- Wolfson Wohl Cancer Research Centre, School of Cancer Sciences, University of Glasgow, Glasgow, United Kingdom
The importance of metabolism to cancer has increasingly been recognised and this is particularly the case for leukaemia. This has opened the possibility of targeting dysregulated metabolism with the aim of increasing the effectiveness of current therapies, some of which are anti-metabolites. One key challenge to be addressed is avoiding negative side effects due to shared metabolic dependencies between leukaemic and normal cells. This Mini Review will discuss how our understanding of wide-ranging effects of metabolism is continuing to evolve thanks to recent discoveries, as well as how metabolism can both directly and indirectly affect leukaemia cell functions. This includes introducing how metabolism is compartmentalised at levels ranging from organelle to whole body as well as how the metabolome can modify other ‘Omes.’ This Mini Review also places a focus on the overlay in metabolic demands of normal haematopoietic and immune cells. Finally, how therapies targeting metabolic processes have already delivered success, as well as the promise of new therapies targeting metabolism that are currently being investigated in clinical trials, will also be discussed.
Introduction
The word metabolism derives from the Greek word metabole “a change”1. Metabolism can be defined as the sum of life-sustaining chemical reactions in each cell of a living organism2. These reactions are needed for meeting critical processes such as generating and storing energy and synthesis of macromolecules such as DNA and proteins. Like other biological processes, there are differences between the metabolic pathways which are active in different cell types. The complexity of metabolism necessitates a certain level of reductionism, such as using in vitro models including cell lines. However, it is critical to consider metabolism at the level of the whole body when aiming to translate in vitro findings. For instance, while mitochondrial metabolism is critical to leukaemic cell function and therapy relapse/escape, translating therapies targeting this to the clinic has been difficult as normal cells and tissues may also depend on the same pathway.
