eye and Head Movements
The first visuo-motor interactions in the newborns comprise eye and head movements, followed by postural adjustments, manual exploration, and locomotion (Adolph and Franchak, 2017). Eye movements constitute a fundamental visuo-motor interaction, combining the perceived changes from the environment (vision) with a rudimental motor reaction (eye movements). The superior colliculus in the midbrain plays a central role in such rudimental visuo-motor interactions, initiating the saccades (Hainline et al., 1984) and being connected to cortico-subcortical circuits to disengage fixation during saccades (Braddick et al., 1992). The fixation disengagement reaches functional maturity between 2 and 5 months (Hood and Atkinson, 1993), possibly reflecting the maturation of frontal cortical regions (Csibra et al., 1998). In order to track moving objects, it is necessary to (i) stabilize the target image on the retina and (ii) follow its displacements. The retinal stabilization can be achieved thanks to the optokinetic nystagmus, which subsequently needs to be inhibited in order to smoothly pursue the target's movements. In newborns, noting such ability depends on the features of the target, with smooth pursuit movements exhibited even at a few weeks of life, but only with slow and large moving targets (Phillips et al., 1997). The fact that infants can also anticipate where a moving target will go by staring at the expected location, supports that rudimental cortical mechanisms for early visuo-motor interactions are already in place in early age (Rosander, 2007).
Eye-Hand Coordination
It is not a secret that the ability to grasp objects contributed fundamentally to render humans one of the most evolutionary successful species worldwide. Grasping is the result of complex interactions between sensory perceptions and motor control, the largest part being taken by the coordination between vision and hand movements. Such an eye-hand coordination widely permeates daily life, including object manipulation, environmental exploration, and social interaction. Without tight eye-hand links, it is doubtful that fundamental human activities like writing or driving (often given for granted, but in fact not obvious), would have evolved at such a large scale, or perhaps they would not have born at all. The existence of visuo-motor links is supported by both behavioral and neural evidence, suggesting the interaction between the ventral and the dorsal streams. Early forms of reaching and grasping emerge around the fourth month of life, supporting that the dorsal stream would be already able to coordinate the motor output in response to the visual input mediated by the ventral stream (Braddick et al., 2003). Between the sixth and ninth month, children almost compulsorily reach and grasp any object within their arm's length (Newman et al., 2001), establishing and reinforcing rich perceptual-motor connections which will constitute scaffold for developing a broad visuo-motor neural architecture able to be activated even by less complex inputs (Pulvermüller, 1999; Martin et al., 2000). For example it has been shown that even just the observation of reaching movements activates the sensorimotor cortex in 14-month-old children with a stronger gradient as a function of older ages (Marshall et al., 2011). Similarly, visual perception of letters is associated with brain activations typical for the execution of handwriting movements (Longcamp et al., 2003), which in turn activate also visual regions typically involved in letter perception (James and Gauthier, 2006). Beyond action execution, vision can contribute also to accurate action planning, including the ability to anticipate the appropriate hand configuration to grasp a specific object (Rosenbaum et al., 1992). Typically, this ability is achieved at about 8 years (Smyth and Mason, 1997), but some delays can be encountered in presence of clinical conditions that are likely affect the interactions between the dorsal stream, ventral stream, and frontal brain areas (Braddick and Atkinson, 2013).
Not only can vision guide movements, but also motor training can affect visual perception. At the behavioral level, visuomotor training improves letter recognition in 5-year-old children (Bara and Bonneton-Botte, 2018). Similarly, handwriting improves after haptic (not visual) exploration of letters even at younger age (Bara et al., 2004), and is associated with better visual recognition of letters with respect to typing (Longcamp et al., 2005) and with better reading in general (Labat et al., 2010). At the neural level, in addition to the anatomo-functional overlap of brain regions activated by “seeing” and “doing” movements (Halje et al., 2015), already in 9-month-old children the motor components of the brain activity associated to observation of reaching actions occur earlier than the associated visual components (Southgate et al., 2009). This supports the existence of visuo-motor anticipation mechanisms based on experience-driven action understanding (Southgate et al., 2010). In addition to reaching and grasping, locomotion occupies an important position in visuo-motor coordination. In typically developing children locomotion emerges around the first year of life, strongly based on the ability of vision to provide information about the target position, possible obstacles, variations of surfaces, and edges. Thus, vision must have tight links also to the neural correlates of locomotion. Indeed already the simple observation of other children crawling or walking activates the sensorimotor cortex in 7–9 month-old children (De Klerk et al., 2015) as well as more frontal motor brain regions controlling locomotion in 14–16 month-old children (Van Elk et al., 2008), a resonance mechanism that persists in adulthood even just imagining to walk (Ionta et al., 2010).
Visuo-Motor Neuropsychology
Conceiving bio-computational models to explain the causal link between dysfunctional neural networks and clinical phenotypes is the major challenge in neuropsychology. The following sessions offer an overview of the most common visual and visuo-motor disorders, including the possible associated neural explanations. Broadly, the following disorders have been classified as “lower” and “higher” level deficits, even if such a sharp distinction might not reflect all the details of each disorder. The “lower-level” classification comprises disorders mostly affecting the perceptual level, with a high importance of basic mechanisms associated with eye movements and convergence. The “higher-level” classification comprises disorders affecting levels beyond visual perception and rather extending to other spheres of human competences, such as motor and cognitive skills, eventually in absence of other possibly coherent deficits.
Lower-Level Dysfunctions
Strabismus
Strabismus is one of the most common visual disorders, affecting the ability to maintain the alignment between the two eyes and therefore causing a binocularity breakdown due to the mismatch of the information provided by each eye to the visual cortex (Cullen, 2015). The importance of cortical mechanisms in the onset of strabismus is shown by the fact that, at least in monkeys, a lack of intervention at the cortical level can nullify the benefits brought by surgical treatment of the eye muscles (Pullela et al., 2018). Both in humans and other mammals, already the first weeks of life are fundamental for a proper oculomotor development leading to accurate eyes alignment (Tychsen, 2007). Due to the immaturity of V2 neurons with respect to V1 neurons, in the infant brain abnormal visual input can dramatically affect the neural wiring especially in V2 (Nakatsuka et al., 2007), the maturation of which could be misled by inappropriate experience/stimulation (Zheng et al., 2007). On this basis and in combination with the above-mentioned tight visuo-motor links, it is not surprising that the incongruent input received by V1 from the two eyes triggers a cascade of neural events ending in incongruent motor commands sent from to the oculomotor brain centers (e.g., the superior colliculus) back to the eyes (Das, 2016; Walton et al., 2017). Thus, the differential visual input of each eye would contribute to the misalignment of the eyes themselves, as supported by the inextricable relationship between sensory input and motor output (Perruchoud et al., 2014), including evidence that the onset of strabismus can derive from aberrant visual input (Chino et al., 1997). In addition to such aberrancies in the visual cortex, also disturbances in other brain areas have been linked to strabismus, such as abnormal visual-oculomotor behaviors in presence of dysfucntions in V5 and superior temporal gyrus (Mustari et al., 2008; Mustari and Ono, 2011), as well as other neuroanatomical aberrancies affecting the ventricles and corpus callosum (Ohtsuki et al., 2000). Such breakdowns in the visuo-motor loop can impair the perception of depth and also contribute to the onset of e.g., amblyopia (Sengpiel and Blakemore, 1996; Niechwiej-Szwedo et al., 2019).
Oculomotor Apraxia
Optic apraxia refers to the impossibility to perform eye movements, resulting in the so-called “sticky” vision: the impossibility to voluntarily shift gaze between different objects (Pena-Casanova et al., 1985). At the neural level, bilateral lesions in a fronto-parietal network comprising the frontal eye fields are considered at the origin of oculomotor apraxia, which therefore would not be necessarily associated strictly with dorsal stream damage (Leigh and Zee, 2015), extending to malformations/dysfunctions in the cerebellum (Shahwan et al., 2006) and midbrain (Jissendi-Tchofo et al., 2009; Merlini et al., 2010). In children, oculomotor apraxia can be present already around the 10th year of life (Tsao and Paulson, 2005), with a mean age of about 7 years and comprised between 2 and 18 years (Le Ber et al., 2003). Anatomo-functional aberrancies of the cerebellum have been repeatedly associated with oculomotor apraxia (Maria et al., 1999; Gleeson et al., 2004), with a particular responsibility for a too small cerebellar vermis (Sargent et al., 1997). The consequences of oculomotor apraxia do not remain limited to the visual domain, but rather spread on cognitive and social skills, especially in the case that oculomotor abilities are recovered too late (Kondo et al., 2007).
Amblyopia
Amblyopia can emerge when the visual input from one eye is not properly processed by the brain, which progressively develops a “preference” for the other eye. It results in atypical vision from one eye that otherwise appears organically normal (Bretas and Soriano, 2016). At the brain level, typical functional abnormalities associated with amblyopia converge in indicating V1 as the most affected brain region (Blakemore and Vital-Durand, 1986). However, the abnormal neural activity associated with amblyopia is not necessarily limited to V1, rather extending also to V2 and V3 (Barnes et al., 2001), even when V1 is normally functioning (Clavagnier et al., 2015). Interestingly, amblyopia patients present larger receptive fields in V1, V2, and V3, possibly as a consequence of the oculomotor instability of the amblyopic eye (Levin et al., 2010). Indeed there seem to be a sort of propagation of dysfunctional neural dynamics from V1 up to V5 (Barnes et al., 2001), which would result in specific deficits in extrastriate functions, including global motion (Simmers et al., 2003) or contrast-based contours (Wong et al., 2001).
Akinetopsia
Our ability to perceive motion allows us to distinguish objects from the background and to move in a three-dimensional world (Barton, 2011). Commonly called also motion blindness, akinetopsia refers to the impossibility to detect moving objects, in absence of scotoma (Zihl et al., 1983), while other low-level aspects like color or shape are normally detected (Zeki, 1991). Typically associated with an extrastriate brain lesion (Zihl et al., 1983; Cooper et al., 2012; Otsuka-Hirota et al., 2014), akinetopsia can indeed be experimentally induced by inhibiting V5 (Beckers and Hömberg, 1992), as well as V1 but at a smaller degree and with specific timing with respect to the visual stimulus (Beckers and Hömberg, 1992). This is in line with the observation that sensitivity to motion can survive cortical blindness (Ruffieux et al., 2016), also in children that present a congenital, but not acquired, lesion of V1 (Tinelli et al., 2013). While blindness to first-order motion (e.g., luminance-based) would result from lesions in V2/V3, blindness to second-order motion (e.g., contrast-based) would derive from lesions in V4/V5 (Cowey et al., 2006). A particular case of motion blindness is represented by the “form-from-motion” blindness, referring to the impossibility to detect forms on the basis of visual motion (Cowey and Vaina, 2000). Indeed form-from-motion blindness with and without akinetopsia are neurally dissociable, being the former associated with lesions in V5 and lateral occipital cortex and the latter with occipito-temporal regions (Blanke et al., 2007). Even if chronic cases have been reported (Cooper et al., 2012), akinetopsia seems a rather transient condition (Shipp et al., 1994), suggesting the existence of functionally neuroplastic changes able to establish alternative neural interactions to restore sensitivity to visual motion. Such a relative ease to naturally react to akinetopsia makes it difficult to detect, especially in populations characterized by high neural plasticity like children, where in fact akinetopsia is relatively rare and usually present in combination with the Alice in Wonderland syndrome as a results of encephalitis (Naarden et al., 2019).
Higher-Level Dysfunctions
Optic Ataxia
Originally described by Rudolph Bálint in 1909 as part of a more complex syndrome (Rudolph Bálint, 1909), optic ataxia refers to the incapacity to perform accurate visually-guided movements, in absence of general motor impairments (Moreaud, 2003). Letting patients misplace the fork outside the plate, grasp a coffee mug from its body instead of its handle, or point to the wrong button on a computer keyboard, optic ataxia is considered the typical visuo-motor integration disorder (Teixeira et al., 2014). Not strictly limited to visuo-motor behaviors of the upper limb (Evans et al., 2013), it can emerge as early as in children aged between 5 (Dutton, 2003) and 10 years (Drummond and Dutton, 2007). Possibly as a consequence of premature birth (Dutton, 2013), optic ataxia has a confirmed association with aberrancies in the (occipital-parietal) dorsal stream (Philip et al., 2016). Indeed, the most accepted neural underpinnings of optic ataxia are comprised within the dorsal stream (Schindler et al., 2004), possibly also in interaction with the ventral stream (Himmelbach and Karnath, 2005). Further investigations reported that optic ataxia affects mainly the peripheral vision (Pisella et al., 2009) and is especially evident in contralesional visuo-motor tasks (Gaveau et al., 2008). This suggests that optic ataxia should not be considered as a unitary deficit, but rather presents various degrees and specifications as a function of the lesioned dorsal stream module responsible to coordinate visual perception and action. Nevertheless, recent evidence is starting to challenge such a sharp dissociation between perception and action in optic ataxia (Rossetti and Pisella, 2018). In particular, optic ataxia would derive from dorsal stream deficits in integrating multimodal sensory input (Jackson, 2010), it can be stimulus/task-specific (Hesse et al., 2014), and it can be bound to specific visuo-motor neurons located in different regions of the dorsal stream and beyond (Cooper and O'sullivan, 2016), especially the premotor cortex (Battaglia-Mayer and Caminiti, 2002) and a parietal-precuneus pathway (Teixeira et al., 2014).
