- 1Institute for General Kinesiology and Exercise Science, Faculty of Sport Science, University of Leipzig, Leipzig, Germany
- 2Department of Neurology, Max Planck Institute for Human Cognitive and Brain Sciences, Leipzig, Germany
Enhancing performance levels of athletes during training and competition is a desired goal in sports. Quantifying training success is typically accompanied by performance diagnostics including the assessment of sports-relevant behavioral and physiological parameters. Even though optimal brain processing is a key factor for augmented motor performance and skill learning, neurodiagnostics is typically not implemented in performance diagnostics of athletes. We propose, that neurodiagnostics via non-invasive brain imaging techniques such as functional near-infrared spectroscopy (fNIRS) will offer novel perspectives to quantify training-induced neuroplasticity and its relation to motor behavior. A better understanding of such a brain-behavior relationship during the execution of sport-specific movements might help to guide training processes and to optimize training outcomes. Furthermore, targeted non-invasive brain stimulation such as transcranial direct current stimulation (tDCS) might help to further enhance training outcomes by modulating brain areas that show training-induced neuroplasticity. However, we strongly suggest that ethical aspects in the use of non-invasive brain stimulation during training and/or competition need to be addressed before neuromodulation can be considered as a performance enhancer in sports.
Introduction
“Citius, Altius, Fortius”—Boosting motor performance and skills in athletes on a relatively short time scale and with little effort is a desired goal in professional sports. Athletes typically need to invest a lot of effort and strenuous successive practice over many years. As a rule of thumb, according to Ericsson et al. (1993), a minimum of 10 years or 10,000 h of intense practice is necessary to become an expert in a specific sports discipline. In almost all sports, performance diagnostics is a vital component for athletes to quantify individual performance levels, to evaluate training success and to guide training regimes. The standard procedure for such diagnostics comprises a combination of sports-related behavioral tasks and selected performance-relevant physiological parameters such as heart rate variability, lactate concentration or oxygen consumption.
However, neurodiagnostic tools to evaluate brain processing during sports-related movements are typically not implemented in performance diagnostics of athletes. This seems to be surprising since the central nervous system initiates voluntary movements by generating neural impulses that control the execution of movements. Furthermore, there is neuroscientific evidence that optimal brain processing is a key factor for enhanced motor performance or skill learning. So why is it that performance diagnostics in athletes is not routinely considering neurodiagnostic tools to assess such a brain-behavior relationship? A better understanding of such a relationship might help to optimize performance and/or learning capabilities in athletes. Although neurodiagnostics is an umbrella term for a huge variety of diagnostic tools, the present perspective paper focuses on the use of selected non-invasive brain imaging techniques for performance diagnostics in sport.
One possible explanation for the lack of use of neurodiagnostic tools in performance diagnostics is that over the past decades, the brain has not been considered as a performance inducing or enhancing determinant in sports. Hence, it is not surprising that only a little attention was paid to the role of optimal brain processing and its effects on motor performance or skill learning in athletes.
However, neurodiagnostic tools such as magnetic resonance imaging (MRI) or functional near-infrared spectroscopy (fNIRS) have been extensively used to assess brain-behavior relationships over the lifespan. Based on these investigations, we know that the brain adapts its function and structure according to environmental changes on a very short time scale (Lin et al., 2018; Burke and Barnes, 2006; Erickson et al., 2013; Smith, 2013; Pauwels et al., 2018). Apart from learning-induced functional brain adaptations, recent studies suggest that the effectiveness of movement control and motor skill learning also depends on the individual brain structure and its neuroplastic adaptation (Draganski et al., 2004; Taubert et al., 2010, 2011; Tomassini et al., 2011; Sampaio-Baptista et al., 2013). However, this accumulative evidence is primarily based on simplified models of movement and skill learning paradigms that do not necessarily reflect relevant neuroplastic adaptations in complex sports scenarios. Therefore, future studies should systematically quantify neural processing during the execution of sports-related movements in real-world settings.
In this perspective article, we discuss why and how neurodiagnostic tools should be implemented in diagnostic and training routines to shed more light on the role of optimal brain processing on performance levels in competitive sports. Furthermore, we argue that a better understanding of such a brain-behavior relationship and its training-induced adaptations might help to enhance performance levels and motor skill learning in athletes.
Neurodiagnostics in Sports: Implications for Performance Enhancement in Athletes
Diagnostics of Neuroplasticity Using Non-invasive Brain Imaging Techniques
Non-invasive brain imaging techniques are capable of quantifying expertise-related brain adaptations in various sports disciplines. For example, it has been shown that efficiency in sports is directly related to an optimized brain functioning. A recent functional MRI (fMRI) study by Naito and Hirose (2014) provided novel evidence that the brain of a top-class football player (Neymar) relies on less neuronal resources in motor-related areas as compared to other athletes. Similar findings have been found in other sports disciplines such as table tennis where athletes show less brain activation during the execution of sports-related and sports-unrelated visuospatial tasks as compared to non-athletes (Guo et al., 2017). Moreover, several studies provide compelling evidence that there is a causal relationship between brain activation and behavioral performance (Orban et al., 2010; Peterson and Fling, 2018) or motor skill learning capabilities (Sun et al., 2007; Wadden et al., 2013), respectively. Apart from functional alterations, regular training is also capable of inducing changes on a structural brain level. Meier et al. (2016) suggested that structural adaptations are even sport-specific and are manifested in brain regions which are essential for neural processing of sport-specific skills. They found an increased gray matter (GM) volume in the hand area of M1 of handball players as compared to non-athletes, whereas ballet dancers showed an increased GM volume in the foot area of M1. The aforementioned findings provide novel evidence that motor expertise is capable of modifying brain processing and morphology in a sports context. Furthermore, these findings implicate that the athlete’s brain seems to work more efficiently (Dunst et al., 2014) as compared to lower-level athletes and/or non-athletes. Similar findings have also been found in musicians (Strait et al., 2009; Medina and Barraza, 2019). However, since the aforementioned evidence about functional brain adaptations is solely based on performing simplified and mostly sport-unspecific movements, there is a lack of knowledge about brain processing and adaptations during the execution of sport-specific movements (see Figure 1A).