Multifaceted roles of cancer metabolism: complexity and multi-layers
In the context of cancer, the first and most well-known example of dysregulated metabolism is a shift to glycolysis that was first described by Otto Warburg in 1923 (1). During this process tumours produce lactate (fermentation) from glucose even in the presence of oxygen. This fermentation yields less energy than would be obtained from the oxidisation of glucose in the mitochondria. However, clinical targeting of this phenotype has not been productive (2). The explosion in number of publications that contain terms ‘cancer’ & ‘metabolism’ is quite recent, with a large spike since the early 2000’s (Figure 1). While the link between immunity and metabolism has been of interest as early as the late 1800s when physicians recognised that metabolic pathologies were associated with infections, the functional requirement of metabolism for immune function/dysfunction in the field of ‘immunometabolism’ research is more recent (3) (Figure 1). Similarly, there also seems to be a recent increase in studies where the words ‘cancer,’ ‘immune’ and ‘metabolism’ co-appear, which is quite interesting given the importance of immunotherapy in treating leukaemia.
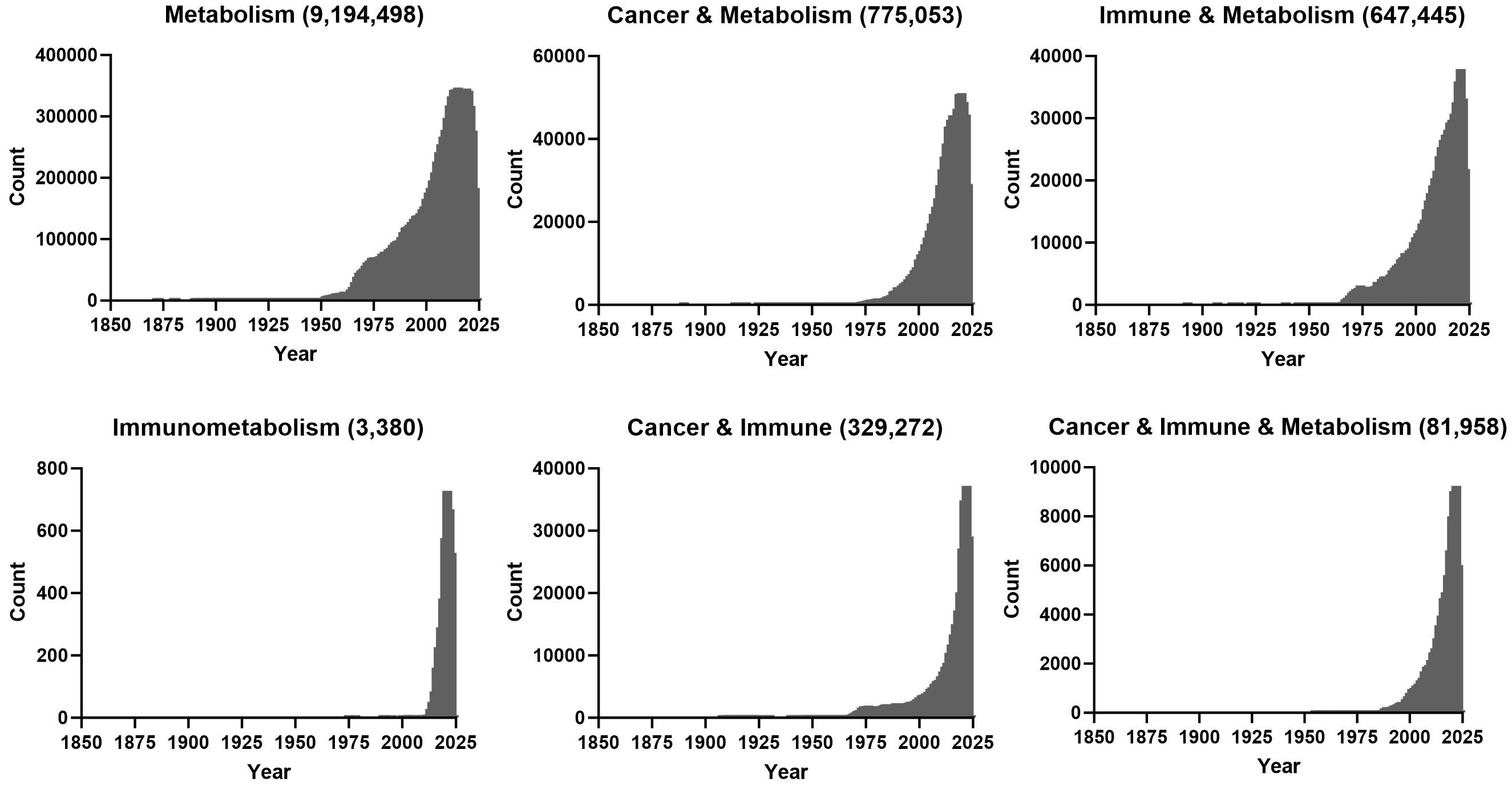
Figure 1 PubMed was queried with the indicated search terms on the 17th of October 2023 and number of publications for indicated years is plotted.
While glycolysis produces less energy (ATP) than oxidative phosphorylation/electron transport chain (OXPHOS/ETC) (Figure 2), it has been reported that the higher rate of energy production when factoring in volume (glycolytic enzymes in cytosol versus mitochondria) is required for maximal proliferation in cancer (4). Interestingly, Bartman et al. showed that the Warburg shift is present in primary solid tumours, but not in metastases or leukaemic cells that have higher OXPHOS flux and ATP production (5).
