- 1Department of Biomedical Engineering, University of Rochester, Rochester, NY, United States
- 2Center for Musculoskeletal Research, University of Rochester Medical Center, Rochester, NY, United States
- 3Wilmot Cancer Institute, University of Rochester Medical Center, Rochester, NY, United States
- 4Department of Pathology and Laboratory Medicine, University of Rochester Medical Center, Rochester, NY, United States
Acute myeloid leukemia (AML) is the most aggressive adult leukemia and results in a dismal 5-year survival rate of less than 30%. While research has primarily focused on identifying intrinsic mutations driving leukemogenesis, the role of the bone marrow microenvironment (BMME) in disease progression remains poorly understood. For this purpose, conventional 2D cultures inadequately replicate the complex BMME interactions crucial for the maintenance of normal hematopoiesis and leukemia pathogenesis. In recent years, 3D cultures or microphysiological systems (MPS), have emerged as promising tools for in vitro modeling of the human BMME. These approaches provide a promise for a more physiologically relevant platform for investigating the mechanistic underpinnings of AML interactions with BMME components, as well as exploring chemoresistance mechanisms and facilitating drug discovery efforts. This review discusses the considerations in biomaterials, biophysical, and biochemical factors to develop the BMME in vitro for AML studies, the state-of-the-art 3D models of the BMME, and the challenges and prospects of adopting MPS for AML research.
1 Introduction
Approximately 300 billion mature blood cells are produced daily originating from a small pool of 11,000 – 50,000 hematopoietic stem cells (HSCs) in the bone marrow (1). Hematopoiesis is the process of producing, differentiating, and mobilizing hematopoietic cells into circulation and secondary lymphoid organs, ensuring a sufficient supply of blood cells for normal physiology and in response to injury or infection. The activity of HSCs is regulated by a complex network of cell-intrinsic factors such as transcriptional, epigenetic, and metabolic regulators as well as local and extrinsic long-range humoral cues (2). The hematopoietic system must efficiently produce and mobilize sufficient blood cells during injury and inflammation without depleting the limited stem cell population (2). In malignancies such as acute myeloid leukemia (AML), this tightly regulated process can be severely disrupted. AML is an aggressive blood cancer in the blood and bone marrow that is associated with failure of the hematopoietic system. It is the most common type of acute leukemia in adults, characterized by the expansion of immature and abnormal blast cells derived from the myeloid lineage (AML blast cells) (3). Initiation of AML is typically thought to involve hematopoietic stem cells and progenitor cells (HSPCs) acquiring mutations and transforming into leukemic stem cells (LSCs), also known as leukemia-initiating cells (LICs). The combinations of cytogenetic and genetic abnormalities seen in AML patients underlie the heterogeneous nature of the disease with various mutations in signaling pathways, DNA methylation, transcription factors, tumor suppressors, etc. (4). AML blast cells accumulate excessively in the bone marrow and peripheral blood, impairing the production of healthy blood cells such as red blood cells, white blood cells, and platelets (4). The loss of normal hematopoiesis is associated with bone marrow failure, anemia, neutropenia, and thrombocytopenia (4). Although AML is relatively uncommon, the steady rise in AML incidences and deaths globally over the last decade is alarming (3, 5, 6).
Incidence is particularly higher in the elderly population with the median age of diagnosis at 68 and overall 5-year survival rates of less than 10% (7). The poor prognosis in this patient population is attributed to poor long-term response to chemotherapy, high relapse rates after treatment, and lack of effective therapy for relapsed patients (3). The standard of care for most AML patients is the ‘3 + 7’ daunorubicin (DNR) and cytrabine (Ara-C) chemotherapy which many patients do not qualify for and nearly 70% of patients in the most affected group succumb to the disease within 1 year of chemotherapy (3, 8–10). This poorly tolerable chemotherapy strategy, which was first discovered in the 1970s, remained largely unchanged (4, 9). Henceforth, identifying novel treatment approaches is crucial for improving care for vulnerable patients who cannot tolerate conventional chemotherapies and stem cell transplantation. Molecular profiling of AML has led to the further stratification of AML subtypes and the development of targeted therapies based on specific genomic alterations. Currently, there are several FDA-approved therapies available including inhibitors of FLT3 (gilteritinib), IDH1 (ivosidenib), and IDH2 (enasidenib). Although therapy options are becoming more available, progress is slower for patients with higher-risk forms of AML such as ones with TP53 mutations, and older adults (11). Overall, although the majority of AML patients undergo intensive induction chemotherapy achieve complete remission, relapse remains high (12). Due to the heterogeneity of AML and variable responses to therapies, finding a one-size-fits-all cure is difficult. Therefore, it is necessary to explore alternative targets that are not exclusive to the blast cells and LICs.
One of the main challenges in improving therapies for AML is the limited understanding of the role of the bone marrow microenvironment (BMME) in the progression and treatment of AML. In various leukemias, research has demonstrated that the interplay between leukemic cells and the BMME influences the progression and relapse of disease, while also modulating drug response and contributing to chemotherapy resistance (13, 14). In vivo and in vitro studies showed that AML blast cells modulate endothelial cell activation, establish crosstalk with fibroblasts, and inhibit osteoblast activity (14–19) (Figure 1). The alteration in the BMME leads to failure of the bone marrow and also provides a leukemia-favorable environment that can support the self-renewal and quiescence of LSCs and the proliferation of AML blast cells (14–19). LSCs that reside in the BMME are often resistant to chemotherapy, and relapse is common due to persistent leukemic cells sequestered in the BMME (associated with minimal residual disease where a certain number of cells are still present after treatment) (4, 15). While chemotherapeutic agents are effective at targeting rapidly cycling AML blasts, they are ineffective against the quiescent LSCs (23). One of the mechanistic explanations for chemoresistance is through cell adhesion mediated by the BMME (24–27). In vivo studies also showed that alterations within the BMME can initiate disease in hematological malignancies such as myelodysplastic syndromes (MDS), chronic myelomonocytic leukemia (CMML), and AML (2, 21, 28, 29). It is no surprise that AML research focus has expanded toward understanding where the BMME fits in the pathological puzzle. What the field knows so far is that the BMME contributes to AML pathophysiology in several ways including: 1) abnormalities in the cellular components can drive neoplasia; 2) remodeling or dysregulation can support the survival and progression of AML cells; and 3) it can act as a sanctuary for AML blast cells and LICs to evade chemotherapy (30).
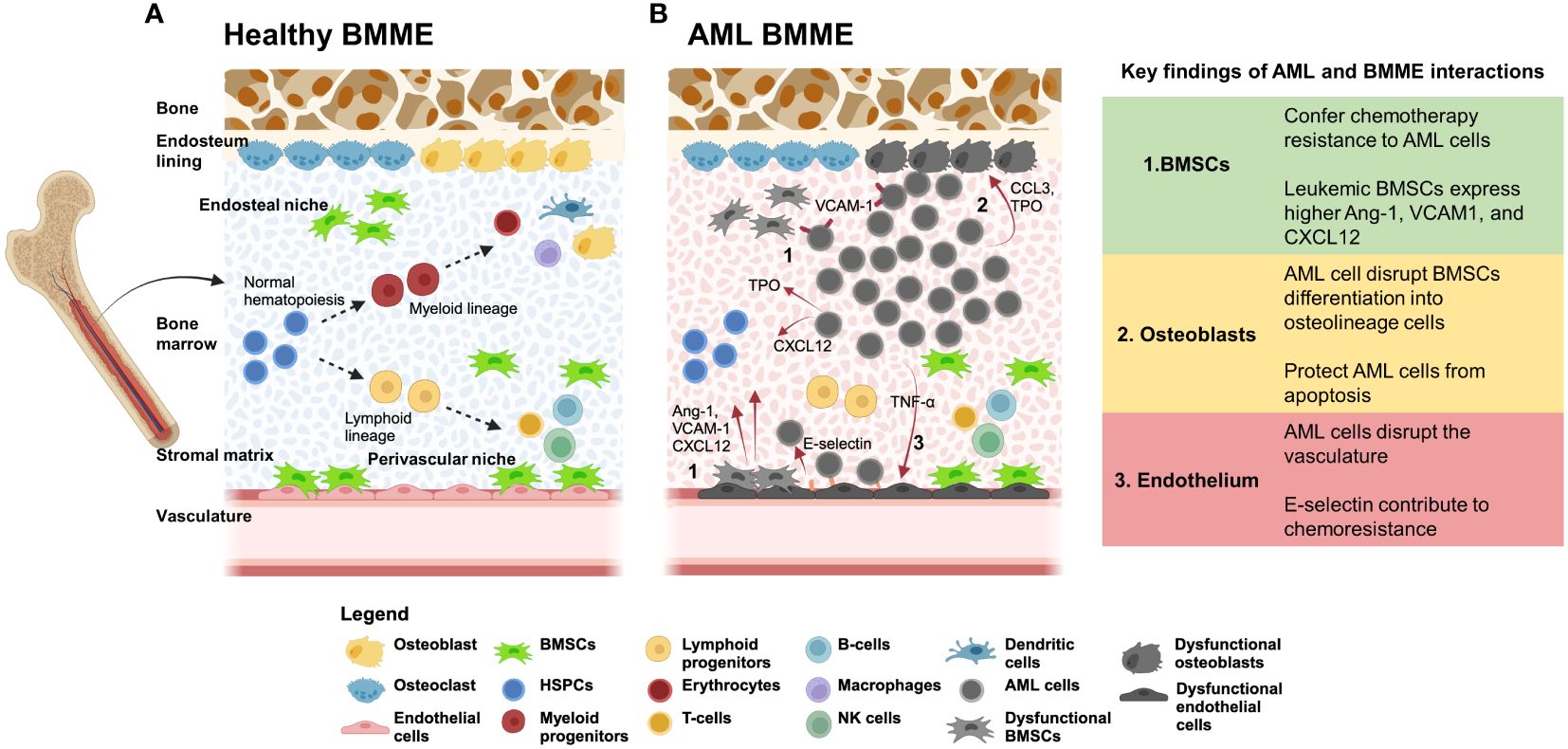
Figure 1 (A) The BMME supports hematopoeiesis through cellular and non-cellular interactions. The BMME is composed of non-hematopoietic cells such as bone marrow stromal/stem cells (BMSCs), endothelial cells, and osteoblasts that regulate proliferation, self-renewal, and lineage determination of HSPCs (15, 20). The complex regulatory network includes interactions with secreted proteins (including CXCL12, IL-6, MCP-1, SCF, and RANKL) and cell-bound proteins (such as VCAM1, E-selectin, and N-cadherin) (15, 21, 22). Disruption of the network can disrupt maintenance of hematopoiesis and can lead to initiation of malignancies. Dotted arrows represent differentiation. (B) AML cell signaling dysregulates BMME components and lead to loss of support for normal hematopoiesis. In vivo and in vitro studies showed that AML cells hijack the BMME to create a permissive environment for AML cell proliferation and maintenance of leukemic stem cells (LSC). (1) During AML, BMSCs are dysregulated and expressed higher Ang-1, VCAM1, and CXCL12. In addition, BMSCs can confer chemotherapy resistance to AML cells via VLA4/VCAM1, E-selectin, and N-cadherin. (2) AML cells can dysregulate osteoblastic cells through CCL3 and TPO (21). Osteopontin secreted from dysregulated osteoblast contribute to protection of AML cells from apoptosis. (3) AML cells release inflammatory cytokines such as TNF-α and CXCL12 that induce increased expression of E-selection on endothelial cells surfaces which AML cells can bind to. These interactions contribute to the cell-adhesion mediated drug resistance (CAM-DR) that sustains LSC in the BMME. The overall functional disruption of the BMME leads to loss of support for HSPCs and dysregulation of normal hematopoiesis. Red arrows indicate signaling from the cell source. Created using BioRender.com.
The expanding understanding of interactions between the BMME and leukemia cells has led to the emergence of innovative therapies that could potentially supplement existing treatments. These newer forms of targeted therapies include targeting supportive cells in the BMME rather than leukemia cells directly such as the ongoing clinical trial of abaloparatide and bevacizumab in myeloid dysplastic syndrome (MDS) (31). Abalopratide is a parathyroid hormone analog that can increase BMSC and osteoblastic support for HSC while bevacizumab blocks VEGF which decreases angiogenesis in the BMME. So far, Phase 1 of the trial showed promising evidence of BMME remodeling as seen with a significant increase in bone marrow stem/stromal cells (BMSCs) in patients (31). Another approach to targeting the BMME involves using uproleselan (GMI-1271), an E-selectin antagonist that disrupts the adhesion of leukemic cells to endothelial cells in the BMME, thereby mitigating adhesion-induced chemotherapy resistance (30, 32). These trials demonstrate a shift in the development of therapies for leukemia, now considering the BMME as a major targetable factor. As research evolves rapidly, the drug discovery tools must keep pace, and 3D culture systems can offer a significant advantage.
2 The need for a 3D culture for the BMME
Most in vitro AML studies rely on conventional 2D cultures of AML cells with HSPCs on a supportive layer of BMSCs (30, 33, 34). Although these models have shown protection of leukemic cells from chemotherapy due to stromal cell interactions, they do not fully reflect the complex interactions within the BMME. Particularly, 2D AML cell cultures lack cues from the extracellular matrix (ECM), growth factors, and signaling molecules stored in the ECM, as well as cellular interactions present in the BMME (24, 35–42). A comprehensive study published recently by Liebers et al. demonstrated the feasibility of ex vivo drug response profiling in guiding the treatment of AML patients undergoing daunorubicin and cytarabine therapy (43). AML cells sampled from either lymph node, peripheral blood, or bone marrow, were tested with chemotherapy agents in 2D culture, and the association between ex vivo response versus in vivo responses was analyzed. The study showed that ex vivo chemotherapy resistance predicted poor in vivo response, with the strongest correlation observed in high-risk AML patients based on the ELN-22 (European LeukemiaNet 2022) (44) stratifications. The authors noted study limitations, including the inability to account for microenvironmental factors that can affect drug response in vivo. Incorporation of microenvironmental factors is imperative to improve prediction accuracy (43).
