- 1School of Earth Sciences, The Ohio State University, Columbus, OH, United States
- 2Byrd Polar and Climate Research Center, The Ohio State University, Columbus, OH, United States
Urban populations and the sprawl of urban environments are increasing in the United States as well as globally. The local hydrologic cycle is directly impacted by urban development through greater generation of surface runoff and export of water through subterranean pipes networks to surface water bodies. These pipe networks carry waters that have potentially dramatic effects on the chemistry of groundwater and surface water bodies. In this work, we sampled waters from the Olentangy River and two subterranean outfalls that flow into the river in Columbus, Ohio United States. We measured the major ion, nutrient, and dissolved silica concentrations of each water source to identify how the urban landscape impacts the chemistry of a river that travels from an agricultural landscape to an urban environment. The outfalls had elevated concentrations of all major ions (Na+, K+, Mg2+, Ca2+, Cl−, SO42-) and H4SiO4. However, the Olentangy river typically had greater NO3− and soluble reactive phosphorus (SRP) concentrations. Sources of elevated ion export include road salts and combined storm runoff (Na+, Cl−), municipal water treatment practices (K+, Na+, SO42-), and concrete pipe weathering (Ca2+, Mg2+, K+, H4SiO4, SO42-). Utilizing stable isotopes of water, δ18O and δ2H, we identified that the water in the pipe networks is typically a mix of multiple precipitation events, but there is evidence of flushing following high-volume precipitation events. The contribution of high TDS waters from subterranean urban outfalls modified the ion abundance in the Olentangy river and produces a tendency towards freshwater salinization syndrome. This is particularly apparent when comparing the chemistry of the urban Olentangy to the agricultural corridor of the river and its other source waters. This research details the transformation of a river as it flows from an agricultural to urban landscape and provides data on the chemistry of source waters that facilitate the river’s chemical changes.
1 Introduction
The investigation of the geochemical quality of water in urban and suburban areas has traditionally been the purview of environmental and sanitary engineers to maintain a potable water supply that is safe for human consumption. The complexity of urban landscapes in terms of age, development, population density, variety of transportation modes make urban areas diverse in their impact on water quality. The interaction of water with human made infrastructure, the disturbance of the natural soil surface and subsurface through building and construction, and the diversion of the hydrologic cycle through increased impervious surfaces and stream channelization have significant consequences on water quality (Gardner and Carey, 2004). For example, the combination of the loss of more natural “green space” and the rapid diversion of water through human drainage pathways changes the hydrological connectivity of the landscape leading to less retention of more particle reactive elements and compounds in the urban setting, allowing direct and rapid introduction into urban aquatic systems. By 2050, approximately 68% of the population is expected to live in urban areas, and within the United State 89% of the population will live in urban areas (United Nations, 2018). This shift in population dynamics will be accompanied by an increase in urban land area, where current urban area composes 3% of the United States landscape and will account for over 6% by 2060 (Center for Sustainable Systems, 2023; references within). To understand the role of urban landscapes on the overall impact of anthropogenic activities on local, regional, and global biogeochemical cycling, there is a need to elucidate and quantify the sources and fates of chemicals in cities (Lyons and Harmon, 2012; Lyons and Harmon, 2012). Geochemical and ecological research originating from the Baltimore Ecosystem Study Long Term Ecological Research (LTER) Network in Baltimore, MD has led research in understanding the complex biogeochemical interactions among human and natural environments (Kaushal and Belt, 2012; Pickett et al., 2020) It is essential to recognize both spatial and temporal (current and legacy) impacts of chemical fluxes from urban landscapes to contrast urban geochemical behavior to cities of different ages, structures, and developmental evolution. It is also vital to compare urban chemical fluxes to those of more natural landscapes (i.e., forests, grasslands, etc.), and other human-managed landscapes, such as agricultural settings (Fitzpatrick et al., 2007; Chambers et al., 2016).
Seminal work by Kaushal and his co-workers have argued that due to variations in infrastructure, neighborhood age and history, population density, vehicle traffic flow and volume, and other environmental constructs, the composition of urban runoff water can vary dramatically. They have termed such water compositions as “chemical cocktails” (Kaushal et al., 2018a, Kaushal et al., 2018b, Kaushal et al., 2019, Kaushal et al., 2020, Kaushal et al., 2022). Variations include introduction of major ions via deicing activities and the chemical weathering of human-built infrastructure such as roads and buildings, nutrient inputs from sewage overflow, fertilizers, and combustion products, and trace elements from industrial wastes, vehicle wear, and fossil fuel combustion products (Steele et al., 2010). Although these studies clearly demonstrate that baseline concentrations of most of these constituents have increased over time, spatial and temporal variations occur from the difference in the biophysical characteristics of each urban watershed.
It has been suggested that there is an evolution of biogeochemical cycling in urban aquatic systems as land use and infrastructure change over time (Kaushal and Belt, 2012). Although recent work has investigated the influence and variation of low-order urban streams to document the transport of urban-introduced chemicals from their source downstream (Kaushal et al., 2020), it has been recognized that vertical water movement in urban settings may also have influence on the overall quality of urban steams and rivers (Kaushal and Belt, 2012). Subsurface urban flow systems have been termed “urban karst” and refers to water movement through a high-density network of pipes, including ones transporting storm overflow, combined storm and sanitary sewer flow, and potable, municipal water, along with building discharge from cooling, heating or other sources, and naturally occurring groundwater (Kaushal and Belt, 2012). This flow can be enhanced by previous construction disturbances, foundation drainage, and leaking older infrastructure. Garcia-Fresca and Sharp (2005) were among the first to recognize the hydrological similarity between urban areas and naturally occurring carbonate karst landscapes in that the shallow subsurface can be dominated by fractures, conduits, and cave-like human produced structures that have produced secondary porosity and increase permeability and can lead to rapid, increased flow during precipitation events. The significance of urban karst on water transport, water budgets, and solute transported have been a topic of more hydrological science interest recently (Bonneau et al., 2017; Galvão et al., 2017; Shepley et al., 2020; D’Aniello et al., 2021). From a geochemical perspective, leakage from watermains has recently been shown to be a heretofore important source of phosphorus in the United States (Flint et al., 2023; Flint et al., 2023), suggesting that aging infrastructure transporting municipal water could be a significant source of certain chemicals into the environment. From a water quality perspective, it is now clear that urban water is a complex mixture of components of different origins that are largely dependent upon the age of the urban infrastructure age, hydrologic connectivity, human population and road density. A better understanding of contaminant loadings is needed to predict the fate and transport of these urban water components (Lapointe et al., 2022), especially if green infrastructure is to be designed and strategically placed to minimize urban water impact on portable water resources (Smith et al., 2023).
In this paper we present 12 months of geochemical data obtained from water samples from 2 outfalls draining the subsurface areas to the east of the Olentangy River, associated with and adjacent to the campus of The Ohio State University, Columbus, Ohio, United States. The goal of this paper is to quantify and characterize the chemistry of urban runoff into the Olentangy River to discern how the urban landscape modifies the chemistry of a river as it travels from an agricultural to urban environment. The objectives of this work were (1) to measure major ions, nutrients, dissolved silica, and stable water isotopes of urban runoff and the Olentangy River and (2) to compare these chemistries to other local waters and water sources to conceptualize the “evolution” of water in urban landscapes. The samples were collected on a semi biweekly basis and their geochemistry compared to data collected at the same time from the river. The outfall waters were initially thought to represent storm water from the local impervious surface surrounding the university, but we now view the outfalls as a mixture of different water sources reflective of the localized urban karst system. The work was undertaken to assess the geochemistry of the outfall waters and how it varies with time and influences the geochemistry of the urban river.