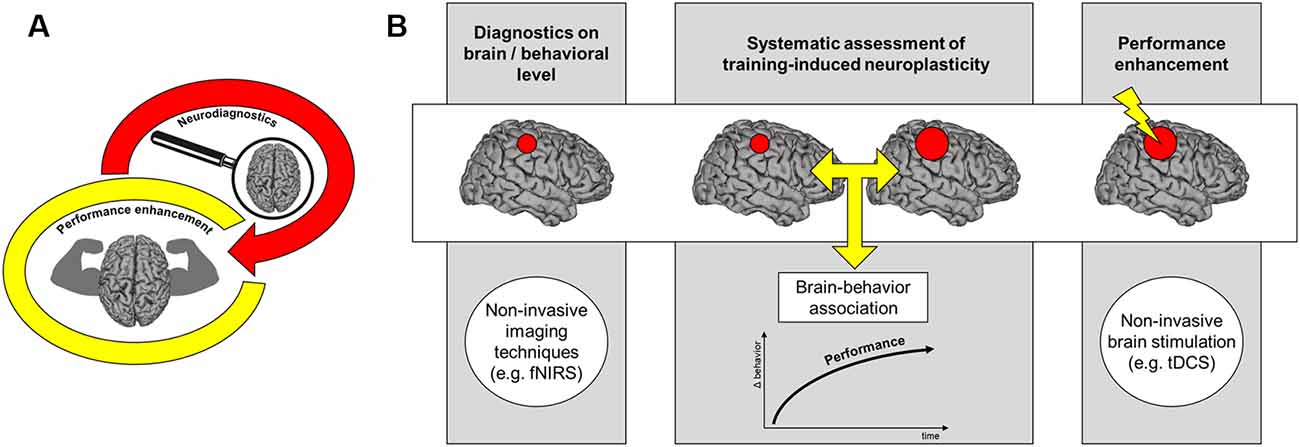
Figure 1. Neurodiagnostics in sports. The figures illustrate how neuroscientific methods might be integrated into behavioral diagnostics in athletes. (A) We propose that neurodiagnostics might help to augment performance levels in athletes. (B) Framework for performance enhancement in athletes using neurodiagnostic tools. Initially, diagnostics of functional and/or structural features of the brain and its relation to performance in sports is an important step towards performance enhancement in athletes. Characterizing training-induced brain changes might help to guide training processes and optimize training outcomes. Finally, targeted non-invasive brain stimulation such as transcranial direct current stimulation (tDCS) might help to enhance performance by modulating brain areas that show training-induced neuroplasticity.
Beyond financial and infrastructural aspects, one crucial limitation in the use of MRI for neurodiagnostics is that sport-specific movements cannot be performed due to the spatial limitations inside the MRI bore and its high susceptibility to motion artifacts (Zaitsev et al., 2015; Havsteen et al., 2017; see Figure 2). Apart from these limitations in the use of MRI, it is reasonable to assume that a better understanding of brain functioning and/or adaptations in brain structure might help to optimize skills and performance in a sports-related context. Alternatively, a better characterization of the athlete’s brain might not only help to predict training success. Brain imaging techniques can additionally be used as a diagnostic tool to identify motor expertise and talent in various fields of sport.
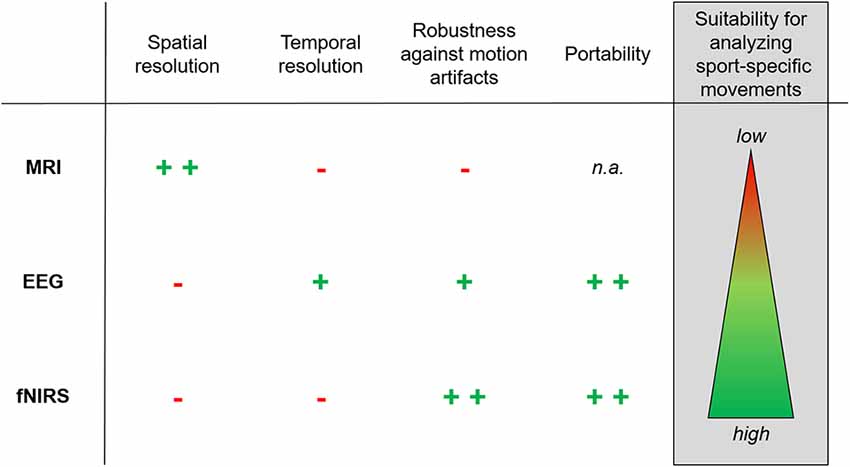
Figure 2. Recommendation for the use of non-invasive brain imaging techniques in neurodiagnostics of sport-specific movements. The figure illustrates advantages and disadvantages of magnetic resonance imaging (MRI), electroencephalography (EEG) and functional near-infrared spectroscopy (fNIRS) with regards to spatial resolution (i.e., where certain brain areas/networks are active), temporal resolution (i.e., when certain brain areas/networks are active), robustness against motion artifacts, portability of the neurodiagnostic tool and suitability for analyzing sport-specific movements (indicating implications for neurodiagnostics). Symbols are defined as follows: + +, very high; +, high; -, low; n.a., not applicable.
One non-invasive brain imaging method that has been widely used in a sports-related context is electroencephalography (EEG). The major advantages of this method are on the one hand its portable application and on the other hand its high temporal resolution (i.e., important for research that aims to characterize when certain brain areas are active during movement execution) (van Gerven et al., 2009; Mehta and Parasuraman, 2013). More importantly, as compared to MRI, EEG provides a direct assessment of brain activity by recording voltage fluctuations at the head surface resulting from ionic current within the neurons of the brain (Light et al., 2010). Hence, EEG has been considered by numerous previous studies as a valuable tool to study neuronal activity during the execution of sports-related movements (Thompson et al., 2008; Park et al., 2015; Cheron et al., 2016). Particularly in sports disciplines characterized by a steady setting and minimal movements such as rifle shooting (Hillman et al., 2000; Doppelmayr et al., 2008), archery (Salazar et al., 1990; Landers et al., 1991) and golf (Babiloni et al., 2008), EEG has been successfully applied to investigate cortical activity during the execution of the respective sports discipline and its relation to the optimal performance or motor expertise. Furthermore, it has been shown that EEG is also feasible for more complex movements such as walking on a treadmill (Severens et al., 2012) and cycling (Brümmer et al., 2011; Ludyga et al., 2016). However, similar to fMRI, major disadvantages of EEG include its high susceptibility to motion artifacts (Symeonidou et al., 2018) and the relatively low spatial resolution (i.e., important for research that aims to characterize where certain brain areas are active during movement execution) as compared to fMRI (van Gerven et al., 2009; Mehta and Parasuraman, 2013; see Figure 2).