In the last decade, researchers have taken an integrated approach to developing 3D cultures or microphysiological system (MPS) models (also Organ-on-chips) of various organs and tissues to facilitate mechanistic studies and drug screening for diseases. Applications of MPS include modeling complex tissue systems such as the blood-brain barrier (BBB), lung-on-a-chip, and cardiovascular tissue models (45–48). The strategy is advantageous as it can recapitulate the complex tissue microstructures while reducing reliance on animal models (49–55). By incorporating human cells from patients or cell lines, 3D models provide a valuable tool for studying disease progression, offering insights that are difficult to obtain from human patients (56). In the United States, the recent announcement of the FDA Modernization Act 2.0 emphasizes the need to utilize alternative testing methods such as MPS in replacement of animal models in the preclinical testing phase for new drug development (48, 57). The goal of this decision could improve clinical outcomes and decrease the number of animals used in drug testing, potentially revolutionizing drug development and personalized medicine. The global interest in MPS is rapidly expanding, as evidenced by the initiation of collaborative networks such as the European Organ-On-Chip Society (EUROoCS), International MPS Society (IMPSS), National Institute of Health (NIH) Tissue Chip Consortiums, IQ Microphysiological Systems Affiliate (IQ-MPS). These networks unite funding agencies, pharmaceutical companies, MPS manufacturers, and academic institutions globally to promote the integration of MPS into biomedical research (58). Meetings and conferences hosted by these organizations facilitate open discussions, allowing the sharing of new technologies and promising advances in the MPS field, while also addressing critical challenges such as standardization, affordability, and adoption.
Recognizing the potential of MPS, several groups have developed 3D in vitro models of the BMME using hydrogel technologies and microfluidics platforms. These models can maintain the long-term function of HSPCs by incorporating hydrogels and niche components known to regulate normal hematopoiesis (59–69). The approach is advantageous in providing a platform that can accommodate various requirements of modeling the physiological BMME which is challenging in 2D culture settings. These requirements include incorporating 1) various cellular components in BMME that are known to support HSPCs; 2) extracellular matrix that provides structural, mechanical, and signaling support for HSPCs; and 3) extrinsic molecular cues such as growth factors and cytokines provided by culture media. A recent review by Busch et al. details the cellular components in BMME that are important for HSPC regulation such as BMSCs, endothelial cells, and osteolineage cells, as well as the biophysical and biochemical cues such as ECM components and mechanical properties found in the native BMME (70). These 3D culture systems can improve in vitro or ex vivo studies by providing higher relevance to human BMME and increasing research throughput. Besides performing physiological functions such as sustaining HSPCs, these platforms have also demonstrated the development of leukemic cell drug resistance conferred by the BMME (60, 62). The models can serve the purpose of elucidating the mechanism of AML interactions with the BMME, identifying new therapeutic targets to treat AML, drug characterization, and toxicity profiling, and high-throughput screening (HTS) studies to test the efficacy of new therapies (34).
In this review, we will highlight the frontline 3D models developed within the last decade that have expanded our understanding of the BMME role in AML. These advances encompass the use of synthetic and natural biomaterials, bioreactors, and microfluidics approaches for applications in mechanistic studies and chemotherapeutic investigations. The focus of the view is on the considerations for the development of 3D models of the AML BMME. This review is performed by literature search using PubMED, PubMED Central, SCOPUS, Web of Science, and Google Scholar for keywords including ‘Acute Myeloid Leukemia’, ‘3D culture’, ‘in vitro’, and ‘bone marrow microenvironment’. We reviewed articles published from 2008 – 2024.
3 The BMME and AML cell interactions
HSPCs reside in specialized ‘niches’ in the BMME including the endosteal surface of trabecular bone (endosteal niche), central marrow region (central medullary niche). And regions close to vascular structures including sinusoidal endothelium (perivascular niche) and arterioles (arteriolar niche) (71). The niches are defined by cellular compositions, biochemical and biophysical properties (30, 71, 72). The cellular components include both hematopoietic cells such as megakaryocytes, macrophages, regulatory T-cells, and non-hematopoietic cells such as MSCs, endothelial cells, osteolineage cells, fibroblasts, neuronal cells, and adipocytes. The cells that make up the niches are interconnected via vascular and innervated networks within the bone marrow (2) (Figure 1A). Together, these interactions play a crucial role in regulating the proliferation, self-renewal, and lineage determination of HSPCs (20, 21, 73). Studies that selectively deplete specific BMME populations demonstrate a loss of support for HSCs and thus reducing quiescent HSCs (13). The BMME actively coordinates the balance between the maintenance of quiescence and the activation of HSPCs during hemostasis or in response to an injury or inflammation event (13).
The two commonly discussed niches are the endosteal and perivascular niches. The first is comprised of osteoblasts and osteocytes that are thought to support HSC quiescence and self-renewal (30, 70) (Figure 1A). Meanwhile, the latter is composed of endothelial cells that line up the arterial and sinusoidal vessels and are thought to promote more proliferative and committed HSPCs (30, 70). Both niches are enriched with supportive BMSCs. However, the function of the specific niches is still subject to research and debate as these regions are so close to each other (30). The endosteal matrix niche is characterized by a stiff extracellular matrix, high ionic calcium levels, and hypoxic (70). The hypoxic environment can promote lower ATP levels in HSCs which supports a quiescent state however the topic of oxygen concentrations within the BMME is still in debate as the precise levels of oxygen in the bone marrow are still unclear (71, 74). In vitro, evidence has shown that hypoxic conditions are potentially better for HSCs maintenance (71). In response to oxygen levels in the BMME, the transcription factor hypoxia-inducible factor α (Hif1-α) controls the expression of CXCL12 and CXCR4 that regulates HSC (74). HSCs in the sinusoidal endothelium are thought to be involved in blood cell production as they are more differentiated and ready to enter the bloodstream (2). The perivascular niche is enriched with perivascular BMSCs and specific subtypes such as CXCL12-abundant reticular cells (CAR cells) and macrophages. The cells release factors such as E-selectin, CXCL12, stem cell factor (SCF), leptin, and nestin.
Cells in the BMME are embedded within a rich ECM containing fibrous proteins such as collagen and elastin, and glycoproteins such as laminin, proteoglycans, vitronectin, and fibronectin that sequester growth factors, cytokines, and metalloproteinases that directly affect HSPC fate (70, 74, 75). These proteins are mostly deposited by cells in the stroma such as BMSCs, endothelial cells, reticular cells, adipocytes, and smooth muscle cells (74). The distribution of proteins may vary between the endosteal and perivascular niches with collagens (type I-XI) making up to 90% of the ECM in the BMME (75). ECM in the endosteal niche is composed predominantly of collagen type I and fibronectin while laminin is more present in vascular regions (70). Mechanical properties of the ECM were predominantly measured using murine or bovine samples, so it remains unclear if human bone marrow exhibits similar values. Reported Young’s modulus of the central marrow to the endosteal surface ranges from 0.1 kPA to 50 kPA (70, 76, 77). The stiffness of the perivascular regions may be similar to the basement membrane measured in endothelial basement membrane at 2–3 kPA (78). The BMME components that support HSPCs including the cell types and ECM components are reviewed in various publications (70, 79–82). Deregulation of the BMME can occur during chronic and acute inflammation, as well as aging and malignancies (13). Malignant cells alter the BMME to create a leukemic niche that supports blast and LISc at the expense of hematopoiesis (83). The comprehensive findings on AML and BMME cell interactions can be found in several published review articles (2, 21, 30). Here we highlight the AML and BMME cell interactions that are to be considered in modeling the BMME in vitro.
3.1 Bone marrow stromal cells
BMSCs are a heterogeneous population within the stroma and make up ~20% of the cellular volume in the bone marrow (84). According to single-cell RNAseq of human bone marrow aspirates, BMSCs are predicted to be the main source of cellular signaling in the BMME (84). BMSCs span multiple cell subtypes based on expressions of markers such as Leptin receptors (Lepr), Adiponectin (Adipoq), NG2 (Cspg4), and CxCL12, and can differentiate into osteoblasts, adipocytes, and chondrocytes (85). BMSC regulates the hematopoietic compartment through several key niche factors such as CxCL12, Kitl (SCF), IL-7, angiopoietin-1 (Ang-1) (84, 85) (Figure 1A). Moreover, these cells can confer chemoresistance to AML cells as reported in vitro and in vivo through VLA-4/VCAM-1 signaling which activates the nuclear factor κB (NF-κB) (21) (Figure 1B). This mechanism is termed cell adhesion-mediated drug resistance (CAM-DR) and is also associated N-cadherin and E-selectin (70). Although the 2D culture of BMSCs with AML cells may involve these pathways, it lacks other adhesion-mediated interactions with niche cells and from the 3D ECM itself. These limitations contribute to the loss of ‘stem-ness’ of LSCs in conventional stroma cultures which can impact the translation of in vitro findings to in vivo and human studies. Additionally, BMSCs give rise to osteolineage cells that include osteoprogenitors, preosteoblasts, and mature osteoblasts (85–88). Osteolineage cells regulate HSPC homing, maturation, and function, and the loss of osteoblastic function is associated with the development of myelodysplasia and leukemia (2, 21).
3.2 Osteolineage cells
Osteoblastic cells mineralize the collagen fiber network to synthesize new bone matrix and regulate electrolyte homeostasis between extracellular fluid and bones (30). Osteoblasts that line up the endosteum play a crucial role in bone remodeling and differentiation of osteoclast precursor cells into mature osteoclasts (30). When terminally differentiated, osteoblasts become osteocytes and account for up to 95% of the total cells in the bone (30). Osteoblasts in the endosteal niche promote HSC quiescence through the Notch pathway, Ang-1, and thrombopoietin (TPO) (70). CXCL12, osteopontin and SCF secreted by osteoblasts are also associated with the maintenance of HSPCs (79). HSPCs retention in the bone marrow by osteoblasts is associated with CXCL12 signaling (79). When G-CSF is reduced, the number of osteoblasts and CXCL12 expression decreases which results in the egress of HSPCs into the blood circulation (79). During AML, leukemic cells can disrupt BMSC differentiation into osteolineage cells (Figure 1B). Single-cell RNA sequencing (scRNA-seq) analysis showed that emerging AML cells impaired osteogenesis in BMSCs and reduced regulatory molecules that support hematopoiesis (85). Additionally, osteoblasts can also regulate the protection of leukemic cells against chemotherapy. Particularly, these cells can secrete osteopontin which binds to the αVβ3 receptor on AML cells and protects the cells from apoptosis (36, 57). Our group also showed that AML can functionally inhibit osteoblastic cell activity through CCL3 signaling as seen in the leukemic mice model, blast crisis chronic myelogenous leukemia (bcCML) (14) (Figure 1B).
3.3 Fibroblasts
BMSCs can also give rise to fibroblasts that support the hematopoietic microenvironment. A subset of fibroblasts in murine BM is shown to express Cxcl12 and Ang-1 which indicate a potential niche regulatory function similar to cancer associated-fibroblasts (CAFs) (85). In solid tumor microenvironment and B cell acute lymphoblastic leukemia (B-ALL), CAFs can support chemoresistance which is associated with poor prognosis (21). In addition, malignant CAFs may remodel the BMME and transform it into a more aggressive niche (89). In a retrospective study by Zhai et al. of 63 primary AML patient bone marrow biopsies, immunohistochemistry showed the abundance of reticular fibers in the bone marrow of AML patients which is associated with a higher frequency of relapse and mortality (90). In the bone marrow samples, CAFs widely expressed high levels of fibroblast-specific protein 1 (FSP-1), alpha smooth muscle actin (α-SMA), and fibroblast activation protein (FAP) (90). When the CAFs were cultured with leukemia cell lines, THP-1 or K562, leukemia cells were seen to be less sensitive to chemotherapy. Previously, In vitro experiments by Ryningen et al. demonstrated that coculturing human AML blast cells with fibroblasts resulted in increased blast cell proliferation, and reduced apoptosis mediated by dysregulated cytokine levels such as IL-8 (18). Although the mechanism underlying the role of CAFs in AML is still being explored, pieces of evidence in AML and other cancers showed that the presence of CAFs in the BMME is associated with bone marrow fibrosis, leukemia progression, and sensitivity to chemotherapies (89).
3.4 Endothelial cells
Bone marrow is highly vascularized allowing hematopoietic cells to leave or enter the circulation (2). Imaging studies show that HSPCs were found co-localizing with vascular structures within the bone marrow (71). In addition, perivascular endothelial cells were shown to be important for HSC activation during bone marrow stess (2, 13, 91). The types of endothelial cells present in the BMME include arteriolar endothelial cells (AECs) and sinusoid endothelial cells (SECs) that vary between marker expressions (2). Evidence has shown that E-selectin coupled with chemotherapy doubled the survival time of leukemic mice compared to receiving chemotherapy alone and thus acts as a potential BMME-targeted therapy to improve AML survival (21). Furthermore, evidence shows remodeling of the vasculature during AML indicated by increased permeability, increased nitric oxide (NO), reduced perfusion (2). In mouse and human AML, an increase in angiogenesis is seen however this result is contradicted by intravital imaging studies that showed a selective reduction of blood vessels near the endosteum (2). The reduction is seen due to the accumulation of pro-inflammatory and antiangiogenic cytokines secreted by AML cells in the BMME such as TNF-α and CXCL12 (21) (Figure 1B). When the vessel defects were rescued, the loss of healthy HSC number was prevented and blast cells were more sensitive to chemotherapy (2, 92). Similarly, when endothelial cell-derived NO is inhibited, vascular leakiness is rescued in AML xenograft experiments (93).
3.5 ECM components and matrix remodeling
The ECM anchors HSC to the endosteal and vascular niches and allows for the appropriate hematopoietic signaling to occur in the BMME. Hijacking of this ECM feature leads AML cells to develop resistance to chemotherapy and sequestering of minimal residual disease in the BMME (94). For example, CD44 expression on LSC and blast cells which bind to hyaluronic acid, osteopontin, fibronectin, selectins, laminins, collagens, and matrix metalloproteinases (MMPs), is associated with increased chemotherapy resistance and cancer aggressiveness (95). CD44 is also implicated in the migration, proliferation, differentiation, and survival of HSPCs. In addition, it also regulates the homing of HSPCs in the bone marrow and the homing of LSCs to intra and extra-medullary niches (96). On the other hand, MMP9 is typically downregulated in AML patients, and its high expression is potentially linked to a better prognosis (94). Furthermore, the biomechanical properties of the ECM may also be altered during AML. An in vitro study comparing ECM deposited by BMSCs from different MDS subtypes and healthy controls found that MDS-derived ECM was thicker and more compliant compared to, measured by atomic force microscopy (97). The ECM content differed from the control samples, exhibiting higher levels of glycosaminoglycans (GAGs), sulfated GAGs, and hyaluronic acid (97).