2 Study site
2.1 The Olentangy River
The Olentangy River watershed covers 1,370 km2 of central Ohio. The watershed is dominated by row-crop agriculture in its northern portion that transitions into suburban areas north of Columbus in both Delaware and Franklin counties (Figure 1). The river flows through glacial tills deposited during the Wisconsinian glaciation and drains Upper Devonian and Lower Mississippian shale in the northern, agricultural region of the watershed. As the river flows south, toward the urban corridor, the river also drains Middle to Lower Devonian limestone (Ohio Division of Geological Survey, 2006).
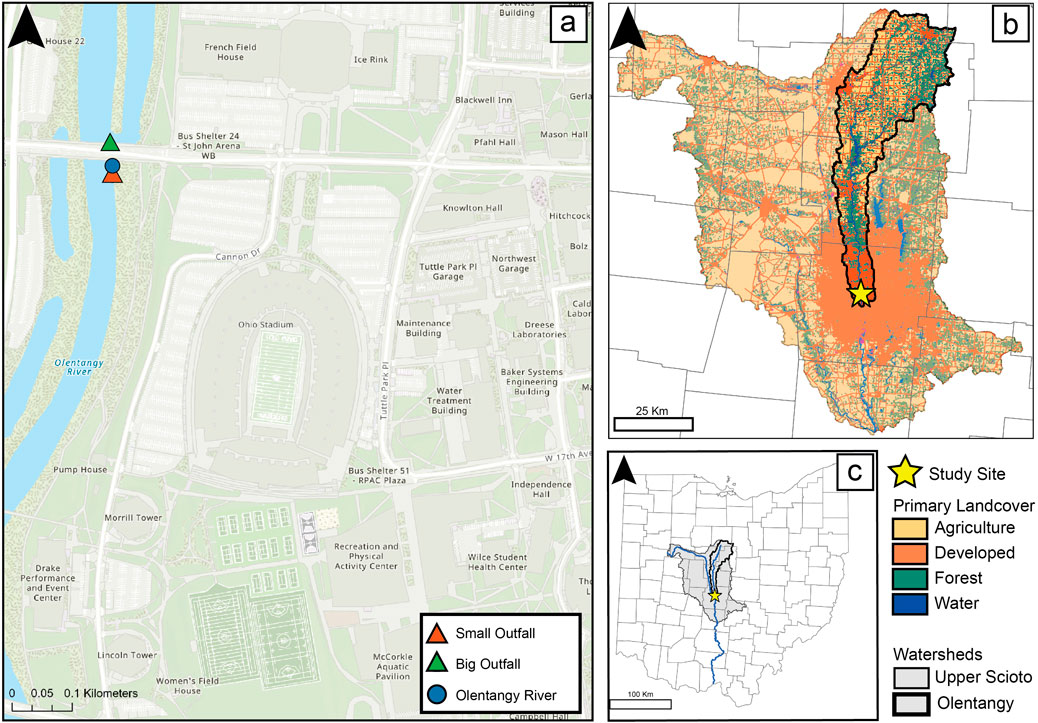
Figure 1. Map of The Ohio State University campus with sample points denoted (A). The Scioto River (faded) and Olentangy River (bold) watersheds with landcover depicted (B). State of Ohio with county boundaries and the upper Scioto and Olentangy watersheds shaded gray (C) (USGS, 2011; USGS, 2023; National Land Cover Database, 2019; The Ohio State University, 2024). Figure modified with additional data from Lyons et al. (2024).
The Olentangy flows through a major environmental transition. North of metropolitan Columbus, a 35 km segment of the river is designated as a Scenic River by the Ohio Department of Natural Resources for its undisturbed riparian buffer. The Olentangy is an important source of drinking water in Delaware county, north of Columbus. The river is also a valued recreation resource. It feeds and drains Delaware Lake and a recreation trail that runs alongside the river as it approaches the center city of Columbus. Below Delaware Lake, the river flows into the populated metropolitan area of Columbus, Ohio, which is the 14th largest city in the United States, with a population density of ∼1,400 people/km2 (United States Census Bureau, 2021). Once reaching the metropolitan area of Columbus, the river is dammed by a series of low-head dams that control water flow. The Olentangy River is a tributary to the Scioto River, which also flows from an agricultural landscape through urban Columbus (Figure 1). The confluence of the two rivers is in center city Columbus, Ohio. As the Olentangy flows through metropolitan Columbus, it receives numerous types of runoff and the input of chemical constituents from various sources, including agriculture, urban and suburban runoff, and other anthropogenic activities (Gardner and Carey, 2004; Wichterich et al., 2024). Both sanitary sewer overflows (SSOs) and combined sewer overflows (CSOs) discharge into the river. These outfalls drain infrastructure, impervious surfaces of streets and parking areas containing stormwater, and greywater. The geochemistry of these outfall waters is the primary focus of this work.
2.2 Sample locations
Olentangy River and outfall waters that drain directly into the river were collected from three locations on The Ohio State University campus (Figure 1). The campus is located approximately 5 km north of the Olentangy-Scioto confluence. The three sample locations include (1) Big Outfall - an outfall fed by a subsurface drainage network that extends toward the north section of The Ohio State University campus and the surrounding area north east of campus (Ohio State Facilities and Engineers, personal communication), (2) Small Outfall - an outfall that is fed by a smaller, subsurface drainage network, which is centered on the eastern portion of Ohio State’s main campus (3) Olentangy River. The Big Outfall is located beneath Woody Hayes Bridge, a major east-west street on campus. The Small Outfall is located approximately 30 m south (downstream) of the Big Outfall location. The Olentangy River Sample was collected directly from the river between the two outfall locations (Figure 1).
Outfall networks on campus are exclusively meant for storm water, while networks off campus are usually sanitary sewer overflows (SSOs) and combined sewer overflows (CSOs). The Big Outfall is a point source with a pipe network that extends into the Columbus’ drainage system and is fed by a combination of Ohio State campus runoff, Columbus City SSOs, and CSOs. It drains a wider area and runs along W. Woodruff Drive and Woody Hayes Drive (Figure 1). The outfall drainage area extends east and north of campus, collecting water within 0.5 km north and east of campus. The Small Outfall is also a point source with a pipe network that drains runoff from campus and does not extend beyond Ohio State. The Small Outfall drains the northern parking lots of Ohio Stadium, Tuttle Park Pl Parking Garage, and Hitchcock Hall (Figure 1). The outfall also received water from Tuttle Park Pl Parking Garage detention basin. The impervious surfaces of both outfall drainages included roadways, parking lots, building roofs, and building structures. The retention time of water within the water network is unknown and may vary depending on precipitation patterns and the volume of water in the pipe network. Water does not constantly flow from the outfalls, and the discharge is dependent on local precipitation.
