- 1Neuroscience Program, Michigan State University, East Lansing, MI, United States
- 2School of Biology and Undergraduate Neuroscience Program, Georgia Institute of Technology, Atlanta, GA, United States
- 3Behavioral Neuroscience Program, Department of Psychology, Michigan State University, East Lansing, MI, United States
- 4Department of Neuroscience, Tufts University School of Medicine, Boston, MA, United States
Upregulation of the inhibitory neurotransmitter, GABA, is involved in many of the behavioral differences between postpartum and nulliparous female rodents. This is evidenced by studies showing that pharmacological blockade of GABAergic activity impairs maternal caregiving and postpartum affective behaviors. However, the influence of motherhood on the capacity for GABA synthesis or release in the medial prefrontal cortex (mPFC; brain region involved in many social and affective behaviors) is not well-understood. Western blotting was used to compare postpartum and nulliparous rats in protein levels of the 65-kD isoform of glutamic acid decarboxylase (GAD65; synthesizes most GABA released from terminals) and vesicular GABA transporter (vGAT; accumulates GABA into synaptic vesicles for release) in the mPFC. We found that postpartum mothers had higher GAD65 and vGAT compared to virgins, but such differences were not found between maternally sensitized and non-sensitized virgins, indicating that reproduction rather than just the display of maternal caregiving is required. To test whether GAD65 and vGAT levels in the mPFC were more specifically related to anxiety-related behavior within postpartum mothers, we selected 8 low-anxiety and 8 high-anxiety dams based on their time spent in the open arms of an elevated plus maze on postpartum day 7. There were no significant differences between the anxiety groups in either GAD65 or vGAT levels. These data further indicate that frontal cortical GABA is affected by female reproduction and more likely contributes to differences in the display of socioemotional behaviors across, but not within, female reproductive state.
Introduction
The early postpartum period is associated with a number of salient behavioral modifications including elevated interest in neonates, the performance of caregiving activities, and a suppression of anxiety- and fear-related behaviors that facilitates mothers' positive focus on the offspring [for reviews see (1, 2)]. The neurochemical underpinnings of these postpartum behaviors include numerous steroids, neuropeptides, and classical neurotransmitters (1, 3, 4). Of the latter, a role for the inhibitory neurotransmitter GABA has received considerable attention. For instance, postpartum rats have higher basal GABA release and turnover in a number of brain areas regulating postpartum behaviors (5, 6). Furthermore, the postpartum GABA system is acutely affected by interactions with offspring, such that cerebrospinal fluid concentrations of GABA are high when mother rats interact with pups, but drop to almost non-detectable levels within hours after the litter is removed (7).
Such changes in GABAergic activity are functionally relevant for mothers' behavior. Peripheral administration of GABAA receptor (GABAAR) agonists or antagonists interfere with most postpartum behaviors, including dams' maternal caregiving and their characteristically low anxiety-related behavior (8–14). Furthermore, experiments targeting specific forebrain sites have identified the mPFC as a locus for GABA's involvement in both maternal caregiving and postpartum affective behaviors (8, 14, 15).
Studies exploring differences between parous and nulliparous female laboratory rodents in their central GABAA or benzodiazepine receptor binding have yielded negative results (16–19). This suggests that it would be more fruitful to delve into the presynaptic mechanisms underlying changes across female reproduction in central GABA synthesis and release, including glutamate decarboxylase (GAD65) and the vesicular GABA transporter (vGAT), which we focus on in the present experiments.
Glutamate is decarboxylated into GABA either by GAD65 or the larger GAD67 isoform, but unlike GAD67 which is found throughout the cytosol and generates the basal GABA necessary for cellular metabolic function, GAD65 is predominantly found in neuronal terminals where it synthesizes most of the GABA packaged into synaptic vesicles (20–23). Therefore, GAD65 is the primary form of GAD necessary for the GABA that is involved in phasic synaptic inhibition (24–26) as well as for the tonic extrasynaptic inhibition occurring from ambient GABA in the extracellular space (27–29). GAD expression is already known to be affected by reproductive state and maternal interactions with pups in at least three forebrain sites—the olfactory bulb, bed nucleus of the accessory olfactory tract, and rostral lateral septum (30–32)—but other sites implicated in postpartum behaviors have not been examined. The other GABA system protein of interest in the present study is vGAT, which is tightly coupled to GAD65 and packages newly synthesized GABA into vesicles for its release at the synapse (23, 33). Although the importance of vGAT for synaptic GABA release is evident by the reduction in inhibitory postsynaptic currents (IPSCs) in brain tissue from vGAT knockout mice (34), the influence of motherhood on vGAT anywhere in the brain is unknown.
An important consideration when investigating neurochemical changes across female reproduction is that nulliparous and parous females not only differ in their endocrine experiences, but also in their behavioral experience. To help distinguish between the neurobiology of female reproductive state and female postpartum behavior, some studies utilize the maternal virgin (or maternally sensitized) model of caregiving (35). Most nulliparous female laboratory rats are not spontaneously maternal but instead find pups aversive. These females can be induced to show maternal behavior, though, by repeatedly exposing them to young pups. Sensitized maternal behavior does not require hormones, and is similar to postpartum caregiving in that it includes nest building, retrieval and licking the pups, nursing-like postures over the litter, and reduced fear and anxiety-like behaviors (8, 36–40). Comparing maternally sensitized virgins to non-sensitized nulliparous females allows insight into the neural basis of maternal socioemotional behaviors without the involvement of pregnancy, parturition, and lactation.
In our first experiment, we compared GAD65 and vGAT levels in the mPFC of postpartum day 7 and virgin females. In a second experiment, we then used the maternal sensitization model to examine levels of GAD65 and vGAT in a group of ovariectomized, nulliparous rats permitted 1 week of maternal experience and in a group of unexposed nulliparous controls. Lastly, to determine whether the capacity for GABA synthesis and release in the mPFC is associated with individual differences in anxiety-related behaviors within postpartum females (41), we measured GAD65 and vGAT in postpartum females that were selected for their relatively high-anxiety or relatively low-anxiety behavior. The results of the three experiments collectively suggest that female reproductive state, but not maternal caregiving alone or degree of postpartum anxiety, is associated with levels of GAD65 and vGAT in the mPFC.
Methods
Subjects
Adult female Long-Evans rats born and raised in our colony were maintained under a 12:12 h light/dark cycle, constant temperature and humidity, and food and water ad libitum as described previously (41). Beginning at 70 days of age, estrous cycles of females intended for the postpartum groups were monitored using a vaginal impedance meter (Fine Science Tools, Foster City, CA), and on a day of proestrus these females were placed in the cage of a sexually experienced Long-Evans male rat for 48 hr. Females were then rehoused with 1 or 2 other mated females for the next 16 days, then singly housed until sacrifice 7 days after parturition (n = 15). Litters were culled to mixed-sex groups of 8 pups within 24 h after parturition. Another group of virgin females were not mated, but vaginally smeared daily and sacrificed on a day of diestrus (n = 15). Diestrus rats were chosen to compare to the day 7 postpartum rats because these groups are behaviorally very different, but are both in a state of diestrus involving low circulating estradiol and moderate levels of circulating progesterone (42–44). Comparing these groups allowed us to infer that any differences in GAD65 or vGAT protein levels would probably not be due to females' ovarian state at sacrifice, but instead other differences between groups in their physiology and behavior.