4 Biomaterial, biophysical, and biochemical considerations to recapitulate the BMME in vitro
4.1 Biomaterials considerations
Tissue engineering strategies often involve the use of hydrogels and complex 3D culture devices to provide a matrix structure and dynamic microenvironment, similar to native tissue. Considerations for designing the BMME in vitro must include selecting the appropriate biomaterials, emulating the biophysical and biochemical cues, and incorporating niche cells (Figure 2). The selection of biomaterials is contingent upon achieving biocompatibility to sustain cell growth, differentiation, and function without inducing immune responses or toxicity reactions (85). Particularly in modeling the BMME, where multiple cell types are involved, considerations must be made for cell-matrix interactions that can influence cellular functions, such as proangiogenic or osteogenic responses in BMSCs, immune cell responses, and HSPC differentiation (57, 98). Mechanical properties of biomaterials like stiffness, elasticity, and viscoelasticity must be considered as they can influence cellular behavior and response (75). Additionally, the porosity and permeability of hydrogels must be tuned specifically to the tissue of interest to ensure appropriate nutrient and oxygen diffusion, waste removal, and cell-cell interactions. A recent study by Li et al. showed that larger pore size (80 um) and higher Young’s modulus of 70 kPA were favorable for the proliferation of HSPCs and maintenance of stemness phenotypes (99). The biomaterials chosen should maintain structural integrity and mechanical properties over extended culture periods to support long-term studies. Various synthetic and naturally derived biomaterials have been used in modeling the bone marrow stroma (Table 1). Decisions made on the use of the desired materials revolve around cost-effectiveness, ease of synthesis, and biocompatibility. The biomaterials include synthetically derived polymers such as polyacrylamide (pAAm), polyurethane (PU), poly-L-lactic acid (PLLA), polyglycolic acid, polystyrene (PS), poly(ethylene glycol) (PEG), and hydroxyapatite. Incorporating cell-adhesive peptides, growth factors, or signaling molecules can also promote cell attachment, differentiation, and tissue organization, thus increasing biocompatibility. On the other hand, various naturally occurring materials have been used to mitigate the biocompatibility of synthetic matrices by using alginate, decellularized Wharton’s jelly matrix, Matrigel, and fibrinogen.
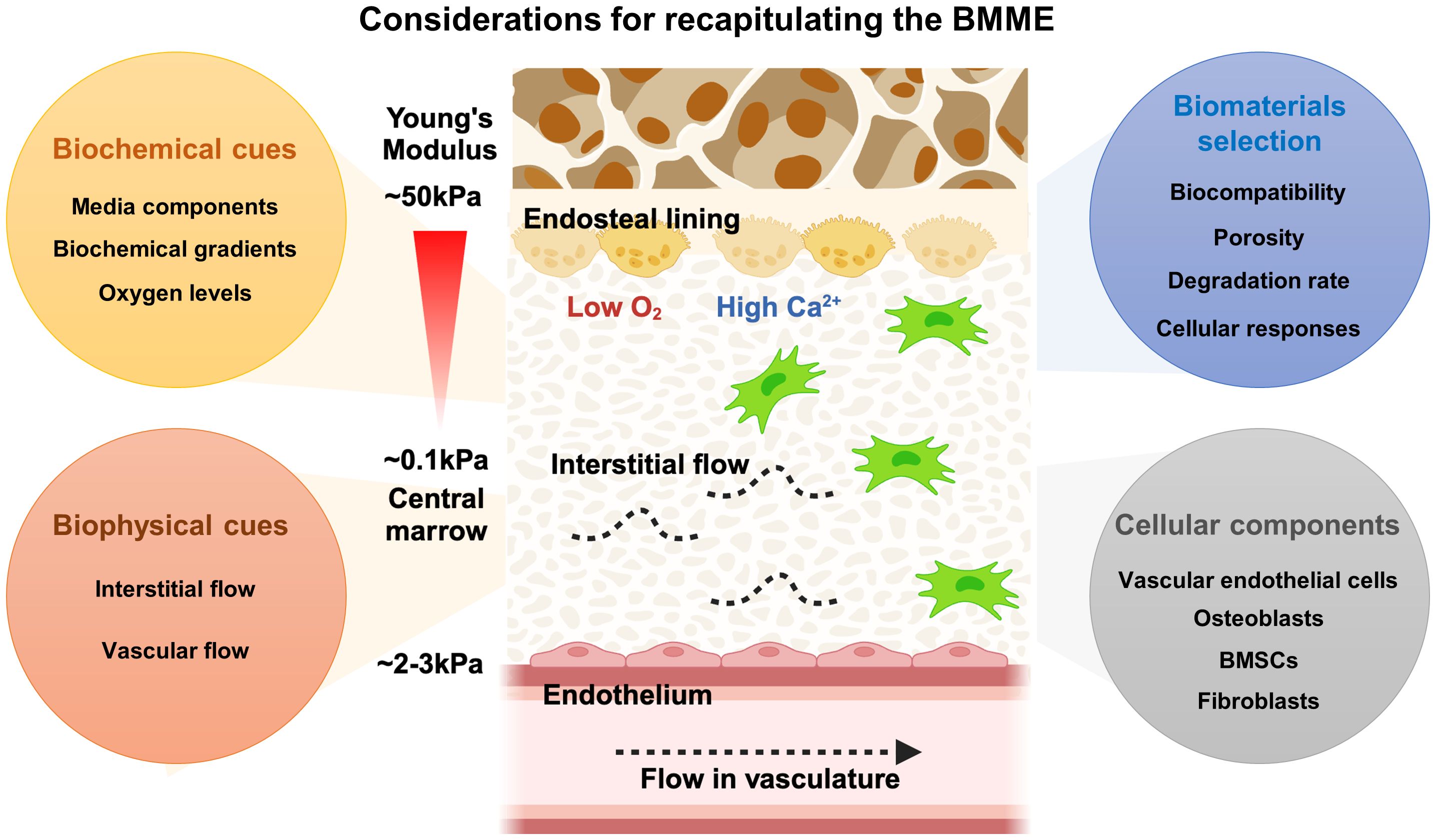
Figure 2 Considerations of designing the BMME in vitro. The BMME is a highly complex network of cells and matrix components that regulate HSPCs self-renewal, proliferation, differentiation, and mobilization. To accurately recapitulate the BMME, it is essential to consider factors such as biomaterials, biophysical properties, biochemical signals, and cellular composition. These elements must be carefully integrated to create a model that closely resembles the native human BMME.
Earlier efforts in culturing AML cells include using synthetically derived-biocompatible hydrogels (24, 37, 100). The benefits of using these hydrogels include the vast tunability of the mechanical properties that can be tailored based on the tissue or disease of interest. For example, bioactive peptides can be incorporated into the hydrogel backbone such as peptides containing the Arg-Gly-Asp (RGD) integrin-binding motif. Dainiak et al., 2008 fabricated a 3D hydrogel composed of pAAm crosslinked with either agmatine-based RGD mimetic or bovine-derived collagen type I (Col1) via cryogelation (100). The resulting hydrogels are macroporous, having a pore diameter of 10–100 μm and very elastic. pAAm-based hydrogel is used as it is drainage-protected, highly tunable, and provides mechanical strength that can resemble tissue-like elasticity. KG-1a cell line (ATCC CCL-246.1), a promyeloblast macrophage cell line isolated from an AML patient, was cultured either in hydrogel containing RGD, hydrogel containing Col 1, or in a 2D culture plate. The authors found that KG-1a cells form aggregates in Col1 and RGD-incorporated pAAM hydrogel while the cells stay as single cells in control unmodified hydrogel. Chemoresistance was tested by treating the culture systems with Ara-C. The authors found that the KG-1a cell aggregates in 3D conditions were more resistant to Ara-C treatment compared to single cells in the unmodified hydrogel. This higher resistance, however, may be due to the protective effect of aggregates. Critically, the high porosity of the hydrogels caused the release of non-adherent KG-1a cells from the unmodified hydrogel into the culture with every media change or addition of treatments. Therefore, the comparison of Ara-C effects on KG-1a cells between the control unmodified hydrogels with RGD and Col1 is not conclusive.
The loss of cells due to the large pores of the hydrogel can be alleviated by fabricating hydrogels with smaller pore sizes. Nair et al. (2015) found that a 60:40 combination of PU and PLLA created a hydrogel with dual nano and microporous property via thermally induced phased separation (TIPS) technique (24). This formulation showed the highest fibronectin adsorption compared to other combinations and thus was used to culture KG-1a cells. VLA-4 integrin expressed on AML cells can bind to stromal fibronectin and the downstream effects confer CAM-DR to chemotherapy (103, 104). The authors found that KG-1a cells cultured in fibronectin-coated PU/PLLA have increased cell adhesion and that the original CD34+/CD33- phenotype was maintained after 7-day culture as compared to a reduction of the phenotype in fibronectin-coated tissue culture plate control (24). CAM-DR was observed by the lack of KG-1a cell death in the hydrogel after treatment of Ara-C as compared to profound cell loss in 2D conditions. When treated with DNR, KG-1a cells in the hydrogel remained drug-resistant for a longer time as compared to controls (24). These findings lead the authors to investigate the mechanism that contributes to chemoresistance in KG-1a cells cultured in the 3D culture. It was shown that the upregulation of the anti-apoptotic Bcl2 and p27kip1, which can result in cell growth arrest, was associated with chemoresistance. Subsequently, the inhibition of Bcl2 via the inhibitor ABT737 increased cell death of KG-1a cells in the presence of Ara-C and DNR (24).
Natural hydrogels can offer superior biocompatibility with cells, which explains why several research groups prefer to explore the utilization of these hydrogels for culturing HSPC and AML cells in vitro. In a paper published by Shin et al. (2016), the researchers used alginate hydrogel ionically crosslinked with RGD peptide to culture leukemic cells with distinct genetic mutations; MOLM-14 (MLL-AF9), U-937 (without MLL-AF9), and K-562 (CML cells with BCR-ABL) in a 96-well plate format. The authors investigated the proliferation of leukemic cell lines in 3D hydrogels with a range of physiological tissue stiffness relevant to the hematopoietic system stiffness (Young’s modulus, E = 0.075 kPa ~ 3 kPa) (37). These cells express α5β1 which binds to the RGD sequence in fibronectin (37). The authors found that leukemia cells formed amorphous aggregates in viscous matrix while forming single large spheroids in solid matrices. Shin et al. (2016) revealed that the MOLM-14 shows increased proliferation responses across matrix stiffness whereas K562 cells show a stiffness-dependent decrease in cell number (37). The authors found that RGD and a softer matrix confer resistance of AML cell lines to multiple drugs. The study advanced the field by showing that cell-extrinsic factors can have different impacts depending on the AML cell used and that genetic mutations and physical environments can have varying relationships (37).
Li et al. (2018) explored the use of human-derived decellularized Wharton’s Jelly matrix processed from umbilical cords (23). The rationale for using this matrix is that it contains similar components to bone marrow ECM, such as collagen, fibronectin, hyaluronic acid, and sulfated proteoglycans. The study used three different cell lines: HL60; Kasumi-1; and MV4-11 and tested the responses of the AML cell lines to Ara-C treatment. The results showed that AML cells in the Wharton’s Jelly matrix had reduced proliferation compared to cells in 2D suspension culture, but there was no difference in apoptosis. Additionally, the cells in the 3D matrix had characteristics of LSCs and a significant population of cells that were resistant to chemotherapy. The study also found that the AML cells in the Wharton’s Jelly matrix had an increased ability to self-renew and were more resistant to the chemotherapy drug Ara-C (23). The study further corroborated the association between elevated N-cadherin expression in 3D cells and heightened chemotherapeutic resistance of AML cells.
In the study by Xu et al. (2019), the authors explored the response signatures of Ara-C in bone marrow mononuclear cells (BMMCs) from both AML patients and healthy patients encapsulated in Matrigel, a commercial hydrogel rich in laminin and collagen IV (38). The researchers first optimized the growth conditions to recapitulate the BMME using BMMCs from healthy patients. They found that BMMSCs were capable of multilineage differentiation, including both hematopoietic lineages and stromal cells. Next, the researchers characterized the ex vivo growth and sensitivity to Ara-C of cryopreserved BMMCs from 49 AML patients. RNA-sequencing analysis was performed to determine differential gene expression patterns between 3D and 2D culture plates. The data showed unique gene expression signatures and novel genetic mutations associated with sensitivity to Ara-C treatment. This publication demonstrates the ability to integrate genomic approaches with 3D cultures of human cells and shows the feasibility of using these platforms to study human cell interactions with chemotherapies.
An alternative natural hydrogel that is commonly used for cell culture is fibrinogen hydrogels. Fibrinogen is derived from the polymerization of fibrin in the presence of thrombin which takes place during blood clotting response. Alhallak et al. (2021) proposed a fibrinogen hydrogel that is incorporated with plasma aspirate from a human donor. The combination of plasma culture with fibrinogen gel is rationalized to promote the proliferation and drug resistance of AML cells (39). Plasma contains a plethora of cytokines, chemokines, and growth factors that mimic the in vivo environment, whereas synthetic hydrogels lack these essential components. In this research, the fibrinogen and plasma culture hydrogels were used to culture both leukemic cell lines (KO52 and NOMO-1) and primary AML cells. The results showed that the AML cell line had a much higher expansion rate at days 3 and 7 in hydrogels compared to 2D cultures. Additionally, primary AML cells cultured in 2D showed no expansion on day 3, but when cultured in 3D hydrogel, the cells expanded 4.5-fold. The researchers also investigated the drug resistance of AML cells to Alisertib (Ali), Ara-C, and Venetoclax. In 2D culture, the drugs induced significant AML cell killing, exceeding 50%. However, in 3D culture, the drugs induced mild to no killing of cells. These findings suggest the chemoresistance of AML cells due to the protective fibrinogen and plasma culture hydrogel similar to native BMME.