Cerebral Visual Impairment
As one of the most common causes of visual impairment of cortical origin, cerebral visual impairment (CVI) can result from early brain damage, including a potentially large panel of correlated deficits beyond vision due to damages of the dorsal stream, the ventral stream, or both (Bennett et al., 2020). Behaviorally, it is possible to detect CVI by means of dedicated questionnaires (Gorrie et al., 2019; Fazzi and Micheletti, 2020). At the neural level, a relatively early detection of CVI is based on the analysis of visual evoked potentials which, already at 6 months of age, can appear abnormal and therefore suggest the presence of CVI (Mercuri et al., 1997b), further depending on the size (Mercuri et al., 1998) and location (Mercuri et al., 1997a) of the brain lesion. In particular, the basal ganglia seem to play a central role in coordinating the information exchanges between the eyes and the visual cortex, as well as in facilitating neural plasticity at the cortical level (Mikellidou et al., 2019), possibly resulting in aberrant patterns of anatomo-functional connectivity between different brain regions (Muñoz-Moreno et al., 2016; Bathelt et al., 2020). It is anyways important to note that CVI can impair a full range of competences at different levels, including purely visual skills (visual field, motion sensitivity, visual exploration) as well as attention, memory, and visuo-motor coordination (Lueck et al., 2019). This is the main reason why current trends in neuro-ophthalmology highlight the importance of considering each patient as an individual case that should be evaluated on the basis of a personalized and multidisciplinary assessment combining ophthalmology, neuropsychology, and pedagogy (Ortibus et al., 2019).
Dorsal Stream Vulnerability
As already outlined, the dorsal stream is considered the main neural architecture processing spatial aspects of vision and their translation into relevant information for functions beyond the mere sight. Converging evidence supports that the dorsal stream is more vulnerable than the ventral stream to developmental disorders (Grinter et al., 2010), due to genetic or contextual factors (Atkinson, 2017) as well as interventional approaches (Tonks et al., 2019). Possibly leading to cognitive decline (Ricci et al., 2015), attentional/visuo-spatial deficits (Tonks et al., 2019), and visuo-motor impairments (Atkinson and Braddick, 2011), the dorsal stream vulnerability can start in early age and keeps affecting the individual competences from early childhood across the life span (Sciberras-Lim and Lambert, 2017). Nevertheless, recent findings are starting to challenge this view, by arguing that dorsal stream vulnerability might be stimulus-specific rather than a general dysfunction (Joshi et al., 2020), as shown for example by the relatively preserved motion sensitivity in amblyopia (Hamm et al., 2014). Beyond the stimulus-specificity, such a controversy might result also from task-specificity since, for example, some dorsal stream functions (e.g., time estimation and attentional tasks) seem more sensitive to developmental disorders than others (e.g., numerical discrimination or mapping). In consideration of such a variability among stimuli and tasks, it is clear that to evaluate a wide range of symptoms like those related to dorsal stream vulnerability implies the need of using multidimensional scales for evaluating dorsal stream vulnerability (Atkinson et al., 2002).
Developmental Coordination Disorder
The diagnosis of Developmental Coordination Disorder (DCD) is based on the presence of motor impairments in absence of other neuropsychological deficits able to explain patient's poor motor performance (cerebral palsy, neurodegeneration, traumatic brain injuries, etc.) (Blank et al., 2019). The characteristics of DCD include impaired control of ocular, postural, and manual tasks, as well as motor imagery (Adams et al., 2014). One of the possible interpretations of DCD explains the disorder as the result of breakdowns in a visuo-motor matching system which would allow to perform movements on the basis of observing the same movements performed by somebody else (Werner et al., 2012). Such a breakdown would affect in particular the ability to process and exploit the temporal binding between vision and movements (Nobusako et al., 2018). The visuo-motor interpretation of DCD is in line with evidence that DCD patients exhibit impaired visuo-motor skills (Reynolds et al., 2017) and decreased brain activation in regions typically involved in visuo-motor imitation (Licari et al., 2015) and action planning (Reynolds et al., 2019). In particular, even if a large consensus has not been reached yet (Brown-Lum and Zwicker, 2015), it seems that the brain dysfunctions associated with DCD are mainly located in associative regions of the parietal and frontal lobe particularly important for visually-based action imitation (Biotteau et al., 2016). In sum, despite the little number of studies and the large variability of their results, the is a tendency to consider DCD as a visuo-motor integration deficit specifically affecting the neural network responsible for visually interpreting actions performed by other people and exploit such information for guiding self-produced movements. However, further studies are required and the present conclusions have to be regarded with caution.
Prosopagnosia
The ability to recognize faces is one of the most important abilities in the human world. The centrality of this function is reflected in the fact that the brain dedicates a specific neural substrate to process face-like visual input (Zeugin et al., 2020), with a particular emphasis on the fusiform face area in the (ventral stream) inferior temporal cortex (Kanwisher et al., 1997). Prosopagnosia refers to the inability to recognize faces (Mayer and Rossion, 2007) associated with occipito-temporal brain activity (Dalrymple et al., 2014) and, in particular, with bilateral lesion of the fusiform face area (Grüter et al., 2008). It is dissociated from other objet-recognition deficits as, for example, there are cases in which in consequence of a bilateral fusiform lesion patients become unable to recognize faces while their performance in object recognition remains at good levels (Moscovitch et al., 1997). Since prosopagnosic people are largely unaware of their deficit, prosopagnosia can dramatically affect the cognitive development of otherwise typically growing children (Schmalzl et al., 2008), including the preference for social identification on the basis of whole-body configuration instead of facial features (Wilson et al., 2010). This conditions can trigger a cascade of aversive events and behaviors also in daily contexts like schools, where both teachers and colleagues would not detect the prosopagnosic deficit and therefore might put disproportionate reactions in place (Wilson et al., 2010). To prevent and possibly overcome this risk, at present there are strong trends toward the development of specific test to assess face perception abilities in children, such as the Dartmouth Database of Children's Faces (Dalrymple et al., 2013) and the Cambridge Face Memory Test for Children (Croydon et al., 2014).
Somatoparaphrenia
The ability to recognize our own body also plays a central role in adaptive behaviors and consciousness (Ionta et al., 2013). Somatoparaphrenia refers to the inability to identify one's own body part as belonging to one self, both at the subjective conscious (Invernizzi et al., 2013) and objective physiological levels (Romano et al., 2014). Despite its psychiatric component (Feinberg and Venneri, 2014), somatoparaphrenia is largely associated with unilateral lesions, mostly in the right hemisphere and therefore affecting the left side of the body (Vallar and Ronchi, 2009). At a more specific neural level the available evidence is controversial, with clinical observations reporting damages in either the dorsal or the ventral stream, as well as other brain regions. Thus, different studies proposed that somatoparaphrenia would derive from lesions in the posterior insula (Baier and Karnath, 2008), supramarginal gyrus (Feinberg et al., 1990), orbito-frontal regions (Feinberg et al., 2010), posterior superior temporal cortex (Vallar and Ronchi, 2009). In addition, recent investigations highlighted the importance of more complex fronto-temporal-parietal cortical networks as well as subcortical circuits (Gandola et al., 2012). Even if somatoparaphrenia is commonly associated with hemispatial neglect, it can be present also in isolation and associated with specific subcortical lesions, comprising the basal ganglia, thalamus, and internal capsule (Invernizzi et al., 2013). Interestingly when somatoparaphrenic patients observe the misrecognized body part in a mirror (as from a third-person perspective), their self-misattribution decreases (Fotopoulou et al., 2011). Already rarely detected in adults, possibly due to its comorbidity with hemispatial neglect and its confusion with asomatognosia, evidence of somatoparaphrenia in children is even more scarce. However, a study implementing a neuroinvestigation technique with high spatial resolution (electrocorticography) in awake humans, reported that following abnormal neural firing in the right occipito-temporo-parietal cortex, a 10-year-old child reported somatoparaphrenic symptoms, being unable to recognize his left hand (Heydrich et al., 2011). Altogether, it seems that the small numbers to somatoparaphrenic reporting reflects a general lack of episodes spontaneously mentioned by the patients together with the confusion with other pathologies by the evaluators. For this reasons, it would be important to explicitly assess somatoparaphrenic symptoms using structured interviews (Brandt et al., 2005) and/or standardized scales especially in developing age.
Hemispatial Neglect
The absence of perception and action in half of the sensory fields and peri-personal space, respectively, defines the hemispatial neglect. Patients suffering from this syndrome to not perceive sensory stimuli in any modality from the neglected hemifield and do not perform movements in that hemi-peri-personal space. The traditional test to assess neglect is the line bisection task, in which patients are presented with a paper sheet with a number of short lines distributed all over a paper sheet. Typically, when patients are asked to draw a line over each short line (bisection), they mark only half of the lines (those located in the non-neglected hemifield). At the neural level, hemispatial neglect seems to derive from dysfunctions in the right inferior parietal lobule, possibly in association with deteriorated input from the ventral stream (Milner and Goodale, 2008) or with impaired ventro-fronto-parietal circuit distinct from the traditional dorsal stream (Husain and Nachev, 2007). However, hemispatial neglect can emerge also following lesions of the frontal cortex (Husain and Kennard, 1996), basal ganglia or thalamus (Mort et al., 2003), as well as from lesions of white matter pathways connecting the parietal and frontal cortex (Bartolomeo et al., 2007). Altogether, it seems that hemispatial neglect may be the result of a lesions in a large-scale cortico-subcortical network, possibly implicated in attention-related abilities. Interestingly, while most intervention protocols eventually produce only temporary improvements, the most efficient and relatively long-lasting treatment is based on the use of prism adaptation (Rossetti et al., 1998). In particular, the visual distortion brought by wearing prism lenses would trigger the activation of otherwise silent visuo-motor circuits as valid alternative neural pathways to allow visuo-motor coordination, with benefit spreading also in the cognitive domain (Rossetti et al., 1998). Even if hemispatial neglect is commonly associated with adult and elderly patients, also children can be affected, and not necessarily only in the visual domain (Martin and Trauner, 2019). Cases of hemispatial neglect have been reported for children as young as 3-year-old (Thompson et al., 1991), 6-year-old (Ferro et al., 1984), and above (Hausmann et al., 2003; Marsh et al., 2009). Actually, also at 6 months after birth, children with pre- or post-natal brain damage can exhibit otherwise unmotivated preference for interacting with objects located in the hemi-peri-personal space ipsilateral to a unilateral lesion in the left or right hemisphere (Trauner, 2003). Most of the studies indicate that children can relatively quickly recover from neglect symptoms within a few weeks (Kleinman et al., 2010) or months (Thompson et al., 1991) after a stroke. Even children that suffered from a perinatal stroke, especially in the right hemisphere, can present hemi-neglect-like symptoms in the left hemi-field and peri-personal space, including visuo-motor deficits (Vicari et al., 1998), reaching and grasping (Trauner, 2003), as well as visual cancellation and manual exploration (Thareja et al., 2012). These studies further showed that, in contrast with the typical right-hemispheric dominance of hemi-spatial neglect in adults, in children a more dramatic bilateral neglect can result from a left-hemispheric lesion (Trauner, 2003; Thareja et al., 2012), whose resolution might require maturation up to adolescence or adulthood (Yousefian et al., 2015).
Final Remarks
Understanding the behavioral and neural fundaments of the complex interaction between vision and other sphere of human life is the prerequisite for better targeted interventional procedures in case of deficits, as well as for more efficient training programs in typically developing populations. As a very general overview, the present paper summarizes some of the most relevant evidence about the neural basis of vision and associated abilities in development and beyond. With the aim of constituting a first-glance reference for researchers and clinicians interested in vision and visuo-motor integration, this review hopes to guide and trigger further investigations toward more specific publications in case of specific interests.
Establishing the neural correlates of aberrant behaviors helps identifying the neural networks responsible for a given function which, in turn, can boost the development of more effective training and rehabilitation protocols. Accordingly, the knowledge summarized here sustains the importance of adopting a systemic approach even in the evaluation of the impact of supposedly purely visual deficits, which indeed can affect also motor skills, cognition, social skills, and emotional processing. Addressing such a complexity is the fundamental requirement of current implementations of systemic approaches for visually-related training in typical conditions or in response to visual disorders, including virtual reality (Adams et al., 2018; Choi et al., 2021), robotics (Mirkowski et al., 2019; Zhexenova et al., 2020), and touch screen technology (Aslam et al., 2016; Sheehan and Uttal, 2016; Dalecki et al., 2019).
Author Contributions
SI conceived the work, performed the literature analysis, and wrote the manuscript.
Funding
This work was supported by the Swiss National Science Foundation (Grant PP00P1_170506/1).
Conflict of Interest
The author declares that the research was conducted in the absence of any commercial or financial relationships that could be construed as a potential conflict of interest.