Thus, fNIRS is considered as a further promising non-invasive brain imaging method. Due to its portable application, fNIRS offers the opportunity to assess neuronal activity during the execution of sport-specific movements with a moderate to low (depending on inter-optode distance but typically in cm range) spatial resolution (van Gerven et al., 2009; Mehta and Parasuraman, 2013) and low susceptibility to movement artifacts (see Figure 2). fNIRS relies, in analogy to fMRI, on the principle of neurovascular coupling also known as the hemodynamic or blood oxygenation level-dependent (BOLD) response (Strangman et al., 2002; Liao et al., 2013). It involves the quantification of chromophore concentrations resolved from the measurement of relative changes in oxygenated (Hb) and deoxygenated hemoglobin (HHb) which are assumed to be indicators for changes in neural processing (Villringer and Chance, 1997; Obrig and Villringer, 2003). However, due to the time delay of hemodynamic response alterations, the temporal resolution of fNIRS recordings is less good as compared to fMRI and EEG (van Gerven et al., 2009; Mehta and Parasuraman, 2013; see Figure 2). Further limitations, as well as current contributions and possible prospects of fNIRS and EEG, are discussed in a recent position article by Perrey and Besson (2018).
Despite its limitations described above, previous studies have demonstrated that fNIRS is an appropriate and reliable method for measuring neural activity during simple and complex motor tasks (Leff et al., 2011; Pinti et al., 2018). Beyond that, portable fNIRS allows quantifying neural activity in real-world settings without movement constraints (Piper et al., 2014; Pinti et al., 2018). Hence, measuring brain activation during the execution of sports-related movements seems feasible. Many previous studies have successfully applied fNIRS to study functional brain adaptations during complex motor tasks such as juggling (Carius et al., 2016), balancing (Seidel et al., 2017), squatting (Kenville et al., 2017), climbing (Carius et al., 2020), playing table tennis (Balardin et al., 2017), running (Suzuki et al., 2004) and cycling (Seidel et al., 2019). Additionally, the focus is increasingly shifting in the direction of investigating neural correlates of motor expertise comparing athletes and non-athletes using fNIRS (Seidel et al., 2017, 2019). In combination with novel approaches such as multi-channel whole-brain fNIRS, multi-distance fNIRS (Kenville et al., 2017; Seidel et al., 2019) and systemic physiological augmented fNIRS (Herold et al., 2018), these studies provide an important basis for neurodiagnostics of motor expertise and talent (see Figure 1B). Furthermore, a combination of non-invasive brain imaging techniques might help to overcome limitations in spatial and/or temporal resolution. For example, simultaneous EEG and fNIRS recordings (Ludyga et al., 2019) might contribute to shed more light on the question when specific brain networks are active during sport-specific movements and if these networks change during the time course of training.
Systematic Assessment of Training-Induced Neuroplasticity
Apart from quantifying brain function and structure in athletes, the question remains whether neuroplasticity in athletes is training-induced or an epiphenomenon of genetic predisposition. To address this question, several longitudinal training interventions have been conducted over the past decade (Draganski et al., 2004; Taubert et al., 2010, 2011; Tomassini et al., 2011; Gryga et al., 2012; Zatorre et al., 2012; Sehm et al., 2014). Using MRI, it has been shown that not only motor skill learning over several weeks, but also short-term training can lead to specific structural and functional brain adaptations (Floyer-Lea and Matthews, 2005; Kwon et al., 2012). Interestingly, the individual training success seems to be associated with neuroplasticity in motor-related brain regions. For example, participants with the highest learning success in a whole-body balancing task where those that showed the strongest structural brain adaptations (Taubert et al., 2010). Even more interesting, the individual training success seems to be predictable by the individual brain structure before motor skill learning. For example, Gryga et al. (2012) found that participants with the highest density of GM in the cerebellum, an area that plays an important role in processing complex movement patterns, were those with the greatest training outcome in a sequential pinch force task.
Apart from these exciting insights, however, the key limitation of the aforementioned studies is that MRI assessments did not allow online measurements of functional neuroplasticity during motor skill learning and/or training of motor abilities in athletes. Therefore, fNIRS seems to be particularly suitable to quantify functional neuroplasticity systematically during training processes. Here, it seems to be important to assess the temporal dynamics of training-induced neuroplasticity during sport-specific training in real-world settings and its behavioral relevance. This knowledge in turn might be used to control and/or optimize training success in various sports disciplines. Furthermore, neurodiagnostics in sports could also be used in the field of talent diagnostics. Here, certain particularities in brain function and/or structure of young athletes might be used as a predictor for their potential of becoming an elite athlete, their prerequisites to acquire specific skills or to improve motor performance or even their suitability to a specific sports discipline.
Performance Enhancement Using Non-invasive Brain Stimulation
Identifying training-induced neuroplasticity is a prerequisite for targeted neuromodulation to augment motor performance and/or sport-specific skills (see Figure 1B). Here, non-invasive brain stimulation methods such as transcranial direct current stimulation (tDCS) are capable of modulating neural processing in specific brain areas and thereby influence motor behavior (Nitsche and Paulus, 2000; Stagg and Nitsche, 2011). While the exact underlying mechanisms of tDCS-induced effects on a cortical and behavioral level remain elusive, there is accumulative evidence that tDCS induces a polarity dependent modulation of the resting membrane potential (Priori et al., 1998; Nitsche and Paulus, 2000). More specifically, anodal tDCS has been shown to increase resting membrane potential while cathodal tDCS decreases it (Nitsche et al., 2003a; Gandiga et al., 2006). This modulation can subsequently lead to either an increase or decrease of neuronal excitability that can outlast the stimulation period by several minutes or even hours (Nitsche and Paulus, 2000, 2001; Lang et al., 2004, 2005; Nitsche et al., 2005).