We further teased apart the behavioral and endocrine influences on central GAD65 and vGAT levels by comparing maternally sensitized virgin rats (n = 12) to a group of non-sensitized virgin controls (n = 11). All subjects were anesthetized with ketamine and xylazine and ovariectomized to ensure that ovarian hormones could not be responsible for differences between groups. Beginning a week after surgery, and using methods similar to our previous studies (36, 45), the to-be-sensitized subjects were exposed in their home cage to three freshly fed 1–7 day old male and female pups obtained from surrogate dams in our colony. Pups were placed in the corner of the cages opposite the subjects' nests. For the next 15 min, females' pup-directed behaviors (sniffing or retrieving each of the three pups, licking the pups, huddling over the pups) were scored by an observer as present or not present. Subjects reached “full maternal behavior” by retrieving all three pups to a single location, licking them, and huddling over them on 2 consecutive test days (46). Each morning the foster pups from the previous day were removed from the subjects' cages, and it was recorded if the pups were warm, to help confirm subjects' maternal or non-maternal state. Those pups were placed back with surrogate dams for at least 2 days to ensure they were well fed before being used again. To better be able to compare the sensitized females in this experiment to the day 7 postpartum females studied in the first experiment, the sensitized females showing full maternal behavior were given an additional 5 days of experience with pups to reach 7 full days of caregiving. This was achieved by continuing to place three freshly-fed pups in the sensitized females' cages each day and observing the subjects for 15 min daily to verify the continuance of their full maternal behavior. The control females never received pups in their cages.
In the final experiment, 40 females from our colony were screened for anxiety-related behavior in an elevated plus maze on postpartum day 7 [see (41) for details]. Briefly, time spent in and frequency of entries into the open and closed arms of an elevated plus maze were recorded during 10-min tests. Subjects were immediately sacrificed after their behavioral test and the 8 highest-anxiety and 8 lowest-anxiety females based on their percentage of time spent in the open arms of the plus maze were selected for brain analysis. All procedures were conducted in compliance with the Institutional Animal Care and Use Committee (IACUC) at Michigan State University.
Tissue Preparation
Animals were rendered unconscious by brief exposure to CO2 and decapitated. Brains were removed, frozen with ice cold isopentane, and stored at −80°C until further processing. Brains were later coronally sectioned with a cryostat (LEICA CM 1950, Nussloch, Germany) into 500 μm-thick sections containing the medial prefrontal cortex (mPFC; ~ +3.2 to 2.2 mm from bregma, plates 8–10 from Swanson's atlas) (47). Tissue punches were made bilaterally using a 1.0 mm-diameter stainless steel brain punch (Stoelting CO # 57397, Wood Dale, IL) and included the prelimbic and infralimbic subregions (Figure 1). The punches were placed in centrifuge tubes containing 50 μl of RIPA buffer that consisted of lysis buffer, protease inhibitor cocktail, sodium orthovanadate, and 10 mg/ml PMSF reagent (Santa Cruz SC-24948, Santa Cruz, CA). Punches were homogenized using a sonic dismembrator (Fisher Scientific, Pittsburgh, PA), centrifuged for 15 min at 4°C at 15,000 rpm, and the supernatants collected. Samples were frozen and stored at −80°C until Western blotting.
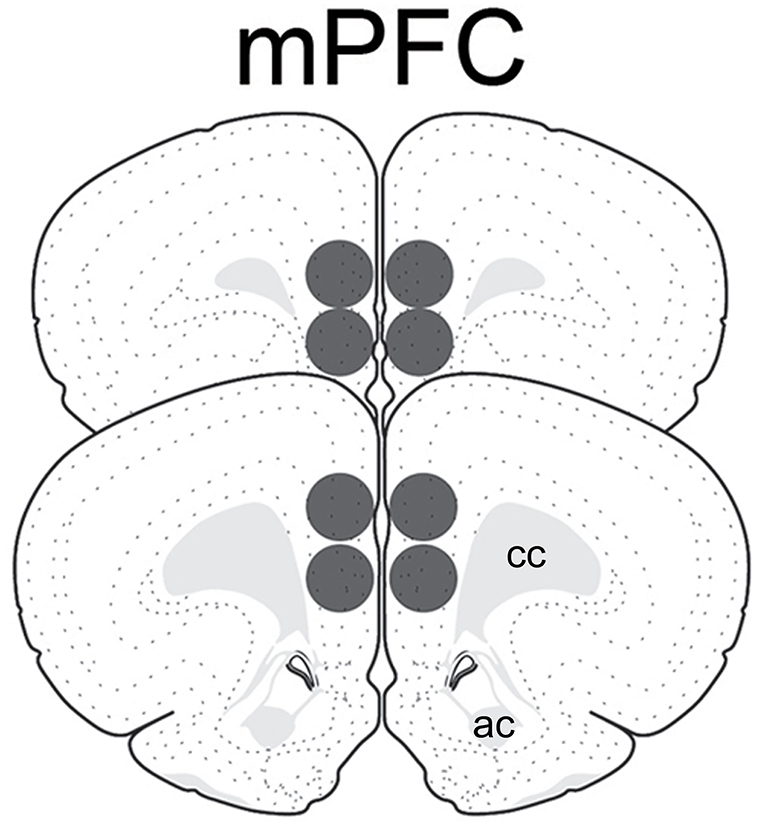
Figure 1. Schematic representation of tissue punches within the medial prefrontal cortex analyzed for GAD65 and vGAT. Images modified from Swanson (47). ac, anterior commissure; cc, corpus callosum.
Western Blotting
Protein concentrations were determined using BCA protein assay kits (Thermo Scientific Pro #23227 Rockford, IL) and a microplate reader (Bio-Rad iMark, Hercules, CA). Samples were immunoblotted for GAD65, vGAT, and then GAPDH as the loading control. Ten micrograms of total protein from each sample were denatured at 95°C for 5 min and gel electrophoresed on 10% Tris-Glycine precast gels (NB10-010; Nusep; Bogart, GA). Each gel contained samples from both groups within each of the three experiments (parous/nulliparous; sensitized/unsensitized; high anxiety/low anxiety). Proteins were transferred to polyvinylidene difluoride membranes using an iBlot system (Invitrogen # iB4010, Grand Island, NY). The membranes were washed three times for 10 min each in TBS-T (TBS containing 0.05% Tween-20) and blocked with 5% non-fat dry milk in TBS-T for 1 h at room temperature to reduce non-specific binding. The membranes were then incubated in a rabbit anti-vGAT primary antiserum (1:1,500; Millipore #AB2257, Temecula, CA) in TBS-T and 0.02% sodium azide overnight at 4°C. Following that incubation, membranes were washed three times for 10 min each in TBS-T, incubated in a peroxidase-conjugated anti-rabbit IgG secondary antiserum (1:5,000; Cell Signaling #7074, St. Louis, MO) for 1 h at room temperature, and rinsed in TBS three times. Detection of immunoreactive bands was accomplished using enhanced Luminol chemiluminescence kits (Santa Cruz SC-2048, Santa Cruz, CA), after which the membranes were exposed to films (Optimum Blue Sensitive film, XR-0810-100, Life Science Products, Frederick, CO) that were developed and fixed (Kodak X-OMAT 1000A, Rochester, NY).