3 Methods
3.1 Sample collection
Surface water samples were collected from November 2021 to November 2022 on a weekly or bi-weekly basis from the Olentangy River and the two outfalls draining the Ohio State and surrounding area (Figure 1). Samples were collected in a 1 L plastic bottle attached to ∼1.3 m PVC pipe that was rinsed three times with running source water prior to collection. The collected sample was divided into two aliquots. The first aliquot was decanted into 250 mL bottle that was pre-cleaned with 18.2 MΩ deionized water. These aliquots were filtered through 0.45 μm pore-size Whatman polypropylene filters using 30 mL previously unused polypropylene Luer Lock syringes in 60 mL pre-cleaned HDPE bottles (Lyons et al., 2021; Lyons et al., 2021). Samples were filtered within 12 h, and most within 2 h, of collection. The second aliquot was decanted into a 20 mL scintillation vials with no headspace for stable water isotope analysis. All samples were immediately transported back to the laboratory and stored at 3°C–4°C in a dark refrigerator until analyzed.
3.2 Analytical methods
Filtered samples were analyzed for anions (Cl−, SO42-, F−, Br−) and cations (Na+, K+, Mg2+, Ca2+) via ion chromatography (Welch et al., 2010), and nutrients (NO3−, soluble reactive phosphorus-SRP) and H4SiO4 via Skalar SAN++ Continuous Flow Analyzer using colorimetric methods. Techniques and procedures are most recently discussed by (Lyons et al., 2021). Bicarbonate (HCO3−) concentrations were determined by the charge imbalance of major anions and cations (Welch et al., 2010). We acknowledge that minor cation concentrations not considered in the calculation of HCO3− have a lesser impact on the concentration of HCO3− that is not accounted for in the reported data. Accuracy for anions and cations was ≤9%, and accuracy for nutrients and H4SiO4 was ≤10%. In-run precision for anions was ≤1%, cations was ≤7%, and nutrients was ≤10%. Detailed description of accuracy and precision calculation for ion and nutrient analyses are published by Wichterich et al. (2024). We calculated Pearson correlation coefficients among ions and nutrients to identify patterns within the datasets, with measured significance at the 95% confidence level.
Unfiltered samples collected in 20 mL scintillation vials with no headspace were analyzed on a Picarro Wavelength Scanned-Cavity Ring Down Spectroscopy Analyzer Model L1102-I for δ18O and δ2H. A sample aliquot of 2 mL was used for analysis, where seven injections of 2.3 μL were made per sample. The first three injections were discarded to avoid memory effects and the last four injections were averaged to obtain the sample δ18O and δ2H raw values. The sample δ18O and δ2H were corrected by internal laboratory standards that had been calibrated to VSMOW (δ18O = 0‰, δ2H = 0‰) at the Institute of Arctic and Alpine Research (INSTAAR) at University of Colorado at Boulder by a dual inlet mass spectrometer. Internal laboratory standards were Colorado (δ18O = −16.53‰; δ2H = −126.3‰), Nevada (δ18O = −14.20‰; δ2H = 104.80‰), Ohio (δ18O = −8.99‰; δ2H = −61.80‰), and Florida (δ18O = −2.09‰; δ2H = −9.69‰). Two internal standards were run at the beginning and end of each sample run and after every fifth sample. Instrument precision was 0.016‰ for δ18O and 0.15‰ δ2H (Smith et al., 2024). Accuracy for samples was determined by the difference between the known value of the standard and the correct value of the standard. In-run accuracy was ≤0.34‰ for δ18O and ≤1.0‰ for δ2H. Instrument uncertainty was calculated by propagating the error of accuracy and precision measurements:
where instrument propagated uncertainty was 0.34‰ for δ18O and 1.0‰ for δ2H. River and outlet sample δ18O and δ2H values were compared to local precipitation values (Smith et al., 2021; Smith et al., 2024). Precipitation volume and temperature data were downloaded from the College of Food, Agricultural, and Environmental Sciences weather station on the Ohio State University Campus (CFAES, 2023). Methods of precipitation collection and measurement are reported in (Smith et al., 2021; Smith et al., 2024).
4 Results
4.1 Geochemical temporal patterns
Throughout the sampling period, major ion concentrations from the Big Outfall and Small Outfall waters were typically greater than the Olentangy River. There was a wide range (<150 to >3,400 µM) of Cl− concentrations measured from the Big and Small Outfalls (Supplementary Table S1). Cl−concentrations measured from the Small Outfall were frequently greater than the EPA Chronic Threshold for aquatic life (6,488 μM, Figure 2A) (Environmental Protection Agency, 2024b). Generally, Cl− concentrations measured in the Big Outfall were lower than the EPA Chronic Threshold, with Cl− values greatest in the early winter months of 2022 that systematically declined into late autumn 2022 (Figure 2A). River concentrations remained below the EPA Chronic threshold and were relatively consistent (<2,500 uM) across the study period. The exception to this pattern were 7 sample events from January to April 2022, when Cl− concentrations were elevated (Figure 2A). Outfall and river Na+ concentrations followed a very similar pattern to Cl− (Figure 2B). All Olentangy River samples plotted on a 1:1 Na:Cl line, with some enrichment in Cl− concentrations (Supplementary Figure S1). In general, the Big Outfall and Small Outfall samples tended to be enriched in Cl− compared to Na+, particularly at higher concentrations (Supplementary Figure S1).
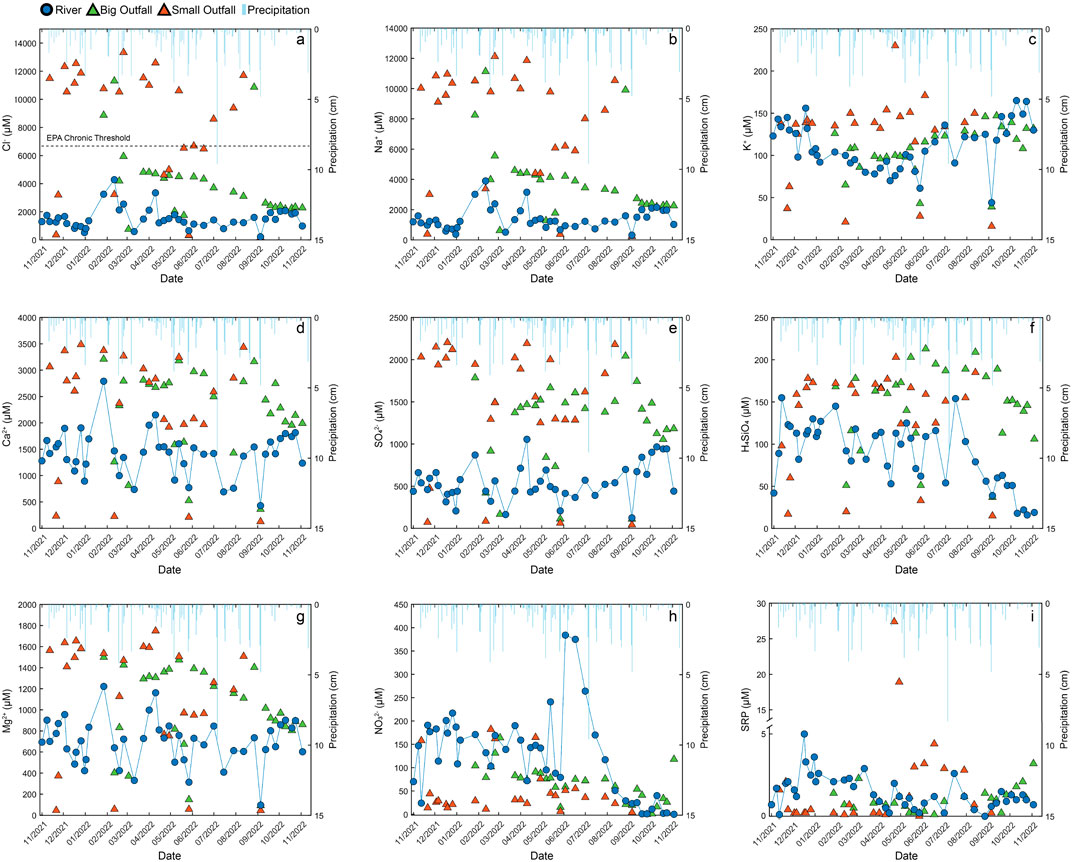
Figure 2. Cl− (A), Na+ (B), K+ (C), Ca2+ (D), SO42- (E), H4SiO4 (F), Mg2+ (G), NO3−(H), soluble reactive phosphorus (SRP) (I), concentrations measured in Olentangy River, Big Outfall and Small Outfall sample locations from November 2021 to November 2022. Precipitation amount is denoted by light blue lines (CFAES, 2023). EPA Chronic Threshold limit for Cl−shown as a dashed line (Environmental Protection Agency, 2024; Environmental Protection Agency, 2024).