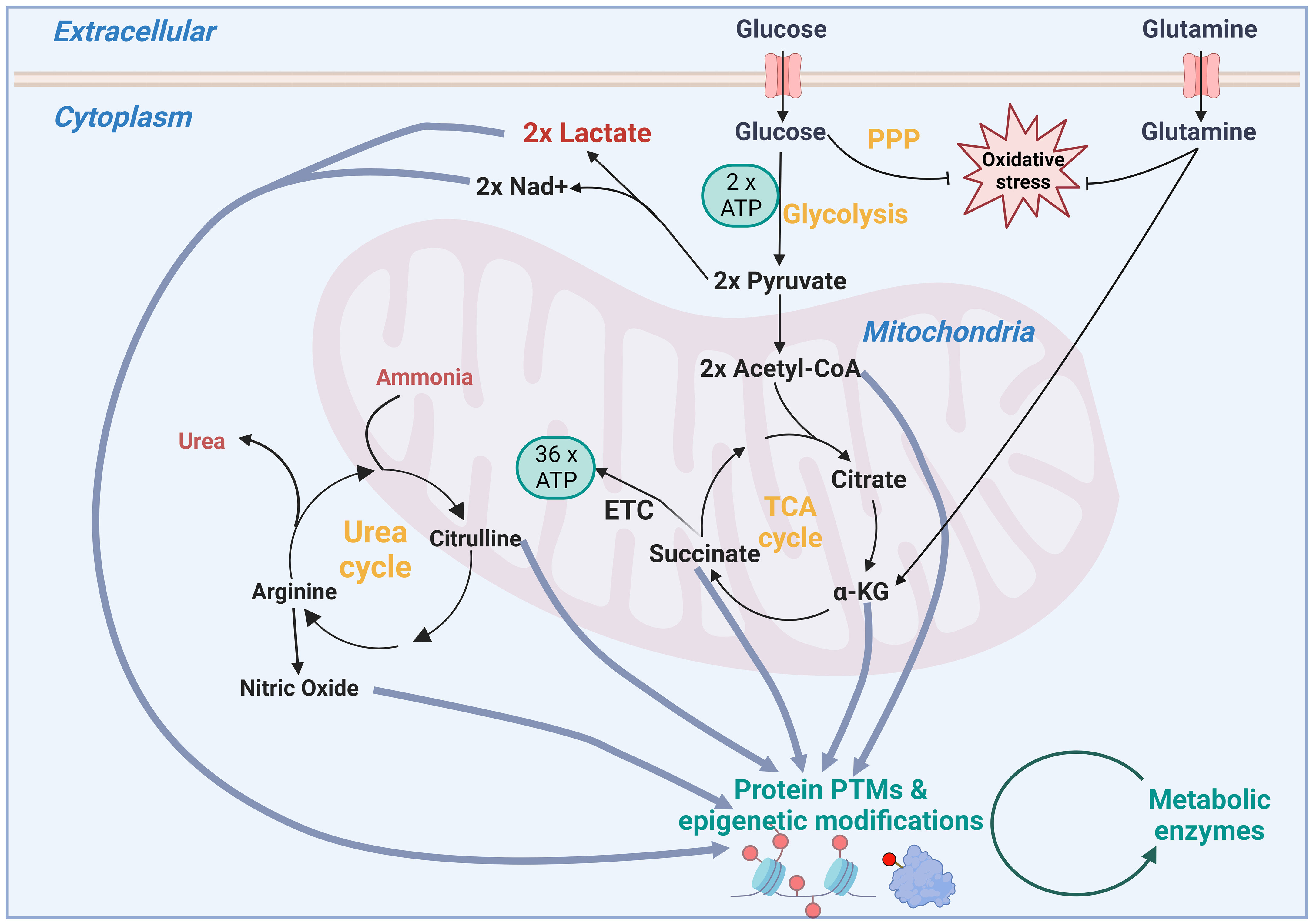
Figure 2 Schematic showing how inputs of metabolism not only fuel energy and redox metabolism, but how intermediates in these pathways can also modulate the epigenome and post translational modifications (PTMs). PPP, pentose phosphate pathway; TCA, tricarboxylic acid; ATP, adenosine triphosphate; Nad+, Nicotinamide adenine dinucleotide; ETC, electron transport chain.