Following immunoblotting for vGAT, the membranes were stripped and reblotted for GAD65. Membranes were rinsed twice for 10 min each time in TBS-T and incubated in stripping buffer (Thermo Scientific #46430, Rockford, IL) for 15 min. After stripping and rinsing four times, blotting was performed as described above but with a rabbit anti-GAD65 antiserum (1:2,000; Cell Signaling # 5843S) overnight at 4°C followed by incubation in a peroxidase-conjugated anti-rabbit IgG secondary antiserum (1:5,000; Cell Signaling #7074) for 1 hr at room temperature. Immunoreactive bands were again detected using chemiluminescence kits and exposed to film as described above. Membranes were then stripped a second time and reprobed as described above using an overnight incubation at 4°C in a mouse anti-GAPDH antiserum (1:500; Millipore #MAB374, Billerica, MA) followed by a peroxidase-conjugated rabbit anti-mouse secondary antiserum (1:80,000; Sigma-Aldrich #A9044, St. Louis, MO) for 1 h at room temperature. Immunoreactive bands were detected and membranes exposed to film as described above.
Image and Data Analysis
A single, dense immunoreactive band at the expected molecular weight was found after blotting with each antiserum (Figure 2). Films were placed on a light box and digital images captured with a Nikon E400 microscope and camera. Image J (NIH, Bethesda, MD) was used to determine the integrated density of the immunoreactive band for each subject's GAD65 and vGAT. As very commonly done with Western blot data, a ratio between the optical density of the bands for each of the two proteins of interest and the optical density of their band for GAPDH was created for each subject (48). Because the GAD65 ratio data from the first experiment comparing postpartum dams to nulliparous females failed Levene's test of homogeneity of variance (p = 0.015), these data were analyzed with a non-parametric Mann-Whitney U-test. The other comparisons were performed with independent t-tests. One pup-exposed nulliparous rat in experiment 2 did not reach the criteria for full maternal behavior, so was removed from the study. vGAT was not detected on the blot of one female from the low-anxiety group and she was omitted from the vGAT analyses in experiment 3. Furthermore, GAD65 data in the high- and low-anxiety comparison study were not normally-distributed and one outlier from the high-anxiety group was detected via a Grubb's test (p < 0.05). Data were normally-distributed after this outlier was removed from the analyses. The null hypotheses for all analyses were that the two groups being compared (parous/nulliparous; sensitized/unsensitized; high anxiety/low anxiety) would not differ in their mean vGAT or GAD65 levels. Effects sizes for all analyses were calculated with Cohen's d. Statistical analyses were conducted using IBM SPSS Version 26 software. Statistical significance was indicated by p < 0.05.
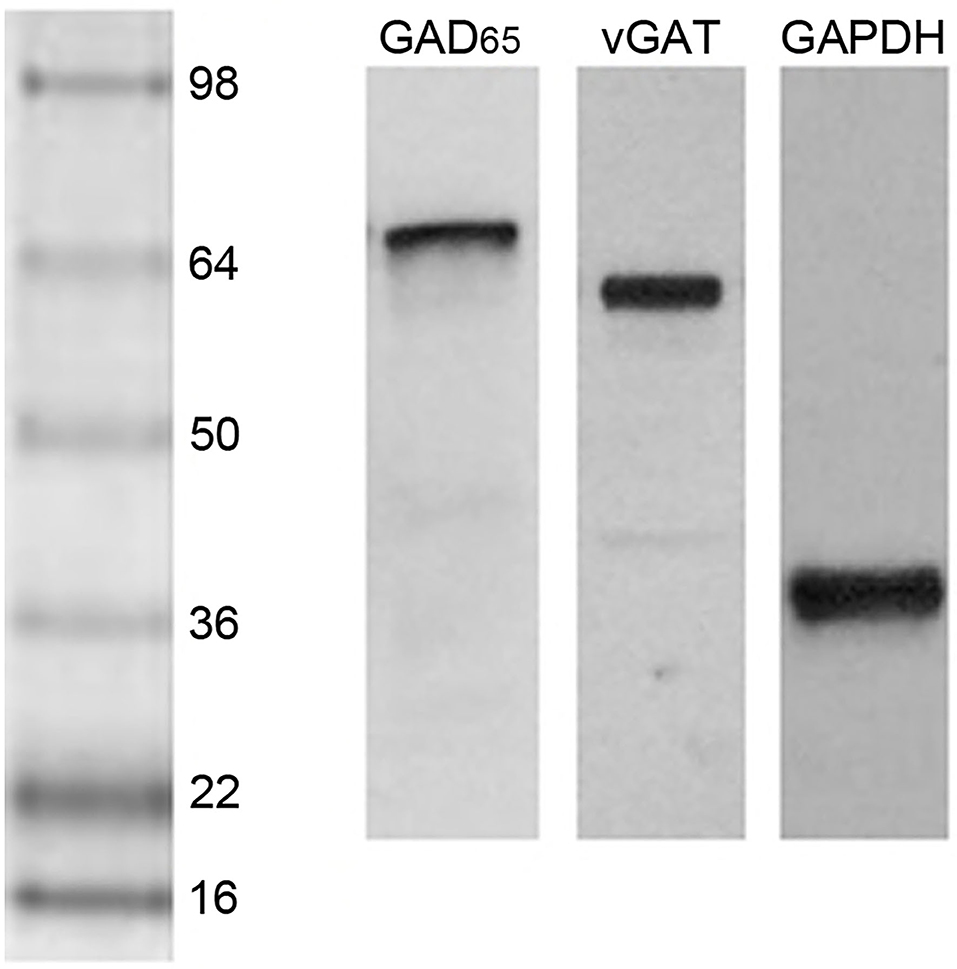
Figure 2. Western blot lanes containing the mPFC of representative postpartum female rats and probed for GAD65, vGAT, and GAPDH (left to right). The protein ladder is shown on the left side.
Results
Nulliparous and parous females differed in their median mPFC levels of GAD65 (Mdn = 1.28, IQR = 0.38 vs. Mdn = 1.53, IQR = 0.78, respectively; U30 = 54, p = 0.02, d = 0.99) and mean mPFC levels of vGAT (M = 0.81, SE = 0.06 vs. M = 1.03, SE = 0.08, respectively; t29 = 2.15, p = 0.04, d = 0.79). Parous females had higher levels of both proteins (Figure 3).