References
Adams, H., Narasimham, G., Rieser, J., Creem-Regehr, S., Stefanucci, J., and Bodenheimer, B. (2018). Locomotive recalibration and prism adaptation of children and teens in immersive virtual environments. IEEE Trans. Vis. Comput. Graph. 24, 1408–1417. doi: 10.1109/TVCG.2018.2794072
Adams, I. L., Lust, J. M., Wilson, P. H., and Steenbergen, B. (2014). Compromised motor control in children with DCD: a deficit in the internal model?—A systematic review. Neurosci. Biobehav. Rev. 47, 225–244. doi: 10.1016/j.neubiorev.2014.08.011
Adolph, K. E., and Franchak, J. M. (2017). The development of motor behavior. Wiley Interdiscip. Rev. Cogn. Sci. 8:e1430. doi: 10.1002/wcs.1430
Almeida, J., Mahon, B. Z., and Caramazza, A. (2010). The role of the dorsal visual processing stream in tool identification. Psychol. Sci. 21, 772–778. doi: 10.1177/0956797610371343
Anderson, J. A., Healey, M. K., Hasher, L., and Peterson, M. A. (2016). Age-related deficits in inhibition in figure-ground assignment. J. Vis. 16:6. doi: 10.1167/16.7.6
Anzai, A., Peng, X., and Van Essen, D. C. (2007). Neurons in monkey visual area V2 encode combinations of orientations. Nat. Neurosci. 10, 1313–1321. doi: 10.1038/nn1975
Arterberry, M. E., and Yonas, A. (2000). Perception of three-dimensional shape specified by optic flow by 8-week-old infants. Percept. Psychophys. 62, 550–556. doi: 10.3758/BF03212106
Arzy, S., Thut, G., Mohr, C., Michel, C. M., and Blanke, O. (2006). Neural basis of embodiment: distinct contributions of temporoparietal junction and extrastriate body area. J. Neurosci. 26, 8074–8081. doi: 10.1523/JNEUROSCI.0745-06.2006
Aslam, T. M., Tahir, H. J., Parry, N. R., Murray, I. J., Kwak, K., Heyes, R., et al. (2016). Automated measurement of visual acuity in pediatric ophthalmic patients using principles of game design and tablet computers. Am. J. Ophthalmol. 170, 223–227. doi: 10.1016/j.ajo.2016.08.013
Atkinson, J. (2017). The Davida Teller Award Lecture, 2016: visual brain development: a review of “dorsal stream vulnerability”-motion, mathematics, amblyopia, actions, and attention. J. Vis. 17:26. doi: 10.1167/17.3.26
Atkinson, J., Anker, S., Rae, S., Hughes, C., and Braddick, O. (2002). A test battery of child development for examining functional vision (ABCDEFV). Strabismus 10, 245–269. doi: 10.1076/stra.10.4.245.13831
Atkinson, J., and Braddick, O. (2011). From genes to brain development to phenotypic behavior: “dorsal-stream vulnerability” in relation to spatial cognition, attention, and planning of actions in Williams syndrome (WS) and other developmental disorders. Prog. Brain. Res. 189, 261–283. doi: 10.1016/B978-0-444-53884-0.00029-4
Atkinson, J., Braddick, O., Anker, S., Nardini, M., Birtles, D., Rutherford, M. A., et al. (2008). Cortical vision, MRI and developmental outcome in preterm infants. Arch. Dis. Childhood Fetal. Neonatal. Edn. 93, F292–F297. doi: 10.1136/adc.2007.116988
Baier, B., and Karnath, H. O. (2008). Tight link between our sense of limb ownership and self-awareness of actions. Stroke 39, 486–488. doi: 10.1161/STROKEAHA.107.495606
Baker, C., Hess, R. F., and Zihl, J. (1991). Residual motion perception in a “motion-blind” patient, assessed with limited-lifetime random dot stimuli. J. Neurosci. 11, 454–461. doi: 10.1523/JNEUROSCI.11-02-00454.1991
Banks, M. S., and Dannemiller, J. L. (1987). Infant visual psychophysics. Handbook Infant Percept. 1, 115–184.
Banks, M. S., and Salapatek, P. (1978). Acuity and contrast sensitivity in 1-, 2-, and 3-month-old human infants. Invest. Ophthalmol. Vis. Sci. 17, 361–365.
Bara, F., and Bonneton-Botte, N. (2018). Learning letters with the whole body: visuomotor versus visual teaching in kindergarten. Percept. Mot. Skills 125, 190–207. doi: 10.1177/0031512517742284
Bara, F., Gentaz, E., Colé, P., and Sprenger-Charolles, L. (2004). The visuo-haptic and haptic exploration of letters increases the kindergarten-children's understanding of the alphabetic principle. Cogn. Dev. 19, 433–449. doi: 10.1016/j.cogdev.2004.05.003
Barnes, G., Hess, R., Dumoulin, S., Achtman, R., and Pike, G. (2001). The cortical deficit in humans with strabismic amblyopia. J. Physiol. 533, 281–297. doi: 10.1111/j.1469-7793.2001.0281b.x
Bartolomeo, P., Thiebaut De Schotten, M., and Doricchi, F. (2007). Left unilateral neglect as a disconnection syndrome. Cereb. Cortex 17, 2479–2490. doi: 10.1093/cercor/bhl181
Barton, J. J. (2011). Disorder of higher visual function. Curr. Opin. Neurol. 24, 1–5. doi: 10.1097/WCO.0b013e328341a5c2
Bathelt, J., Dale, N. J., De Haan, M., and Clark, C. A. (2020). Brain structure in children with congenital visual disorders and visual impairment. Dev. Med. Child Neurol. 62, 125–131. doi: 10.1111/dmcn.14322
Battaglia-Mayer, A., and Caminiti, R. (2002). Optic ataxia as a result of the breakdown of the global tuning fields of parietal neurones. Brain 125, 225–237. doi: 10.1093/brain/awf034
Beckers, G., and Hömberg, V. (1992). Cerebral visual motion blindness: transitory akinetopsia induced by transcranial magnetic stimulation of human area V5. Proc. R. Soc. London Ser. B Biol. Sci. 249, 173–178. doi: 10.1098/rspb.1992.0100
Bennett, C. R., Bauer, C. M., Bailin, E. S., and Merabet, L. B. (2020). Neuroplasticity in cerebral visual impairment (CVI): assessing functional vision and the neurophysiological correlates of dorsal stream dysfunction. Neurosci. Biobehav. Rev. 108, 171–181. doi: 10.1016/j.neubiorev.2019.10.011
Berman, N., and Murphy, E. H. (1981). The critical period for alteration in cortical binocularity resulting from divergent and convergent strabismus. Brain Res. 254, 181–202. doi: 10.1016/0165-3806(81)90031-6
Biagi, L., Crespi, S. A., Tosetti, M., and Morrone, M. C. (2015). BOLD response selective to flow-motion in very young infants. PLoS. Biol. 13:e1002260. doi: 10.1371/journal.pbio.1002260
Biotteau, M., Chaix, Y., Blais, M., Tallet, J., Péran, P., and Albaret, J.-M. (2016). Neural signature of DCD: a critical review of MRI neuroimaging studies. Front. Neurol. 7:227. doi: 10.3389/fneur.2016.00227
Blakemore, C., and Vital-Durand, F. (1986). Effects of visual deprivation on the development of the monkey's lateral geniculate nucleus. J. Physiol. 380, 493–511. doi: 10.1113/jphysiol.1986.sp016298
Blank, R., Barnett, A. L., Cairney, J., Green, D., Kirby, A., Polatajko, H., et al. (2019). International clinical practice recommendations on the definition, diagnosis, assessment, intervention, and psychosocial aspects of developmental coordination disorder. Dev. Med. Child Neurol. 61, 242–285. doi: 10.1111/dmcn.14132
Blanke, O., Brooks, A., Mercier, M., Spinelli, L., Adriani, M., Lavanchy, L., et al. (2007). Distinct mechanisms of form-from-motion perception in human extrastriate cortex. Neuropsychologia 45, 644–653. doi: 10.1016/j.neuropsychologia.2006.07.019
Bohon, K. S., Hermann, K. L., Hansen, T., and Conway, B. R. (2016). Representation of perceptual color space in macaque posterior inferior temporal cortex (the V4 complex). eNeuro 3, 1–28. doi: 10.1523/ENEURO.0039-16.2016
Bondarenko, V. M., Semenov, L. A., Solnushkin, S. D., and Chikhman, V. N. (2010). Age related size invariance in perception of illusory and fragmented contours. Fiziol. Cheloveka 36, 41–47. doi: 10.1134/S0362119710060058
Booth, A. E., Pinto, J., and Bertenthal, B. I. (2002). Perception of the symmetrical patterning of human gait by infants. Dev. Psychol. 38:554. doi: 10.1037/0012-1649.38.4.554
Bourne, J. A., and Morrone, M. C. (2017). Plasticity of visual pathways and function in the developing brain: is the pulvinar a crucial player? Front. Syst. Neurosci. 11:3. doi: 10.3389/fnsys.2017.00003
Braddick, O., and Atkinson, J. (2011). Development of human visual function. Vis. Res. 51, 1588–1609. doi: 10.1016/j.visres.2011.02.018
Braddick, O., and Atkinson, J. (2013). Visual control of manual actions: brain mechanisms in typical development and developmental disorders. Dev. Med. Child Neurol. 55(Suppl. 4), 13–18. doi: 10.1111/dmcn.12300
Braddick, O., Atkinson, J., Akshoomoff, N., Newman, E., Curley, L. B., Gonzalez, M. R., et al. (2017). Individual differences in children's global motion sensitivity correlate with TBSS-based measures of the superior longitudinal fasciculus. Vis. Res. 141, 145–156. doi: 10.1016/j.visres.2016.09.013
Braddick, O., Atkinson, J., Hood, B., Harkness, W., Jackson, G., and Vargha-Khademt, F. (1992). Possible blindsight in infants lacking one cerebral hemisphere. Nature 360, 461–463. doi: 10.1038/360461a0
Braddick, O., Atkinson, J., Newman, E., Akshoomoff, N., Kuperman, J. M., Bartsch, H., et al. (2016). Global visual motion sensitivity: associations with parietal area and children's mathematical cognition. J. Cogn. Neurosci. 28, 1897–1908. doi: 10.1162/jocn_a_01018
Braddick, O., Atkinson, J., and Wattam-Bell, J. (2003). Normal and anomalous development of visual motion processing: motion coherence and ‘dorsal-stream vulnerability'. Neuropsychologia 41, 1769–1784. doi: 10.1016/S0028-3932(03)00178-7
Braddick, O., Wattam-Bell, J., and Atkinson, J. (1986). Orientation-specific cortical responses develop in early infancy. Nature 320, 617–619. doi: 10.1038/320617a0
Braddick, O. J., O'brien, J. M., Wattam-Bell, J., Atkinson, J., Hartley, T., and Turner, R. (2001). Brain areas sensitive to coherent visual motion. Perception 30, 61–72. doi: 10.1068/p3048
Brandt, C., Brechtelsbauer, D., Bien, C., and Reiners, K. (2005). Out-of-body experience as possible seizure symptom in a patient with a right parietal lesion. Nervenarzt 76, 1261–1252. doi: 10.1007/s00115-005-1904-y
Bretas, C. C., and Soriano, R. N. (2016). Amblyopia: neural basis and therapeutic approaches. Arq. Bras. Oftalmol. 79, 346–351. doi: 10.5935/0004-2749.20160099
Bronson, G. (1974). The postnatal growth of visual capacity. Child Dev. 45, 873–890. doi: 10.2307/1128073
Brooks, C. R., and Clair, T. N. (1971). Relationships among visual figure-ground perception, word recognition, IQ, and chronological age. Percept. Mot. Skills 33, 59–62. doi: 10.2466/pms.1971.33.1.59
Brown, A. M. (1990). Development of visual sensitivity to light and color vision in human infants: a critical review. Vis. Res. 30, 1159–1188. doi: 10.1016/0042-6989(90)90173-I
Brown, A. M. (1997). Vernier acuity in human infants: rapid emergence shown in a longitudinal study. Optometry Vision Sci. 74, 732–740. doi: 10.1097/00006324-199709000-00021
Brown-Lum, M., and Zwicker, J. G. (2015). Brain imaging increases our understanding of developmental coordination disorder: a review of literature and future directions. Curr. Dev. Disord. Rep. 2, 131–140. doi: 10.1007/s40474-015-0046-6
Bushneil, I., Sai, F., and Mullin, J. (1989). Neonatal recognition of the mother's face. Br. J. Dev. Psychol. 7, 3–15. doi: 10.1111/j.2044-835X.1989.tb00784.x
Candidi, M., Urgesi, C., Ionta, S., and Aglioti, S. M. (2008). Virtual lesion of ventral premotor cortex impairs visual perception of biomechanically possible but not impossible actions. Soc. Neurosci. 3, 388–400. doi: 10.1080/17470910701676269
Candy, T. R., Skoczenski, A. M., and Norcia, A. M. (2001). Normalization models applied to orientation masking in the human infant. J. Neurosci. 21, 4530–4541. doi: 10.1523/JNEUROSCI.21-12-04530.2001
Chakraborty, A., Anstice, N. S., Jacobs, R. J., Paudel, N., Lagasse, L. L., Lester, B. M., et al. (2015). Global motion perception is independent from contrast sensitivity for coherent motion direction discrimination and visual acuity in 4.5-year-old children. Vis. Res. 115, 83–91. doi: 10.1016/j.visres.2015.08.007
Chakraborty, A., Anstice, N. S., Jacobs, R. J., Paudel, N., Lagasse, L. L., Lester, B. M., et al. (2017). Global motion perception is related to motor function in 4.5-year-old children born at risk of abnormal development. Vis. Res. 135, 16–25. doi: 10.1016/j.visres.2017.04.005