Like patterns observed for Cl− concentrations, K+ concentrations measured in the Small Outfall were typically greater than the Big Outfall and the Olentangy River (Figure 2C). K+ concentrations in the Olentangy River displayed a pattern of elevated values in November 2021 that fell in winter 2022, and slowly increased over the course of the calendar year (Figure 2C). This general pattern was only disrupted by precipitation and corresponding dilution events, where K+ concentrations declined following large volume events (Figure 2C). The Small Outfall and Big Outfall also exhibited evidence of dilution following precipitation events (Figure 2C).
Unlike Cl− and K+, there was no distinct difference between the outfalls for Ca2+, SO42-, H4SiO4 and Mg2+ (Figure 2; Supplementary Table S1). Ca2+ concentrations measured in the Big Outfall (∼360–3,200 µM), Small Outfall (∼130–3,500 µM), and Olentangy River (∼430–2,800 µM) varied over the course of the study period (Figure 2D; Supplementary Table S1). The means of the Big Outfall (2,238 µM) and the Small Outfall (2,294 µM) were similar, but the Olentangy River mean Ca2+ concentration was lower (1,432 µM) (Supplementary Table S1). After a large precipitation event or multiple smaller events in quick succession, Ca2+ concentrations in the Olentangy declined, however this dilution pattern was inconsistent for the outfall waters. Temporal patterns for SO42- and H4SiO4 were similar to that of Ca2+ (Figures 2E, F). Big Outfall means for SO42-(1,220 µM), and H4SiO4 (148 µM) were similar to the Small Outfall SO42- (1,475 µM), and H4SiO4 (134 µM) concentrations, while the Olentangy River SO42- (548 µM) and H4SiO4 (88 µM) were substantially lower (Supplementary Table S1).
NO3− concentrations in the Olentangy River were typically <220 µM and greater than concentrations measured in the Big Outfall and Small Outfall (Figure 2H). Riverine concentration rose to ∼400 µM in late spring and early summer, which is greater than half of the United States Environmental Protection Agency maximum contaminant level for N-NO3- in drinking water (714 µM) (Environmental Protection Agency, 2024a). NO3− concentrations declined into late autumn of 2022. NO3− concentrations measured in the Small Outfall typically had the lowest values of the three sample locations, with a mean of 51 µM compared to the Big Outfall (65 µM) and the Olentangy (125 µM). (Supplementary Table S1). Evidence of dilution was not consistently apparent in the NO3− concentration patterns (Figure 2H). Instead, elevated NO3− concentrations sometimes corresponding to high volume precipitation events, particularly in late spring and early summer, suggesting that NO3− was being flushed into the river system during these high-volume precipitation events.
SRP concentrations of the outfalls were typically lower than the river SRP concentrations (Figure 2I). However, elevated concentrations were measured in the Small Outfall between April and August. Patterns of dilution were evident in the Olentangy River but did not necessarily follow the same dilution patterns as NO3− concentrations.
4.2 Geochemical correlations and spatial patterns
Pearson correlations of major ions, H4SiO4, and NO3− for the Big Outfall, Small Outfall, and Olentangy presented further differences among the samples’ geochemistries. Ca2+ concentrations exhibited robust, significant correlations with SO42-, H4SiO4 and Mg2+ concentrations (Table 1). This same finding was true for the Small Outfall, where Ca2+ concentrations also had significant robust correlations with Cl− and K+. This pattern was similar for the Olentangy River, however correlations of Ca2+ – K+, Ca2+ – H4SiO4, and Ca2+ – SO42- were weaker in relation to the outfalls (Table 1). NO3− concentrations did not show a strong positive correlation with any other analyte, except for a significant relationship with H4SiO4 for the Olentangy River. Small Outfall Cl− concentrations showed strong, significant correlations with all other analytes except for NO3−, and while correlations among Cl− and other analytes were significant in the Big Outfall and Olentangy, the correlation coefficients were not consistently as large as the Small Outfall.
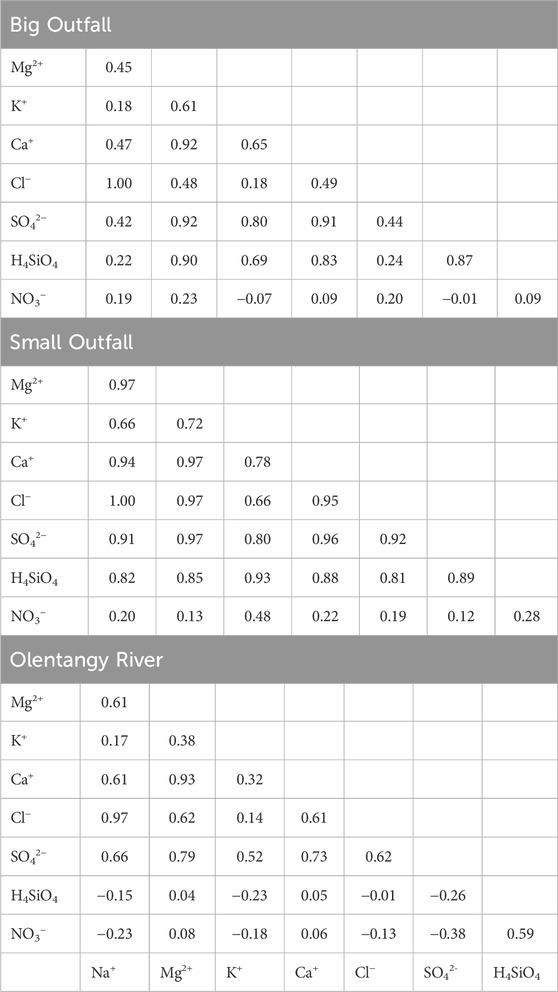
Table 1. Correlation coefficients (R) of major ions, nutrients, and dissolved silica concentrations of samples collected from the Big Outfall, Small Outfall, and Olentangy River from November 2021 to November 2022. Bolded values show correlations are significant at the 95% confidence level.