4.2 Introduction of niche cells into hydrogels
4.2.1 BMSC stromal cells
The incorporation of niche cells into hydrogels is an important next step to improve the modeling the BMME. Aljitawi et al. (2014) took the approach of incorporating stromal support into a porous hydrogel by co-culturing the leukemic cells with human BMSCs. In the study, a 3D porous hydrogel was created using a combination of 90:10 polyglycolic acid and PLLA. The hydrogel was highly porous, allowing for the free diffusion of molecules up to 1000 Dalton (35). The study aimed to investigate the cytotoxic and apoptotic effects of chemotherapy agents on leukemic cell lines HL-60, Kasumi-1, and MV4-11 in co-culture with BMSCs. The results showed that the proliferative response to chemotherapy in 3D conditions was cell-line specific, with Kasumi-1 cells having a higher proliferative response across all DNR treatment doses compared to HL-60 cells. Additionally, HL-60 cells were found to be more resistant to drug-induced apoptosis in 3D conditions compared to 2D stromal culture and suspension culture. The study also found that protection from chemotherapy in 3D conditions may be due to soluble factors released by the cells and that higher doses of chemotherapy can abolish this protective effect. The study also investigated whether the protection was due to the CAM-DR mediated by N-cadherin and found that HL-60 in 3D had higher N-cadherin expression compared to 2D, whereas Kasumi-1 cells did not express significant differences in N-cadherin in 2D and 3D. Similar to Shin et al. (2016), these findings suggest that the mechanism of chemoresistance can vary between cell lines and that consideration of which cell type to use for studies is highly crucial (35, 37).
Shen et al. (2016) co-cultured osteoblasts differentiated from BMSCs of leukemia patients with MV4–11 cell line on hydrogel sheets made out of PS. This model attempted to emulate the interactions between the leukemic BMME and leukemia cells. The model is composed of four-layer PS porous sheets arranged within a Transwell insert, featuring pores ranging in size from 150 μm to 200 μm in diameter. The model is in contrast with the previously discussed hydrogel systems that contained dense and randomized 3D microstructures that can absorb and retain liquid. The culture received osteogenic differentiation media which included dexamethasone, β-glycerophosphate, and retinoic acid to induce differentiation of leukemic BMSCs into mature osteoblasts. The authors investigated the effect of blocking the interaction of osteopontin and the receptors, αvβ3 and CD44, via a cyclic peptide containing the RGD sequence that binds to osteopontin. The authors found that BMSCs cultured in 3D secrete more alkaline phosphatase (ALP) and osteopontin compared to 2D culture of BMSCs in culture plate (36). In the presence of the osteoblasts in 2D and 3D, MV4–11 cells are more resistant to treatment with Ara-C indicated by reduced apoptosis. When the cyclic RGD peptide was introduced into the culture, the adhesion and migration between MV4–11 cells and the matrix were disrupted which caused the leukemia cell to leave the 3D environment into the media and become more sensitive to Ara-C treatment (36). Another publication from the same group also showed that treatment with AMD3100 and G-CSF with Ara-C reduced the apoptosis of leukemia cells in 3D compared to 2D culture with osteoblast (105). These findings validated the protective role of the microenvironment, in particular osteoblastic cells, to leukemia cells in vitro.
Borella et al. (2021) created a hydrogel composed of 70% hydroxyapatite and 30% collagen to culture AML cells collected from pediatric patients with leukemic BMSCs isolated from the same patient or healthy donors. Leukemic BMSCs were isolated at the time of diagnosis and cultured for 7 days in the 3D hydrogel before adding AML cells. The models allow for the culture of primary AML cells for at least 21 days. The authors explored three different cell-stroma conditions which are leukemic BMSCs only, leukemic BMSCs with BMSCs-derived osteoblasts, and leukemic BMSCs with HUVECs and their effects on AML cell proliferation. It was found that AML cells formed a physical connection with BMSCs indicated by the presence of membrane nanotubes and gap junctions positive for connexin-43 (CX43) staining. The authors found that this interaction modulates transcriptome reprogramming which includes aberrant cell proliferation and differentiation and compromises their immunomodulatory capacity (40). Using this 3D model, the researchers conducted a high-throughput screening using 480 candidates and found Lercanidipine as a potential drug to target leukemic BMSCs. This molecule was able to reduce the proliferation rate of leukemic BMSCs without toxicity to healthy CD34+ HSPCs cells (40). The authors investigated the potential of dual targeting the AML cells and BMSCs using Ara-C and Lercanidipine to reduce leukemia proliferation and found a synergistic effect in reducing the proliferation of leukemic cells in 3D. This dual approach also showed success in AML mice when treated with both Ara-C and Lercanidipine (40).
4.2.2 Endothelial cells
Vascular cell incorporation has also been studied as an additional component to recapitulate the AML BMME in vitro. Bray et al. (2017) aimed to create a vascular niche for leukemia cells using a star-peg heparin gel with adhesion ligands and pro-angiogenic factors (102). The gel was made of MMP-sensitive PEG and heparin that was functionalized with adhesion ligands and pro-angiogenic factors. The resultant gel had a storage modulus of 200–300 Pa, which is optimal for endothelial network formation. As the hydrogel is MMP-sensitive, cells can cleave and remodel their environment during the culturing period. The vascular niche was created by culturing human mesenchymal stem cells (hMSCs) and human umbilical vein endothelial cells (HUVECs) together with leukemia cell lines KG1a, MOLM13, MV4–11, and OCI-AML3, or patient samples. The researchers explored the effects of chemotherapy on the cells grown in this matrix and compared it to a 2D suspension culture. Leukemia cells grown in the 3D matrix were more resistant to the Ara-C and DNR compared to 2D culture but with variable effects depending on the cell types and drugs. Particularly, MOLM13 cells were more resistant to DNR doses in 3D than in 2D, and MV4–11 cells and OCI-AML3 cells had increased resistance to both DNR and Ara-C in 3D compared to 2D (102). The authors investigated the effect of inhibiting the CXCR4/CXCR12 axis that plays a protective role against chemotherapy via the stromal microenvironment (106–108). AMD3100, CXCR4 inhibitor, showed mobilization of AML cells from the vascular network but it does not increase the efficiency of DNR in 3D cultures. In both cell lines and patient cells, this inhibition showed variable effects on cell-adhesion. The authors also observed that AML cell lines formed a heterogeneous mixture of spheroids and loose cell clumps in contact with the vascular network in the hydrogel (102). Meanwhile, primary AML cells proliferated slower compared to AML cell lines and formed clumps rather than spheroids in the hydrogel. Particularly, the cells exhibited a preference for single cell adherence and growth to the HUVEC-hMSC networks which resemble AML cells phenotype in vivo (102). Flow cytometry showed similar marker expression of cells in 2D and 3D, with a varying change that depended on the type of cells used. The combination of DNR and Ara-C completely obliterated cells in 3D cultures of primary AML cells and cell lines at day 14 after treatment (102).
A recent research article by Alhattab et al. (2023) uses robotic 3D printing to establish a high throughput BMME drug screening platform (Figure 2) (109). The authors explored a class of ultrashort tetramer peptides (IIZK, Ac-Ile-Ile-Cha-Lys-NH2) capable of self-assembling into stable hydrogels, which formed a highly porous network of nanofibers with mechanical properties akin to the stromal matrix. Leveraging automated robotic bioprinting, the aim was to fabricate a 3D BMME-like structure comprising primary leukemia cells or cell lines, BMSCs, and endothelial cells. The investigation encompassed assessments of cell biocompatibility, functionalities, and drug responses, alongside RNAseq and gene expression analyses. Notably, BMSCs grown in 3D exhibited elevated expression of osteogenic and adipogenic differentiation markers, including osteopontin (spp1), BMP-2, and FOXO-1 (109). The hydrogel supported the growth of primary AML cells, endothelial cells, and BMSCs, with high viability observed in leukemia cell lines KG1a, HL-60, and MV 4–11. AML cells cultured in 3D displayed quiescence and chemoresistance, along with increased colony formation compared to 2D cultures. Furthermore, BMSCs in 3D demonstrated enhanced protection of leukemia cells against chemotherapy agents. RNAseq analysis revealed potential pathways contributing to AML drug resistance and disease relapse through the modulated expression of HGF, FGF1, CCL2, and IL6.
4.3 Biochemical cues in 3D BMME
Velliou et al. (2015) explored the effect of environmental factors on the proliferation and metabolic evolution of AML cells in 3D and 2D. In this model, a similar hydrogel was used as described before by Nair et al. (24). In this model, K-562 cell line (ATCC CCL-243), lymphoblast cells isolated from the bone marrow of a 53-year-old patient with chronic myelogenous leukemia (CML), were cultured in a PU-based 3D hydrogel coated with Col1 (101). The authors investigated the effects of different levels of oxidative stress and glucose on the phenotype of K-562 in 3D culture. The PU-based hydrogel showed great maintenance of K-562 cells in vitro as compared to other hydrogels tested (110). In this study, varying glucose concentrations were tested which were: (1) optimal tissue culture media concentration (4.3 g/L); (2) maximum physiological glucose concentration (1.3 g/L); and (3) minimum physiological glucose concentration (0.6 g/L). The glucose concentrations were tested against hypoxic (5% O2) and normoxic (20% O2) conditions. Understanding the impact of these factors on 3D culture systems is essential for future drug testing, as the microenvironment may synergistically interact with environmental factors, influencing drug response (101). All in all, the authors found varying effects of oxidative stress and glucose levels between 2D and 3D cultures. Specifically, under hypoxia-induced oxidative stress, glucose levels had a more profound impact on the proliferation of K-562 cells in 3D compared to 2D. In 3D under hypoxia, the highest level of glucose induces higher cell proliferation meanwhile the lowest level of glucose suppresses the expansion of K-562 cells (101). In addition, cells cultured in 2D showed higher metabolic activity indicated by increased metabolite levels in media such as lactate and glutamate. The findings suggest that the mode of culture can have a significant impact on the responses towards oxidative stress and glucose levels.
4.4 Incorporation of biophysical cues
4.4.1 Mimicking interstitial flow and osteoblastic niche
While hydrogels can be valuable in providing the initial 3D environment for cells, they often lack precise control of biophysical stimulation (111). Therefore, microfluidic and perfusable hydrogels platforms have been popular in tissue-on-chip approaches as it provide an opportunity to incorporate flow to mimic the endothelial blood flow or interstitial fluid flow (Figure 3). These platforms can provide spatial refinements that can recreate tissue inter-compartmentalization. The combination of both hydrogels and microfluidics approaches represents a highly advantageous strategy, offering a dynamic 3D environment to recapitulate the complex BMME interactions. More recently, several groups including ours have published multi-niche BMME microfluidics systems containing the endosteal and/or vascular niche as a physiological microenvironment to culture HSPCs in vitro (62, 113–115).
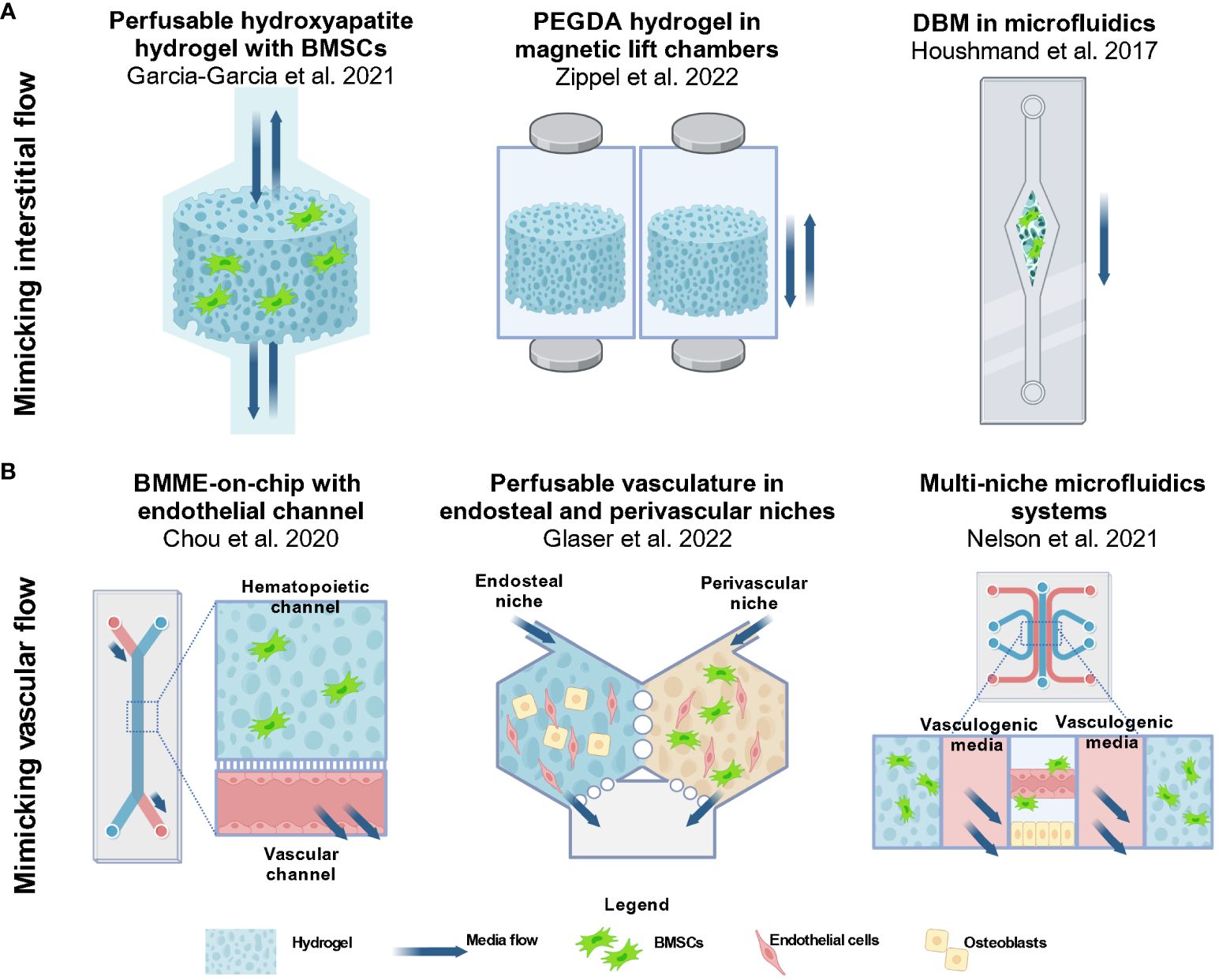
Figure 3 Approaches in 3D modeling of the BMME for AML studies involve utilizing perfusable hydrogels and microfluidics devices. (A) Several groups aimed to create dynamic models with biophysical factors that are present in the BMME. To incorporate interstitial flow in the matrix, groups have incorporated perfusability in the design of hydrogel scaffolds (42, 112). The approach includes a bioreactor system with media flowing through a porous hydroxyapatite hydrogel by Garcia-Garcia et al., 2021 and magneticized PEGDA hydrogel enabling non-contact perfusion in a culture chamber as demonstrated by Zippel et al., 2022. An alternative way to introduce flow is via microfluidics as demonstrated by Houshmand et al., 2017 where a demineralized bone matrix scaffold is placed in a single channel microfluidics systems (41). Images were reproduced using BioRender.com. (B) The functional vasculature is an important component of the BMME that supports the niches and cells present. Among the approaches include a BMME-on-chip microfluidic device by Chou et al., 2020 that consist of a a hematopoietic channel with a fibrinogen matrix and an endothelial channel with media flow (60). Glaser et al. and Nelson et al. designed microfluidics devices with multiple niches to recapitulate the perivascular niche and endosteal niche (62, 113). Currently these models are not being used for AML studies, however they provide innovative approaches to recapitulating the dynamic BMME in vitro. BMSC, bone marrow stromal/stem cells; MSC, mesenchymal stromal/stem cells; HUVEC, human umbilical vein endothelial cells; OB, osteoblastic cells; EC, endothelial cells. Images were reproduced from (60, 62, 113) using BioRender.com.