Chalupa, L. M. (2004). Complete restoration of visual cortical responses is possible late in development. Focus on “recovery of cortical binocularity and orientation selectivity after the critical period for ocular dominance plasticity”. J. Neurophysiol. 92, 1969–1970. doi: 10.1152/jn.00497.2004
Chino, Y. M., Smith Iii, E. L., Hatta, S., and Cheng, H. (1997). Postnatal development of binocular disparity sensitivity in neurons of the primate visual cortex. J. Neurosci. 17, 296–307. doi: 10.1523/JNEUROSCI.17-01-00296.1997
Choi, J. Y., Yi, S. H., Ao, L., Tang, X., Xu, X., Shim, D., et al. (2021). Virtual reality rehabilitation in children with brain injury: a randomized controlled trial. Dev. Med. Child Neurol. 63, 480–487. doi: 10.1111/dmcn.14762
Clavagnier, S., Dumoulin, S. O., and Hess, R. F. (2015). Is the cortical deficit in amblyopia due to reduced cortical magnification, loss of neural resolution, or neural disorganization? J. Neurosci. 35, 14740–14755. doi: 10.1523/JNEUROSCI.1101-15.2015
Cobbett, S., and Snelgrove-Clarke, E. (2016). Virtual versus face-to-face clinical simulation in relation to student knowledge, anxiety, and self-confidence in maternal-newborn nursing: a randomized controlled trial. Nurse Educ. Today 45, 179–184. doi: 10.1016/j.nedt.2016.08.004
Cook, R., Bird, G., Catmur, C., Press, C., and Heyes, C. (2014). Mirror neurons: from origin to function. Behav. Brain Sci. 37, 177–192. doi: 10.1017/S0140525X13000903
Cooper, S. A., Joshi, A. C., Seenan, P. J., Hadley, D. M., Muir, K. W., Leigh, R. J., et al. (2012). Akinetopsia: acute presentation and evidence for persisting defects in motion vision. J. Neurol. Neurosurg. Psychiatry 83, 229–230. doi: 10.1136/jnnp.2010.223727
Cooper, S. A., and O'sullivan, M. (2016). Here, there and everywhere: higher visual function and the dorsal visual stream. Pract. Neurol. 16, 176–183. doi: 10.1136/practneurol-2015-001168
Costantini, M., Urgesi, C., Galati, G., Romani, G. L., and Aglioti, S. M. (2011). Haptic perception and body representation in lateral and medial occipito-temporal cortices. Neuropsychologia 49, 821–829. doi: 10.1016/j.neuropsychologia.2011.01.034
Cowey, A., Campana, G., Walsh, V., and Vaina, L. M. (2006). The role of human extra-striate visual areas V5/MT and V2/V3 in the perception of the direction of global motion: a transcranial magnetic stimulation study. Exp. Brain Res. 171, 558–562. doi: 10.1007/s00221-006-0479-6
Cowey, A., and Vaina, L. (2000). Blindness to form from motion despite intact static form perception and motion detection. Neuropsychologia 38, 566–578. doi: 10.1016/S0028-3932(99)00117-7
Croydon, A., Pimperton, H., Ewing, L., Duchaine, B. C., and Pellicano, E. (2014). The Cambridge Face Memory Test for Children (CFMT-C): A new tool for measuring face recognition skills in childhood. Neuropsychologia 62, 60–67. doi: 10.1016/j.neuropsychologia.2014.07.008
Csibra, G., Tucker, L. A., and Johnson, M. H. (1998). Neural correlates of saccade planning in infants: a high-density ERP study. Int. J. Psychophysiol. 29, 201–215. doi: 10.1016/S0167-8760(98)00016-6
Cullen, K. E. (2015). Neural circuits that drive binocular eye movements: implications for understanding and correcting strabismus. Invest. Ophthalmol. Vis. Sci. 56:20. doi: 10.1167/iovs.14-16177
Dalecki, M., Gorbet, D. J., and Sergio, L. E. (2019). Development of rule-based eye-hand-decoupling in children and adolescents. Child Neuropsychol. 25, 1098–1115. doi: 10.1080/09297049.2019.1578342
Dalrymple, K. A., Davies-Thompson, J., Oruc, I., Handy, T. C., Barton, J. J., and Duchaine, B. (2014). Spontaneous perceptual facial distortions correlate with ventral occipitotemporal activity. Neuropsychologia 59, 179–191. doi: 10.1016/j.neuropsychologia.2014.05.005
Dalrymple, K. A., Gomez, J., and Duchaine, B. (2013). The dartmouth database of children's faces: acquisition and validation of a new face stimulus set. PLoS ONE 8:e79131. doi: 10.1371/journal.pone.0079131
Das, V. E. (2016). Strabismus and the oculomotor system: insights from macaque models. Annu. Rev. Vis. Sci. 2, 37–59. doi: 10.1146/annurev-vision-111815-114335
De Azevedo Neto, R. M., and Amaro Junior, E. (2018). Bilateral dorsal fronto-parietal areas are associated with integration of visual motion information and timed motor action. Behav. Brain. Res. 337, 91–98. doi: 10.1016/j.bbr.2017.09.046
De Haan, E. H., and Cowey, A. (2011). On the usefulness of ‘what'and ‘where'pathways in vision. Trends Cogn. Sci. 15, 460–466. doi: 10.1016/j.tics.2011.08.005
De Haan, M., Johnson, M. H., and Halit, H. (2003). Development of face-sensitive event-related potentials during infancy: a review. Int. J. Psychophysiol. 51, 45–58. doi: 10.1016/S0167-8760(03)00152-1
De Heering, A., and Rossion, B. (2015). Rapid categorization of natural face images in the infant right hemisphere. Elife 4:e06564. doi: 10.7554/eLife.06564
De Klerk, C. C., Johnson, M. H., Heyes, C. M., and Southgate, V. (2015). Baby steps: investigating the development of perceptual-motor couplings in infancy. Dev. Sci. 18, 270–280. doi: 10.1111/desc.12226
Deen, B., Richardson, H., Dilks, D. D., Takahashi, A., Keil, B., Wald, L. L., et al. (2017). Organization of high-level visual cortex in human infants. Nat. Commun. 8, 1–10. doi: 10.1038/ncomms13995
Dehmel, S., and Lowel, S. (2014). Cortico-cortical interactions influence binocularity of the primary visual cortex of adult mice. PLoS ONE 9:e105745. doi: 10.1371/journal.pone.0105745
Dekker, T. M., Ban, H., Van Der Velde, B., Sereno, M. I., Welchman, A. E., and Nardini, M. (2015). Late development of cue integration is linked to sensory fusion in cortex. Curr. Biol. 25, 2856–2861. doi: 10.1016/j.cub.2015.09.043
Di Stefano, M., and Gargini, C. (2002). Cortical binocularity in convergent strabismus after section of the optic chiasm. Exp. Brain Res. 147, 64–70. doi: 10.1007/s00221-002-1190-x
Downey, C., Pace, G., Seemiller, E., Candy, R., and Cormack, L. (2017). Dynamic characteristics of 5 to 22 week-old infants' accommodation and vergence tracking responses. J. Vision 17, 443–443. doi: 10.1167/17.10.443
Downing, P. E., Jiang, Y., Shuman, M., and Kanwisher, N. (2001). A cortical area selective for visual processing of the human body. Science 293, 2470–2473. doi: 10.1126/science.1063414
Drummond, S. R., and Dutton, G. N. (2007). Simultanagnosia following perinatal hypoxia: a possible pediatric variant of Balint syndrome. J. AAPOS. 11, 497–498. doi: 10.1016/j.jaapos.2007.03.007
Dubner, R., and Zeki, S. (1971). Response properites and receptive fields of cells in an anatomically defined region of the superior temporal sulcus in the monkey. Brain Res. 35, 528–532. doi: 10.1016/0006-8993(71)90494-X
Dursteler, M., Wurtz, R. H., and Newsome, W. T. (1987). Directional pursuit deficits following lesions of the foveal representation within the superior temporal sulcus of the macaque monkey. J. Neurophysiol. 57, 1262–1287. doi: 10.1152/jn.1987.57.5.1262
Dutton, G. N. (2003). Cognitive vision, its disorders and differential diagnosis in adults and children: knowing where and what things are. Eye 17, 289–304. doi: 10.1038/sj.eye.6700344
Dutton, G. N. (2013). The spectrum of cerebral visual impairment as a sequel to premature birth: an overview. Doc. Ophthalmol. 127, 69–78. doi: 10.1007/s10633-013-9382-1
Elberger, A. J., and Smith, E. L. 3rd. (1985). The critical period for corpus callosum section to affect cortical binocularity. Exp. Brain Res. 57, 213–223. doi: 10.1007/BF00236526
Evans, C., Milner, A. D., Humphreys, G. W., and Cavina-Pratesi, C. (2013). Optic ataxia affects the lower limbs: evidence from a single case study. Cortex 49, 1229–1240. doi: 10.1016/j.cortex.2012.07.008
Fazzi, E., and Micheletti, S. (2020). Questionnaires as screening tools for children with cerebral visual impairment. Dev. Med. Child Neurol. 62:891. doi: 10.1111/dmcn.14497
Feinberg, T. E., Haber, L. D., and Leeds, N. E. (1990). Verbal asomatognosia. Neurology 40, 1391–1391. doi: 10.1212/WNL.40.9.1391
Feinberg, T. E., and Venneri, A. (2014). Somatoparaphrenia: evolving theories and concepts. Cortex 61, 74–80. doi: 10.1016/j.cortex.2014.07.004
Feinberg, T. E., Venneri, A., Simone, A. M., Fan, Y., and Northoff, G. (2010). The neuroanatomy of asomatognosia and somatoparaphrenia. J. Neurol. Neurosurg. Psychiatry 81, 276–281. doi: 10.1136/jnnp.2009.188946
Felleman, D. J., and Van Essen, D. C. (1991). Distributed hierarchical processing in the primate cerebral cortex. Cereb. Cortex 1, 1–47. doi: 10.1093/cercor/1.1.1
Ferrari, F., Manzotti, R., Nalin, A., Benatti, A., Cavallo, R., Torricelli, A., et al. (1986). Visual orientation to the human face in the premature and fullterm newborn. Ital. J. Neurol. Sci. Suppl. 5, 53–60.
Ferri, S., Kolster, H., Jastorff, J., and Orban, G. A. (2013). The overlap of the EBA and the MT/V5 cluster. Neuroimage 66, 412–425. doi: 10.1016/j.neuroimage.2012.10.060
Ferro, J. M., Martins, I. P., and Távora, L. (1984). Neglect in children. Ann. Neurol. 15, 281–284. doi: 10.1002/ana.410150314
Ffytche, D. H., and Zeki, S. (1996). Brain activity related to the perception of illusory contours. Neuroimage 3, 104–108. doi: 10.1006/nimg.1996.0012
Fotopoulou, A., Jenkinson, P. M., Tsakiris, M., Haggard, P., Rudd, A., and Kopelman, M. D. (2011). Mirror-view reverses somatoparaphrenia: dissociation between first- and third-person perspectives on body ownership. Neuropsychologia 49, 3946–3955. doi: 10.1016/j.neuropsychologia.2011.10.011
Freeman, R. D., Sclar, G., and Ohzawa, I. (1982). Cortical binocularity is disrupted by strabismus more slowly than by monocular deprivation. Brain Res. 255, 311–316. doi: 10.1016/0165-3806(82)90031-1
Furlan, M., and Smith, A. T. (2016). Global motion processing in human visual cortical areas V2 and V3. J. Neurosci. 36, 7314–7324. doi: 10.1523/JNEUROSCI.0025-16.2016
Galletti, C., Gamberini, M., Kutz, D. F., Fattori, P., Luppino, G., and Matelli, M. (2001). The cortical connections of area V6: an occipito-parietal network processing visual information. Eur. J. Neurosci. 13, 1572–1588. doi: 10.1046/j.0953-816x.2001.01538.x
Gandola, M., Invernizzi, P., Sedda, A., Ferrè, E. R., Sterzi, R., Sberna, M., et al. (2012). An anatomical account of somatoparaphrenia. Cortex 48, 1165–1178. doi: 10.1016/j.cortex.2011.06.012
Gaveau, V., Pélisson, D., Blangero, A., Urquizar, C., Prablanc, C., Vighetto, A., et al. (2008). Saccade control and eye–hand coordination in optic ataxia. Neuropsychologia 46, 475–486. doi: 10.1016/j.neuropsychologia.2007.08.028
Giaschi, D., Zwicker, A., Young, S. A., and Bjornson, B. (2007). The role of cortical area V5/MT+ in speed-tuned directional anisotropies in global motion perception. Vis. Res. 47, 887–898. doi: 10.1016/j.visres.2006.12.017
Gleeson, J. G., Keeler, L. C., Parisi, M. A., Marsh, S. E., Chance, P. F., Glass, I. A., et al. (2004). Molar tooth sign of the midbrain–hindbrain junction: occurrence in multiple distinct syndromes. Am. J. Med. Genet. Part A 125, 125–134. doi: 10.1002/ajmg.a.20437
Goodale, M. A. (2013). Separate visual systems for perception and action: a framework for understanding cortical visual impairment. Dev. Med. Child Neurol. 55(Suppl. 4), 9–12. doi: 10.1111/dmcn.12299
Goodale, M. A., Króliczak, G., and Westwood, D. A. (2005). Dual routes to action: contributions of the dorsal and ventral streams to adaptive behavior. Progress Brain Res. 149, 269–283. doi: 10.1016/S0079-6123(05)49019-6
Goren, C. C., Sarty, M., and Wu, P. Y. (1975). Visual following and pattern discrimination of face-like stimuli by newborn infants. Pediatrics 56, 544–549.