The mean cation abundance in the urban corridor of the Olentangy River was Ca2+ > Na+ > Mg2+ > K+, compared to the cation abundance of the upstream, agricultural corridor of the Olentangy River, which was Ca2+ > Mg2+ > Na+ > K+. The mean cation abundance of both outfalls was Na+ > Ca2+ > Mg2+ > K+ (Supplementary Tables S1, S2). Anion mean abundance of the urban sector of the Olentangy River was Cl− > SO42- > NO3− and the abundance of the outfalls were Cl− >> SO42- > NO3− (Supplementary Table S1). While Cl− was the dominant anion for the river and outfalls, the average Cl− concentration of the outfall was ∼3,800 µM (Big Outfall) to ∼8,300 µM (Small Outfall), much greater than the Olentangy River (∼1,500 µM). Using the mean values from the Olentangy River and other urban water types, ternary plots were generated with Geochemist Workbench® to characterize each water type. The ternary plots (Figure 3) show this transition of the Olentangy River from its agricultural to urban corridor along with reference waters, such as the Scioto River downstream of the Olentangy–Scioto confluence (Wichterich et al., 2024), precipitation (Carey et al., 2016; Lyons et al., 2024), tap water from buildings on Ohio State’s campus (Zic, 2022), a first-order urban stream draining into the Olentangy north of Ohio State campus (Stucker, 2013; Stucker and Lyons, 2017), and urban highway runoff from a highway that runs parallel to Ohio State’s campus (Gardner and Carey, 2004). The mean values of each dataset fall along a line from the agricultural corridor of the Olentangy to the highway runoff dataset, representing increasing urban influence. In the anion ternary diagram, tap water from Ohio State’s campus plots outside of this linear relationship due to a lower proportion of Cl− in the anion composition of the water (Figure 3).
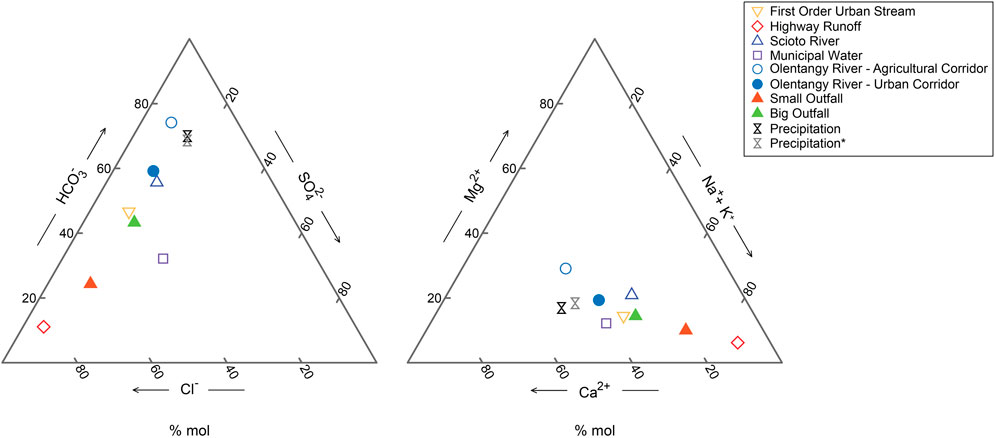
Figure 3. Ternary diagrams showing mean values of datasets from the Olentangy River, Big Outfall, and Small Outfall from this study, precipitation (Carey et al., 2016; Lyons et al., 2024), road (highway) runoff (Gardner and Carey, 2004), Scioto River downstream of the Olentangy confluence (Wichterich et al., 2024), tap water on Ohio State’s campus (Zic, 2022), a first order urban stream (Stucker, 2013; Stucker and Lyons, 2017), agricultural corridor of the Olentangy River at Waldo, Ohio. Mean ion concentrations for all samples are provided in Supplementary Table S2. Precipitation* revised dataset means are also provided in Supplementary Table S2, and description of correction is provided in section 4.2.
The precipitation samples were collected in an open bucket collector approximately 4 km north of Ohio State’s campus (Carey et al., 2016). Precipitation samples reflected atmospheric deposition and were also influenced by urban-derived Ca-rich dust deposited as dry deposition (Carey et al., 2016; Grider et al., 2023). Two precipitation sample means are shown on the diagrams from the same set of data. The unaltered sample set includes all Ca2+ measurements, with a mean of 34 μM, and the altered sample set has sample values greater than 125 µM removed because these high Ca2+ concentration samples were the result of Ca2+-rich dry deposition, rather than wet atmospheric deposition. The mean of the revised dataset was moderately reduced by the exclusion of six samples to 27 µM (Supplementary Table S2). Despite the removal of samples with excessively high Ca2+ concentrations, the cation proportion only moderately changed (Figure 3). The prevalence of urban Ca2+-rich dust deposition seemed to affect all precipitation samples, as has been demonstrated in previous work (Carey et al., 2016; Grider et al., 2023).
4.3 Stable water isotopes
The Olentangy River, Big, and Small Outfalls had similar δ18O values that ranged from −14.67‰ to −2.99‰. These water samples did now show the degree of isotopic variation displayed in precipitation δ18O values (Figure 4). However, when a large precipitation event had a depleted (more negative) isotopic signature or an enriched (less negative) signature, the river and outfall water responded in kind (Figure 4). The river and outfall samples fell close to the LMWL and global meteoric water line (GMWL) (Craig, 1961; Smith et al., 2021; Smith et al., 2024). The mean δ18O and δ2H values of the Olentangy River (−6.66‰, −42.3‰), Big Outfall (−7.20‰, −46.5‰) and Small Outfall (−7.35‰, −47.4‰) were very similar to the mean weighted annual average precipitation isotopic composition (−7.40‰, −48.2‰) (Smith et al., 2021) (Supplementary Figure S2). The observation that the river mean was more enriched than the precipitation perhaps suggests the loss of the lighter isotope through evaporation along the flow path of the river-reservoir system.
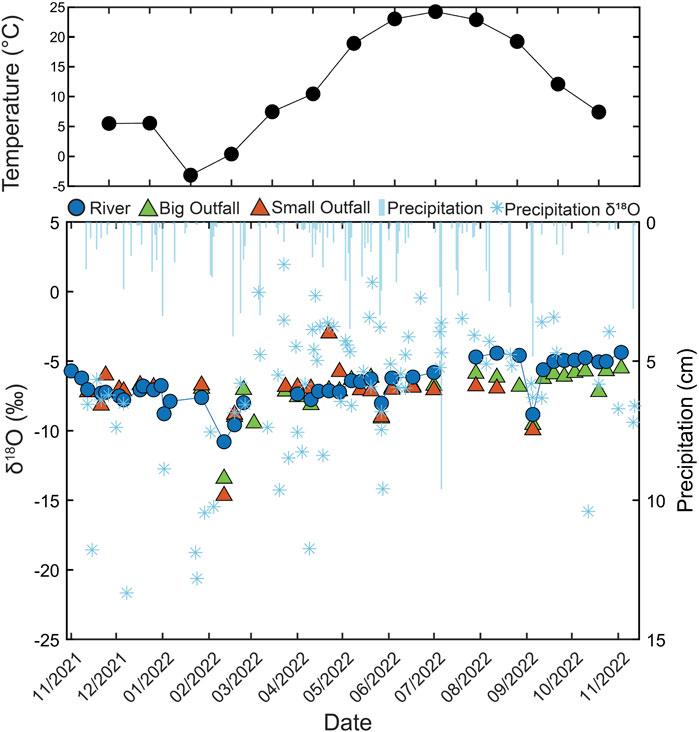
Figure 4. δ18O values measured in Olentangy River, Big Outfall and Small Outfall sample locations from November 2021 to November 2022. Precipitation amount is denoted by light blue lines and precipitation δ18O values are denoted by blue stars. Monthly mean temperature is plotted as black dots (CFAES, 2023). Figure modified with additional data from Lyons et al. (2024).