For example, a single tDCS session has been shown to increase motor performance or skill learning (Nitsche et al., 2003b; Vollmann et al., 2013; Ammann et al., 2016; Kaminski et al., 2016; Jackson et al., 2019; Kumari et al., 2019). tDCS-induced performance enhancement has not only been described for simple motor tasks such as tapping (Saimpont et al., 2016) and reaction time tasks (Nitsche et al., 2003b; Drummond et al., 2017; Hupfeld et al., 2017), but also for complex whole-body tasks such as balancing (Dutta et al., 2014; Kaminski et al., 2016). Moreover, further studies demonstrated that tDCS is capable of increasing endurance performance during cycling (Okano et al., 2015; Vitor-Costa et al., 2015; Angius et al., 2018a; Park et al., 2019) and running (Park et al., 2019) as well as leg muscle power (Tanaka et al., 2009). Tanaka et al. (2009) revealed that a single session of anodal tDCS transiently enhanced maximal leg pinch force by approx. 15% in normal volunteers. Imagine the importance of such performance enhancements via tDCS in competitive sports where even a subtle change in performance decides about winning or losing.
Neuromodulation to augment performance in sports is no science fiction. Several opinion papers and systematic review and meta-analysis articles discussed the feasibility of tDCS as a performance enhancer in athletes (Bolognini et al., 2009; Banissy and Muggleton, 2013; Davis, 2013; Reardon, 2016; Edwards et al., 2017; Angius et al., 2018b; Machado et al., 2019). Interestingly, tDCS is capable of increasing isometric strength (Hazime et al., 2017; Vargas et al., 2018), countermovement jump performance (Lattari et al., 2020) and endurance performance (Okano et al., 2015) even in trained athletes. These findings indicate that tDCS, if suitably applied, might potentially have positive effects on the athlete’s performance. However, the effectiveness and relevance of tDCS in a sport-specific context has to be investigated more thoroughly in future studies. Furthermore, the exact parameters for tDCS applications in sports remain elusive. For example, it needs to be further clarified e.g., when tDCS should be applied to successfully modulate performance in athletes, i.e., before, during or after training sessions? How long and intense should be stimulated? How often should tDCS be applied concerning training and competition? Days, hours or minutes before the competition? How long-lasting are tDCS effects?
Regardless of these open methodological questions, it is by no means clear if tDCS outside highly controlled laboratory settings is at all effective in boosting performance during competition, especially in highly trained athletes. On the one hand, tDCS is known to induce very variable effects on a behavioral level (Bashir et al., 2019). On the other hand, it is important to keep in mind that athletes already show a kind of ceiling effect in their performance which might potentially lead to no detectable tDCS effects or even a decrement in performance. Furthermore, it is necessary to consider the ethical aspects of the use of tDCS to improve sporting performance (Banissy and Muggleton, 2013). This, in turn, will also be a challenge not only for sports authorities to determine where the tDCS application fits into the regulatory framework at the elite level (Edwards et al., 2017). To date, there are no reliable data on possible negative long-term effects of tDCS, especially after repeated and regular use. Hence, it seems to be reasonable to first understand the exact underlying mechanisms and to quantify optimal stimulation parameters to use non-invasive brain stimulation in athletes more effectively.
Conclusion
In summary, we suggest that neurodiagnostic tools such as MRI, EEG or fNIRS should be implemented in performance diagnostics in sports. Since optimal brain processing is a key factor for efficient motor control and performance, characterizing adaptational brain alterations as a consequence of systematic training might open novel perspectives to augment training success in athletes. Therefore, a desirable goal of future neurodiagnostics is to identify brain networks that contribute to performance improvement in general and, beyond that, brain networks that are particularly responsible for the execution of specific sports disciplines. Furthermore, neurodiagnostics might help to identify youth athletes with the potential of becoming elite athletes. Additionally, neuromodulation might be an alternative way to optimize training outcomes by a selective modulation of performance-relevant brain regions. However, it first has to be shown that tDCS in athletes is at all capable of enhancing motor skill learning and/or motor performance, and if so, that this is performance-relevant and beneficial in specific sports disciplines. Finally, the development and application of neuromodulation in sports must be accompanied by a continuous discussion concerning framework conditions such as ethical aspects, risks, and implementation in the field.
Author Contributions
All authors contributed to the manuscript, reviewed it, approved the final version content and agree to be accountable for all aspects of the work. All persons designated as authors qualify for authorship, and all those who qualified for authorship are listed.
Conflict of Interest
The authors declare that the research was conducted in the absence of any commercial or financial relationships that could be construed as a potential conflict of interest.