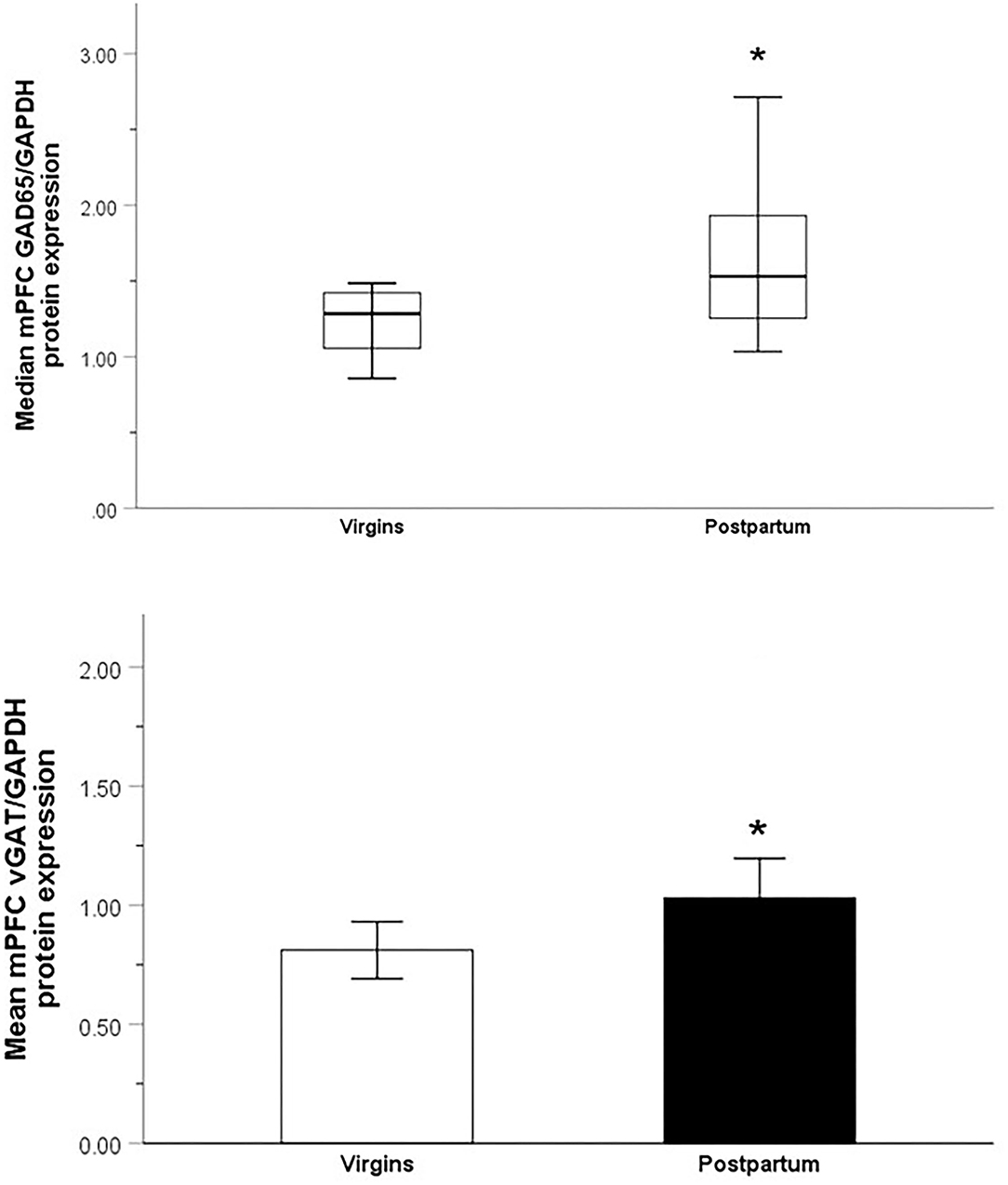
Figure 3. Relative optical density of GAD65-immunoreactive bands (top; Median ± IQR) and vGAT-immunoreactive bands (bottom; Mean ± SEM) from the mPFC of nulliparous virgin and postpartum rats. *p < 0.05.
The maternally-sensitized nulliparous females took a median of 4 days (mean = 4.8, range = 3–8 days) from the start of pup exposure to show the 2 days of pup responsiveness to reach the criteria for full maternal behavior. They then received an additional 5 days of experience with pups (total 7 days) in order to match the maternal experience of the dams in the first experiment. Despite this extensive experience with pups, sensitized and unexposed control females did not differ in their mean levels of GAD65 [(M = 0.16, SE = 0.001 vs. M = 0.18, SE = 0.12, respectively; t21 = 1.11, p = 0.28, d = 0.46, 95% CI (−0.17, 0.06)], or vGAT [(M = 0.55, SE = 0.65 vs. M = 0.66, SE = 0.90, respectively; t21 = 1.10, p = 0.28, d = 0.46, CI (−0.12, 0.34)], in the mPFC.
As could be expected, the low-anxiety dams from this sample spent 23 ± 3% of their time in open arms of the elevated plus maze whereas the high-anxiety dams spent 6 ± 1% [t13 = 5.24, p < 0.001, d = 2.18]. However, the low-anxiety dams and high-anxiety dams did not differ in their mean GAD65 [M = 28.17, SE = 2.65 vs. M = 24.94, SE = 1.58, respectively; t13 = 1.01, p = 0.33, d = 0.52 (CI −3.70, 10.16)], or mean vGAT (M = 27.64, SE = 5.72 vs. M = 36.89, SE = 8.54, respectively; t13 = 0.92, p = 0.37, d = 0.48, (CI −30.95, 12.46)], levels in the mPFC. There were also no significant correlations between the dams' percentage of time spent in the open arms and their levels of GAD65 [r15 = 0.49, p = 0.09 (CI −0.001, 0.017)] or vGAT [r15 = −0.36, p = 0.23, (CI −0.005, 0.001)] in the mPFC.
Discussion
Postpartum and nulliparous female laboratory rodents differ in numerous aspects of their central GABA system. GABA concentrations in cerebrospinal fluid, and GABA release or metabolism in a number of forebrain sites (e.g., mPFC, mPOA, and BST), are higher in postpartum mothers compared to non-mothers and decrease when the offspring are removed (5–7, 49). Furthermore, GAD levels in the olfactory bulb, bed nucleus of the accessory olfactory tract, and lateral septum are higher in postpartum rats compared to nulliparous females (30–32). The first goal of the present experiments was to expand upon these findings by comparing nulliparous and parous rats in their protein levels of GAD65 (the isoform found in neuronal terminals and necessary for synthesizing GABA for synaptic release) and vGAT (transports GABA into synaptic vesicles) in the mPFC. We found that postpartum female rats had higher levels of both proteins, suggesting that these mothers have increased capacity for GABA synthesis and release. This is consistent with earlier work showing that basal extracellular GABA and turnover are higher in the mPFC of postpartum rats compared to that of cycling virgins (5, 6).
One consequence of elevated GABA synthesis, packaging and release in the postpartum mPFC may be greater high-intensity phasic synaptic inhibition, which requires high GABA production and release. In contrast, the tonic GABA inhibition mediated by high-affinity extrasynaptic GABAARs occurs under relatively low ambient GABA concentrations (50). Elevated GABA-mediated phasic inhibition in the postpartum mPFC could be involved in a number of behavioral or physiological changes occurring at this time of female reproduction. Related to behavior, the mPFC has widespread cortical and subcortical connections (51, 52) through which it evaluates an array of internal and external signals to bias the processing of competing neural inputs; it thus act as a higher-order, experience-informed regulator of behavioral responding (53, 54). This includes the execution or inhibition of goal-directed and affective behaviors, such as those saliently modified during the postpartum period. In support of a role in caregiving behaviors, the postpartum rat mPFC responds electroencephalographically to nest odors (55) and electrophysiologically to snout contact with offspring (56). Lesioning or chemically inactivating the postpartum mPFC impairs pup retrieval and other maternal behaviors (15, 57, 58). Most GABAergic neurons in the mPFC are interneurons modulating the activity of projection cells (59). However, there must be limits to any benefit of mPFC disinhibition for maternal behaviors because: (1) as indicated just above, additional postpartum inhibition of the mPFC by GABAAR agonism or anesthetic-based deactivation impairs pup-directed behaviors (15, 58) and (2) high impulsivity that can result from low mPFC top-down control is associated with poor mothering in female rats (60).