Several groups have incorporated fluid flow by incorporating perfusion-based approaches designed based on the principle of interstitial flow through the trabecular-like bone matrix and bone marrow ECM (Figure 3A). Garcia-Garcia et al. (2021) used a bioreactor-based 3D system with a perfusable porous hydrogel containing an osteoblastic niche by using a hydroxyapatite hydrogel seeded with BMSCs and HSPCs (42). Hydroxyapatite was chosen because it shares similar mineral components to the trabecular bone. Similar to Shin et al. (2016), this model allowed the researchers to investigate the fate of HSPCs and AML cells that remained in the niche, and those that exited the hydrogel (37). Previously it was shown that HSPCs that remained in the hydrogel were quiescent while more committed populations were found in the supernatant (64). This differential effect was also seen in the AML model which incorporates CD34+ BM cells from patients with AML and myeloproliferative neoplasms (MPN) at least 3 weeks in culture. The authors found that leukemic committed progenitor cells were enriched in the hydrogel while mature leukemic cells (CD34-CD38-) and mature leukemic blasts (CD33+) were found in the supernatant. Gene expression analysis showed that cells in the niche region highly expressed CXCL12, VCAM1, and IL6 which regulate leukemic cells. Meanwhile, cells in the supernatant expressed macrophage colony-stimulating factor (M-CSF) that attracts and regulates mature myeloid cell differentiation. The results showed that the distribution and differentiation of AML cells were dependent on niche-specific signals similar to the native BMME. The authors also explored the cell interaction with an angiogenic niche by culturing human adipose-derived stromal vascular fraction (SVF) cells within a collagen-based hydrogel. These cells contain both stromal progenitors and endothelial lineage cells. In the hydrogel, the cells form 3D networks with positive CD31+ and NG2+ staining indicating the presence of endothelial cells and perivascular cells. To investigate the behavior of malignant cells in the distinct niches, UCSD-AML1 cell line was cultured in either the hydrogel with the osteoblastic niche or the SVF. A significant observation found was that UCSD-AML1 cells cultured in the SVF-containing hydrogel had lower expression of ANG1, higher levels of ANG2 and proinflammatory cytokines within 1 week of culture as compared to cells cultured in the osteoblastic niche hydrogel. The data suggest that the different niches can distinctly regulate leukemic cell expansion and differentiation (42).
A publication from Zippel et al. (2022) detailed a similar approach of culturing HSPC, AML cells, and BMSCs in perfusable porous hydrogels (112). The hydrogel is composed of a PEG diacrylate (PEGDA) backbone crosslinked with a cell adhesive peptide sequence RGD (RGD-acrylate) and, methacrylated magnetic nanoparticles (Figure 3A). The magnetic hydrogels are suspended in the wells of deep 12-well plates housed in a magnetic lift system which provides a controlled movement of the hydrogel. The models overcome the diffusion-limited transport of growth factors and media components in 3D culture without the need for stirring, shaking, or pumping (116). In addition, the parallelized system allows for simultaneous testing for up to 12 conditions or treatments at a time. AML cell line KG-1a, a promyeloblast macrophage line, was cultured in either a coculture system with BMSCs isolated from human BM or a triculture system with umbilical blood-derived HSPCs and BMSCs in the magnetic hydrogel. Treatments of the hydrogels with cyclophosphamide (CPA) and 5-fluorouracil (5-FU) showed a higher cell viability of KG-1a cells in 3D coculture as compared to static 2D culture indicating a higher chemotherapeutic resistance in 3D (112). To enable continuous monitoring of the cell conditions, the authors proposed non-invasive metabolic profiling of the supernatant during chemotherapeutic treatments. Particularly, lactate, glucose, and adenosine concentrations were measured at different days after treatments. These metabolites are chosen because active cells consume glucose and produce lactate and adenosine (112). The authors showed that the metabolic profile can potentially vary depending on the chemotherapeutic chosen. In the 3D coculture of KG-1a cells with BMSCs, CPA caused a decreased metabolic activity while imatinib (IMA) caused the opposite reaction.
Houshmand et al., 2017 described a hydrogel system composed of demineralized bone matrix (DBM) coated with collagen in a single-channel microfluidics assembly to mimic interstitial fluid flow in the bone marrow stroma (41) (Figure 3A). TF-1 cells were cultured with BMSCs in the 3D hydrogel in a microfluidics setup and 2D condition. Scanning electron microscopy showed that TF-1 cells are in proximity with BMSCs, indicating that the cells invaded the microenvironment and formed a comfortable niche in the 3D culture. The findings also show that in the 3D condition, TF-1 cells exhibited retained CD34+/CD38- phenotype, increased proliferation, and elevated chemoresistance to azacitidine and Ara-C in 3D culture as compared to 2D microfluidic culture. qPCR showed that BCL2 is elevated after chemotherapy treatment which recapitulated one of the mechanisms of drug resistance shown before (95).
4.4.2 Mimicking vasculature flow and osteoblastic niche
Incorporation of the vascular component with media flow can mimic the blood flow in microvasculature in the BMME. We believe that a dynamic vascular component is a requirement for future BMME MPS developments. Recreating distinct arteriole and sinusoidal niches requires either intricate cell isolation procedures from these niches or robust differentiation protocols that can generate both components. However, neither approach has been fully established yet. Currently, state of the art approaches includes creating an endothelium mimetic with flow in a microfluidic channels or perfusable self-assembled endothelium tubes in hydrogels (Figure 3B).
In 2020, the Chou et al. published an article demonstrating the design of a primary human bone marrow chip in PDMS microfluidic device with two-channels separated by a porous membrane (60) (Figure 3B). The top channel is the hematopoietic channel with a fibrin hydrogel containing BMSC, CD34+ HSPCs while the bottom vascular channel consist of an endothelium lining each surface of the channel. The authors showed sustained myeloerythroid proliferation of HSPCs and predicted clinically relevant hematopoietic toxicity to 5-FU (60). Our approach relied on the use of a commercial version of the described platform by systems from Emulate Inc, Chip-S1®. By using a commercially available device, we can focus on studying the BMME cell interactions with HSPCs without spending the resources on fabrication. In this setup, we incorporated an endosteal niche by culturing osteoblastic cells differentiated from BMSCs, BMSCs, and HSPCs in fibrin hydrogel in the top channel, and endothelial cell-lined bottom channel with fluid flow. We showed that HSPCs from mice were maintained for at least 2 weeks in culture and were able to engraft in mice and differentiate into mature myeloid and lymphoid cells (114).
The approach described in Glaser et al. (2022), consists of a two hexagonal chambers connected by two-way ports that separate an osteoblastic niche and a perivascular niche (113) (Figure 3B). The vascular niche is created using human cord blood-derived endothelial cells and primary human BMSC in a fibrin hydrogel. When interstitial flow is introduced, the endothelial cells self-assemble into perfusable microvascular network via vasculogenesis. The osteoblastic niche consists of osteoblast cell line hFOB 1.19 with endothelial cells in fibrin. After 7 days of culture, SCF, ICAM-1, VCAM-1 and E-selectin were observed on the vessels of both chambers. The authors indicated that the presence of VCAM-1 may demonstrate recapitulation of arterioles in the bone marrow (113). The approach resulted in both chambers with perfusable vasculature networks with different permeability that support CD34+ HSPCs. Nelson et al. (2021), took a similar approach of developing a perivascular niche and a vascularized endosteal niche in a custom-made 5-channel PDMS microfluidics systems using soft-lithography (62) (Figure 3B). The resulting device consists of various cells including BMSCs, pericytes, osteoblasts, CAR cells, hematopoietic cells, and adipose cells. Among the analyses performed was an investigation into the protection of HSPCs from ionizing radiation. The authors demonstrated that the endosteal niche reduced apoptosis in HSPCs (62). Although these systems are not yet optimized for AML studies, the findings demonstrate that culturing endosteal and vascular niches present in the BMME is feasible, highlighting the need for further research to develop a physiological BMME in vitro.
5 Considerations and future directions
5.1 The need for validation of in vivo studies in the MPS model
Validation of clinical and physiological relevance of in vitro findings from MPS models are still a major obstacle. Currently, there is still a lack of conclusive evidence that 3D cell-based systems can accurately recapitulate disease pathophysiology (117). Sufficient quantitative and reproducible data must be provided to replace current 2D models already used in academic and industry laboratories (117). Indeed, the majority of 3D culture models discussed in this review have shown that when AML cells, whether derived from cell lines or primary AML cells, are cultured within hydrogel systems, they exhibit chemoresistance. While the findings from these studies validate a crucial phenotype within the leukemic BMME, there remain additional phenotypes that require validation to ensure the achievement of a comprehensive physiological recapitulation of the BMME. The majority of the articles reviewed in the previous section have been mainly focused on validating CAM-DR that is seen in vivo, one that conventional 2D cultures struggle to replicate. Particularly, the findings supported the idea that BMSCs, osteoblastic cells, endothelial cells, and matrix interactions can provide a protective niche for leukemic cells to evade chemotherapies. In addition, the goal of the 3D culture systems was focused on maintaining AML cell numbers and stemness in the presence of an artificial matrix or BMME niche components. AML drug research that uses established cell lines often lacks proper healthy cell controls such as healthy HSPCs (118). For drug discovery purposes, the drugs must show efficacy to AML cells and at the same time the absence of toxicity to the BMME cells and HSPCs (118).
More importantly, we believe that there needs to be an emphasis on the validation of key phenotypes of AML interactions with BMME components that were shown to promote disease progression. For example, we and others have shown that AML cells can modulate the BMME by functionally inhibiting osteoblastic cell activity and BMSC differentiation into mature osteoblasts (14, 92, 119–122). Leukemic cells can disrupt osteoblastic cell activity through secreted factors such as TPO and CCL3 (14, 21). As osteoblastic cells and BMSCs modulate HSPC quiescence, the modulation of their function negatively impacts the hematopoietic compartment in the bone marrow. In addition, in AML, the endosteal vascular niche is remodeled from anti-angiogenic and inflammatory cytokines secreted by leukemic cells such as TNF and CXCL12 (21). The downstream effects of these interactions include damage to BMSCs, endothelial cells, and osteoblasts which lead to impaired ability of the endosteal niche to support HSC maintenance (21). Findings such as these, which were shown in vivo experiments, must be validated in 3D culture systems to ensure that the system can mimic not only the AML maintenance but also the leukemic cell interactions with the BMME components in vitro. Validation of works performed in vitro and in vivo must be done to ensure a faithful recapitulation of the AML BMME. Findings from such experiments can provide evidence to drive the adoption of 3D culture systems in AML research.
5.2 Accounting cytogenetic heterogeneity in AML
The underlying genomic and molecular complexity of AML makes it challenging to find therapies that will benefit the majority of AML patients. As evident from previous studies, responses to hydrogel materials and chemotherapies in 3D culture can vary depending on the AML cell source (35, 39, 102, 109). These works suggest the need for exploring multiple cell types, either primary cells or cell lines to capture the heterogenous responses associated with cytogenetic abnormalities in AML. Multiple immortalized cell lines have been established throughout the years to study AML cell mechanisms, chemoresistance, and drug discovery which have provided valuable findings to the AML field. Skopek et al. (2023) provided a comprehensive review of the AML cell types commonly used in research (118). A summary of the cell lines used in the 3D culture systems is listed in Table 2. MPS developers must carefully select AML cell lines that take into account the genetic mutations. For example, the NPM1 mutations are the most common genetic alteration in adult AML, but the occurrence of the mutation is only in about 30% of total cases (118, 125). For instance, researchers exploring phenotypes linked to NPM1 mutations may find it beneficial to select cell lines like OCI-AML3 to narrow down the focus of their 3D culture investigations.