References
Ammann, C., Spampinato, D., and Márquez-Ruiz, J. (2016). Modulating motor learning through transcranial direct-current stimulation: an integrative view. Front. Psychol. 7:1981. doi: 10.3389/fpsyg.2016.01981
Angius, L., Mauger, A. R., Hopker, J., Pascual-Leone, A., Santarnecchi, E., and Marcora, S. M. (2018a). Bilateral extracephalic transcranial direct current stimulation improves endurance performance in healthy individuals. Brain Stimul. 11, 108–117. doi: 10.1016/j.brs.2017.09.017
Angius, L., Pascual-Leone, A., and Santarnecchi, E. (2018b). Brain stimulation and physical performance. Prog. Brain Res. 240, 317–339. doi: 10.1016/bs.pbr.2018.07.010
Babiloni, C., Del Percio, C., Iacoboni, M., Infarinato, F., Lizio, R., Marzano, N., et al. (2008). Golf putt outcomes are predicted by sensorimotor cerebral EEG rhythms. J. Physiol. 586, 131–139. doi: 10.1113/jphysiol.2007.141630
Balardin, J. B., Zimeo Morais, G. A., Furucho, R. A., Trambaiolli, L., Vanzella, P., Biazoli, C., et al. (2017). Imaging brain function with functional near-infrared spectroscopy in unconstrained environments. Front. Hum. Neurosci. 11:258. doi: 10.3389/fnhum.2017.00258
Banissy, M. J., and Muggleton, N. G. (2013). Transcranial direct current stimulation in sports training: potential approaches. Front. Hum. Neurosci. 7:129. doi: 10.3389/fnhum.2013.00129
Bashir, S., Ahmad, S., Alatefi, M., Hamza, A., Sharaf, M., Fecteau, S., et al. (2019). Effects of anodal transcranial direct current stimulation on motor evoked potentials variability in humans. Physiol. Rep. 7:e14087. doi: 10.14814/phy2.14087
Bolognini, N., Pascual-Leone, A., and Fregni, F. (2009). Using non-invasive brain stimulation to augment motor training-induced plasticity. J. Neuroeng. Rehabil. 6:8. doi: 10.1186/1743-0003-6-8
Brümmer, V., Schneider, S., Strüder, H. K., and Askew, C. D. (2011). Primary motor cortex activity is elevated with incremental exercise intensity. Neuroscience 181, 150–162. doi: 10.1016/j.neuroscience.2011.02.006
Burke, S. N., and Barnes, C. A. (2006). Neural plasticity in the ageing brain. Nat. Rev. Neurosci. 7, 30–40. doi: 10.1038/nrn1809
Carius, D., Andrä, C., Clauß, M., Ragert, P., Bunk, M., and Mehnert, J. (2016). Hemodynamic response alteration as a function of task complexity and expertise-an fNIRS study in jugglers. Front. Hum. Neurosci. 10:126. doi: 10.3389/fnhum.2016.00126
Carius, D., Hörnig, L., Ragert, P., and Kaminski, E. (2020). Characterizing cortical hemodynamic changes during climbing and its relation to climbing expertise. Neurosci. Lett. 715:134604. doi: 10.1016/j.neulet.2019.134604
Cheron, G., Petit, G., Cheron, J., Leroy, A., Cebolla, A., Cevallos, C., et al. (2016). Brain oscillations in sport: toward EEG biomarkers of performance. Front. Psychol. 7:246. doi: 10.3389/fpsyg.2016.00246
Davis, N. J. (2013). Neurodoping: brain stimulation as a performance-enhancing measure. Sports Med. 43, 649–653. doi: 10.1007/s40279-013-0027-z
Doppelmayr, M., Finkenzeller, T., and Sauseng, P. (2008). Frontal midline theta in the pre-shot phase of rifle shooting: differences between experts and novices. Neuropsychologia 46, 1463–1467. doi: 10.1016/j.neuropsychologia.2007.12.026
Draganski, B., Gaser, C., Busch, V., Schuierer, G., Bogdahn, U., and May, A. (2004). Neuroplasticity: changes in grey matter induced by training. Nature 427, 311–312. doi: 10.1038/427311a
Drummond, N. M., Hayduk-Costa, G., Leguerrier, A., and Carlsen, A. N. (2017). Effector-independent reduction in choice reaction time following bi-hemispheric transcranial direct current stimulation over motor cortex. PLoS One 12:e0172714. doi: 10.1371/journal.pone.0172714
Dunst, B., Benedek, M., Jauk, E., Bergner, S., Koschutnig, K., Sommer, M., et al. (2014). Neural efficiency as a function of task demands. Intelligence 42, 22–30. doi: 10.1016/j.intell.2013.09.005
Dutta, A., Chugh, S., Banerjee, A., and Dutta, A. (2014). Point-of-care-testing of standing posture with Wii balance board and Microsoft Kinect during transcranial direct current stimulation: a feasibility study. NeuroRehabilitation 34, 789–798. doi: 10.3233/nre-141077
Edwards, D. J., Cortes, M., Wortman-Jutt, S., Putrino, D., Bikson, M., Thickbroom, G., et al. (2017). Transcranial direct current stimulation and sports performance. Front. Hum. Neurosci. 11:243. doi: 10.3389/fnhum.2017.00243
Erickson, K. I., Gildengers, A. G., and Butters, M. A. (2013). Physical activity and brain plasticity in late adulthood. Dialogues Clin. Neurosci. 15, 99–108.
Ericsson, K. A., Krampe, R. T., and Tesch-Römer, C. (1993). The role of deliberate practice in the acquisition of expert performance. Psychol. Rev. 100, 363–406. doi: 10.1037/0033-295x.100.3.363
Floyer-Lea, A., and Matthews, P. M. (2005). Distinguishable brain activation networks for short- and long-term motor skill learning. J. Neurophysiol. 94, 512–518. doi: 10.1152/jn.00717.2004
Gandiga, P. C., Hummel, F. C., and Cohen, L. G. (2006). Transcranial DC stimulation (tDCS): a tool for double-blind sham-controlled clinical studies in brain stimulation. Clin. Neurophysiol. 117, 845–850. doi: 10.1016/j.clinph.2005.12.003
Gryga, M., Taubert, M., Dukart, J., Vollmann, H., Conde, V., Sehm, B., et al. (2012). Bidirectional gray matter changes after complex motor skill learning. Front. Syst. Neurosci. 6:37. doi: 10.3389/fnsys.2012.00037
Guo, Z., Li, A., and Yu, L. (2017). “Neural efficiency” of athletes’ brain during visuo-spatial task: an fMRI study on table tennis players. Front. Behav. Neurosci. 11:72. doi: 10.3389/fnbeh.2017.00072
Havsteen, I., Ohlhues, A., Madsen, K. H., Nybing, J. D., Christensen, H., and Christensen, A. (2017). Are movement artifacts in magnetic resonance imaging a real problem?-A narrative review. Front. Neurol. 8:232. doi: 10.3389/fneur.2017.00232
Hazime, F. A., da Cunha, R. A., Soliaman, R. R., Romancini, A. C. B., Pochini, A. D. C., Ejnisman, B., et al. (2017). Anodal transcranial direct current stimulation (TDCS) increases isometric strength of shoulder rotators muscles in handball players. Int. J. Sports Phys. Ther. 12, 402–407.