Interpreting the functional significance of our first set of findings comparing across female reproductive state is complicated by the fact that parous females are not only behaviorally different from nulliparae but also differ from them physiologically. For instance, the maternal brain must coordinate numerous physiological adaptations including blunting of the HPA axis, the neuroendocrinological control of milk production and milk letdown, and changes in energy balance (61–65). To help us understand if the changes in GAD65 and vGAT in the first experiment were more due to behavioral or physiological adaptations, we then compared groups of virgin ovariectomized female rats that received a maternal sensitization procedure, and then allowed up to a week of caregiving experience with pups to match the experience of the postpartum dams in the first experiment. The sensitized and non-sensitized females did not differ in their mPFC GAD65 or vGAT levels. This indicates that the mPFC differences in the first study between parous and nulliparous females were more likely related to postpartum physiological adaptations, although it is also possible that the natural and sensitized models of maternal behavior produce different changes in the GABA system or even utilize different neurochemical systems to converge upon the same behavioral outcome. It is also the case that maternally sensitized virgins show somewhat inferior maternal caregiving behaviors compared to postpartum rats (8, 36–38, 40, 66, 67). The more robust and complete display of the behaviors characteristic of postpartum mothers may require the changes in GAD65 and vGAT revealed in the first study. Experiments determining the effects of promoting or inhibiting mPFC GABA signaling on maternal behaviors in sensitized nulliparous rats would help elucidate these possibilities.
It was also possible that our parity differences in mPFC GAD65 and vGAT in the first study were related to parity differences in behaviors other than maternal caregiving. As mentioned above, most early postpartum rats show less anxiety-related behavior when compared to diestrous virgins, a phenomenon requiring that dams have recent physical contact with the litter and is partly mediated by high maternal central GABAAR activity (12, 13, 68). Sabihi et al. (14) recently found that the low anxiety state of postpartum rats tested in an EPM was temporarily prevented by GABAAR antagonism with bicuculline infused into the mPFC. Conversely, mothers separated from their pups for 4 h before testing showed relatively high anxiety, but not if the GABAAR agonist muscimol was infused into their mPFC (14). Muscimol infusion did not further decrease anxiety in the non-separated mothers, indicating that they were already at a ceiling for the effects of mPFC GABAAR on postpartum anxiety-related behaviors. Consistent with these results, we here found that within postpartum rats kept with their pups until testing, GAD67 and vGAT levels in the mPFC were unrelated to whether dams showed high anxiety or low anxiety behavior in an EPM. Thus, it appears that as long as mothers have reached a particular (i.e., high) threshold of GABAergic activity in their mPFC, exceeding it through natural variation or pharmacological methods may be mostly irrelevant to maternal affective behaviors.
Since maternal caregiving or anxiety state were apparently not alone responsible for differences among parous and nulliparous groups in their GAD67 and vGAT expression, differences in their endocrine histories surely contributed instead. Both groups of females were in a diestrous state at sacrifice, but it is notable that serum concentrations of the progesterone metabolite and neurosteroid, allopregnanalone, are significantly higher in postpartum day 8 rats compared to diestrus cycling females (69). Relatively rapid fluctuations in peripheral and central allopregnanalone and other neurosteroids that occur across reproductive states (16, 70) strongly affect central GABA signaling (71–75), but it is yet unknown if the persistent neurosteroid elevations at the end of the first week postpartum are relevant or not to our findings. Assessing GAD65 and vGAT after giving dams a 5-α-reductase inhibitor, which prevents progesterone's conversion into its neurosteroid metabolites, would help determine this. Parous females also differ from nulliparous females in the pulses of central and peripheral oxytocin and prolactin release that occur during interactions with pups. These neuropeptides also affect the GABA system and vice-versa (1, 76–78) so could have contributed to the reproductive state differences we found.
It would be valuable for future studies to assess reproductive-state changes in other aspects of the GABA system in the mPFC, as well as elsewhere in the brain. As noted above, studies of female reproductive state differences in GABAA and benzodiazepine receptor binding in numerous brain sites have found mostly negative results (16–19), but reproductive experience does affect the expression of some of the 19 unique receptor subunit proteins that constellate to form GABAARs (16, 79–81). The subunit makeup of GABAARs has tremendous consequences for their sensitivity and function (71, 82, 83). In fact, we have found that expression of the delta subunit of the GABAAR, which is found extrasynaptically and mediates tonic inhibition under low ambient GABA concentrations, is significantly higher in the midbrain periaqueductal gray [PAG—a site involved in postpartum nursing, anxiety, and aggression (36, 84, 85)] of postpartum rats compared to diestrus virgins (Ahmed et al., unpublished data). To gain an even better picture of how female reproductive state affects the central GABA system, it would also be valuable to measure mPFC levels of the GABA plasma membrane transporter (GAT1), which can remove GABA from the extracellular space as well as increase GABA concentrations via a non-vesicular reverse transport mechanism (86).
Data Availability Statement
The raw data supporting the conclusions of this article will be made available by the authors, without undue reservation.
Ethics Statement
The animal study was reviewed and approved by Institutional Animal Care and Use Committee (IACUC) at Michigan State University.
Author Contributions
CR: contributed to running animal and Western blot experiments, analyzing data, creating figures, and manuscript writing. EA: ran Western blot experiments and conducted data analyses. EV: ran animal Western blot experiments and conducted data analyses. KL-D: ran Western blot experiments. SM-S: contributed to the experiments' conceptualization, developed the Western blot protocols, conducted the pilot experiments, and contributed to the manuscript writing. JM: contributed to discussions about analyzing, interpreting the data, and manuscript editing. JL: contributed by conceptualizing the experiments, analyzing data, creating figures, writing, and editing the manuscript. All authors contributed to the article and approved the submitted version.
Funding
This research was supported in part by NICHD Grant R01HD057962 to JL.
Conflict of Interest
The authors declare that the research was conducted in the absence of any commercial or financial relationships that could be construed as a potential conflict of interest.
Publisher's Note
All claims expressed in this article are solely those of the authors and do not necessarily represent those of their affiliated organizations, or those of the publisher, the editors and the reviewers. Any product that may be evaluated in this article, or claim that may be made by its manufacturer, is not guaranteed or endorsed by the publisher.
References
1. Lonstein JS, Pereira M, Morrell JI, Marler CA. Parental behavior. In: Plant TM., Zeleznik AJ, eds. Knobil and Neill's Physiology of Reproduction, 4th ed. New York, NY: Elsevier. (2014).