5.3 Considerations for sex, race and ethnicities considerations
The American Cancer Society estimated that there will be 62,770 new cases and 23,670 deaths of leukemia in 2024 based on the NCI SEER cancer statistics (126). Specifically in AML, the estimated new cases and deaths for males are higher as compared to females. In most invasive cancers, men are at higher risk of being diagnosed with cancer (41.6%) compared to women (39.6%). This is believed to be caused by greater exposure to carcinogenic environmental and lifestyle factors (126, 127). However, male predominance is still largely unexplained by risk factors and therefore the role of sex-related biological factors such as endogenous hormone exposure and immune function must be further investigated (126–128). Most AML cell lines were derived from male patients of white or Asian descent potentially due to the predominance of AML incidence in males (Table 2). In B-cell chronic lymphocytic leukemia (CLL), in which the predominance is also in males, varying DNA methylation patterns between men and women may contribute to the sex-related difference in CLL risk (129). Although results in intrinsic sex differences are still limited, 3D culture systems could potentially serve as a tool to study cellular sex differences in the BMME and malignancy.
Although the role of race and ethnicity is not fully understood in cell culture, the potential implications should be considered especially in the context of preclinical drug testing. The lack of racial and ethnic diversity can potentially contribute to cancer health disparities. A review in lung cancer recently published found over 800 lung cancer cell lines came mostly from white and Asian patients although lung cancer disproportionately impacts Black individuals in the US (130). In addition, the authors highlighted that no cell lines were identified from other groups such as Hispanic/latin(x), American Indian/American Native, or Native Hawaiian or other Pacific Islander (130). The review paper highlighted the need to establish additional cell lines to ensure the representation of all population groups in critical pre-clinical research. In the context of AML, the disparities in clinical trial enrollment, cancer cell biobanks, and in-hospital death rates in AML, highlight the need to acknowledge the lack of representation in research and care settings, and preclinical studies (131). In terms of cytogenetic landscape, multiple findings have associated ancestry-associated differences that contribute to molecular features in AML (131). For example, studies have reported that Black AML patients have lower frequencies of NPM1 and WT1 mutations and higher frequencies of IDH1/2-mutation (131, 132). As the understanding of the influence of race and ethnicity emerges, the field should anticipate the integration of these factors into basic and preclinical research.
5.4 Readiness of 3D culture platforms in AML biomedical research
MPS and 3D culture platforms are advantageous in drug discovery as they can recapitulate the complex tissue microstructures while reducing the exhaustive requirements of animal models (49–54). The field is still at its early stages with new models developed with modified approaches such as composite hydrogels with varying stiffness and bioprinted HTS systems (109, 133). However, the adoption of MPS and 3D culture approaches in AML research remains limited. MPS is still an emerging field facing challenges with validation, standardization, and acceptance (134). Standardization will be necessary to ensure the adoptability of 3D culture systems into preclinical studies. One of the primary hurdles in embracing 3D culture techniques, particularly concerning hydrogel construction in AML research, stems from the need to adopt the material fabrication techniques and equipment associated. As evident in this review, researchers must also select from a wide range of biomaterials as there is no standard hydrogel. This is a significant challenge for basic science or clinical labs lacking backgrounds in tissue engineering and biomaterials. Most AML 3D culture systems detailed in literature are custom-built, leading to significant challenges in seamlessly transferring these systems to other research laboratories. In this regard, using a commercially available device may be beneficial; however, cost must be considered, as these devices are newer to the market and can be expensive. Consideration must also be given to the cost of animal models, including the development of new syngeneic or genetic models to study specific BMME and AML cell interactions. Additionally, there is a cost associated with untranslatable findings from 2D culture models to clinical trials.
So far, this review has covered various 3D models encompassing static hydrogels, perfusable hydrogels, and microfluidics-based bone marrow on-a-chip. These models have provided findings that showed recapitulation of chemotherapy resistance of AML cells from matrix and niche cell interactions, and establishment of functional niches that support HSPC maintenance. In addition, the models have also shown distinct responses dependent on AML cell sources, whether primary or cell lines, that may reflect patient-specific responses to chemotherapies and thus provide more relevance to human studies compared to 2D or animal studies. To facilitate the adoption of 3D culture systems into preclinical studies, developers perhaps should consider aligning their approach to a specific context of use (CoU). Specific CoUs for MPS platforms currently include toxicology pharmacokinetics (ADME), pharmacodynamics, efficacy, and drug safety. By defining the CoU or particular goals of the devices, developers can define the strengths and limitations of their platform to convince stakeholders and researchers to adopt their systems. Examples of CoU or goals that are ready to be investigated in the 3D culture of AML BMME include investigating: 1) gender disparities using different cell sources; 2) aging BMME and AML cell progression; 3) initiation of disease due to perturbation of BMME in human cells; 4) development of therapy-related AML (t-AML); and 5) polypharmacy effects in BMME.
Author contributions
AS: Conceptualization, Visualization, Writing – original draft, Writing – review & editing. BF: Conceptualization, Writing – review & editing.
Funding
The author(s) declare financial support was received for the research, authorship, and/or publication of this article. This work was supported by funding from the Wilmot Cancer Institute and the American Cancer Society Discovery grant (RSG #135889) awarded to BF.
Acknowledgments
We would like to acknowledge Dr. Danielle Benoit, at the University of Oregon for her mentorship and support for AS’s thesis research. We also thank the reviewers for providing feedback and comments that helped refined the writing of this review article.
Conflict of interest
The authors declare that the research was conducted in the absence of any commercial or financial relationships that could be construed as a potential conflict of interest.
Publisher’s note
All claims expressed in this article are solely those of the authors and do not necessarily represent those of their affiliated organizations, or those of the publisher, the editors and the reviewers. Any product that may be evaluated in this article, or claim that may be made by its manufacturer, is not guaranteed or endorsed by the publisher.
References
1. Hoggatt J, Pelus LM. The Factory of Blood Production: Hematopoietic Stem Cells. In: Pelus LM, Hoggatt J, editors. Hematopoietic Stem Cells: Methods and Protocols. Springer US, New York, NY (2023). p. 3–7.
2. Pinho S, Frenette PS. Haematopoietic stem cell activity and interactions with the niche. Nat Rev Mol Cell Biol. (2019) 20:303–20. doi: 10.1038/s41580-019-0103-9
3. Wiese M, Daver N. Unmet clinical needs and economic burden of disease in the treatment landscape of acute myeloid leukemia. Am J Manag Care. (2018) 24:S347–s55.
4. Khwaja A, Bjorkholm M, Gale RE, Levine RL, Jordan CT, Ehninger G, et al. Acute myeloid leukaemia. Nat Rev Dis Primers. (2016) 2:16010. doi: 10.1038/nrdp.2016.10
5. Shallis RM, Wang R, Davidoff A, Ma X, Zeidan AM. Epidemiology of acute myeloid leukemia: Recent progress and enduring challenges. Blood Rev. (2019) 36:70–87. doi: 10.1016/j.blre.2019.04.005
6. Yi M, Li A, Zhou L, Chu Q, Song Y, Wu K. The global burden and attributable risk factor analysis of acute myeloid leukemia in 195 countries and territories from 1990 to 2017: estimates based on the global burden of disease study 2017. J Hematol Oncol. (2020) 13:72. doi: 10.1186/s13045-020-00908-z
7. Surveillance, Epidemiology, and End Results (SEER). SEER*Explorer Database: Acute Myeloid Leukemia (AML) SEER Relative Survival by Time Since Diagnosis, 2000-2018 (2000-2018). Available online at: www.seer.cancer.goxhttps://seer.cancer.gov/statistics-network/explorer/application.html (Accessed March 12 2023).
8. Acute Myeloid Leukemia (AML) SEER 5-year Relative Survival Rates, 2012-2018 (2012-2018). Available online at: https://seer.cancer.gov/statistics-network/explorer/application.html (Accessed March 12 2023).
9. Kantarjian H, Kadia T, DiNardo C, Daver N, Borthakur G, Jabbour E, et al. Acute myeloid leukemia: current progress and future directions. Blood Cancer J. (2021) 11:41–. doi: 10.1038/s41408-021-00425-3
10. Roussel X, Daguindau E, Berceanu A, Desbrosses Y, Warda W, Neto da Rocha M, et al. Acute myeloid leukemia: from biology to clinical practices through development and pre-clinical therapeutics. Front Oncol. (2020) 10:599933–. doi: 10.3389/fonc.2020.599933
11. Bhansali RS, Pratz KW, Lai C. Recent advances in targeted therapies in acute myeloid leukemia. J Hematol Oncol. (2023) 16:29. doi: 10.1186/s13045-023-01424-6
12. Döhner H, Weisdorf DJ, Bloomfield CD. Acute myeloid leukemia. N Engl J Med. (2015) 373:1136–52. doi: 10.1056/NEJMra1406184
13. Fröbel J, Landspersky T, Percin G, Schreck C, Rahmig S, Ori A, et al. The hematopoietic bone marrow niche ecosystem. Front Cell Dev Biol. (2021) 9:705410. doi: 10.3389/fcell.2021.705410
14. Frisch BJ, Ashton JM, Xing L, Becker MW, Jordan CT, Calvi LM. Functional inhibition of osteoblastic cells in an in vivo mouse model of myeloid leukemia. Blood. (2012) 119:540–50. doi: 10.1182/blood-2011-04-348151
15. Shafat MS, Gnaneswaran B, Bowles KM, Rushworth SA. The bone marrow microenvironment – Home of the leukemic blasts. Blood Rev. (2017) 31:277–86. doi: 10.1016/j.blre.2017.03.004
16. Staversky RJ, Byun DK, Georger MA, Zaffuto BJ, Goodman A, Becker MW, et al. The chemokine CCL3 regulates myeloid differentiation and hematopoietic stem cell numbers. Sci Rep. (2018) 8:14691. doi: 10.1038/s41598-018-32978-y
17. Krevvata M, Silva BC, Manavalan JS, Galan-Diez M, Kode A, Matthews BG, et al. Inhibition of leukemia cell engraftment and disease progression in mice by osteoblasts. Blood J Am Soc Hematol. (2014) 124:2834–46. doi: 10.1182/blood-2013-07-517219
18. Ryningen A, Wergeland L, Glenjen N, Gjertsen BT, Bruserud Ø. In vitro crosstalk between fibroblasts and native human acute myelogenous leukemia (AML) blasts via local cytokine networks results in increased proliferation and decreased apoptosis of AML cells as well as increased levels of proangiogenic Interleukin 8. Leuk Res. (2005) 29:185–96. doi: 10.1016/j.leukres.2004.06.008
19. Pezeshkian B, Donnelly C, Tamburo K, Geddes T, Madlambayan GJ. Leukemia mediated endothelial cell activation modulates leukemia cell susceptibility to chemotherapy through a positive feedback loop mechanism. PloS One. (2013) 8:e60823. doi: 10.1371/journal.pone.0060823