New potential targets are being revealed by examining whether cancer cells differentially uptake either metabolic inputs or intermediates of biosynthesis pathways (2). These can be exploited using restricted diet or enzymes to deplete cancer cells of individual nutrients, as well as inhibitors that block their import into the cell or their relative metabolism pathway inside the cell. These vulnerabilities can be revealed by tracing the fate of nutrients. Examples of these nutrients include glucose, amino acids and fatty acids, some of which can be used for multiple purposes including fuelling the tricarboxylic acid (TCA) cycle, as well as controlling oxidative stress (Figure 2). In turn, the TCA cycle can be used to supply intermediates for modification of the epigenome, such as Acetyl CoA for acetylation and alpha-ketoglutarate for demethylation (6). It is important to note that cofactors such as NAD+, produced by metabolic enzymes such as lactate dehydrogenase, are required by sirtuins for deacetylation (7). This is just one example of energy production maintaining the necessary redox balance for regulatory and biosynthetic enzymes. Additionally, post-translational modifications using lactate (lactylation), succinate (succinylation), nitric oxide (nitrosylation), palmitate (palmitoylation) add to the potential for metabolic intermediates to modulate control of other proteins’ activity including metabolic proteins themselves (Figure 2). Adding to this complexity is the concept of moonlighting, which is where a metabolic enzyme can take a role beyond its main metabolic activity. For example, Ma et al. recently reported that elevated levels of glycolytic enzyme enolase 1 binds to choline kinase α to prevent its ubiquitin-dependent degradation, thereby promoting phosphatidylcholine production in multiple types of cancer including glioblastoma (8).
Finally, the role of compartmentalisation, both within the cell and at the whole-body level, gives additional control of cellular and whole-body metabolism, respectively. For instance, parts of mitochondrial metabolic pathways such as the TCA cycle and one carbon metabolism have corresponding cytoplasmic equivalents. These include the malate-aspartate and glycerol shuttles that can help maintain and balance cellular NAD+ levels. The lysosome is a critical organelle in supporting oncogenic growth, especially in nutrient limiting conditions (9). This organelle ensures the turnover of macromolecules from processes such as autophagy, serves as a reservoir for critical nutrients such as amino acids, lipids and ions, and is the location at where the mechanistic target of rapamycin complex 1 (mTORC1) is activated. Lysosomes have been implicated in normal and abnormal processes. For example, Liang et al. demonstrated that the maintenance of quiescence and repopulation potential of hematopoietic stem cells (HSCs) is due to elevated levels of large lysosomes. Conversely, targeting lysosomal biology and autophagy in leukaemic stem cells has shown promise in pre-clinical models of chronic myeloid leukaemia (CML) (10, 11). Further insight into how metabolism is organised has been gained with the discovery of multi-protein complexes such as purinosomes and mitochondrial respiratory super complexes (12, 13). Xu et al. recently discovered a further example of compartmentalisation where a phosphate-sensing organelle regulates phosphate levels (14). Given the critical role of phosphate in signalling (phospho-proteome) in haematopoiesis, immunity, and leukaemia, it will be interesting so see if this organelle is important in these contexts. At the highest level, animal models are invaluable in studying metabolic communication between organs with respect to whole body metabolism. For instance, Hui et al. showed that most of glucose enters the TCA cycle via circulating lactate (15). Given that metabolic therapies are often systemic and can reach multiple organs and tissues, the information of these studies is critical to determine whether effects of these therapies on leukaemic cells are direct or indirect. The latter could be useful when predicating non-specific toxicities.
Metabolic demands of leukaemic cells vs normal cells
Similar to hyper proliferation of leukaemic cells necessitating upregulation of multiple metabolic pathways, maintaining haematopoiesis and a functional immune system imposes high metabolic demands, some of which are context dependent (16). For example, there is increased normal haematopoiesis after myeloablative therapies or bone marrow transplants. Similarly, to resolve infection, rapid expansion of cells of the adaptive immune system or elevated antibody production is required. Here the metabolic requirements needed to facilitate proliferation and protein synthesis can overlap with leukaemic metabolism, including leukaemic stem cells that can drive therapy relapse. For example, while we have shown that mitochondrial pyruvate metabolism can be therapeutically targeted in CML stem cells (17), Ramstead et al. showed that pyruvate metabolism is required for T-cell homeostasis (18). The nexus of leukaemic and immune metabolism is critical when considering anti-cancer immune therapies that are increasingly being employed against leukaemia. While exploitation of arginine depletion is being tested against several cancers, including acute myeloid leukaemia (AML), Mussai et al. showed that arginine depletion impairs the effectiveness of Chimeric Antigen Receptor-T cell (CAR-T) therapy against AML blasts (19). Interestingly, Mussai et al. had previously shown that AML blasts secrete arginase that depleted arginine in a way identical to arginine depletion therapy (20). This in turn created an immunosuppressive microenvironment, highlighting the importance of metabolic competition between normal and leukaemic cells. While there may be overlap between metabolism in normal and leukaemic cells, it is important to note that this can be context dependent and there can still be therapeutic windows to exploit.