2. Lonstein JS. Regulation of anxiety during the postpartum period. Front Neuroendocrinol. (2007) 28:115. doi: 10.1016/j.yfrne.2007.05.002
3. Bridges RS. Long-term alterations in neural and endocrine processes induced by motherhood in mammals. Horm Behav. (2016) 77:193–203. doi: 10.1016/j.yhbeh.2015.09.001
4. Numan M. The Parental Brain: Mechanisms, Development, and Evolution. Oxford: Oxford University Press. (2020).
5. Arriaga-Avila V, Martinez-Abundis E, Cardenas-Morales B, Mercado-Gomez O, Aburto-Arciniega E, Miranda-Martinez A, et al. Lactation reduces stress-caused dopaminergic activity and enhances GABAergic activity in the rat medial prefrontal cortex. J Mol Neurosci. (2014) 52:515–24. doi: 10.1007/s12031-013-0104-7
6. Kornblatt JJ, Grattan DR. Lactation alters gamma-aminobutyric acid neuronal activity in the hypothalamus and cerebral cortex in the rat. Neuroendocrinology. (2001) 73:175–84. doi: 10.1159/000054634
7. Qureshi GA, Hansen S, Sodersten P. Offspring control of cerebrospinal fluid GABA concentrations in lactating rats. Neurosci Lett. (1987) 75:85–8. doi: 10.1016/0304-3940(87)90080-2
8. Arrati PG, Carmona C, Dominguez G, Beyer C, Rosenblatt JS. GABA receptor agonists in the medial preoptic area and maternal behavior in lactating rats. Physiol Behav. (2006) 87:51–65. doi: 10.1016/j.physbeh.2005.08.048
9. Ferreira A, Picazo O, Uriarte N, Pereira M, Fernandez-Guasti A. Inhibitory effect of buspirone and diazepam, but not of 8-OH-DPAT, on maternal behavior and aggression. Pharmacol Biochem Behav. (2000) 66:389–96. doi: 10.1016/S0091-3057(00)00211-2
10. Hansen S, Ferreira A. Effects of bicuculline infusions in the ventromedial hypothalamus and amygdaloid complex on food intake and affective behavior in mother rats. Behav Neurosci. (1986) 100:410–5. doi: 10.1037/0735-7044.100.3.410
11. Lee G, Gammie SC. GABAA receptor signaling in the lateral septum regulates maternal aggression in mice. Behav Neurosci. (2009) 123:1169–77. doi: 10.1037/a0017535
12. Miller SM, Piasecki CC, Peabody MF, Lonstein JS. GABAA receptor antagonism in the ventrocaudal periaqueductal gray increases anxiety in the anxiety-resistant postpartum rat. Pharmacol Biochem Behav. (2010) 95:457–65. doi: 10.1016/j.pbb.2010.03.007
13. Miller SM, Piasecki CC, Lonstein JS. Use of the light-dark box to compare the anxiety-related behavior of virgin and postpartum female rats. Pharmacol Biochem Behav. (2011) 100:130–7. doi: 10.1016/j.pbb.2011.08.002
14. Sabihi S, Goodpaster C, Maurer S, Leuner B, GABA. in the medial prefrontal cortex regulates anxiety-like behavior during the postpartum period. Behav Brain Res. (2021) 398:112967. doi: 10.1016/j.bbr.2020.112967
15. Febo M, Felix-Ortiz AC, Johnson TR. Inactivation or inhibition of neuronal activity in the medial prefrontal cortex largely reduces pup retrieval and grouping in maternal rats. Brain Res. (2010) 1325:77–88. doi: 10.1016/j.brainres.2010.02.027
16. Concas A, Follesa P, Barbaccia ML, Purdy RH, Biggio G. Physiological modulation of GABAA receptor plasticity by progesterone metabolites. Eur J Pharmacol. (1999) 375:225–35. doi: 10.1016/S0014-2999(99)00232-0
17. Ferreira A, Hansen S, Nielsen M, Archer T, Minor BG. Behavior of mother rats in conflict tests sensitive to antianxiety agents. Behav Neurosci. (1989) 103:193–201. doi: 10.1037/0735-7044.103.1.193
18. Majewska MD, Ford-Rice F, Falkay G. Pregnancy-induced alterations of GABAA receptor sensitivity in maternal brain: an antecedent of post-partum ‘blues'? Brain Res. (1989) 482:397–401. doi: 10.1016/0006-8993(89)91208-0
19. Miller SM, Lonstein JS. Autoradiographic analysis of GABAA receptor binding in the neural anxiety network of postpartum and non-postpartum laboratory rats. Brain Res Bull. (2011) 86:60–4. doi: 10.1016/j.brainresbull.2011.05.013
20. Asada H, Kawamura Y, Maruyama K, Kume H, Ding RG, Kanbara N, et al. Cleft palate and decreased brain gamma-aminobutyric acid in mice lacking the 67-kDa isoform of glutamic acid decarboxylase. ProcNatlAcad Sci USA. (1997) 94:6496–9. doi: 10.1073/pnas.94.12.6496
21. Buddhala C, Hsu CC, Wu JY. A novel mechanism for GABA synthesis and packaging into synaptic vesicles. Neurochem Int. (2009) 55:9–12. doi: 10.1016/j.neuint.2009.01.020
22. Esclapez M, Tillakaratne NJ, Kaufman DL, Tobin AJ, Houser CR. Comparative localization of two forms of glutamic acid decarboxylase and their mRNAs in rat brain supports the concept of functional differences between the forms. J Neurosci. (1994) 14:1834–55. doi: 10.1523/JNEUROSCI.14-03-01834.1994
23. Jin H, Wu H, Osterhaus G, Wei J, Davis K, Sha D, et al. Demonstration of functional coupling between gamma-aminobutyric acid (GABA) synthesis and vesicular GABA transport into synaptic vesicles. Proc Natl Acad Sci USA. (2003) 100:4293–8. doi: 10.1073/pnas.0730698100
24. Choi SY, Morales B, Lee HK, Kirkwood A. Absence of long-term depression in the visual cortex of glutamic acid decarboxylase-65 knock-out mice. J Neurosci. (2002) 22:5271–6. doi: 10.1523/JNEUROSCI.22-13-05271.2002
25. Tian N, Petersen C, Kash S, Baekkeskov S, Copenhagen D, Nicoll R. The role of the synthetic enzyme GAD65 in the control of neuronal gamma-aminobutyric acid release. Proc Natl Acad Sci USA. (1999) 96:12911–6. doi: 10.1073/pnas.96.22.12911
26. Wu H, Jin Y, Buddhala C, Osterhaus G, Cohen E, Jin H, et al. Role of glutamate decarboxylase (GAD) isoform, GAD65, in GABA synthesis and transport into synaptic vesicles-Evidence from GAD65-knockout mice studies. Brain Res. (2007) 1154:80–3. doi: 10.1016/j.brainres.2007.04.008
27. Kubo K, Nishikawa K, Hardy-Yamada M, Ishizeki J, Yanagawa Y, Saito S. Altered responses to propofol, but not ketamine, in mice deficient in the 65-kilodalton isoform of glutamate decarboxylase. J Pharmacol Exp Therap. (2009) 329:592–9. doi: 10.1124/jpet.109.151456
28. Walls AB, Nilsen LH, Eyjolfsson EM, Vestergaard HT, Hansen SL, Schousboe A, et al. GAD65 is essential for synthesis of GABA destined for tonic inhibition regulating epileptiform activity. J Neurochem. (2010) 115:1398–408. doi: 10.1111/j.1471-4159.2010.07043.x
29. Wei W, Zhang N, Peng Z, Houser CR, Mody I. Perisynaptic localization of delta subunit-containing GABAA receptors and their activation by GABA spillover in the mouse dentate gyrus. J Neurosci. (2003) 23:10650–61. doi: 10.1523/JNEUROSCI.23-33-10650.2003
30. Munaro NI. Maternal behavior: glutamic acid decarboxylase activity in the olfactory bulb of the rat. Pharmacol Biochem Behav. (1990) 36:81–4. doi: 10.1016/0091-3057(90)90129-6
31. Rodriguez C, Guillamon A, Pinos H, Collado P. Postpartum changes in the GABAergic system in the bed nucleus of the accessory olfactory tract. Neurochem Int. (2004) 44:179–83. doi: 10.1016/S0197-0186(03)00115-3
32. Zhao C, Driessen T, Gammie SC. Glutamic acid decarboxylase 65 and 67 expression in the lateral septum is up-regulated in association with the postpartum period in mice. Brain Res. (2012) 1470:35–44. doi: 10.1016/j.brainres.2012.06.002
33. McIntire SL, Reimer RJ, Schuske K, Edwards RH, Jorgensen EM. Identification and characterization of the vesicular GABA transporter. Nature. (1997) 389:870–6. doi: 10.1038/39908
34. Wojcik SM, Katsurabayashi S, Guillemin I, Friauf E, Rosenmund C, Brose N, et al. A shared vesicular carrier allows synaptic corelease of GABA and glycine. Neuron. (2006) 50:575–87. doi: 10.1016/j.neuron.2006.04.016
35. Rosenblatt JS. Nonhormonal basis of maternal behavior in the rat. Science. (1967) J156:1512–4. doi: 10.1126/science.156.3781.1512
36. Lonstein JS, Wagner CK, De Vries GJ. Comparison of the “nursing” and other parental behaviors of nulliparous and lactating female rats. Horm Behav. (1999) 36:242–51. doi: 10.1006/hbeh.1999.1544
37. Ferreira A, Pereira M, Agrati D, Uriarte N, Fernández-Guasti A. Role of maternal behavior on aggression, fear and anxiety. Physiol Behav. (2002) 77:197–204. doi: 10.1016/S0031-9384(02)00845-4
38. Pereira M, Uriarte N, Agrati D, Zuluaga MJ, Ferreira A. Motivational aspects of maternal anxiolysis in lactating rats. Psychopharmacology. (2005) 180:241–8. doi: 10.1007/s00213-005-2157-y
39. Agrati D, Zuluaga MJ, Fernández-Guasti A, Meikle A, Ferreira A. Maternal condition reduces fear behaviors but not the endocrine response to an emotional threat in virgin female rats. Horm Behav. (2006) 53:232–40. doi: 10.1016/j.yhbeh.2007.10.002
40. Fleming AS, Rosenblatt JS. Maternal behavior in the virgin and lactating rat. J Comp Physiol Psychol. (1974) 86:957–72.