20. Ngo S, Papazoglou D, Huerga Encabo H, Bonnet D. Exploring the intricate cross-talk between clonal expansion and the bone marrow niche. Front Hematol. (2024) 3. doi: 10.3389/frhem.2024.1334807
21. Soto CA, Lo Celso C, Purton LE, Frisch BJ. From the niche to Malignant hematopoiesis and back: reciprocal interactions between leukemia and the bone marrow microenvironment. JBMR Plus. (2021) 5:e10516. doi: 10.1002/jbm4.10516
22. Arai F, Hosokawa K, Toyama H, Matsumoto Y, Suda T. Role of N-cadherin in the regulation of hematopoietic stem cells in the bone marrow niche. Ann N Y Acad Sci. (2012) 1266:72–7. doi: 10.1111/j.1749-6632.2012.06576.x
23. Li D, Lin TL, Lipe B, Hopkins RA, Shinogle H, Aljitawi OS. A novel extracellular matrix-based leukemia model supports leukemia cells with stem cell-like characteristics. Leuk Res. (2018) 72:105–12. doi: 10.1016/j.leukres.2018.08.012
24. Nair MS, Mony U, Menon D, Koyakutty M, Sidharthan N, Pavithran K, et al. Development and molecular characterization of polymeric micro-nanofibrous scaffold of a defined 3-D niche for in vitro chemosensitivity analysis against acute myeloid leukemia cells. Int J Nanomed. (2015) 10:3603–22. doi: 10.2147/ijn.s80397
25. Huang Y, Wang Y, Tang J, Qin S, Shen X, He S, et al. CAM-DR: mechanisms, roles and clinical application in tumors. Front Cell Dev Biol. (2021) 9. doi: 10.3389/fcell.2021.698047
26. Kim HN, Ruan Y, Ogana H, Kim Y-M. Cadherins, selectins, and integrins in CAM-DR in leukemia. Front Oncol. (2020) 10. doi: 10.3389/fonc.2020.592733
27. Zhuang L, Darley R, Ottmann OG, Zabkiewicz J, Alvares C. Bone marrow stromal cells mediate adhesion based drug resistance in acute myeloid leukaemia through reciprocal feedback of the β-catenin/CD44 signalling axis. Blood. (2018) 132:2776. doi: 10.1182/blood-2018-99-113811
28. Raaijmakers MH, Mukherjee S, Guo S, Zhang S, Kobayashi T, Schoonmaker JA, et al. Bone progenitor dysfunction induces myelodysplasia and secondary leukaemia. Nature. (2010) 464:852–7. doi: 10.1038/nature08851
29. Kode A, Manavalan JS, Mosialou I, Bhagat G, Rathinam CV, Luo N, et al. Leukaemogenesis induced by an activating β-catenin mutation in osteoblasts. Nature. (2014) 506:240–4. doi: 10.1038/nature12883
30. Dozzo A, Galvin A, Shin J-W, Scalia S, O’Driscoll CM, Ryan KB. Modelling acute myeloid leukemia (AML): What’s new? A transition from the classical to the modern. Drug Deliv Transl Res. (2022) 13(8):2110–41. doi: 10.1007/s13346-022-01189-4
31. Moore JW, Mendler JH, Loh KP, Azadniv M, O'Dwyer KM, Huselton EJ, et al. Therapeutic modulation of the marrow microenvironment in MDS: A phase I trial of abaloparatide and bevacizumab. Blood. (2022) 140:4050–2. doi: 10.1182/blood-2022-159515
32. DeAngelo DJ, Jonas BA, Liesveld JL, Bixby DL, Advani AS, Marlton P, et al. Phase 1/2 study of uproleselan added to chemotherapy in patients with relapsed or refractory acute myeloid leukemia. Blood. (2022) 139:1135–46. doi: 10.1182/blood.2021010721
33. Chramiec A, Vunjak-Novakovic G. Tissue engineered models of healthy and Malignant human bone marrow. Adv Drug Delivery Rev. (2019) 140:78–92. doi: 10.1016/j.addr.2019.04.003
34. Cartledge Wolf DM, Langhans SA. Moving myeloid leukemia drug discovery into the third dimension. Front Pediatr. (2019) 7. doi: 10.3389/fped.2019.00314
35. Aljitawi OS, Li D, Xiao Y, Zhang D, Ramachandran K, Stehno-Bittel L, et al. A novel three-dimensional stromal-based model for in vitro chemotherapy sensitivity testing of leukemia cells. Leuk Lymph. (2014) 55:378–91. doi: 10.3109/10428194.2013.793323
36. Shen ZH, Zeng DF, Wang XY, Ma YY, Zhang X, Kong PY. Targeting of the leukemia microenvironment by c(RGDfV) overcomes the resistance to chemotherapy in acute myeloid leukemia in biomimetic polystyrene scaffolds. Oncol Lett. (2016) 12:3278–84. doi: 10.3892/ol.2016.5042
37. Shin JW, Mooney DJ. Extracellular matrix stiffness causes systematic variations in proliferation and chemosensitivity in myeloid leukemias. Proc Natl Acad Sci U S A. (2016) 113:12126–31. doi: 10.1073/pnas.1611338113
38. Xu H, Muise ES, Javaid S, Chen L, Cristescu R, Mansueto MS, et al. Identification of predictive genetic signatures of Cytarabine responsiveness using a 3D acute myeloid leukaemia model. J Cell Mol Med. (2019) 23:7063–77. doi: 10.1111/jcmm.14608
39. Alhallak K, de la Puente P, Jeske A, Sun J, Muz B, Rettig MP, et al. 3D tissue engineered plasma cultures support leukemic proliferation and induces drug resistance. Leuk Lymph. (2021) 62:2457–65. doi: 10.1080/10428194.2021.1919657
40. Borella G, Da Ros A, Borile G, Porcù E, Tregnago C, Benetton M, et al. Targeting the plasticity of mesenchymal stromal cells to reroute the course of acute myeloid leukemia. Blood. (2021) 138:557–70. doi: 10.1182/blood.2020009845
41. Houshmand M, Soleimani M, Atashi A, Saglio G, Abdollahi M, Nikougoftar Zarif M. Mimicking the acute myeloid leukemia niche for molecular study and drug screening. Tissue Eng Part C Methods. (2017) 23:72–85. doi: 10.1089/ten.tec.2016.0404
42. García-García A, Klein T, Born G, Hilpert M, Scherberich A, Lengerke C, et al. Culturing patient-derived Malignant hematopoietic stem cells in engineered and fully humanized 3D niches. Proc Natl Acad Sci U.S.A. (2021) 118. doi: 10.1073/pnas.2114227118
43. Liebers N, Bruch P-M, Terzer T, Hernandez-Hernandez M, Paramasivam N, Fitzgerald D, et al. Ex vivo drug response profiling for response and outcome prediction in hematologic Malignancies: the prospective non-interventional SMARTrial. Nat Cancer. (2023) 4:1648–59. doi: 10.1038/s43018-023-00645-5
44. Döhner H, Wei AH, Appelbaum FR, Craddock C, DiNardo CD, Dombret H, et al. Diagnosis and management of AML in adults: 2022 recommendations from an international expert panel on behalf of the ELN. Blood. (2022) 140:1345–77. doi: 10.1182/blood.2022016867
45. Truskey GA. Human microphysiological systems and organoids as in vitro models for toxicological studies. Front Public Health. (2018) 6. doi: 10.3389/fpubh.2018.00185
46. Baptista LS, Porrini C, Kronemberger GS, Kelly DJ, Perrault CM. 3D organ-on-a-chip: The convergence of microphysiological systems and organoids. Front Cell Dev Biol. (2022) 10. doi: 10.3389/fcell.2022.1043117
47. Campisi M, Lim SH, Chiono V, Kamm RD. 3D self-organized human blood-brain barrier in a microfluidic chip. Methods Mol Biol. (2021) 2258:205–19. doi: 10.1007/978-1-0716-1174-6_14
48. Criscione J, Rezaei Z, Hernandez Cantu CM, Murphy S, Shin SR, Kim D-H. Heart-on-a-chip platforms and biosensor integration for disease modeling and phenotypic drug screening. Biosensors Bioelectron. (2023) 220:114840. doi: 10.1016/j.bios.2022.114840
49. Kelm JM, Lal-Nag M, Sittampalam GS, Ferrer M. Translational in vitro research: integrating 3D drug discovery and development processes into the drug development pipeline. Drug Discovery Today. (2019) 24:26–30. doi: 10.1016/j.drudis.2018.07.007
50. Low LA, Tagle DA. Tissue chips - innovative tools for drug development and disease modeling. Lab Chip. (2017) 17:3026–36. doi: 10.1039/C7LC00462A
51. Huh D, Hamilton GA, Ingber DE. From 3D cell culture to organs-on-chips. Trends Cell Biol. (2011) 21:745–54. doi: 10.1016/j.tcb.2011.09.005
52. Low LA, Tagle DA. Microphysiological systems (Tissue chips) and their utility for rare disease research. Adv Exp Med Biol. (2017) 1031:405–15. doi: 10.1007/978-3-319-67144-4_23
53. Low LA, Sutherland M, Lumelsky N, Selimovic S, Lundberg MS, Tagle DA. Organs-on-a-chip. Adv Exp Med Biol. (2020) 1230:27–42. doi: 10.1007/978-3-030-36588-2_3
54. Osaki T, Sivathanu V, Kamm RD. Vascularized microfluidic organ-chips for drug screening, disease models and tissue engineering. Curr Opin Biotechnol. (2018) 52:116–23. doi: 10.1016/j.copbio.2018.03.011
55. U.S. Food, Drug Administration. Advancing Alternative Methods at FDA . Available online at: https://www.fda.gov/science-research/about-science-research-fda/advancing-alternative-methods-fda (Accessed January 5, 2020).
56. Chatterjee C, Schertl P, Frommer M, Ludwig-Husemann A, Mohra A, Dilger N, et al. Rebuilding the hematopoietic stem cell niche: Recent developments and future prospects. Acta Biomater. (2021) 132:129–48. doi: 10.1016/j.actbio.2021.03.061
57. Zushin PH, Mukherjee S, Wu JC. FDA Modernization Act 2.0: transitioning beyond animal models with human cells, organoids, and AI/ML-based approaches. J Clin Invest. (2023) 133. doi: 10.1172/jci175824
58. da Silva RGL, Blasimme A. Organ chip research in Europe: players, initiatives, and policies. Front Bioeng Biotechnol. (2023) 11:1237561. doi: 10.3389/fbioe.2023.1237561
59. Sieber S, Wirth L, Cavak N, Koenigsmark M, Marx U, Lauster R, et al. Bone marrow-on-a-chip: Long-term culture of human haematopoietic stem cells in a three-dimensional microfluidic environment. J Tissue Eng Regener Med. (2018) 12:479–89. doi: 10.1002/term.2507
60. Chou DB, Frismantas V, Milton Y, David R, Pop-Damkov P, Ferguson D, et al. On-chip recapitulation of clinical bone marrow toxicities and patient-specific pathophysiology. Nat Biomed Eng. (2020) 4:394–406. doi: 10.1038/s41551-019-0495-z
61. Di Maggio N, Piccinini E, Jaworski M, Trumpp A, Wendt DJ, Martin I. Toward modeling the bone marrow niche using scaffold-based 3D culture systems. Biomaterials. (2011) 32:321–9. doi: 10.1016/j.biomaterials.2010.09.041
62. Nelson MR, Ghoshal D, Mejías JC, Rubio DF, Keith E, Roy K. A multi-niche microvascularized human bone marrow (hBM) on-a-chip elucidates key roles of the endosteal niche in hBM physiology. Biomaterials. (2021) 270:120683. doi: 10.1016/j.biomaterials.2021.120683
63. Cook MM, Futrega K, Osiecki M, Kabiri M, Kul B, Rice A, et al. Micromarrows—Three-dimensional coculture of hematopoietic stem cells and mesenchymal stromal cells. Tissue Eng Part C: Methods. (2011) 18:319–28. doi: 10.1089/ten.tec.2011.0159
64. Bourgine PE, Klein T, Paczulla AM, Shimizu T, Kunz L, Kokkaliaris KD, et al. In vitro biomimetic engineering of a human hematopoietic niche with functional properties. Proc Natl Acad Sci. (2018) 115:E5688–e95. doi: 10.1073/pnas.1805440115
65. Congrains A, Bianco J, Rosa RG, Mancuso RI, Saad STO. 3D scaffolds to model the hematopoietic stem cell niche: applications and perspectives. Mater (Basel). (2021) 14:569. doi: 10.3390/ma14030569
66. Torisawa YS, Spina CS, Mammoto T, Mammoto A, Weaver JC, Tat T, et al. Bone marrow-on-a-chip replicates hematopoietic niche physiology in vitro. Nat Methods. (2014) 11:663–9. doi: 10.1038/nmeth.2938
67. Rödling L, Schwedhelm I, Kraus S, Bieback K, Hansmann J, Lee-Thedieck C. 3D models of the hematopoietic stem cell niche under steady-state and active conditions. Sci Rep. (2017) 7:4625. doi: 10.1038/s41598-017-04808-0
68. Abarrategi A, Mian SA, Passaro D, Rouault-Pierre K, Grey W, Bonnet D. Modeling the human bone marrow niche in mice: From host bone marrow engraftment to bioengineering approaches. J Exp Med. (2018) 215:729–43. doi: 10.1084/jem.20172139
69. Khan AO, Rodriguez-Romera A, Reyat JS, Olijnik AA, Colombo M, Wang G, et al. Human bone marrow organoids for disease modeling, discovery, and validation of therapeutic targets in hematologic Malignancies. Cancer Discovery. (2023) 13:364–85. doi: 10.1158/2159-8290.CD-22-0199
70. Busch C, Nyamondo K, Wheadon H. Complexities of modeling the bone marrow microenvironment to facilitate hematopoietic research. Exp Hematol. (2024). 135:104233. doi: 10.1016/j.exphem.2024.104233
71. Choi JS, Mahadik BP, Harley BAC. Engineering the hematopoietic stem cell niche: Frontiers in biomaterial science. Biotechnol J. (2015) 10:1529–45. doi: 10.1002/biot.201400758
72. Hoggatt J, Pelus LM. Mobilization of hematopoietic stem cells from the bone marrow niche to the blood compartment. Stem Cell Res Ther. (2011) 2:13. doi: 10.1186/scrt54
73. Schofield R. The relationship between the spleen colony-forming cell and the haemopoietic stem cell. Blood Cells. (1978) 4:7–25.