Within leukaemic cells, while mutations in isocitrate dehydrogenase 1 or 2 (IDH1 or 2) are a classic example of aberrant metabolism causing disease (21), there are other instances where dysregulated metabolism occurs downstream of oncogenes such as Abelson murine leukaemia (ABL) and fms like tyrosine kinase 3 (FLT3), the former being demonstrated to occur in leukaemic stem cells (22, 23). These oncogenes, which are sufficient to drive this metabolic reprogramming, can be targeted themselves with highly specific tyrosine kinase inhibitors (24, 25). Given the importance of oncoproteins to leukaemia initiation and progression, this begs the question whether in these instances if dysregulated metabolism is a driver of disease progression or therapy relapse or simply an incidental passenger. Therefore, it is important to not only evaluate the functional requirement of aberrant metabolism in leukaemia, but also to assess if it persists or is important in the presence of therapies targeting the relevant oncogene.
Metabolism in the clinic: successes in leukaemia
While targeting glycolysis or OXPHOS in cancer patients so far has not been successful, inhibitors that target other metabolic pathways are now commonly used to treat cancer patients. Prominent amongst these are anti-metabolites, chemicals that inhibit use of metabolites in normal metabolic pathways. This includes analogues of metabolites in pyrimidine, purine and folate metabolic pathways, which are needed for DNA synthesis and are widely used in treating leukaemia. It is worth noting that rapidly proliferating normal cells, including immune cells, are affected, hence low blood cell counts is a common side effect of treatment. As mentioned above, use of therapeutic enzymes to deplete amino acids is already being used (i.e., asparaginase) in the clinic or tested in clinical trials (arginase or arginine deiminase against leukaemia (2). These types of therapies are widely used against metabolic deficiencies or other non-cancer diseases with over 30 interventions approved by the European Medicines Agency (EMA) (26). Finally, IDH 1 and/or 2 mutant inhibitors ivosidenib (orphan status) and vorasidenib, are currently approved by both the US Food and Drug Administration and EMA as therapeutic options for IDH-mutated AML.
Future directions
The importance of metabolism for malignancies is now well accepted with dysregulating cancer listed as a key hallmark (27). While the anti-metabolite class of inhibitors is widely used in the clinic, their adverse effects on normal cells, including haematopoietic and immune cells leaves much to be desired. A fuller understanding of metabolism itself from the subcellular to whole body level is required to identify more selective targets for therapies, predict side effects as well as identifying new targets. One area of interest will be systematically identifying the substrates (proteins) for the lesser studied post-translational modifications and if any of these are relevant to leukaemia or solid tumours. Similarly, given the recent discovery of organelles such as purinosomes and phosphate-sensing organelles, it will be worth investigating if these impact existing therapies that target nucleotide and phosphorylation/dephosphorylation pathways, respectively. In the case where existing therapies have some efficacy, it will be important to ensure that metabolism that is dysregulated in patients upon diagnosis is relevant upon relapse. It would be interesting in the cases where frontline therapies are ineffective if metabolic pathway inhibition might reveal new vulnerabilities.
Author contributions
KR: Conceptualization, Funding acquisition, Investigation, Supervision, Visualization, Writing – original draft, Writing – review & editing.
Funding
The author declares financial support was received for the research, authorship, and/or publication of this article. KMR was supported by funding from the Medical Research Council grants 219390/Z/19/Z, MC_PC_19039, and MR/X502807/1.
Acknowledgments
The author thanks Eric R. Kalkman and G. Vignir Helgason for proofreading the manuscript. Figure 1 was prepared using GraphPad Prism (version10) and Figure 2 was produced using BioRender: agreement number TF261KRAG9.
Conflict of interest
The author declares that the research was conducted in the absence of any commercial or financial relationships that could be construed as a potential conflict of interest.