Gorrie, F., Goodall, K., Rush, R., and Ravenscroft, J. (2019). Towards population screening for cerebral visual impairment: validity of the five questions and the CVI questionnaire. PLoS ONE 14:e0214290. doi: 10.1371/journal.pone.0214290
Granrud, C. E. (2006). Size constancy in infants: 4-month-olds' responses to physical versus retinal image size. J. Exp. Psychol. Human Percept. Perform. 32:1398. doi: 10.1037/0096-1523.32.6.1398
Grant, S., Suttle, C., Melmoth, D. R., Conway, M. L., and Sloper, J. J. (2014). Age-and stereovision-dependent eye–hand coordination deficits in children with amblyopia and abnormal binocularity. Investig. Ophthalmol. Visual Sci. 55, 5687–5701. doi: 10.1167/iovs.14-14745
Greulich, R. S., Adam, R., Everling, S., and Scherberger, H. (2020). Shared functional connectivity between the dorso-medial and dorso-ventral streams in macaques. Sci. Rep. 10, 1–17. doi: 10.1038/s41598-020-75219-x
Grinter, E. J., Maybery, M. T., and Badcock, D. R. (2010). Vision in developmental disorders: is there a dorsal stream deficit? Brain Res. Bull. 82, 147–160. doi: 10.1016/j.brainresbull.2010.02.016
Grossberg, S. (2014). How visual illusions illuminate complementary brain processes: illusory depth from brightness and apparent motion of illusory contours. Front. Hum. Neurosci. 8:854. doi: 10.3389/fnhum.2014.00854
Grüter, T., Grüter, M., and Carbon, C. C. (2008). Neural and genetic foundations of face recognition and prosopagnosia. J. Neuropsychol. 2, 79–97. doi: 10.1348/174866407X231001
Hainline, L., Turkel, J., Abramov, I., Lemerise, E., and Harris, C. M. (1984). Characteristics of saccades in human infants. Vis. Res. 24, 1771–1780. doi: 10.1016/0042-6989(84)90008-7
Halje, P., Seeck, M., Blanke, O., and Ionta, S. (2015). Inferior frontal oscillations reveal visuo-motor matching for actions and speech: evidence from human intracranial recordings. Neuropsychologia 79, 206–214. doi: 10.1016/j.neuropsychologia.2015.08.015
Hamm, L. M., Black, J., Dai, S., and Thompson, B. (2014). Global processing in amblyopia: a review. Front. Psychol. 5:583. doi: 10.3389/fpsyg.2014.00583
Handa, T., and Mikami, A. (2018). Neuronal correlates of motion-defined shape perception in primate dorsal and ventral streams. Eur. J. Neurosci. 48, 3171–3185. doi: 10.1111/ejn.14121
Handa, T., Unno, S., and Mikami, A. (2017). Temporal property of single-cell activity in response to motion-defined shapes in monkey dorsal and ventral cortical areas. Neuroreport 28, 793–799. doi: 10.1097/WNR.0000000000000826
Hausmann, M., Waldie, K. E., Allison, S. D., and Corballis, M. C. (2003). Line bisection following hemispherectomy. Neuropsychologia 41, 1523–1530. doi: 10.1016/S0028-3932(03)00076-9
Hess, R., Baker, C., and Zihl, J. (1989). The “motion-blind” patient: low-level spatial and temporal filters. J. Neurosci. 9, 1628–1640. doi: 10.1523/JNEUROSCI.09-05-01628.1989
Hesse, C., Ball, K., and Schenk, T. (2014). Pointing in visual periphery: is DF's dorsal stream intact? PLoS ONE 9:e91420. doi: 10.1371/journal.pone.0091420
Heydrich, L., Lopez, C., Seeck, M., and Blanke, O. (2011). Partial and full own-body illusions of epileptic origin in a child with right temporoparietal epilepsy. Epilepsy Behav. 20, 583–586. doi: 10.1016/j.yebeh.2011.01.008
Hickok, G., and Poeppel, D. (2004). Dorsal and ventral streams: a framework for understanding aspects of the functional anatomy of language. Cognition 92, 67–99. doi: 10.1016/j.cognition.2003.10.011
Himmelbach, M., and Karnath, H. O. (2005). Dorsal and ventral stream interaction: contributions from optic ataxia. J. Cogn. Neurosci. 17, 632–640. doi: 10.1162/0898929053467514
Himmelbach, M., Nau, M., Zundorf, I., Erb, M., Perenin, M. T., and Karnath, H. O. (2009). Brain activation during immediate and delayed reaching in optic ataxia. Neuropsychologia 47, 1508–1517. doi: 10.1016/j.neuropsychologia.2009.01.033
Hood, B. M., and Atkinson, J. (1993). Disengaging visual attention in the infant and adult. Infant Behav. Dev. 16, 405–422. doi: 10.1016/0163-6383(93)80001-O
Horwood, A. M., and Riddell, P. M. (2008). Gender differences in early accommodation and vergence development. Ophthalmic Physiol. Optics 28, 115–126. doi: 10.1111/j.1475-1313.2008.00547.x
Hubel, D. H., and Wiesel, T. N. (1977). Ferrier lecture-Functional architecture of macaque monkey visual cortex. Proc. R. Soc. London Ser. B Biol. Sci. 198, 1–59. doi: 10.1098/rspb.1977.0085
Husain, M., and Kennard, C. (1996). Visual neglect associated with frontal lobe infarction. J. Neurol. 243, 652–657. doi: 10.1007/BF00878662
Husain, M., and Nachev, P. (2007). Space and the parietal cortex. Trends Cogn. Sci. 11, 30–36. doi: 10.1016/j.tics.2006.10.011
Huttenlocher, P. R., De Courten, C., Garey, L. J., and Van Der Loos, H. (1982). Synaptogenesis in human visual cortex—evidence for synapse elimination during normal development. Neurosci. Lett. 33, 247–252. doi: 10.1016/0304-3940(82)90379-2
Invernizzi, P., Gandola, M., Romano, D., Zapparoli, L., Bottini, G., and Paulesu, E. (2013). What is mine? Behavioral and anatomical dissociations between somatoparaphrenia and anosognosia for hemiplegia. Behav. Neurol. 26, 139–150. doi: 10.1155/2013/548467
Ionta, S., Costantini, M., Ferretti, A., Galati, G., Romani, G. L., and Aglioti, S. M. (2020). Visual similarity and psychological closeness are neurally dissociable in the brain response to vicarious pain. Cortex 133, 295–308. doi: 10.1016/j.cortex.2020.09.028
Ionta, S., Ferretti, A., Merla, A., Tartaro, A., and Romani, G. L. (2010). Step-by-step: the effects of physical practice on the neural correlates of locomotion imagery revealed by fMRI. Hum. Brain Mapp. 31, 694–702. doi: 10.1002/hbm.20898
Ionta, S., Sforza, A., Funato, M., and Blanke, O. (2013). Anatomically plausible illusory posture affects mental rotation of body parts. Cogn. Affect. Behav. Neurosci. 13, 197–209. doi: 10.3758/s13415-012-0120-z
Jackson, S. R. (2010). Is the visual dorsal stream really very visual after all? Cogn. Neurosci. 1, 68–69. doi: 10.1080/17588920903513177
James, K. H., and Gauthier, I. (2006). Letter processing automatically recruits a sensory–motor brain network. Neuropsychologia 44, 2937–2949. doi: 10.1016/j.neuropsychologia.2006.06.026
Jissendi-Tchofo, P., Doherty, D., Mcgillivray, G., Hevner, R., Shaw, D., Ishak, G., et al. (2009). Pontine tegmental cap dysplasia: MR imaging and diffusion tensor imaging features of impaired axonal navigation. Am. J. Neuroradiol. 30, 113–119. doi: 10.3174/ajnr.A1305
Johnson, S. P., and Mason, U. (2002). Perception of kinetic illusory contours by two-month-old infants. Child Dev. 73, 22–34. doi: 10.1111/1467-8624.00389
Joshi, M. R., Simmers, A. J., and Jeon, S. T. (2020). Implied motion from form shows motion aids the perception of global form in amblyopia. Investig. Ophthalmol. Visual Sci. 61, 58–58. doi: 10.1167/iovs.61.5.58
Jurcoane, A., Choubey, B., Muckli, L., and Sireteanu, R. (2007). A pilot study for investigating cortical binocularity in humans using fMRI adaptation. Strabismus 15, 33–37. doi: 10.1080/09273970601180172
Kameyama, K., Sohya, K., Ebina, T., Fukuda, A., Yanagawa, Y., and Tsumoto, T. (2010). Difference in binocularity and ocular dominance plasticity between GABAergic and excitatory cortical neurons. J. Neurosci. 30, 1551–1559. doi: 10.1523/JNEUROSCI.5025-09.2010
Kanisza, G. (1955). Margini quasi-percettivi in campi con stimolazione omogenea. Riv. Psicol. 49, 7–30.
Kanwisher, N., Mcdermott, J., and Chun, M. M. (1997). The fusiform face area: a module in human extrastriate cortex specialized for face perception. J Neurosci. 17, 4302–4311. doi: 10.1523/JNEUROSCI.17-11-04302.1997
Kavšsek, M. J. (2002). The perception of static subjective contours in infancy. Child Dev. 73, 331–344. doi: 10.1111/1467-8624.00410
Kellman, P. J., and Spelke, E. S. (1983). Perception of partly occluded objects in infancy. Cogn. Psychol. 15, 483–524. doi: 10.1016/0010-0285(83)90017-8
Kleinman, J. T., Gailloud, P., and Jordan, L. C. (2010). Recovery from spatial neglect and hemiplegia in a child despite a large anterior circulation stroke and Wallerian degeneration. J. Child Neurol. 25, 500–503. doi: 10.1177/0883073809339060
Kondo, A., Saito, Y., Floricel, F., Maegaki, Y., and Ohno, K. (2007). Congenital ocular motor apraxia: clinical and neuroradiological findings, and long-term intellectual prognosis. Brain Dev. 29, 431–438. doi: 10.1016/j.braindev.2007.01.002
Koyama, S., Sasaki, Y., Andersen, G. J., Tootell, R. B., Matsuura, M., and Watanabe, T. (2005). Separate processing of different global-motion structures in visual cortex is revealed by FMRI. Curr. Biol. 15, 2027–2032. doi: 10.1016/j.cub.2005.10.069
Krahe, T. E., and Medina, A. E. (2010). Activation of NMDA receptors is necessary for the recovery of cortical binocularity. J. Neurophysiol. 103, 2700–2706. doi: 10.1152/jn.00442.2009
Labat, H., Ecalle, J., and Magnan, A. (2010). Effet d'entraînements bimodaux à la connaissance des lettres. Étude transversale chez des enfants de trois et cinq ans. Psychol. Fran Çaise 55, 113–127. doi: 10.1016/j.psfr.2009.12.002
Le Ber, I., Moreira, M. C., Rivaud-Pechoux, S., Chamayou, C., Ochsner, F., Kuntzer, T., et al. (2003). Cerebellar ataxia with oculomotor apraxia type 1: clinical and genetic studies. Brain 126, 2761–2772. doi: 10.1093/brain/awg283
Lepage, J. F., and Théoret, H. (2007). The mirror neuron system: grasping others' actions from birth? Dev. Sci. 10, 513–523. doi: 10.1111/j.1467-7687.2007.00631.x
Levin, N., Dumoulin, S. O., Winawer, J., Dougherty, R. F., and Wandell, B. A. (2010). Cortical maps and white matter tracts following long period of visual deprivation and retinal image restoration. Neuron 65, 21–31. doi: 10.1016/j.neuron.2009.12.006
Licari, M. K., Billington, J., Reid, S. L., Wann, J. P., Elliott, C. M., Winsor, A. M., et al. (2015). Cortical functioning in children with developmental coordination disorder: a motor overflow study. Exp. Brain Res. 233, 1703–1710. doi: 10.1007/s00221-015-4243-7
Liu, Y., Li, M., Zhang, X., Lu, Y., Gong, H., Yin, J., et al. (2020). Hierarchical representation for chromatic processing across macaque V1, V2, and V4. Neuron 108, 538–550 e535. doi: 10.1016/j.neuron.2020.07.037
Longcamp, M., Anton, J.-L., Roth, M., and Velay, J.-L. (2003). Visual presentation of single letters activates a premotor area involved in writing. Neuroimage 19, 1492–1500. doi: 10.1016/S1053-8119(03)00088-0
Longcamp, M., Zerbato-Poudou, M.-T., and Velay, J.-L. (2005). The influence of writing practice on letter recognition in preschool children: a comparison between handwriting and typing. Acta Psychol. 119, 67–79. doi: 10.1016/j.actpsy.2004.10.019
Lueck, A. H., Dutton, G. N., and Chokron, S. (2019). Profiling children with cerebral visual impairment using multiple methods of assessment to aid in differential diagnosis. Semin. Pediatr. Neurol. 31, 5–14. doi: 10.1016/j.spen.2019.05.003
Lui, L. L., Bourne, J. A., and Rosa, M. G. (2006). Functional response properties of neurons in the dorsomedial visual area of New World monkeys (Callithrix jacchus). Cereb. Cortex 16, 162–177. doi: 10.1093/cercor/bhi094
Luppino, G., Ben Hamed, S., Gamberini, M., Matelli, M., and Galletti, C. (2005). Occipital (V6) and parietal (V6A) areas in the anterior wall of the parieto-occipital sulcus of the macaque: a cytoarchitectonic study. Eur. J. Neurosci. 21, 3056–3076. doi: 10.1111/j.1460-9568.2005.04149.x
Lv, X., Chen, Y., Tan, W., Yu, Y., Zou, H., Shao, Y., et al. (2020). Functional neuroanatomy of the human accommodation response to an “E” target varying from-3 to-6 diopters. Front. Integr. Neurosci. 14:29. doi: 10.3389/fnint.2020.00029
Machner, B., Klein, C., Sprenger, A., Baumbach, P., Pramstaller, P. P., Helmchen, C., et al. (2010). Eye movement disorders are different in Parkin-linked and idiopathic early-onset PD. Neurology 75, 125–128. doi: 10.1212/WNL.0b013e3181e7ca6d
Maglione, A. G., Brizi, A., Vecchiato, G., Rossi, D., Trettel, A., Modica, E., et al. (2017). A neuroelectrical brain imaging study on the perception of figurative paintings against only their color or shape contents. Front. Hum. Neurosci. 11:378. doi: 10.3389/fnhum.2017.00378
Manny, R. (1988). The visually evoked potential in response to vernier offsets in infants. Human Neurobiol 6, 273–279.