41. Ragan CM, Lonstein JS. Differential postpartum sensitivity to the anxiety-modulating effects of offspring contact is associated with innate anxiety and brainstem levels of dopamine beta-hydroxylase in female laboratory rats. Neuroscience. (2014) 256:433–44. doi: 10.1016/j.neuroscience.2013.10.014
42. Smith MS, Neill JD. Inhibition of gonadotropin secretion during lactation in the rat: relative contribution of suckling and ovarian steroids. Biol Reprod. (1977) 17:255–61. doi: 10.1095/biolreprod17.2.255
43. Hansen S, Sodersten P, Eneroth P. Mechanisms regulating hormone release and the duration of dioestrus in the lactating rat. J Endocrinol. (1983) 99:173–80. doi: 10.1677/joe.0.0990173
44. Yoshinaga K, Moudgal NR, Greep RO. Progestin secretion by the ovary in lactating rats: effect of LH-antiserum, LH and prolactin. Endocrinology. (1971) 88:1126–30. doi: 10.1210/endo-88-5-1126
45. Smith CD, Holschbach MA, Olsewicz J, Lonstein JS. Effects of noradrenergic alpha-2 receptor antagonism or noradrenergic lesions in the ventral bed nucleus of the stria terminalis and medial preoptic area on maternal care in female rats. Psychopharmacology. (2012) 24:263–76. doi: 10.1007/s00213-012-2749-2
46. Bridges RS. A quantitative analysis of the roles of dosage, sequence, and duration of estradiol and progesterone exposure in the regulation of maternal behavior in the rat. Endocrinology. (1984) 114:930–40. doi: 10.1210/endo-114-3-930
47. Swanson LW. Brain Maps, Third Edition: Structure of the Rat Brain. New York, NY, Elsevier (2003).
48. Taylor SC, Posch A. The design of a quantitative western blot experiment. Biomed Res Int. (2014) 2014:361590. doi: 10.1155/2014/361590
49. Kendrick KM, Keverne EB, Hinton MR, Goode JA. Oxytocin, amino acid and monoamine release in the region of the medial preoptic area and bed nucleus of the stria terminalis of the sheep during parturition and suckling. Brain Res. (1992) 569:199–209. doi: 10.1016/0006-8993(92)90631-I
50. Farrant M, Nusser Z. Variations on an inhibitory theme: phasic and tonic activation of GABAA receptors. Nat Rev Neurosci. (2005) 6:215–29. doi: 10.1038/nrn1625
51. Gabbott PL, Warner TA, Jays PR, Salway P, Busby SJ. Prefrontal cortex in the rat: projections to subcortical autonomic, motor, limbic centers. J Comp Neurol. (2005) 492:145–77. doi: 10.1002/cne.20738
52. Vertes RP. Differential projections of the infralimbic and prelimbic cortex in the rat. Synapse. (2004) 51:32–58. doi: 10.1002/syn.10279
53. Heidbreder CA, Groenewegen HJ. The medial prefrontal cortex in the rat: evidence for a dorso-ventral distinction based upon functional and anatomical characteristics. Neurosci Biobehav Rev. (2003) 27:555–79. doi: 10.1016/j.neubiorev.2003.09.003
54. Miller EK, Cohen JD. An integrative theory of prefrontal cortex function. Ann Rev Neurosci. (2001) 24:167–202. doi: 10.1146/annurev.neuro.24.1.167
55. Hernandez-Gonzalez M, Prieto-Beracoechea C, Navarro-Meza M, Ramos-Guevara JP, Reyes-Cortes R, Guevara MA. Prefrontal and tegmental electrical activity during olfactory stimulation in virgin and lactating rats. Physiol Behav. (2005) 83:749–58. doi: 10.1016/j.physbeh.2004.09.013
56. Febo M. Firing patterns of maternal rat prelimbic neurons during spontaneous contact with pups. Brain Res Bull. (2012) 88:534–42. doi: 10.1016/j.brainresbull.2012.05.012
57. Afonso VM, Sison M, Lovic V, Fleming AS. Medial prefrontal cortex lesions in the female rat affect sexual and maternal behavior and their sequential organization. Behav Neurosci. (2007) 121:515–26. doi: 10.1037/0735-7044.121.3.515
58. Pereira M, Morrell JI. Functional mapping of the neural circuitry of rat maternal motivation: effects of site-specific transient neural inactivation. J Neuroendocrinol. (2011) 23:1020–35. doi: 10.1111/j.1365-2826.2011.02200.x
59. Gupta A, Wang Y, Markram H. Organizing principles for a diversity of GABAergic interneurons and synapses in the neocortex. Science. (2000) 287:273–8. doi: 10.1126/science.287.5451.273
60. Lovic V, Palombo DJ, Fleming AS. Impulsive rats are less maternal. Dev Psychobiol. (2011) 53:13–22. doi: 10.1002/dev.20481
61. Brunton PJ, Russell JA, Douglas AJ. Adaptive responses of the maternal hypothalamic-pituitary-adrenal axis during pregnancy and lactation. J Neuroendocrinol. (2008) 20:764–76. doi: 10.1111/j.1365-2826.2008.01735.x
62. Ladyman SR, Khant Aung Z, Grattan DR. Impact of pregnancy and lactation on the long-term regulation of energy balance in female mice. Endocrinology. (2018) 159:2324–36. doi: 10.1210/en.2018-00057
63. Anderson SM, MacLean PS, McManaman JL, Neville MC. Lactation and its Hormonal Control. Knobil and Neill's Physiology of Reproduction. 4 ed. New York, NY: Elsevier (2015). p. 2055–105.