Publisher’s note
All claims expressed in this article are solely those of the authors and do not necessarily represent those of their affiliated organizations, or those of the publisher, the editors and the reviewers. Any product that may be evaluated in this article, or claim that may be made by its manufacturer, is not guaranteed or endorsed by the publisher.
Footnotes
References
1. Warburg O, Wind F, Negelein E. The metabolism of tumors in the body. J Gen Physiol (1927) 8:519–30. doi: 10.1085/jgp.8.6.519
2. Rattigan KM, Zarou MM, Helgason GV. Metabolism in stem cell–driven leukemia: parallels between hematopoiesis and immunity. Blood (2023) 141:2553–65. doi: 10.1182/blood.2022018258
3. Hotamisligil GS. Foundations of immunometabolism and implications for metabolic health and disease. Immunity (2017) 47:406–20. doi: 10.1016/j.immuni.2017.08.009
4. Salamanca-Cardona KRKL, Shah H, Poot AJ, Correa FM, Gialleonardo V, Lui H, et al. Limits of aerobic metabolism in cancer cells. Sci Rep (2017) 551:1336–47. doi: 10.1038/s41598-017-14071-y
5. Bartman CR, Weilandt DR, Shen Y, Lee WD, Han Y, TeSlaa T, et al. Slow TCA flux and ATP production in primary solid tumours but not metastases. Nature (2023) 614:349–57. doi: 10.1038/s41586-022-05661-6
6. Intlekofer AM, Finley LWS. Metabolic signatures of cancer cells and stem cells. Nat Metab (2019) 1:177–88. doi: 10.1038/s42255-019-0032-0
7. Hallows WC, Lee S, Denu JM. Sirtuins deacetylate and activate mammalian acetyl-CoA synthetases. Proc Natl Acad Sci (2006) 103:10230–5. doi: 10.1073/pnas.0604392103
8. Ma Q, Jiang H, Ma L, Zhao G, Xu Q, Guo D, et al. The moonlighting function of glycolytic enzyme enolase-1 promotes choline phospholipid metabolism and tumor cell proliferation. Proc Natl Acad Sci (2023) 120:e2209435120. doi: 10.1073/pnas.2209435120
9. Settembre C, Perera RM. Lysosomes as coordinators of cellular catabolism, metabolic signalling and organ physiology. Nat Rev Mol Cell Biol 1–23 (2023). doi: 10.1038/s41580-023-00676-x
10. Baquero P, Dawson A, Mukhopadhyay A, Kuntz EM, Mitchell R, Olivares O, et al. Targeting quiescent leukemic stem cells using second generation autophagy inhibitors. Leukaemia (2019) 33:981–94. doi: 10.1038/s41375-018-0252-4
11. Ianniciello A, Zarou MM, Rattigan KM, Scott M, Dawson A, Dunn K, et al. ULK1 inhibition promotes oxidative stress–induced differentiation and sensitizes leukemic stem cells to targeted therapy. Sci Trans Med (2021) 13:5016. doi: 10.1126/scitranslmed.abd5016
12. An S, Kumar R, Sheets ED, Benkovic SJ. Reversible compartmentalization of de novo purine biosynthetic complexes in living cells. Science (2008) 320:103–6. doi: 10.1126/science.1152241
13. Schägger H, Pfeiffer K. Supercomplexes in the respiratory chains of yeast and mammalian mitochondria. EMBO J (2000) 19:1777–83. doi: 10.1093/emboj/19.8.1777
14. Xu C, Xu J, Tang H-W, Ericsson M, Weng J-H, DiRusso J, et al. A phosphate-sensing organelle regulates phosphate and tissue homeostasis. Nature (2023) 617:798–806. doi: 10.1038/s41586-023-06039-y
15. Hui S, Ghergurovich JM, Morscher RJ, Jang C, Teng X, Lu W, et al. Glucose feeds the TCA cycle via circulating lactate. Nature (2017) 551–115–8. doi: 10.1038/nature24057
16. Bantug GR, Galluzzi L, Kroemer G, Hess C. The spectrum of T cell metabolism in health and disease. Nat Rev Immunol (2018) 18:19–34. doi: 10.1038/nri.2017.99