Herold, F., Wiegel, P., Scholkmann, F., and Müller, N. G. (2018). Applications of functional near-infrared spectroscopy (fnirs) neuroimaging in exercise—cognition science: a systematic, methodology-focused review. J. Clin. Med. 7:466. doi: 10.3390/jcm7120466
Hillman, C. H., Apparies, R. J., Janelle, C. M., and Hatfield, B. D. (2000). An electrocortical comparison of executed and rejected shots in skilled marksmen. Biol. Psychol. 52, 71–83. doi: 10.1016/s0301-0511(99)00021-6
Hupfeld, K. E., Ketcham, C. J., and Schneider, H. D. (2017). Transcranial direct current stimulation (tDCS) to the supplementary motor area (SMA) influences performance on motor tasks. Exp. Brain Res. 235, 851–859. doi: 10.1007/s00221-016-4848-5
Jackson, A. K., de Albuquerque, L. L., Pantovic, M., Fischer, K. M., Guadagnoli, M. A., Riley, Z. A., et al. (2019). Cerebellar transcranial direct current stimulation enhances motor learning in a complex overhand throwing task. Cerebellum 18, 813–816. doi: 10.1007/s12311-019-01040-6
Kaminski, E., Steele, C. J., Hoff, M., Gundlach, C., Rjosk, V., Sehm, B., et al. (2016). Transcranial direct current stimulation (tDCS) over primary motor cortex leg area promotes dynamic balance task performance. Clin. Neurophysiol. 127, 2455–2462. doi: 10.1016/j.clinph.2016.03.018
Kenville, R., Maudrich, T., Carius, D., and Ragert, P. (2017). Hemodynamic response alterations in sensorimotor areas as a function of barbell load levels during squatting: an fNIRS study. Front. Hum. Neurosci. 11:241. doi: 10.3389/fnhum.2017.00241
Kumari, N., Taylor, D., and Signal, N. (2019). The effect of cerebellar transcranial direct current stimulation on motor learning: a systematic review of randomized controlled trials. Front. Hum. Neurosci. 13:328. doi: 10.3389/fnhum.2019.00328
Kwon, Y. H., Nam, K. S., and Park, J. W. (2012). Identification of cortical activation and white matter architecture according to short-term motor learning in the human brain: functional MRI and diffusion tensor tractography study. Neurosci. Lett. 520, 11–15. doi: 10.1016/j.neulet.2012.05.005
Landers, D. M., Petruzzello, S. J., Salazar, W., Crews, D. J., Kubitz, K. A., Gannon, T. L., et al. (1991). The influence of electrocortical biofeedback on performance in pre-elite archers. Med. Sci. Sports Exerc. 23, 123–129. doi: 10.1249/00005768-199101000-00018
Lang, N., Nitsche, M. A., Paulus, W., Rothwell, J. C., and Lemon, R. N. (2004). Effects of transcranial direct current stimulation over the human motor cortex on corticospinal and transcallosal excitability. Exp. Brain Res. 156, 439–443. doi: 10.1007/s00221-003-1800-2
Lang, N., Siebner, H. R., Ward, N. S., Lee, L., Nitsche, M. A., Paulus, W., et al. (2005). How does transcranial DC stimulation of the primary motor cortex alter regional neuronal activity in the human brain? Eur. J. Neurosci. 22, 495–504. doi: 10.1111/j.1460-9568.2005.04233.x
Lattari, E., Campos, C., Lamego, M. K., Legey, S., Neto, G. M., Rocha, N. B., et al. (2020). Can transcranial direct current stimulation improve muscle power in individuals with advanced resistance training experience? J. Strength Cond. Res. 34, 97–103. doi: 10.1519/JSC.0000000000001956
Leff, D. R., Orihuela-Espina, F., Elwell, C. E., Athanasiou, T., Delpy, D. T., Darzi, A. W., et al. (2011). Assessment of the cerebral cortex during motor task behaviours in adults: a systematic review of functional near infrared spectroscopy (fNIRS) studies. NeuroImage 54, 2922–2936. doi: 10.1016/j.neuroimage.2010.10.058
Liao, L.-D., Tsytsarev, V., Delgado-Martínez, I., Li, M.-L., Erzurumlu, R., Vipin, A., et al. (2013). Neurovascular coupling: in vivo optical techniques for functional brain imaging. Biomed. Eng. Online 12:38. doi: 10.1186/1475-925x-12-38
Light, G. A., Williams, L. E., Minow, F., Sprock, J., Rissling, A., Sharp, R., et al. (2010). Electroencephalography (EEG) and event-related potentials (ERPs) with human participants. Curr. Protoc. Neurosci. 52, 6.25.1–6.25.24. doi: 10.1002/0471142301.ns0625s52
Lin, T.-W., Tsai, S.-F., and Kuo, Y.-M. (2018). Physical exercise enhances neuroplasticity and delays Alzheimer’s disease. Brain Plast. 4, 95–110. doi: 10.3233/bpl-180073
Ludyga, S., Gronwald, T., and Hottenrott, K. (2016). The athlete’s brain: cross-sectional evidence for neural efficiency during cycling exercise. Neural Plast. 2016:4583674. doi: 10.1155/2016/4583674
Ludyga, S., Mücke, M., Colledge, F. M. A., Pühse, U., and Gerber, M. (2019). A combined EEG-fNIRS study investigating mechanisms underlying the association between aerobic fitness and inhibitory control in young adults. Neuroscience 419, 23–33. doi: 10.1016/j.neuroscience.2019.08.045
Machado, D. G. D. S., Unal, G., Andrade, S. M., Moreira, A., Altimari, L. R., Brunoni, A. R., et al. (2019). Effect of transcranial direct current stimulation on exercise performance: a systematic review and meta-analysis. Brain Stimul. 12, 593–605. doi: 10.1016/j.brs.2018.12.227
Medina, D., and Barraza, P. (2019). Efficiency of attentional networks in musicians and non-musicians. Heliyon 5:e01315. doi: 10.1016/j.heliyon.2019.e01315
Mehta, R. K., and Parasuraman, R. (2013). Neuroergonomics: a review of applications to physical and cognitive work. Front. Hum. Neurosci. 7:889. doi: 10.3389/fnhum.2013.00889
Meier, J., Topka, M. S., and Hanggi, J. (2016). Differences in cortical representation and structural connectivity of hands and feet between professional handball players and ballet dancers. Neural Plast. 2016:6817397. doi: 10.1155/2016/6817397