64. Slattery DA, Neumann ID. No stress please! Mechanisms of stress hyporesponsiveness of the maternal brain. J Physiol. (2008) 586:377–85. doi: 10.1113/jphysiol.2007.145896
65. Woodside B, Budin R, Wellman MK, Abizaid A. Many mouths to feed: the control of food intake during lactation. Front Neuroendocrinol. (2012) 33:301–14. doi: 10.1016/j.yfrne.2012.09.002
66. Erskine MS, Barfield RJ, Goldman BD. Postpartum aggression in rats: II. Dependence on maternal sensitivity to young and effects of experience with pregnancy and parturition. J Comp Physiol Psychol. (1980) 94:495–505. doi: 10.1037/h0077677
67. Gandelman R, Simon NG. Postpartum fighting in the rat: nipple development and the presence of young. Behav Neural Biol. (1980) 28:350–60. doi: 10.1016/S0163-1047(80)92357-2
68. Lonstein JS. Reduced anxiety in postpartum rats requires recent physical interactions with pups, but is independent of suckling and peripheral sources of hormones. Horm Behav. (2005) 47:241–55. doi: 10.1016/j.yhbeh.2004.11.001
69. Kellogg CK, Barrett KA. Reduced progesterone metabolites are not critical for plus-maze performance of lactating female rats. Pharmacol Biochem Behav. (1999) 63:441–8. doi: 10.1016/S0091-3057(99)00041-6
70. Frye CA, Bayon LE. Mating stimuli influence endogenous variations in the neurosteroids 3alpha,5alpha-THP and 3alpha-Diol. J Neuroendocrinol. (1999) 11:839–47. doi: 10.1046/j.1365-2826.1999.00379.x
71. Maguire J. Neuroactive steroids and GABAergic involvement in the neuroendocrine dysfunction associated with major depressive disorder and postpartum depression. Front Cell Neurosci. (2019) 13:83. doi: 10.3389/fncel.2019.00083
72. Maguire J, Mody I. Steroid hormone fluctuations and GABAAR plasticity. Psychoneuroendocrinology. (2009) 34(Suppl 1):S84–90. doi: 10.1016/j.psyneuen.2009.06.019
73. Mostallino MC, Sanna E, Concas A, Biggio G, Follesa P. Plasticity and function of extrasynaptic GABAA receptors during pregnancy and after delivery. Psychoneuroendocrinology. (2009) 34(Suppl 1):S74–83. doi: 10.1016/j.psyneuen.2009.06.013
74. Lovick TA, Devall AJ. Progesterone withdrawal-evoked plasticity of neural function in the female periaqueductal grey matter. Neural Plasticity. (2009) 2009:730902. doi: 10.1155/2009/730902
75. Smith SS, Shen H, Gong QH, Zhou X. Neurosteroid regulation of GABAA receptors: focus on the alpha4 and delta subunits. Pharmacol Therap. (2007) 116:58–76. doi: 10.1016/j.pharmthera.2007.03.008
76. Duvilanski BH, Seilicovich A, Lasaga M, del Carmen Diaz M, Debeljuk L. Mechanisms of endogenous GABA release from hypothalamic fragments effect of prolactin. Neuroendocrinology. (1987) 46:504–10. doi: 10.1159/000124873
77. Duvilanski BH, Seilicovich A, Diaz MC, Lasaga M, Debeljuk L. The effect of prolactin on glutamate decarboxylase activity and GABA concentration in hypothalamic slices. Psychoneuroendocrinology. (1987) 12:107–16. doi: 10.1016/0306-4530(87)90041-2
78. Theodosis DT, Poulain DA. Maternity leads to morphological synaptic plasticity in the oxytocin system. Prog Brain Res. (2001) 133:49–58. doi: 10.1016/S0079-6123(01)33004-2
79. Follesa P, Floris S, Tuligi G, Mostallino MC, Concas A, Biggio G. Molecular and functional adaptation of the GABAA receptor complex during pregnancy and after delivery in the rat brain. Eur J Neurosci. (1998) 10:2905–12. doi: 10.1111/j.1460-9568.1998.00300.x
80. Sanna E, Mostallino MC, Murru L, Carta M, Talani G, Zucca S, et al. Changes in expression and function of extrasynaptic GABAA receptors in the rat hippocampus during pregnancy and after delivery. J Neurosci. (2009) 29:1755–65. doi: 10.1523/JNEUROSCI.3684-08.2009
81. Byrnes EM, Lee JO, Bridges RS. Alterations in GABAA receptor alpha2 subunit mRNA expression following reproductive experience in rats. Neuroendocrinology. (2007) 85:148–56. doi: 10.1159/000102535
82. Maguire J, Mody I. GABAAR plasticity during pregnancy: relevance to postpartum depression. Neuron. (2008) 59:207–13. doi: 10.1016/j.neuron.2008.06.019
83. Maguire J, Ferando I, Simonsen C, Mody I. Excitability changes related to GABAA receptor plasticity during pregnancy. J Neurosci. (2009) 29:9592–601. doi: 10.1523/JNEUROSCI.2162-09.2009
84. Lonstein JS, Stern JM. Role of the midbrain periaqueductal gray in maternal nurturance and aggression: c-fos and electrolytic lesion studies in lactating rats. J Neurosci. (1997) 17:3364–78. doi: 10.1523/JNEUROSCI.17-09-03364.1997
85. Lonstein JS, Simmons DA, Stern JM. Functions of the caudal periaqueductal gray in lactating rats: kyphosis, lordosis, maternal aggression, and fearfulness. Behav Neurosci. (1998) 112:1502–18. doi: 10.1037/0735-7044.112.6.1502
Keywords: anxiety, GABA, lactation, maternal behavior, medial prefrontal cortex, reproductive state
Citation: Ragan CM, Ahmed EI, Vitale EM, Linning-Duffy K, Miller-Smith SM, Maguire J and Lonstein JS (2022) Postpartum State, but Not Maternal Caregiving or Level of Anxiety, Increases Medial Prefrontal Cortex GAD65 and vGAT in Female Rats. Front. Glob. Womens Health 2:746518. doi: 10.3389/fgwh.2021.746518
Received: 24 July 2021; Accepted: 27 December 2021;
Published: 08 February 2022.
Edited by:
Lauren M. Osborne, Johns Hopkins University, United StatesReviewed by:
Anne T. M. Konkle, University of Ottawa, CanadaStephen Kennedy, University of Oxford, United Kingdom
Copyright © 2022 Ragan, Ahmed, Vitale, Linning-Duffy, Miller-Smith, Maguire and Lonstein. This is an open-access article distributed under the terms of the Creative Commons Attribution License (CC BY). The use, distribution or reproduction in other forums is permitted, provided the original author(s) and the copyright owner(s) are credited and that the original publication in this journal is cited, in accordance with accepted academic practice. No use, distribution or reproduction is permitted which does not comply with these terms.
*Correspondence: Joseph S. Lonstein, bG9uc3RlaW5AbXN1LmVkdQ==