17. Rattigan KM, Brabcova Z, Sarnello D, Zarou MM, Roy K, Kwan R, et al. Pyruvate anaplerosis is a targetable vulnerability in persistent leukaemic stem cells. Nat Commun (2023) 14:4634. doi: 10.1038/s41467-023-40222-z
18. Ramstead AG, Wallace JA, Lee S-H, Bauer KM, Tang WW, Ekiz HA, et al. Mitochondrial pyruvate carrier 1 promotes peripheral T cell homeostasis through metabolic regulation of thymic development. Cell Rep (2020) 30:2889–2899.e6. doi: 10.1016/j.celrep.2020.02.042
19. Mussai F, Wheat R, Sarrou E, Booth S, Stavrou V, Fultang L, et al. Targeting the arginine metabolic brake enhances immunotherapy for leukaemia. Int J Cancer (2018) 145–2201–8. doi: 10.1002/ijc.32028
20. Mussai F, Santo CD, Abu-Dayyeh I, Booth S, Quek L, Mcewen-Smith RM, et al. Acute myeloid leukaemia creates an arginase-dependent immunosuppressive microenvironment. Blood (2013) 122:749–58. doi: 10.1182/blood-2013-01-480129
21. Chou W-C, Hou H-A, Chen C-Y, Tang J-L, Yao M, Tsay W, et al. Distinct clinical and biologic characteristics in adult acute myeloid leukaemia bearing the isocitrate dehydrogenase 1 mutation. Blood (2010) 115:2749–54. doi: 10.1182/blood-2009-11-253070
22. Kuntz EM, Baquero P, Michie AM, Dunn K, Tardito S, Holyoake TL, et al. Targeting mitochondrial oxidative phosphorylation eradicates therapy-resistant chronic myeloid leukemia stem cells. Nat Med (2017) 23:1234–40. doi: 10.1038/nm.4399
23. Gallipoli P, Giotopoulos G, Tzelepis K, Costa ASH, Vohra S, Medina-Perez P, et al. Glutaminolysis is a metabolic dependency in FLT3ITDacute myeloid leukaemia unmasked by FLT3 tyrosine kinase inhibition. Blood (2018) 131(15):1639–53. doi: 10.1182/blood-2017-12-820035
24. Druker BJ, Tamura S, Buchdunger E, Ohno S, Segal GM, Fanning S, et al. Effects of a selective inhibitor of the Abl tyrosine kinase on the growth of Bcr–Abl positive cells. Nat Med (1996) 2:561–6. doi: 10.1038/nm0596-561
25. Weisberg E, Boulton C, Kelly LM, Manley P, Fabbro D, Meyer T, et al. Inhibition of mutant FLT3 receptors in leukemia cells by the small molecule tyrosine kinase inhibitor PKC412. Cancer Cell (2002) 1:433–43. doi: 10.1016/S1535-6108(02)00069-7
26. de la Fuente M, Lombardero L, Gómez-González A, Solari C, Angulo-Barturen I, Acera A, et al. Enzyme therapy: current challenges and future perspectives. Int J Mol Sci. (2021) 22:9181. doi: 10.3390/ijms22179181
Keywords: metabolism, leukaemia, haematopoiesis, immunity, stem cell (SC)
Citation: Rattigan KM (2024) Mini review of metabolism in leukaemia: from complexity to the clinic. Front. Hematol. 2:1353994. doi: 10.3389/frhem.2023.1353994
Received: 11 December 2023; Accepted: 26 December 2023;
Published: 11 January 2024.
Edited by:
Marcus O. Muench, Vitalant Research Institute, United StatesReviewed by:
Pilar Giraldo, University of Zaragoza, SpainCopyright © 2024 Rattigan. This is an open-access article distributed under the terms of the Creative Commons Attribution License (CC BY). The use, distribution or reproduction in other forums is permitted, provided the original author(s) and the copyright owner(s) are credited and that the original publication in this journal is cited, in accordance with accepted academic practice. No use, distribution or reproduction is permitted which does not comply with these terms.
*Correspondence: Kevin M. Rattigan, S2V2aW4uUmF0dGlnYW5AZ2xhc2dvdy5hYy51aw==