Maria, B. L., Quisling, R. G., Rosainz, L. C., Yachnis, A. T., Gitten, J., Dede, D., et al. (1999). Molar tooth sign in Joubert syndrome: clinical, radiologic, and pathologic significance. J. Child Neurol. 14, 368–376. doi: 10.1177/088307389901400605
Marsh, E. B., Newhart, M., Kleinman, J. T., Heidler-Gary, J., Vining, E. P., Freeman, J. M., et al. (2009). Hemispherectomy sustained before adulthood does not cause persistent hemispatial neglect. Cortex 45, 677–685. doi: 10.1016/j.cortex.2008.06.012
Marshall, P. J., Young, T., and Meltzoff, A. N. (2011). Neural correlates of action observation and execution in 14-month-old infants: an event-related EEG desynchronization study. Dev. Sci. 14, 474–480. doi: 10.1111/j.1467-7687.2010.00991.x
Martin, A., Ungerleider, L. G., and Haxby, J. V. (2000). “Categoryspecificity and the brain: the sensory-motor model of semantic representations of objects,” in The New Cognitive Neurosciences, 2nd Edn., ed M. S. Gazzaniga (Cambridge, MA: MIT Press), 1023–1036.
Martin, K., and Trauner, D. A. (2019). Auditory neglect in children following perinatal stroke. Behav. Brain Res. 359, 878–885. doi: 10.1016/j.bbr.2018.06.026
Maruko, I., Zhang, B., Tao, X., Tong, J., Smith Iii, E. L., and Chino, Y. M. (2008). Postnatal development of disparity sensitivity in visual area 2 (v2) of macaque monkeys. J. Neurophysiol. 100, 2486–2495. doi: 10.1152/jn.90397.2008
Mason, A., Braddick, O., and Wattam-Bell, J. (2003). Motion coherence thresholds in infants—different tasks identify at least two distinct motion systems. Vis. Res. 43, 1149–1157. doi: 10.1016/S0042-6989(03)00077-4
Maunsell, J. H., and Van Essen, D. C. (1983). The connections of the middle temporal visual area (MT) and their relationship to a cortical hierarchy in the macaque monkey. J. Neurosci. 3, 2563–2586. doi: 10.1523/JNEUROSCI.03-12-02563.1983
Mayer, E., and Rossion, B. (2007). “Prosopagnosia,” in The Behavioral and Cognitive Neurology of Stroke, eds O. Godefroy, and J. Bogousslavsky (Cambridge: Cambridge University Press), 315–334.
Meier, K., and Giaschi, D. (2014). The maturation of global motion perception depends on the spatial and temporal offsets of the stimulus. Vis. Res. 95, 61–67. doi: 10.1016/j.visres.2013.12.007
Meltzoff, A. N., and Moore, M. K. (1977). Imitation of facial and manual gestures by human neonates. Science 198, 75–78. doi: 10.1126/science.198.4312.75
Mercuri, E., Atkinson, J., Braddick, O., Anker, S., Cowan, F., Rutherford, M., et al. (1997a). Basal ganglia damage and impaired visual function in the newborn infant. Arch. Dis. Childhood Fetal Neonatal Edn. 77, F111–F114. doi: 10.1136/fn.77.2.F111
Mercuri, E., Atkinson, J., Braddick, O., Anker, S., Cowan, F., Rutherford, M., et al. (1997b). Visual function in full-term infants with hypoxic-ischaemic encephalopathy. Neuropediatrics 28, 155–161. doi: 10.1055/s-2007-973693
Mercuri, E., Braddick, O., Atkinson, J., Cowan, F., Anker, S., Andrew, R., et al. (1998). Orientation-reversal and phase-reversal visual evoked potentials in full-term infants with brain lesions: a longitudinal study. Neuropediatrics 29, 169–174. doi: 10.1055/s-2007-973556
Merlini, L., Vargas, M. I., De Haller, R., Rilliet, B., and Fluss, J. (2010). MRI with fibre tracking in Cogan congenital oculomotor apraxia. Pediatr. Radiol. 40, 1625–1633. doi: 10.1007/s00247-010-1653-3
Mikellidou, K., Arrighi, R., Aghakhanyan, G., Tinelli, F., Frijia, F., Crespi, S., et al. (2019). Plasticity of the human visual brain after an early cortical lesion. Neuropsychologia 128, 166–177. doi: 10.1016/j.neuropsychologia.2017.10.033
Milner, A. D., and Goodale, M. A. (2008). Two visual systems re-viewed. Neuropsychologia 46, 774–785. doi: 10.1016/j.neuropsychologia.2007.10.005
Mirkowski, M., Mcintyre, A., Faltynek, P., Sequeira, N., Cassidy, C., and Teasell, R. (2019). Nonpharmacological rehabilitation interventions for motor and cognitive outcomes following pediatric stroke: a systematic review. Eur. J. Pediatr. 178, 433–454. doi: 10.1007/s00431-019-03350-7
Mirzajani, A., Ghorbani, M., Rasuli, B., and Mahmoud-Pashazadeh, A. (2017). Effect of induced high myopia on functional MRI signal changes. Phys. Med. 37, 32–36. doi: 10.1016/j.ejmp.2017.04.004
Morris, J. S., Degelder, B., Weiskrantz, L., and Dolan, R. J. (2001). Differential extrageniculostriate and amygdala responses to presentation of emotional faces in a cortically blind field. Brain 124, 1241–1252. doi: 10.1093/brain/124.6.1241
Morrone, M. C., Atkinson, J., Cioni, G., Braddick, O. J., and Fiorentini, A. (1999). Developmental changes in optokinetic mechanisms in the absence of unilateral cortical control. Neuroreport 10, 2723–2729. doi: 10.1097/00001756-199909090-00006
Mort, D. J., Malhotra, P., Mannan, S. K., Rorden, C., Pambakian, A., Kennard, C., et al. (2003). The anatomy of visual neglect. Brain 126, 1986–1997. doi: 10.1093/brain/awg200
Moscovitch, M., Winocur, G., and Behrmann, M. (1997). What is special about face recognition? Nineteen experiments on a person with visual object agnosia and dyslexia but normal face recognition. J. Cogn. Neurosci. 9, 555–604. doi: 10.1162/jocn.1997.9.5.555
Muñoz-Moreno, E., Fischi-Gomez, E., Batalle, D., Borradori-Tolsa, C., Eixarch, E., Thiran, J.-P., et al. (2016). Structural brain network reorganization and social cognition related to adverse perinatal condition from infancy to early adolescence. Front. Neurosci. 10:560. doi: 10.3389/fnins.2016.00560
Mustari, M. J., and Ono, S. (2011). Neural mechanisms for smooth pursuit in strabismus. Ann. N. Y. Acad. Sci. 1233, 187–193. doi: 10.1111/j.1749-6632.2011.06117.x
Mustari, M. J., Ono, S., and Vitorello, K. C. (2008). How disturbed visual processing early in life leads to disorders of gaze-holding and smooth pursuit. Progress Brain Res. 171, 487–495. doi: 10.1016/S0079-6123(08)00670-5
Myers, A., and Sowden, P. T. (2008). Your hand or mine? The extrastriate body area. Neuroimage 42, 1669–1677. doi: 10.1016/j.neuroimage.2008.05.045
Naarden, T., Ter Meulen, B. C., Van Der Weele, S. I., and Blom, J. D. (2019). Alice in Wonderland syndrome as a presenting manifestation of Creutzfeldt-Jakob disease. Front. Neurol. 10:473. doi: 10.3389/fneur.2019.00473
Nakatsuka, C., Zhang, B., Watanabe, I., Zheng, J., Bi, H., Ganz, L., et al. (2007). Effects of perceptual learning on local stereopsis and neuronal responses of V1 and V2 in prism-reared monkeys. J. Neurophysiol. 97, 2612–2626. doi: 10.1152/jn.01001.2006
Nardini, M., Bedford, R., and Mareschal, D. (2010). Fusion of visual cues is not mandatory in children. Proc. Natl. Acad. Sci. U.S.A. 107, 17041–17046. doi: 10.1073/pnas.1001699107
Newman, C., Atkinson, J., and Braddick, O. (2001). The development of reaching and looking preferences in infants to objects of different sizes. Dev. Psychol. 37:561. doi: 10.1037/0012-1649.37.4.561
Newsome, W. T., and Pare, E. B. (1988). A selective impairment of motion perception following lesions of the middle temporal visual area (MT). J. Neurosci. 8, 2201–2211. doi: 10.1523/JNEUROSCI.08-06-02201.1988
Niechwiej-Szwedo, E., Colpa, L., and Wong, A. M. F. (2019). Visuomotor behaviour in amblyopia: deficits and compensatory adaptations. Neural. Plast. 2019:6817839. doi: 10.1155/2019/6817839
Nobusako, S., Sakai, A., Tsujimoto, T., Shuto, T., Nishi, Y., Asano, D., et al. (2018). Deficits in visuo-motor temporal integration impacts manual dexterity in probable developmental coordination disorder. Front. Neurol. 9:114. doi: 10.3389/fneur.2018.00114
Norcia, A. M., and Tyler, C. W. (1985). Spatial frequency sweep VEP: visual acuity during the first year of life. Vis. Res. 25, 1399–1408. doi: 10.1016/0042-6989(85)90217-2
Ohtsuki, H., Yoshifumi, K., Hasebe, S., Kono, R., and Harada, Y. (2000). Comparative study of brain lesions detected by magnetic resonance imaging between strabismus and nonstrabismus in infancy. Ophthalmologica 214, 105–110. doi: 10.1159/000027476
Okamoto, Y., Kosaka, H., Kitada, R., Seki, A., Tanabe, H. C., Hayashi, M. J., et al. (2017). Age-dependent atypicalities in body- and face-sensitive activation of the EBA and FFA in individuals with ASD. Neurosci. Res. 119, 38–52. doi: 10.1016/j.neures.2017.02.001
Ortibus, E., Fazzi, E., and Dale, N. (2019). Cerebral visual impairment and clinical assessment: the european perspective. Semin. Pediatr. Neurol. 31, 15–24. doi: 10.1016/j.spen.2019.05.004
Otsuka, Y., and Yamaguchi, M. K. (2003). Infants' perception of illusory contours in static and moving figures. J. Exp. Child Psychol. 86, 244–251. doi: 10.1016/S0022-0965(03)00126-7
Otsuka-Hirota, N., Yamamoto, H., Miyashita, K., and Nagatsuka, K. (2014). Invisibility of moving objects: a core symptom of motion blindness. Case. Rep. 2014:bcr2013201233. doi: 10.1136/bcr-2013-201233
Pavlova, M., Sokolov, A., Staudt, M., Marconato, F., Birbaumer, N., and Krägeloh-Mann, I. (2005). Recruitment of periventricular parietal regions in processing cluttered point-light biological motion. Cereb. Cortex 15, 594–601. doi: 10.1093/cercor/bhh162
Peelen, M. V., and Downing, P. E. (2007). The neural basis of visual body perception. Nat. Rev. Neurosci. 8, 636–648. doi: 10.1038/nrn2195