74. Krause DS, Scadden DT, Preffer FI. The hematopoietic stem cell niche—home for friend and foe? Cytom Part B: Clin Cytom. (2013) 84B:7–20. doi: 10.1002/cyto.b.21066
75. Gattazzo F, Urciuolo A, Bonaldo P. Extracellular matrix: a dynamic microenvironment for stem cell niche. Biochim Biophys Acta. (2014) 1840:2506–19. doi: 10.1016/j.bbagen.2014.01.010
76. Shin JW, Buxboim A, Spinler KR, Swift J, Christian DA, Hunter CA, et al. Contractile forces sustain and polarize hematopoiesis from stem and progenitor cells. Cell Stem Cell. (2014) 14:81–93. doi: 10.1016/j.stem.2013.10.009
77. Swift J, Ivanovska IL, Buxboim A, Harada T, Dingal PC, Pinter J, et al. Nuclear lamin-A scales with tissue stiffness and enhances matrix-directed differentiation. Science. (2013) 341:1240104. doi: 10.1126/science.1240104
78. Akhmanova M, Osidak E, Domogatsky S, Rodin S, Domogatskaya A. Physical, spatial, and molecular aspects of extracellular matrix of in vivo niches and artificial scaffolds relevant to stem cells research. Stem Cells Int. (2015) 2015:167025. doi: 10.1155/2015/167025
79. Klamer S, Voermans C. The role of novel and known extracellular matrix and adhesion molecules in the homeostatic and regenerative bone marrow microenvironment. Cell Adh Migr. (2014) 8:563–77. doi: 10.4161/19336918.2014.968501
80. He N, Zhang L, Cui J, Li Z. Bone marrow vascular niche: home for hematopoietic stem cells. Bone Marrow Res. (2014) 2014:128436. doi: 10.1155/2014/128436
81. Morrison SJ, Scadden DT. The bone marrow niche for haematopoietic stem cells. Nature. (2014) 505:327–34. doi: 10.1038/nature12984
82. Greim H, Kaden DA, Larson RA, Palermo CM, Rice JM, Ross D, et al. The bone marrow niche, stem cells, and leukemia: impact of drugs, chemicals, and the environment. Ann N Y Acad Sci. (2014) 1310:7–31. doi: 10.1111/nyas.12362
83. Wobus M, Bornhäuser M. Editorial: Deciphering the bone marrow microenvironment in hematologic Malignancies. Front Oncol. (2023) 13. doi: 10.3389/fonc.2023.1231467
84. Chen L, Pronk E, van Dijk C, Bian Y, Feyen J, van Tienhoven T, et al. A single-cell taxonomy predicts inflammatory niche remodeling to drive tissue failure and outcome in human AML. Blood Cancer Discovery. (2023) 4:394–417. doi: 10.1158/2643-3230.BCD-23-0043
85. Baryawno N, Przybylski D, Kowalczyk MS, Kfoury Y, Severe N, Gustafsson K, et al. A cellular taxonomy of the bone marrow stroma in homeostasis and leukemia. Cell. (2019) 177:1915–32.e16. doi: 10.1016/j.cell.2019.04.040
86. Park D, Spencer JA, Koh BI, Kobayashi T, Fujisaki J, Clemens TL, et al. Endogenous bone marrow MSCs are dynamic, fate-restricted participants in bone maintenance and regeneration. Cell Stem Cell. (2012) 10:259–72. doi: 10.1016/j.stem.2012.02.003
87. Worthley DL, Churchill M, Compton JT, Tailor Y, Rao M, Si Y, et al. Gremlin 1 identifies a skeletal stem cell with bone, cartilage, and reticular stromal potential. Cell. (2015) 160:269–84. doi: 10.1016/j.cell.2014.11.042
88. Zhou BO, Yue R, Murphy MM, Peyer JG, Morrison SJ. Leptin-receptor-expressing mesenchymal stromal cells represent the main source of bone formed by adult bone marrow. Cell Stem Cell. (2014) 15:154–68. doi: 10.1016/j.stem.2014.06.008
89. Gu L, Liao P, Liu H. Cancer-associated fibroblasts in acute leukemia. Front Oncol. (2022) 12. doi: 10.3389/fonc.2022.1022979
90. Zhai Y, Zhang J, Wang H, Lu W, Liu S, Yu Y, et al. Growth differentiation factor 15 contributes to cancer-associated fibroblasts-mediated chemo-protection of AML cells. J Exp Clin Cancer Res. (2016) 35:147. doi: 10.1186/s13046-016-0405-0
91. Scadden DT. Nice neighborhood: Emerging concepts of the stem cell niche. Cell. (2014) 157:41–50. doi: 10.1016/j.cell.2014.02.013
92. Duarte D, Hawkins ED, Akinduro O, Ang H, De Filippo K, Kong IY, et al. Inhibition of endosteal vascular niche remodeling rescues hematopoietic stem cell loss in AML. Cell Stem Cell. (2018) 22:64–77.e6. doi: 10.1016/j.stem.2017.11.006
93. Passaro D, Di Tullio A, Abarrategi A, Rouault-Pierre K, Foster K, Ariza-McNaughton L, et al. Increased vascular permeability in the bone marrow microenvironment contributes to disease progression and drug response in acute myeloid leukemia. Cancer Cell. (2017) 32:324–41.e6. doi: 10.1016/j.ccell.2017.08.001
94. Izzi V, Lakkala J, Devarajan R, Savolainen ER, Koistinen P, Heljasvaara R, et al. Expression of a specific extracellular matrix signature is a favorable prognostic factor in acute myeloid leukemia. Leuk Res Rep. (2018) 9:9–13. doi: 10.1016/j.lrr.2017.12.001
95. Zöller M. CD44, hyaluronan, the hematopoietic stem cell, and leukemia-initiating cells. Front Immunol. (2015) 6. doi: 10.3389/fimmu.2015.00235
96. Izzi V, Lakkala J, Devarajan R, Ruotsalainen H, Savolainen ER, Koistinen P, et al. An extracellular matrix signature in leukemia precursor cells and acute myeloid leukemia. Haematologica. (2017) 102:e245–e8. doi: 10.3324/haematol.2017.167304
97. Bains AK, Behrens Wu L, Rivière J, Rother S, Magno V, Friedrichs J, et al. Bone marrow mesenchymal stromal cell-derived extracellular matrix displays altered glycosaminoglycan structure and impaired functionality in Myelodysplastic Syndromes. Front Oncol. (2022) 12. doi: 10.3389/fonc.2022.961473
98. Salazar-Terreros MJ, Vernot JP. In vitro and in vivo modeling of normal and leukemic bone marrow niches: cellular senescence contribution to leukemia induction and progression. Int J Mol Sci. (2022) 23:7350. doi: 10.3390/ijms23137350
99. Li W, Liang H, Ao Y, Tang B, Li J, Li N, et al. Biophysical cues of bone marrow-inspired scaffolds regulate hematopoiesis of hematopoietic stem and progenitor cells. Biomaterials. (2023) 298:122111. doi: 10.1016/j.biomaterials.2023.122111
100. Dainiak MB, Savina IN, Musolino I, Kumar A, Mattiasson B, Galaev IY. Biomimetic macroporous hydrogel scaffolds in a high-throughput screening format for cell-based assays. Biotechnol Prog. (2008) 24:1373–83. doi: 10.1002/btpr.30
101. Velliou EG, Dos Santos SB, Papathanasiou MM, Fuentes-Gari M, Misener R, Panoskaltsis N, et al. Towards unravelling the kinetics of an acute myeloid leukaemia model system under oxidative and starvation stress: a comparison between two- and three-dimensional cultures. Bioprocess Biosyst Eng. (2015) 38:1589–600. doi: 10.1007/s00449-015-1401-z
102. Bray LJ, Binner M, Körner Y, von Bonin M, Bornhäuser M, Werner C. A three-dimensional ex vivo tri-culture model mimics cell-cell interactions between acute myeloid leukemia and the vascular niche. Haematologica. (2017) 102:1215–26. doi: 10.3324/haematol.2016.157883
103. Matsunaga T, Fukai F, Miura S, Nakane Y, Owaki T, Kodama H, et al. Combination therapy of an anticancer drug with the FNIII14 peptide of fibronectin effectively overcomes cell adhesion-mediated drug resistance of acute myelogenous leukemia. Leukemia. (2008) 22:353–60. doi: 10.1038/sj.leu.2405017
104. Matsunaga T, Takemoto N, Sato T, Takimoto R, Tanaka I, Fujimi A, et al. Interaction between leukemic-cell VLA-4 and stromal fibronectin is a decisive factor for minimal residual disease of acute myelogenous leukemia. Nat Med. (2003) 9:1158–65. doi: 10.1038/nm909
105. Shen ZH, Zeng DF, Kong PY, Ma YY, Zhang X. AMD3100 and G-CSF disrupt the cross-talk between leukemia cells and the endosteal niche and enhance their sensitivity to chemotherapeutic drugs in biomimetic polystyrene scaffolds. Blood Cells Mol Dis. (2016) 59:16–24. doi: 10.1016/j.bcmd.2016.03.009
106. Tavor S, Petit I. Can inhibition of the SDF-1/CXCR4 axis eradicate acute leukemia? Semin Cancer Biol. (2010) 20:178–85. doi: 10.1016/j.semcancer.2010.07.001
107. Burger JA, Tsukada N, Burger M, Zvaifler NJ, Dell'Aquila M, Kipps TJ. Blood-derived nurse-like cells protect chronic lymphocytic leukemia B cells from spontaneous apoptosis through stromal cell-derived factor-1. Blood. (2000) 96:2655–63. doi: 10.1182/blood.V96.8.2655
108. Burger JA, Kipps TJ. CXCR4: a key receptor in the crosstalk between tumor cells and their microenvironment. Blood. (2006) 107:1761–7. doi: 10.1182/blood-2005-08-3182
109. Alhattab DM, Isaioglou I, Alshehri S, Khan ZN, Susapto HH, Li Y, et al. Fabrication of a three-dimensional bone marrow niche-like acute myeloid Leukemia disease model by an automated and controlled process using a robotic multicellular bioprinting system. Biomater Res. (2023) 27:111. doi: 10.1186/s40824-023-00457-9
110. Blanco TM, Mantalaris A, Bismarck A, Panoskaltsis N. The development of a three-dimensional scaffold for ex vivo biomimicry of human acute myeloid leukaemia. Biomaterials. (2010) 31:2243–51. doi: 10.1016/j.biomaterials.2009.11.094
111. Maharjan S, Ma C, Singh B, Kang H, Orive G, Yao J, et al. Advanced 3D imaging and organoid bioprinting for biomedical research and therapeutic applications. Adv Drug Delivery Rev. (2024) 115237. doi: 10.1016/j.addr.2024.115237
112. Zippel S, Dilger N, Chatterjee C, Raic A, Brenner-Weiß G, SChadzek P, et al. A parallelized, perfused 3D triculture model of leukemia for in vitro drug testing of chemotherapeutics. Biofabrication. (2022) 14:035011. doi: 10.1088/1758-5090/ac6a7e
113. Glaser DE, Curtis MB, Sariano PA, Rollins ZA, Shergill BS, Anand A, et al. Organ-on-a-chip model of vascularized human bone marrow niches. Biomaterials. (2022) 280:121245. doi: 10.1016/j.biomaterials.2021.121245
114. Sharipol A, Lesch ML, Soto CA, Frisch BJ. Bone marrow microenvironment-on-chip for culture of functional hematopoietic stem cells. Front Bioeng Biotechnol. (2022) 10. doi: 10.3389/fbioe.2022.855777
115. Cairns J, Leonard E, Khan K, Parks C, Maglennon G, Zhang B, et al. Optimal experimental design for efficient toxicity testing in microphysiological systems: A bone marrow application. Front Pharmacol. (2023) 14:1142581. doi: 10.3389/fphar.2023.1142581
116. Rödling L, Volz EM, Raic A, Brändle K, Franzreb M, Lee-Thedieck C. Magnetic macroporous hydrogels as a novel approach for perfused stem cell culture in 3D scaffolds via contactless motion control. Adv Healthc Mater. (2018) 7:1701403. doi: 10.1002/adhm.201701403
117. Carragher N, Piccinini F, Tesei A, Trask OJ Jr, Bickle M, Horvath P. Concerns, challenges and promises of high-content analysis of 3D cellular models. Nat Rev Drug Discov. (2018) 17:606–. doi: 10.1038/nrd.2018.99
118. Skopek R, Palusińska M, Kaczor-Keller K, Pingwara R, Papierniak-Wyglądała A, Schenk T, et al. Choosing the right cell line for acute myeloid leukemia (AML) research. Int J Mol Sci. (2023) 24:5377. doi: 10.3390/ijms24065377
119. Ackun-Farmmer MA, Soto CA, Lesch ML, Byun D, Yang L, Calvi LM, et al. Reduction of leukemic burden via bone-targeted nanoparticle delivery of an inhibitor of C-chemokine (C-C motif) ligand 3 (CCL3) signaling. FASEB J. (2021) 35:e21402. doi: 10.1096/fj.202000938rr
120. Tomasoni C, Arsuffi C, Donsante S, Corsi A, Riminucci M, Biondi A, et al. AML alters bone marrow stromal cell osteogenic commitment via Notch signaling. Front Immunol. (2023) 14:1320497. doi: 10.3389/fimmu.2023.1320497
121. Schroeder T, Geyh S, Germing U, Haas R. Mesenchymal stromal cells in myeloid Malignancies. Blood Res. (2016) 51:225–32. doi: 10.5045/br.2016.51.4.225
122. Shahrokh B, Allahbakhshian FM, Ahmad G, Fatemeh F, Hossein MM. AML-derived extracellular vesicles negatively regulate stem cell pool size: A step toward bone marrow failure. Curr Res Transl Med. (2023) 71:103375. doi: 10.1016/j.retram.2022.103375
123. Bairoch A. The cellosaurus, a cell-line knowledge resource. J Biomol Tech. (2018) 29(2):25. doi: 10.7171/jbt.18-2902-002
124. Oval J, Smedsrud M, Taetle R. Expression and regulation of the evi-1 gene in the human factor-dependent leukemia cell line, UCSD/AML1. Leukemia. (1992) 6(5):446–51.
125. Falini B, Brunetti L, Sportoletti P, Martelli MP. NPM1-mutated acute myeloid leukemia: from bench to bedside. Blood. (2020) 136:1707–21. doi: 10.1182/blood.2019004226
126. Siegel RL, Giaquinto AN, Jemal A. Cancer statistics, 2024. CA: A Cancer J Clin. (2024) 74:12–49. doi: 10.3322/caac.21820
127. Jackson SS, Marks MA, Katki HA, Cook MB, Hyun N, Freedman ND, et al. Sex disparities in the incidence of 21 cancer types: Quantification of the contribution of risk factors. Cancer. (2022) 128:3531–40. doi: 10.1002/cncr.34390
128. Klein SL, Flanagan KL. Sex differences in immune responses. Nat Rev Immunol. (2016) 16:626–38. doi: 10.1038/nri.2016.90
129. Lin S, Liu Y, Goldin LR, Lyu C, Kong X, Zhang Y, et al. Sex-related DNA methylation differences in B cell chronic lymphocytic leukemia. Biol Sex Differ. (2019) 10:2. doi: 10.1186/s13293-018-0213-7
130. Leon C, Manley E, Neely AM, Castillo J, Ramos Correa M, Velarde DA, et al. Lack of racial and ethnic diversity in lung cancer cell lines contributes to lung cancer health disparities. Front Oncol. (2023) 13. doi: 10.3389/fonc.2023.1187585
131. Eisfeld A-K. Disparities in acute myeloid leukemia treatments and outcomes. Curr Opin Hematol. (2024) 31:58–63. doi: 10.1097/MOH.0000000000000797
132. Bhatnagar B, Kohlschmidt J, Mrózek K, Zhao Q, Fisher JL, Nicolet D, et al. Poor survival and differential impact of genetic features of black patients with acute myeloid leukemia. Cancer Discovery. (2021) 11:626–37. doi: 10.1158/2159-8290.CD-20-1579
133. Hong J, Zhu Z, Cui L, Wang Z, Hao Y, Tian X, et al. Bone marrow-inspired hydrogel/graphene composite scaffolds to support in vitro expansion of hematopoietic stem cells. J Mater Chem B. (2024) 12:2354–63. doi: 10.1039/D3TB02448B
Keywords: acute myeloid leukemia, in vitro, bone marrow microenvironment, microphysiological systems, 3D culture, AML, MPS, BMME
Citation: Sharipol A and Frisch BJ (2024) Are we ready to integrate 3D culture systems in acute myeloid leukemia and bone marrow microenvironment research? Front. Hematol. 3:1407698. doi: 10.3389/frhem.2024.1407698
Received: 27 March 2024; Accepted: 02 July 2024;
Published: 06 August 2024.
Edited by:
Spiros Vlahopoulos, University of Athens, GreeceReviewed by:
Diana Passaro, INSERM U1016 Institut Cochin, FranceSyed A. Mian, The Francis Crick Institute, United Kingdom
Manja Wobus, Technical University Dresden, Germany
Copyright © 2024 Sharipol and Frisch. This is an open-access article distributed under the terms of the Creative Commons Attribution License (CC BY). The use, distribution or reproduction in other forums is permitted, provided the original author(s) and the copyright owner(s) are credited and that the original publication in this journal is cited, in accordance with accepted academic practice. No use, distribution or reproduction is permitted which does not comply with these terms.
*Correspondence: Benjamin J. Frisch, YmVuamFtaW5fZnJpc2NoMkB1cm1jLnJvY2hlc3Rlci5lZHU=