5 Discussion
5.1 Urban sources
5.1.1 Road salt application
Elevated Cl− and Na+ concentrations in the two outfalls were likely derived from road salts applied to Ohio State’s campus and the surrounding area (Figures 2A, B), as has been demonstrated locally (Gardner and Carey, 2004; Dailey et al., 2014; Stucker and Lyons, 2017). To support this assertion, we calculated the Cl−/Br− ratios of the Olentangy River and the outfalls (Supplementary Table S1). Elevated Cl−/Br− ratios are an indicator of road salt (halite) application. Halite has a high Cl−/Br− ratio that is driven by the process of formation, where the high solubility of bromide salts compared to chloride result in preferential precipitation of chloride in halite formation (Davis et al., 1998). Cl−/Br− ratios ranged from ∼550 to 6,900 in the Olentangy River and ∼1,100 to 7,000 in the outfalls. Ratios were greatest for the Olentangy River and outfalls in the winter months (Supplementary Table S1). The range Cl−/Br− ratios match results of Dailey et al. (2014) for Ohio rivers and ratios greater than 1,000 support conclusions that elevated Na+ and Cl− are derived from halite dissolution (Davis et al., 1998).
Clearly elevated Na+ and Cl− are not just a problem in Ohio rivers. There is strong evidence that increases on Na+ and Cl− are both a national and international problem (Kaushal et al., 2005; Kaushal et al., 2018b; Kaushal et al., 2020; Kaushal et al., 2022; Kaushal et al., 2024; Connor et al., 2014; Han and Xu, 2022; Hintz et al., 2022a; Hintz et al., 2022b; Dugan et al., 2023; Rossi et al., 2023). Long-term datasets from the Baltimore metropolitan region have shown increasing Cl− concentrations in urban and exurban watersheds due to road salt application and to a lesser extent, sewage effluent (Kaushal et al., 2005; Bird et al., 2018; Castiblanco et al., 2023). In addition, elevated Na+ and Cl− concentrations have been observed in regions where deicing salts have not been used as extensively as in the northeast and Midwest of the United States, indicating other anthropogenic sources into urban rivers (Schoonover, 2005; Rose, 2007; Steele and Aitkenhead-Peterson, 2011; Kaushal et al., 2024). The corresponding increase in other base cations and alkalinity with Cl− is referred to as freshwater salinization (FSS), which has multiple negative human and environmental health impacts (Kaushal et al., 2018a; Cruz et al., 2022; Hintz et al., 2022b). Sixty-nine percent of the samples in the Small Outfall had Cl− concentrations greater than the EPA Chronic threshold (Figure 2A). The Small Outfall drains a portion of the university’s campus that includes a major parking lots around Ohio Stadium (Figure 1). The period of elevated concentration extended beyond road salt application season (typically November–March). The Na:Cl ratios from both outfalls were enriched in Cl− (Supplementary Figure S1). This is indicative of a road salt source, where Cl− will move through the environment conservatively but Na+ can have cation exchange with K+, Ca2+, Mg2+ on soils and sediments (Kelly et al., 2012; Dailey et al., 2014).
Gardner and Carey (2004) demonstrated that evidence of road salts can be observed in storm drain effluent up to 7 months after last application. Dailey et al. (2014) built upon this finding to show that conventional fertilizers may also result in drained waters with elevated Cl− in spring and summer months. Interestingly, the temporal trends of Cl− and Na+ concentrations in the Big Outfall, which drains a much broader area, are much more predictable, showing elevated concentrations in the road-salt application season (winter) and declining through summer months into autumn. We suggest this is the result of a dilution pattern that is driven by the amalgamation of waters from a wider watershed that includes campus and the surrounding neighborhood. This dilution pattern is well documented in other urban systems (Kaushal et al., 2018a). Nevertheless, the elevated concentrations of Cl− and corresponding Na+ suggest that there is a risk of freshwater salinization effect in the urban corridor pf the Olentangy River (Figures 2A,B; Supplementary Figure S1). This is apparent when comparing the urban and agricultural corridors of the river (Figure 3).
5.1.2 Municipal water contribution
While the primary role of the subsurface drainage of the Small Outfall is to collect and export storm water, it can receive water from a facilities building on Ohio State’s campus (Ohio State Facilities and Engineers, personal communication). The mean concentration of K+ in the Small Outfall (123 µM) was within the range of mean K+ concentration measured in the three Columbus municipal water plans (120–128 µM) (City of Columbus, 2022). K+ is added as a water softener in Columbus municipal waters. Unlike the Small Outfall, the Big Outfall receives storm flow and combined sewage, but only in event conditions. The concentrations measured in the Big Outfall effluent match those of the Olentangy, and all sample types demonstrate evidence of dilution in precipitation events (Figure 2C). Due to the lack of correlation among Na+-Cl--K+ (Table 1) we do not propose the primary source of K+ additions are from sewage, although this has been established in as a source in previous studies (Rose, 2007; Tao et al., 2021). The Big Outfall and the Olentangy River demonstrate a pattern of increasing K+ concentration during the late spring through autumn. The mean K+ value of the urban Olentangy and the Big Outfall (∼110 µM) are only slightly elevated from the agricultural corridor of the Olentangy upstream (93 µM) (Supplementary Tables S1, S2). These data suggest that the source of K+ in the urban Olentangy and Big Outfall is primarily derived from a weathering source, as the river runs through Devonian-age shale and siltstone bedrock and some Devonian-age carbonates (Ohio Division of Geological Survey, 2006). The pattern of increasing K+ concentration in spring and summer is likely representative of seasonal hydrology, where seasonal low flow and base flow tend to dominate in from late spring to autumn (Gardner et al., 2023). The same pattern was apparent in the urban Olentangy SO42- concentrations, which increased throughout the summer and into autumn months, only to be disrupted by dilution events that corresponded to a high volume or consecutive precipitation events (Figure 2E).
Additional ions are added to Columbus municipal water in the treatment process. Sodium carbonate (NaCO3) is added to soften waters that are naturally high in Ca2+ and Mg2+ ions from weathering of limestone bedrock, and alum (Al2(SO4)3) is added as a coagulant in the treatment process. Fluoride is also added as part of the treatment process (City of Columbus, 2022). Mean fluoride concentrations were greater for the outfalls compared to the Olentangy River (Supplementary Table S1). These water treatment additions likely contribute to elevated concentrations measured in the Small Outfall (Figures 2D,G).
5.1.3 Pipe weathering
Further additions of major ions to the outfall waters were derived from the weathering of subsurface infrastructure in which the surface runoff water is transported. There are multiple unknows regarding the subsurface transportation of water running off Ohio State’s campus and the surrounding neighborhood. In addition to the lack of information on direct flow path, the residence time of water in the subsurface system was unknown. A portion of storm and surface runoff traveling through subsurface pipes is lost to groundwater through leaky pipes, which can be driven by high hydraulic conductivity ratios, shallow and low gradient pipe systems (Shepley et al., 2020). Furthermore, groundwater can contribute to water in subsurface pipes during baseflow discharge conditions (Kaushal and Belt, 2012). These potential loses and additions of water make it difficult to define the residence of water in subsurface pipe networks.