Peelen, M. V., Glaser, B., Vuilleumier, P., and Eliez, S. (2009). Differential development of selectivity for faces and bodies in the fusiform gyrus. Dev. Sci. 12, F16–25. doi: 10.1111/j.1467-7687.2009.00916.x
Pena-Casanova, J., Roig-Rovira, T., Bermudez, A., and Tolosa-Sarro, E. (1985). Optic aphasia, optic apraxia, and loss of dreaming. Brain Lang. 26, 63–71. doi: 10.1016/0093-934X(85)90028-8
Perruchoud, D., Michels, L., Piccirelli, M., Gassert, R., and Ionta, S. (2016). Differential neural encoding of sensorimotor and visual body representations. Sci. Rep. 6:37259. doi: 10.1038/srep37259
Perruchoud, D., Murray, M. M., Lefebvre, J., and Ionta, S. (2014). Focal dystonia and the sensory-motor integrative loop for enacting (SMILE). Front. Hum. Neurosci. 8:458. doi: 10.3389/fnhum.2014.00458
Philip, S. S., Mani, S. E., and Dutton, G. N. (2016). Pediatric Balint's syndrome variant: a possible diagnosis in children. Case. Rep. Ophthalmol. Med. 2016:3806056. doi: 10.1155/2016/3806056
Phillips, J. O., Finocchio, D. V., Ong, L., and Fuchs, A. F. (1997). Smooth pursuit in 1-to 4-month-old human infants. Vis. Res. 37, 3009–3020. doi: 10.1016/S0042-6989(97)00107-7
Pisella, L., Sergio, L., Blangero, A., Torchin, H., Vighetto, A., and Rossetti, Y. (2009). Optic ataxia and the function of the dorsal stream: contributions to perception and action. Neuropsychologia 47, 3033–3044. doi: 10.1016/j.neuropsychologia.2009.06.020
Pitzalis, S., Fattori, P., and Galletti, C. (2013). The functional role of the medial motion area V6. Front. Behav. Neurosci. 6:91. doi: 10.3389/fnbeh.2012.00091
Pitzalis, S., Fattori, P., and Galletti, C. (2015). The human cortical areas V6 and V6A. Vis. Neurosci. 32:E007. doi: 10.1017/S0952523815000048
Ponce, C. R., Lomber, S. G., and Livingstone, M. S. (2017). Posterior inferotemporal cortex cells use multiple input pathways for shape encoding. J. Neurosci. 37, 5019–5034. doi: 10.1523/JNEUROSCI.2674-16.2017
Pullela, M., Agaoglu, M. N., Joshi, A. C., Agaoglu, S., Coats, D. K., and Das, V. E. (2018). Neural plasticity following surgical correction of strabismus in monkeys. Invest. Ophthalmol. Vis. Sci. 59, 5011–5021. doi: 10.1167/iovs.18-25245
Pulvermüller, F. (1999). Words in the brain s language. Behav. Brain Sci. 22, 253–279. doi: 10.1017/S0140525X9900182X
Qiu, F. T., and Von Der Heydt, R. (2005). Figure and ground in the visual cortex: V2 combines stereoscopic cues with Gestalt rules. Neuron 47, 155–166. doi: 10.1016/j.neuron.2005.05.028
Reynolds, J. E., Billington, J., Kerrigan, S., Williams, J., Elliott, C., Winsor, A. M., et al. (2019). Mirror neuron system activation in children with developmental coordination disorder: a replication functional MRI study. Res. Dev. Disabil. 84, 16–27. doi: 10.1016/j.ridd.2017.11.012
Reynolds, J. E., Kerrigan, S., Elliott, C., Lay, B. S., and Licari, M. K. (2017). Poor imitative performance of unlearned gestures in children with probable developmental coordination disorder. J. Motor Behav. 49, 378–387. doi: 10.1080/00222895.2016.1219305
Ricci, D., Chieffo, D., Battaglia, D., Brogna, C., Contaldo, I., De Clemente, V., et al. (2015). A prospective longitudinal study on visuo-cognitive development in Dravet syndrome: is there a “dorsal stream vulnerability?” Epilepsy Res. 109, 57–64. doi: 10.1016/j.eplepsyres.2014.10.009
Richter, H. O., Lee, J. T., and Pardo, J. V. (2000). Neuroanatomical correlates of the near response: voluntary modulation of accommodation/vergence in the human visual system. Eur. J. Neurosci. 12, 311–321. doi: 10.1046/j.1460-9568.2000.00962.x
Rizzolatti, G., and Matelli, M. (2003). Two different streams form the dorsal visual system. Exp. Brain Res. 153:146. doi: 10.1007/s00221-003-1588-0
Robertson, C. E., Thomas, C., Kravitz, D. J., Wallace, G. L., Baron-Cohen, S., Martin, A., et al. (2014). Global motion perception deficits in autism are reflected as early as primary visual cortex. Brain 137, 2588–2599. doi: 10.1093/brain/awu189
Roe, A. W., Chelazzi, L., Connor, C. E., Conway, B. R., Fujita, I., Gallant, J. L., et al. (2012). Toward a unified theory of visual area V4. Neuron 74, 12–29. doi: 10.1016/j.neuron.2012.03.011
Romano, D., Gandola, M., Bottini, G., and Maravita, A. (2014). Arousal responses to noxious stimuli in somatoparaphrenia and anosognosia: clues to body awareness. Brain 137, 1213–1223. doi: 10.1093/brain/awu009
Rosa Salva, O., Regolin, L., and Vallortigara, G. (2012). Inversion of contrast polarity abolishes spontaneous preferences for face-like stimuli in newborn chicks. Behav. Brain Res. 228, 133–143. doi: 10.1016/j.bbr.2011.11.025
Rosander, K. (2007). Visual tracking and its relationship to cortical development. Progress Brain Res. 164, 105–122. doi: 10.1016/S0079-6123(07)64006-0
Rosenbaum, D. A., Vaughan, J., Barnes, H. J., and Jorgensen, M. J. (1992). Time course of movement planning: selection of handgrips for object manipulation. J. Exp. Psychol. Learn. Memory Cogn. 18:1058. doi: 10.1037/0278-7393.18.5.1058
Rossetti, Y., and Pisella, L. (2018). Optic ataxia: beyond the dorsal stream cliche. Handb. Clin. Neurol. 151, 225–247. doi: 10.1016/B978-0-444-63622-5.00011-5
Rossetti, Y., Pisella, L., and Mcintosh, R. D. (2017). Rise and fall of the two visual systems theory. Ann. Phys. Rehabil. Med. 60, 130–140. doi: 10.1016/j.rehab.2017.02.002
Rossetti, Y., Rode, G., Pisella, L., Farné, A., Li, L., Boisson, D., et al. (1998). Prism adaptation to a rightward optical deviation rehabilitates left hemispatial neglect. Nature 395, 166–169. doi: 10.1038/25988
Rudolph Bálint, D. (1909). Seelenlähmung des “Schauens”, optische Ataxie, räumliche Störung der Aufmerksamkeit. pp. 67–81. Euro. Neurol. 25, 67–81. doi: 10.1159/000210465
Ruffieux, N., Ramon, M., Lao, J., Colombo, F., Stacchi, L., Borruat, F.-X., et al. (2016). Residual perception of biological motion in cortical blindness. Neuropsychologia 93, 301–311. doi: 10.1016/j.neuropsychologia.2016.11.009
Sargent, M. A., Poskitt, K. J., and Jan, J. E. (1997). Congenital ocular motor apraxia: imaging findings. Am. J. Neuroradiol. 18, 1915–1922.
Saygin, A. P., Wilson, S. M., Hagler, D. J. Jr., Bates, E., and Sereno, M. I. (2004). Point-light biological motion perception activates human premotor cortex. J. Neurosci. 24, 6181–6188. doi: 10.1523/JNEUROSCI.0504-04.2004
Schindler, I., Rice, N. J., Mcintosh, R. D., Rossetti, Y., Vighetto, A., and Milner, A. D. (2004). Automatic avoidance of obstacles is a dorsal stream function: evidence from optic ataxia. Nat. Neurosci. 7, 779–784. doi: 10.1038/nn1273
Schmalzl, L., Palermo, R., Green, M., Brunsdon, R., and Coltheart, M. (2008). Training of familiar face recognition and visual scan paths for faces in a child with congenital prosopagnosia. Cogn. Neuropsychol. 25, 704–729. doi: 10.1080/02643290802299350
Sciberras-Lim, E. T., and Lambert, A. J. (2017). Attentional orienting and dorsal visual stream decline: review of behavioral and EEG studies. Front. Aging Neurosci. 9:246. doi: 10.3389/fnagi.2017.00246
Sengpiel, F., and Blakemore, C. (1996). The neural basis of suppression and amblyopia in strabismus. Eye 10(Pt 2), 250–258. doi: 10.1038/eye.1996.54
Serra, C., Galletti, C., Di Marco, S., Fattori, P., Galati, G., Sulpizio, V., et al. (2019). Egomotion-related visual areas respond to active leg movements. Hum. Brain Mapp. 40, 3174–3191. doi: 10.1002/hbm.24589
Shahwan, A., Byrd, P. J., Taylor, A. M., Nestor, T., Ryan, S., and King, M. D. (2006). Atypical presentation of ataxia-oculomotor apraxia type 1. Dev. Med. Child Neurol. 48, 529–532. doi: 10.1017/S0012162206001113
Sheehan, K. J., and Uttal, D. H. (2016). Children's learning from touch screens: a dual representation perspective. Front. Psychol. 7:1220. doi: 10.3389/fpsyg.2016.01220
Shipp, S. (2004). The brain circuitry of attention. Trends Cogn. Sci. 8, 223–230. doi: 10.1016/j.tics.2004.03.004
Shipp, S., Blanton, M., and Zeki, S. (1998). A visuo-somatomotor pathway through superior parietal cortex in the macaque monkey: cortical connections of areas V6 and V6A. Eur. J. Neurosci. 10, 3171–3193. doi: 10.1046/j.1460-9568.1998.00327.x
Shipp, S., Jong, B. D., Zihl, J., Frackowiak, R., and Zeki, S. (1994). The brain activity related to residual motion vision in a patient with bilateral lesions of V5. Brain 117, 1023–1038. doi: 10.1093/brain/117.5.1023
Simion, F., Di Giorgio, E., Leo, I., and Bardi, L. (2011). The processing of social stimuli in early infancy: from faces to biological motion perception. Progress. Brain Res. 189, 173–193. doi: 10.1016/B978-0-444-53884-0.00024-5
Simmers, A. J., Ledgeway, T., Hess, R. F., and Mcgraw, P. V. (2003). Deficits to global motion processing in human amblyopia. Vis. Res. 43, 729–738. doi: 10.1016/S0042-6989(02)00684-3
Simpson, E. A., Murray, L., Paukner, A., and Ferrari, P. F. (2014). The mirror neuron system as revealed through neonatal imitation: presence from birth, predictive power and evidence of plasticity. Philos. Trans. R. Soc. B Biol. Sci. 369:20130289. doi: 10.1098/rstb.2013.0289
Sincich, L. C., Park, K. F., Wohlgemuth, M. J., and Horton, J. C. (2004). Bypassing V1: a direct geniculate input to area MT. Nat. Neurosci. 7, 1123–1128. doi: 10.1038/nn1318
Skoczenski, A. M., and Norcia, A. M. (1999). Development of VEP Vernier acuity and grating acuity in human infants. Investig. Ophthalmol. Visual Sci. 40, 2411–2417.