Naito, E., and Hirose, S. (2014). Efficient foot motor control by Neymar’s brain. Front. Hum. Neurosci. 8:594. doi: 10.3389/fnhum.2014.00594
Nitsche, M. A., Nitsche, M. S., Klein, C. C., Tergau, F., Rothwell, J. C., and Paulus, W. (2003a). Level of action of cathodal DC polarisation induced inhibition of the human motor cortex. Clin. Neurophysiol. 114, 600–604. doi: 10.1016/s1388-2457(02)00412-1
Nitsche, M. A., Schauenburg, A., Lang, N., Liebetanz, D., Exner, C., Paulus, W., et al. (2003b). Facilitation of implicit motor learning by weak transcranial direct current stimulation of the primary motor cortex in the human. J. Cogn. Neurosci. 15, 619–626. doi: 10.1162/089892903321662994
Nitsche, M. A., and Paulus, W. (2000). Excitability changes induced in the human motor cortex by weak transcranial direct current stimulation. J. Physiol. 527, 633–639. doi: 10.1111/j.1469-7793.2000.t01-1-00633.x
Nitsche, M. A., and Paulus, W. (2001). Sustained excitability elevations induced by transcranial DC motor cortex stimulation in humans. Neurology 57, 1899–1901. doi: 10.1212/wnl.57.10.1899
Nitsche, M. A., Seeber, A., Frommann, K., Klein, C. C., Rochford, C., Nitsche, M. S., et al. (2005). Modulating parameters of excitability during and after transcranial direct current stimulation of the human motor cortex. J. Physiol. 568, 291–303. doi: 10.1113/jphysiol.2005.092429
Obrig, H., and Villringer, A. (2003). Beyond the visible—imaging the human brain with light. J. Cereb. Blood Flow Metab. 23, 1–18. doi: 10.1097/01.wcb.0000043472.45775.29
Okano, A. H., Fontes, E. B., Montenegro, R. A., Farinatti Pde, T. V., Cyrino, E. S., Li, L. M., et al. (2015). Brain stimulation modulates the autonomic nervous system, rating of perceived exertion and performance during maximal exercise. Br. J. Sports Med. 49, 1213–1218. doi: 10.1136/bjsports-2012-091658
Orban, P., Peigneux, P., Lungu, O., Albouy, G., Breton, E., Laberenne, F., et al. (2010). The multifaceted nature of the relationship between performance and brain activity in motor sequence learning. NeuroImage 49, 694–702. doi: 10.1016/j.neuroimage.2009.08.055
Park, J. L., Fairweather, M. M., and Donaldson, D. I. (2015). Making the case for mobile cognition: EEG and sports performance. Neurosci. Biobehav. Rev. 52, 117–130. doi: 10.1016/j.neubiorev.2015.02.014
Park, S.-B., Sung, D. J., Kim, B., Kim, S., and Han, J.-K. (2019). Transcranial direct current stimulation of motor cortex enhances running performance. PLoS One 14:e0211902. doi: 10.1371/journal.pone.0211902
Pauwels, L., Chalavi, S., and Swinnen, S. P. (2018). Aging and brain plasticity. Aging 10, 1789–1790. doi: 10.18632/aging.101514
Perrey, S., and Besson, P. (2018). Studying brain activity in sports performance: contributions and issues. Prog. Brain Res. 240, 247–267. doi: 10.1016/bs.pbr.2018.07.004
Peterson, D. S., and Fling, B. W. (2018). How changes in brain activity and connectivity are associated with motor performance in people with MS. Neuroimage Clin. 17, 153–162. doi: 10.1016/j.nicl.2017.09.019
Pinti, P., Aichelburg, C., Gilbert, S., Hamilton, A., Hirsch, J., Burgess, P., et al. (2018). A review on the use of wearable functional near-infrared spectroscopy in naturalistic environments. Jpn. Psychol. Res. 60, 347–373. doi: 10.1111/jpr.12206
Piper, S. K., Krueger, A., Koch, S. P., Mehnert, J., Habermehl, C., Steinbrink, J., et al. (2014). A wearable multi-channel fNIRS system for brain imaging in freely moving subjects. NeuroImage 85, 64–71. doi: 10.1016/j.neuroimage.2013.06.062
Priori, A., Berardelli, A., Rona, S., Accornero, N., and Manfredi, M. (1998). Polarization of the human motor cortex through the scalp. Neuroreport 9, 2257–2260. doi: 10.1097/00001756-199807130-00020
Reardon, S. (2016). ‘Brain doping’ may improve athletes’ performance. Nature 531, 283–284. doi: 10.1038/nature.2016.19534
Saimpont, A., Mercier, C., Malouin, F., Guillot, A., Collet, C., Doyon, J., et al. (2016). Anodal transcranial direct current stimulation enhances the effects of motor imagery training in a finger tapping task. Eur. J. Neurosci. 43, 113–119. doi: 10.1111/ejn.13122
Salazar, W., Landers, D. M., Petruzzello, S. J., Han, M., Crews, D. J., and Kubitz, K. A. (1990). Hemispheric asymmetry, cardiac response, and performance in elite archers. Res. Q. Exerc. Sport 61, 351–359. doi: 10.1080/02701367.1990.10607499
Sampaio-Baptista, C., Khrapitchev, A. A., Foxley, S., Schlagheck, T., Scholz, J., Jbabdi, S., et al. (2013). Motor skill learning induces changes in white matter microstructure and myelination. J. Neurosci. 33, 19499–19503. doi: 10.1523/JNEUROSCI.3048-13.2013
Sehm, B., Taubert, M., Conde, V., Weise, D., Classen, J., Dukart, J., et al. (2014). Structural brain plasticity in Parkinson’s disease induced by balance training. Neurobiol. Aging 35, 232–239. doi: 10.1016/j.neurobiolaging.2013.06.021
Seidel, O., Carius, D., Kenville, R., and Ragert, P. (2017). Motor learning in a complex balance task and associated neuroplasticity: a comparison between endurance athletes and nonathletes. J. Neurophysiol. 118, 1849–1860. doi: 10.1152/jn.00419.2017
Seidel, O., Carius, D., Roediger, J., Rumpf, S., and Ragert, P. (2019). Changes in neurovascular coupling during cycling exercise measured by multi-distance fNIRS: a comparison between endurance athletes and physically active controls. Exp. Brain Res. 237, 2957–2972. doi: 10.1007/s00221-019-05646-4