Based on the variations in major ion concentrations (Ca2+, Mg2+, SO42- and H4SiO4) we expect the shortest residence time (hours) occurred following high-volume precipitation events when the outfall effluent resembled the chemistry of the urban Olentangy. We expect that the longest residence time was on the order of days to weeks, when the outfall effluent chemical cocktail was distinctly different from the urban Olentangy (Supplementary Table S2). As mentioned above, SO42- was derived, at least partially, from bedrock weathering and municipal water treatment additions. Ca2+ was partially derived from a natural weathering process of limestone, as both the agricultural and urban corridors of the river had high average concentrations (∼1,600 μM, 1,400 µM) compared to the world average (world average, 365 µM Ca2+, Berner and Berner, 2012) (Supplementary Table S2). Additional sources of Ca2+ in the agricultural corridor may be liming, where excess Ca2+ and/or Mg2+ are added to increase soil pH. Liming processes can increase the concentration of Ca2+ and alkalinity surface waters (Oh and Raymond, 2006). Sources of increased Ca2+ and alkalinity in urban areas can be from weathering of impervious surfaces (Kaushal et al., 2013), and these results align with interpretation of long-term elevated Ca2+, Mg2+, and HCO3− in an urban environment from the LTER Baltimore Ecosystem Study (BES) (Bird et al., 2018).
Weathering of soils, impervious surfaces, and increased erosion in agricultural and urban watersheds also increase H4SiO4 concentrations and silica-rich chemical cocktails in surface waters (Kaushal et al., 2020). Higher concentrations of Ca2+, SO42-, and H4SiO4 measured in the outfalls were likely due to the weathering of concrete pipes (Figures 2D–F). The contribution of concrete pipe weathering has been shown to result in major modification of water ion chemistry (Davies et al., 2010; Wright et al., 2011; Müller et al., 2020) and the rate of infrastructure weathering needs to be considered from a water quality perspective when designing subsurface drainage. Weathering of impervious surface in urban environments results in increased ion loading and chemical cocktails of runoff waters (Kaushal et al., 2018a). Subsurface pipe networks, particularly concrete pipes, should also be viewed as water interacting with an impervious surface. Concrete is commonly comprised of three major compounds: calcium silicate hydrates (C-S-H), Portlandite (Ca(OH)2), and Ettringite (Ca6 [Al(OH)6]2(SO4)3∙26H2O), composing ∼60%, 25%, and 15% of cement paste, respectively (Saleh and Eskander, 2020; Davies et al., 2010; Davies et al., 2010). In addition to the aforementioned primary compounds, Mg2+, Na+, and K+ oxides are included in cement paste (Mindess et al., 2003; Mindess et al., 2003). Furthermore, gypsum (CaSO4∙H2O) is frequently added to the paste to slow aluminate hydration and increase durability of concrete (Neto et al., 2021). The dissolution of concrete pipes via urban water flow and the production of Ca+, K+, HCO3− and SO42- has been documented (Davies et al., 2010).
Subsurface pipes are not always constructed out of concrete; PVC piping is another common material used in subsurface systems (Müller et al., 2020). In fact, multiple types of material are commonly used within a pipe network, facilitating the exchange of groundwater with water transported in the pipes that contributes to the urban karst phenomenon (Kaushal and Belt, 2012). Both the Small Outfall and the Big Outfall waters exhibited significant, robust correlations among Ca2+, H4SiO4, SO42-, Mg2+, and to a lesser extent, K+ (Table 1). These correlations were not as robust for the Olentangy River, and the relationships between Ca2+ and H4SiO4 (Supplementary Figure S3), Mg2+ and H4SiO4, and SO42- and H4SiO4 were not significant (Table 1). K+ showed significant correlations with SO42- and Ca2+ (Table 1). These data indicate that weathering of subsurface concrete pipes acts as a substantial control on outfall chemistry. Except for high-volume precipitation events that caused dilution, concentrations of Ca2+ and H4SiO4 concentrations were always greater than the urban Olentangy River water (Figures 2D,F). The dissolution of concrete and other impervious surfaces contribute ions to urban rivers, but the fluctuations in ion and dissolved silica concentrations suggest that samples collected following high volume precipitation events were dominated by event water. Nevertheless, all samples were a mixture of runoff, CSO, and municipal (Small Outfall) water sources.
5.1.4 Upstream agriculture
The increased intensity of industrial-scale agriculture in the United States, and around the world, has transformed the delivery of nutrients to surface and groundwaters. NO3− concentrations in the urban Olentangy river were the result of agricultural influence upstream (Figure 3). NO3− was also delivered to the river by urban sources, as was apparent from the fluctuating NO3− concentrations in the outfalls (Figure 2H). Recent work has clearly documented that urban input can be a major source of NO3− to riverine budgets (Chung et al., 2023). The Small Outfall commonly had the lowest NO3− concentrations, except for four samples, with concentration values greater than or equal to the Big Outfall and the Olentangy river. NO3− sources to urban drainage include chemical fertilizers, natural atmospheric deposition, vehicle emissions, soil organic N, and sewage and/or animal waste, (Toor et al., 2017; Yang and Toor, 2017). Given the relatively smaller drainage area of the Small Outfall, the input of NO3− in these instances may be due to animal waste, lawn irrigation surface runoff, and atmospheric deposition related to vehicle emissions (Toor et al., 2017). The Big Outfall exhibited higher NO3 concentrations that were often similar to the concentration measured in the urban Olentangy River during the same sample collection. These data suggest that the aforementioned combination of urban sources, including sewage, contributed to NO3− loading in the urban Olentangy and the outfall. Urban sources were overwhelmed by the upstream agricultural input in late spring and early summer following fertilization of large agricultural fields in the upper portion of the watershed (Figure 2H) (Wichterich et al., 2024). The pattern of increased NO3− concentrations in central Ohio surface waters, particularly agricultural corridors, has been previously documented (Dailey et al., 2014; Leslie and Lyons, 2018; Wichterich et al., 2024) and aligns with large scale studies (Tian et al., 2020; Tian et al., 2020). The average NO3− concentration of the Olentangy river in the upstream agricultural corridor was 170 µM (n = 3) (Supplementary Table S2). However, two samples collected in June 2020 and May 2021 had concentrations that ranged from 225 to 235 µM compared to the sample collected in August 2021 with a concentration of 48 µM (Supplementary Table S2). These measurements from previous years align with measurements made from samples in 2022 and support the cyclic loading of NO3− in the agricultural corridor of the Olentangy.
Interestingly, the same pattern was not apparent in SRP concentrations (Figure 2I). The Small Outfall exhibited elevated concentrations of SRP on the 22nd and 29th of April. These might have been from a fertilizer source on Ohio State’s campus, as NO3− concentration was also elevated. In addition to the agricultural source attributed to NO3− and SRP concentrations in the urban Olentangy, upstream agricultural was likely an additional source of K+ from fertilizer application (Kaushal et al., 2018b). In most oxygenated waters nitrate and phosphate have different physicochemical forms, where nitrate remains dissolved in solution while phosphate is primarily thought to be adsorbed onto particles, such as Fe-oxyhydroxides. For example, numerous studies have demonstrated that in urban road runoff and stormwater flow much of the total P present is associated with particulate matter (Hobbie et al., 2017; Pamuru et al., 2022; Silva et al., 2023). This is also the case for Columbus stormwater runoff (Smith et al., 2020; Smith et al., 2023). As pointed out by Hobbie et al. (2017), different management practices are needed to minimize the input of N and P into natural river systems from urban sources.
Upstream agricultural management practices can exert a long-term impact on water quality downstream into urban environments. Work by Howden et al. (2010) utilized long-term data from the Thames to investigate the variations in NO3− loading along the river as it travels from agricultural to urban landscape to show that increased NO3− in the river was derived from agricultural and land changes, rather than urban development. The findings of our work exemplify the outcome determined by Howden and colleagues (2010), which is to say that a more robust understanding of solute input within the entire catchment area is needed not only to assess the source of particular elements and compounds, but also to determine the best management practices to maintain water quality.