Skoczenski, A. M., and Norcia, A. M. (2002). Late maturation of visual hyperacuity. Psychol. Sci. 13, 537–541. doi: 10.1111/1467-9280.00494
Slater, A., Morison, V., and Somers, M. (1988). Orientation discrimination and cortical function in the human newborn. Perception 17, 597–602. doi: 10.1068/p170597
Smith, A. T., Beer, A. L., Furlan, M., and Mars, R. B. (2018). Connectivity of the cingulate sulcus visual area (CSv) in the human cerebral cortex. Cereb. Cortex 28, 713–725. doi: 10.1093/cercor/bhx002
Smyth, M. M., and Mason, U. C. (1997). Planning and execution of action in children with and without developmental coordination disorder. J. Child Psychol. Psychiatry 38, 1023–1037. doi: 10.1111/j.1469-7610.1997.tb01619.x
Southgate, V., Johnson, M. H., Karoui, I. E., and Csibra, G. (2010). Motor system activation reveals infants' on-line prediction of others' goals. Psychol. Sci. 21, 355–359. doi: 10.1177/0956797610362058
Southgate, V., Johnson, M. H., Osborne, T., and Csibra, G. (2009). Predictive motor activation during action observation in human infants. Biol. Lett. 5, 769–772. doi: 10.1098/rsbl.2009.0474
Spencer, J., O'brien, J., Riggs, K., Braddick, O., Atkinson, J., and Wattam-Bell, J. (2000). Motion processing in autism: evidence for a dorsal stream deficiency. Neuroreport 11, 2765–2767. doi: 10.1097/00001756-200008210-00031
Stepniewska, I., Cerkevich, C. M., and Kaas, J. H. (2016). Cortical connections of the caudal portion of posterior parietal cortex in prosimian galagos. Cereb. Cortex 26, 2753–2777. doi: 10.1093/cercor/bhv132
Strasburger, H., Huber, J., and Rose, D. (2018). Ewald Hering's (1899) on the limits of visual acuity: a translation and commentary: with a supplement on Alfred Volkmann's (1863) physiological investigations in the field of optics. i-Perception 9:2041669518763675. doi: 10.1177/2041669518763675
Tadros, M. A., Lim, R., Hughes, D. I., Brichta, A. M., and Callister, R. J. (2015). Electrical maturation of spinal neurons in the human fetus: comparison of ventral and dorsal horn. J. Neurophysiol. 114, 2661–2671. doi: 10.1152/jn.00682.2015
Teixeira, S., Machado, S., Velasques, B., Sanfim, A., Minc, D., Peressutti, C., et al. (2014). Integrative parietal cortex processes: neurological and psychiatric aspects. J. Neurol. Sci. 338, 12–22. doi: 10.1016/j.jns.2013.12.025
Thareja, T., Ballantyne, A. O., and Trauner, D. A. (2012). Spatial analysis after perinatal stroke: patterns of neglect and exploration in extra-personal space. Brain Cogn. 79, 107–116. doi: 10.1016/j.bandc.2012.02.009
Thompson, N. M., Ewing-Cobbs, L., Fletcher, J. M., Miner, M. E., and Levin, H. S. (1991). Left unilateral neglect in a preschool child. Dev. Med. Child Neurol. 33, 640–644. doi: 10.1111/j.1469-8749.1991.tb14935.x
Thorn, F., Gwiazda, J., Cruz, A., Bauer, J. A., and Held, R. (1994). The development of eye alignment, convergence, and sensory binocularity in young infants. Investig. Ophthalmol. Visual Sci. 35, 544–553.
Tinelli, F., Cicchini, G. M., Arrighi, R., Tosetti, M., Cioni, G., and Morrone, M. C. (2013). Blindsight in children with congenital and acquired cerebral lesions. Cortex 49, 1636–1647. doi: 10.1016/j.cortex.2012.07.005
Ting Siok, W., Kay, P., Wang, W. S., Chan, A. H., Chen, L., Luke, K. K., et al. (2009). Language regions of brain are operative in color perception. Proc. Natl. Acad. Sci. U.S.A. 106, 8140–8145. doi: 10.1073/pnas.0903627106
Tong, F. (2003). Primary visual cortex and visual awareness. Nat. Rev. Neurosci. 4, 219–229. doi: 10.1038/nrn1055
Tonks, J., Cloke, G., Lee-Kelland, R., Jary, S., Thoresen, M., Cowan, F. M., et al. (2019). Attention and visuo-spatial function in children without cerebral palsy who were cooled for neonatal encephalopathy: a case-control study. Brain Inj. 33, 894–898. doi: 10.1080/02699052.2019.1597163
Tran, T. H., Guyader, N., Guerin, A., Despretz, P., and Boucart, M. (2011). Figure ground discrimination in age-related macular degeneration. Invest. Ophthalmol. Vis. Sci. 52, 1655–1660. doi: 10.1167/iovs.10-6003
Trauner, D. A. (2003). Hemispatial neglect in young children with early unilateral brain damage. Dev. Med. Child Neurol. 45, 160–166. doi: 10.1111/j.1469-8749.2003.tb00924.x
Tsao, C. Y., and Paulson, G. (2005). Type 1 ataxia with oculomotor apraxia with aprataxin gene mutations in two American children. J. Child Neurol. 20, 619–620. doi: 10.1177/08830738050200071701
Tychsen, L. (2007). Causing and curing infantile esotropia in primates: the role of decorrelated binocular input (an American Ophthalmological Society thesis). Trans. Am. Ophthalmol. Soc. 105, 564–593.
Ungerleider, L. G., and Desimone, R. (1986). Cortical connections of visual area MT in the macaque. J. Compar. Neurol. 248, 190–222. doi: 10.1002/cne.902480204
Urgesi, C., Candidi, M., Ionta, S., and Aglioti, S. M. (2007). Representation of body identity and body actions in extrastriate body area and ventral premotor cortex. Nat. Neurosci. 10, 30–31. doi: 10.1038/nn1815
Vachon, P., Voss, P., Lassonde, M., Leroux, J. M., Mensour, B., Beaudoin, G., et al. (2009). Global motion stimuli and form-from-motion stimuli: common characteristics and differential activation patterns. Int. J. Neurosci. 119, 1584–1601. doi: 10.1080/00207450802328367
Vallar, G., and Ronchi, R. (2009). Somatoparaphrenia: a body delusion. A review of the neuropsychological literature. Exp. Brain Res. 192, 533–551. doi: 10.1007/s00221-008-1562-y
Van Elk, M., Van Schie, H. T., Hunnius, S., Vesper, C., and Bekkering, H. (2008). You'll never crawl alone: neurophysiological evidence for experience-dependent motor resonance in infancy. Neuroimage 43, 808–814. doi: 10.1016/j.neuroimage.2008.07.057
Van Polanen, V., and Davare, M. (2015). Interactions between dorsal and ventral streams for controlling skilled grasp. Neuropsychologia 79, 186–191. doi: 10.1016/j.neuropsychologia.2015.07.010
Vicari, S., Stiles, J., Stern, C., and Resca, A. (1998). Spatial grouping activity in children with early cortical and subcortical lesions. Dev. Med. Child Neurol. 40, 90–94. doi: 10.1111/j.1469-8749.1998.tb15367.x
Von Der Heydt, R., Peterhans, E., and Baumgartner, G. (1984). Illusory contours and cortical neuron responses. Science 224, 1260–1262. doi: 10.1126/science.6539501
Von Der Heydt, R., Zhou, H., and Friedman, H. S. (2000). Representation of stereoscopic edges in monkey visual cortex. Vis. Res. 40, 1955–1967. doi: 10.1016/S0042-6989(00)00044-4
Von Hofsten, O., Von Hofsten, C., Sulutvedt, U., Laeng, B., Brennen, T., and Magnussen, S. (2014). Simulating newborn face perception. J. Vis. 14:16. doi: 10.1167/14.13.16
Walbrin, J., Mihai, I., Landsiedel, J., and Koldewyn, K. (2020). Developmental changes in visual responses to social interactions. Dev. Cogn. Neurosci. 42:100774. doi: 10.1016/j.dcn.2020.100774
Walton, M. M. G., Pallus, A., Fleuriet, J., Mustari, M. J., and Tarczy-Hornoch, K. (2017). Neural mechanisms of oculomotor abnormalities in the infantile strabismus syndrome. J. Neurophysiol. 118, 280–299. doi: 10.1152/jn.00934.2016
Warner, C. E., Goldshmit, Y., and Bourne, J. A. (2010). Retinal afferents synapse with relay cells targeting the middle temporal area in the pulvinar and lateral geniculate nuclei. Front. Neuroanatomy 4:8. doi: 10.3389/neuro.05.008.2010
Warner, C. E., Kwan, W. C., and Bourne, J. A. (2012). The early maturation of visual cortical area MT is dependent on input from the retinorecipient medial portion of the inferior pulvinar. J. Neurosci. 32, 17073–17085. doi: 10.1523/JNEUROSCI.3269-12.2012
Wattam-Bell, J. (1991). Development of motion-specific cortical responses in infancy. Vis. Res. 31, 287–297. doi: 10.1016/0042-6989(91)90119-P
Wattam-Bell, J. (1992). The development of maximum displacement limits for discrimination of motion direction in infancy. Vis. Res. 32, 621–630. doi: 10.1016/0042-6989(92)90178-L
Wattam-Bell, J., Birtles, D., Nyström, P., Von Hofsten, C., Rosander, K., Anker, S., et al. (2010). Reorganization of global form and motion processing during human visual development. Curr. Biol. 20, 411–415. doi: 10.1016/j.cub.2009.12.020
Weigelt, S. (2007). Seeing things: illusory contours in the human visual brain. J. Neurosci. 27, 5269–5270. doi: 10.1523/JNEUROSCI.1126-07.2007
Werner, J. M., Cermak, S. A., and Aziz-Zadeh, L. (2012). Neural correlates of developmental coordination disorder: the mirror neuron system hypothesis. J. Behav. Brain Sci. 2, 258–268. doi: 10.4236/jbbs.2012.22029
Wilson, C. E., Palermo, R., Schmalzl, L., and Brock, J. (2010). Specificity of impaired facial identity recognition in children with suspected developmental prosopagnosia. Cogn. Neuropsychol. 27, 30–45. doi: 10.1080/02643294.2010.490207
Wong, E. H., Levi, D. M., and Mcgraw, P. V. (2001). Is second-order spatial loss in amblyopia explained by the loss of first-order spatial input? Vis. Res. 41, 2951–2960. doi: 10.1016/S0042-6989(01)00189-4
Wuerger, S. M., Parkes, L., Lewis, P. A., Crocker-Buque, A., Rutschmann, R., and Meyer, G. F. (2012). Premotor cortex is sensitive to auditory-visual congruence for biological motion. J. Cogn. Neurosci. 24, 575–587. doi: 10.1162/jocn_a_00173
Yonas, A., Elieff, C. A., and Arterberry, M. E. (2002). Emergence of sensitivity to pictorial depth cues: charting development in individual infants. Infant Behav. Dev. 25, 495–514. doi: 10.1016/S0163-6383(02)00147-9
Yoto, A., Katsuura, T., Iwanaga, K., and Shimomura, Y. (2007). Effects of object color stimuli on human brain activities in perception and attention referred to EEG alpha band response. J. Physiol. Anthropol. 26, 373–379. doi: 10.2114/jpa2.26.373
Yousefian, O., Ballantyne, A. O., Doo, A., and Trauner, D. A. (2015). Clock drawing in children with perinatal stroke. Pediatric Neurol. 52, 592–598. doi: 10.1016/j.pediatrneurol.2014.07.036
Yuodelis, C., and Hendrickson, A. (1986). A qualitative and quantitative analysis of the human fovea during development. Vis. Res. 26, 847–855. doi: 10.1016/0042-6989(86)90143-4
Zanker, J., Mohn, G., Weber, U., Zeitler-Driess, K., and Fahle, M. (1992). The development of vernier acuity in human infants. Vis. Res. 32, 1557–1564. doi: 10.1016/0042-6989(92)90211-Z
Zeki, S. (1991). Cerebral akinetopsia (visual motion blindness). A review. Brain 114(Pt 2), 811–824. doi: 10.1093/brain/114.2.811
Zeugin, D., Notter, M. P., Knebel, J. F., and Ionta, S. (2020). Temporo-parietal contribution to the mental representations of self/other face. Brain Cogn. 143:105600. doi: 10.1016/j.bandc.2020.105600
Zheng, J., Zhang, B., Bi, H., Maruko, I., Watanabe, I., Nakatsuka, C., et al. (2007). Development of temporal response properties and contrast sensitivity of V1 and V2 neurons in macaque monkeys. J. Neurophysiol. 97, 3905–3916. doi: 10.1152/jn.01320.2006
Zhexenova, Z., Amirova, A., Abdikarimova, M., Kudaibergenov, K., Baimakhan, N., Tleubayev, B., et al. (2020). A comparison of social robot to tablet and teacher in a new script learning context. Front. Robot. AI 7:99. doi: 10.3389/frobt.2020.00099
Keywords: vision, movement, brain, child, sensorimotor
Citation: Ionta S (2021) Visual Neuropsychology in Development: Anatomo-Functional Brain Mechanisms of Action/Perception Binding in Health and Disease. Front. Hum. Neurosci. 15:689912. doi: 10.3389/fnhum.2021.689912
Received: 01 April 2021; Accepted: 06 May 2021;
Published: 31 May 2021.
Edited by:
Cosimo Urgesi, University of Udine, ItalyCopyright © 2021 Ionta. This is an open-access article distributed under the terms of the Creative Commons Attribution License (CC BY). The use, distribution or reproduction in other forums is permitted, provided the original author(s) and the copyright owner(s) are credited and that the original publication in this journal is cited, in accordance with accepted academic practice. No use, distribution or reproduction is permitted which does not comply with these terms.
*Correspondence: Silvio Ionta, aW9udGEuc2lsdmlvJiN4MDAwNDA7Z21haWwuY29t