Severens, M., Nienhuis, B., Desain, P., and Duysens, J. (2012). Feasibility of measuring event related desynchronization with electroencephalography during walking. Conf. Proc. IEEE Eng. Med. Biol. Soc. 2012, 2764–2767. doi: 10.1109/EMBC.2012.6346537
Stagg, C. J., and Nitsche, M. A. (2011). Physiological basis of transcranial direct current stimulation. Neuroscientist 17, 37–53. doi: 10.1177/1073858410386614
Strait, D. L., Kraus, N., Skoe, E., and Ashley, R. (2009). Musical experience and neural efficiency: effects of training on subcortical processing of vocal expressions of emotion. Eur. J. Neurosci. 29, 661–668. doi: 10.1111/j.1460-9568.2009.06617.x
Strangman, G., Culver, J. P., Thompson, J. H., and Boas, D. A. (2002). A quantitative comparison of simultaneous BOLD fMRI and NIRS recordings during functional brain activation. NeuroImage 17, 719–731. doi: 10.1006/nimg.2002.1227
Sun, F. T., Miller, L. M., Rao, A. A., and D’Esposito, M. (2007). Functional connectivity of cortical networks involved in bimanual motor sequence learning. Cereb. Cortex 17, 1227–1234. doi: 10.1093/cercor/bhl033
Suzuki, M., Miyai, I., Ono, T., Oda, I., Konishi, I., Kochiyama, T., et al. (2004). Prefrontal and premotor cortices are involved in adapting walking and running speed on the treadmill: an optical imaging study. NeuroImage 23, 1020–1026. doi: 10.1016/j.neuroimage.2004.07.002
Symeonidou, E.-R., Nordin, A. D., Hairston, W. D., and Ferris, D. P. (2018). Effects of cable sway, electrode surface area, and electrode mass on electroencephalography signal quality during motion. Sensors 18:E1073. doi: 10.3390/s18041073
Tanaka, S., Hanakawa, T., Honda, M., and Watanabe, K. (2009). Enhancement of pinch force in the lower leg by anodal transcranial direct current stimulation. Exp. Brain Res. 196, 459–465. doi: 10.1007/s00221-009-1863-9
Taubert, M., Draganski, B., Anwander, A., Müller, K., Horstmann, A., Villringer, A., et al. (2010). Dynamic properties of human brain structure: learning-related changes in cortical areas and associated fiber connections. J. Neurosci. 30, 11670–11677. doi: 10.1523/jneurosci.2567-10.2010
Taubert, M., Lohmann, G., Margulies, D. S., Villringer, A., and Ragert, P. (2011). Long-term effects of motor training on resting-state networks and underlying brain structure. NeuroImage 57, 1492–1498. doi: 10.1016/j.neuroimage.2011.05.078
Thompson, T., Steffert, T., Ros, T., Leach, J., and Gruzelier, J. (2008). EEG applications for sport and performance. Methods 45, 279–288. doi: 10.1016/j.ymeth.2008.07.006
Tomassini, V., Jbabdi, S., Kincses, Z. T., Bosnell, R., Douaud, G., Pozzilli, C., et al. (2011). Structural and functional bases for individual differences in motor learning. Hum. Brain Mapp. 32, 494–508. doi: 10.1002/hbm.21037
van Gerven, M., Farquhar, J., Schaefer, R., Vlek, R., Geuze, J., Nijholt, A., et al. (2009). The brain-computer interface cycle. J. Neural Eng. 6:41001. doi: 10.1088/1741-2560/6/4/041001
Vargas, V. Z., Baptista, A. F., Pereira, G. O. C., Pochini, A. C., Ejnisman, B., Santos, M. B., et al. (2018). Modulation of isometric quadriceps strength in soccer players with transcranial direct current stimulation: a crossover study. J. Strength Cond. Res. 32, 1336–1341. doi: 10.1519/jsc.0000000000001985
Villringer, A., and Chance, B. (1997). Non-invasive optical spectroscopy and imaging of human brain function. Trends Neurosci. 20, 435–442. doi: 10.1016/s0166-2236(97)01132-6
Vitor-Costa, M., Okuno, N. M., Bortolotti, H., Bertollo, M., Boggio, P. S., Fregni, F., et al. (2015). Improving cycling performance: transcranial direct current stimulation increases time to exhaustion in cycling. PLoS One 10:e0144916. doi: 10.1371/journal.pone.0144916
Vollmann, H., Conde, V., Sewerin, S., Taubert, M., Sehm, B., Witte, O. W., et al. (2013). Anodal transcranial direct current stimulation (tDCS) over supplementary motor area (SMA) but not pre-SMA promotes short-term visuomotor learning. Brain Stimul. 6, 101–107. doi: 10.1016/j.brs.2012.03.018
Wadden, K., Brown, K., Maletsky, R., and Boyd, L. A. (2013). Correlations between brain activity and components of motor learning in middle-aged adults: an fMRI study. Front. Hum. Neurosci. 7:169. doi: 10.3389/fnhum.2013.00169
Zaitsev, M., Maclaren, J., and Herbst, M. (2015). Motion artifacts in MRI: a complex problem with many partial solutions. J. Magn. Reson. Imaging 42, 887–901. doi: 10.1002/jmri.24850
Keywords: neuroplasticity, fNIRS, non-invasive brain stimulation, performance enhancement, neurodiagnostic, athletes, neuromodulation
Citation: Seidel-Marzi O and Ragert P (2020) Neurodiagnostics in Sports: Investigating the Athlete’s Brain to Augment Performance and Sport-Specific Skills. Front. Hum. Neurosci. 14:133. doi: 10.3389/fnhum.2020.00133
Received: 15 January 2020; Accepted: 23 March 2020;
Published: 09 April 2020.
Edited by:
Stephane Perrey, Université de Montpellier, FranceReviewed by:
Carmelo Chisari, Pisana University Hospital, ItalyZhishan Hu, Beijing Normal University, China
Copyright © 2020 Seidel-Marzi and Ragert. This is an open-access article distributed under the terms of the Creative Commons Attribution License (CC BY). The use, distribution or reproduction in other forums is permitted, provided the original author(s) and the copyright owner(s) are credited and that the original publication in this journal is cited, in accordance with accepted academic practice. No use, distribution or reproduction is permitted which does not comply with these terms.
*Correspondence: Patrick Ragert, cGF0cmljay5yYWdlcnRAdW5pLWxlaXB6aWcuZGU=