5.2 Water sources
The subsurface network of pipes draining The Ohio State University’s campus and the surrounding neighborhood remain a black-box system in many ways. The leading questions surround the proportion of different water sources and water residence time in the system. Stable water isotopes can elucidate water sources within the pipe system. Overall, the δ18O values of outfall waters are similar to the urban Olentangy (Lyons et al., 2024) (Figure 4). However, most of the Big Outfall samples (74%) and Small Outfall samples (84%) have δ18O values that fall outside the instrument propagated uncertainty when compared to the Olentangy River samples. These data suggest that while the water is mixed, the isotopic composition of water in the outfalls is more responsive to recent precipitation events than the river and is a mixture of recent of seasonal precipitation. This is likely due to the stark difference in water volume between the outfalls and the Olentangy River. Previous work shows that the Scioto River, which the Olentangy feeds into, reflects a dampened seasonal pattern of precipitation δ18O and δ2H (Lyons et al., 2024; Smith et al., 2024). There has also been documentation of minimal isotopic change apart from enrichment (less negative) δ18O values below reservoirs that result from evaporation or elongated residence time in the Scioto system. The weekly samples collected in this study do not show strong evidence of seasonal enrichment corresponding to temperature increase associated with midlatitude precipitation and surface waters (Kendall and Coplen, 2001; Dutton et al., 2005), and observed in the Scioto (Smith et al., 2024).
Previous work has demonstrated that a greater proportion of watershed urban land cover shortens the mean water transit time within a catchment (Soulsby et al., 2014). In our study, transit times were difficult to identify and proportion of water sources remained unknown due to time lapse between sample events and the multiple sources of water. Beyond identifying seasonal patterns, the outfalls did not consistently respond to precipitation events. Low volume precipitation events did not result in the same isotopic composition from outfall waters (Figure 4). Mixing models were not employed in this study due to time lapse between sample events and the multiple unknown sources of water. However, after high volume precipitations events there was an apparent response from the rivers and outfalls. The river and outfall response was particularly evident for depleted (more negative δ18O values) precipitation events, for example, on May 27, where δ18O values in the outfalls showed depleted signatures in response to the precipitation and indicated rapid transport of event water. The assertion that isotopic depletion is a reflection of the antecedent precipitation event is supported by major ion dilution patterns that occurred simultaneously, as discussed in section 5.1. In these scenarios, the residence time was likely on the order of hours and the largest proportion of source water was the precipitation event water. However, for most of the study period, the consistent δ18O and δ2H values suggest that water was well mixed within the subsurface system. The residence time was heavily dependent on the amount of and the rate of precipitation.
5.3 River geochemical evolution from an agricultural to urban landscape
Subsurface outfalls that drain urban landscapes are major sources of elevated ion and nutrient concentrations to the Olentangy River, as demonstrated by the elevated concentrations of almost all major ions and nutrients from outfalls referenced in this work. Long-term research in Baltimore has shown that the concentration of major ions in urban streams continue to increase despite stable land use over time, demonstrating the legacy outfall and runoff waters (Bird et al., 2018). As reflected in previous sections, an important question to consider is how were these different waters chemically altered, and is there a systematic, even predictable biogeochemical “evolution” of these waters? The concept of the geochemical evolution of natural waters was developed around the concentration of solutes through water loss to evaporation and the precipitation of simple salts as their saturation index is reached (Mackenzie and Garrels, 1967; Eugster and Jones, 1979). In the case of urban water, the question centers around how non-urban water is chemically modified in the urban setting. The ternary plots that display averages of various water types and sources of water to the Olentangy River describe the evolution of an agricultural to urban river system (Figure 3). A prominent observation is the clear mixing line from the agricultural corridor of the Olentangy River and precipitation to the road runoff sample, representing chemistry of runoff from a highway parallel to Ohio State’s campus (Gardner and Carey, 2004). The urban Olentangy, Small Outfall, and Big Outfall plot along this line in both ternary diagrams. The Scioto River samples collected just downstream of the Olentangy-Scioto confluence had similar ion compositions to the Olentangy. Previous work by Wichterich et al. (2024) describes the urban influence on the Scioto. While the river systems differ for a myriad of anthropogenically-derived and natural reasons, they are both surface waters that transition from agricultural to urban corridors and as a result of this similarity, the geochemical patterns aligned.
The relationship among these waters can be further investigated by considering the chemical transformation of precipitation. In Columbus proper, the precipitation falls to the landscape surface and is transported into both the subaerial and subterranean pipes. These two water types then flow into surface water systems (e.g., first order streams and higher order rivers). Previous work showed that the precipitation in Columbus is enriched in Ca2+ due to Ca2+-rich dry and wet deposition (Carey et al., 2016; Grider et al., 2023). Once deposited onto the landscape, the runoff is further enriched in Ca2+ and other solutes. The TDS increases as the rain interacts with the motorway, urban streets, and the relative anion abundance is modified. The pattern of increasing Na+ and Cl− dominance and the overall increase in Ca2+, Mg2+, SO42−, and HCO3− concentrations in the Olentangy suggest freshwater salinization syndrome is a major concern, as it is in many other urban rivers (Kaushal et al., 2018b; Tao et al., 2021). As discussed in section 5.1.1, the major ion composition of the outfalls and road runoff indicate that the urban Olentangy FSS is caused by surface runoff transported through subsurface networks (Figure 3). FSS of surface waters that supply municipal water can cause an increase in Na+ and Cl− concentrations in drinking waters that may have health ramifications for the population. Increases in drinking water Cl− concentrations have been observed in Columbus drinking waters starting in January and declining into May. The increase was attributed to road salt application and water residence time in surface water reservoirs that supply Columbus drinking water (Leslie and Lyons, 2018). In addition to road salt application, other anthropogenic activities likely also contribute to river FSS. Clearly, geography plays a role, as one would expect more contributions from road salts in colder climates where snow and ice are major contributors to precipitation. However high concentrations of Na+ and Cl− are not observed solely in higher latitude urban settings (Rose, 2007; Steele and Aitkenhead-Peterson, 2011). Urban inputs are major contributions. However, previous research has demonstrated that FSS syndrome can also be instigated by salinization of groundwaters that contribute to surface water systems (Rossi et al., 2023). The combination of both salinization of urban Olentangy baseflow input and outflow effluent drive the shift in ion dominance (Figure 3).
The ion composition of the Big Outfall was similar to the Columbus urban first-order stream (Figure 3) (Stucker and Lyons, 2017). The first-order stream receives both SSO and CSO effluent from systems like the Big Outfall. Stucker and Lyons (2017) observed that minor differences in land cover of urban landscapes do not significantly alter the chemistry of receiving waterbodies. Although the first-order urban stream and the Big Outfall drain different areas of Columbus, they are similar in chemistry. This supports the notion of Stucker and Lyons (2017) that there may be a minimum impervious surface within an urban first order watershed that leads to the homogenization of urban storm runoff. Conversely, the Small Outfall, draining mostly impervious surfaces of parking lots exhibits an ion composition with greater Cl− and Na+ dominance, more like the highway runoff in storm events and further demonstrates the persistence of road salt in the environment after application (Gardner and Carey, 2004).
In few cases there are data available spanning all the potential water types from cities as we have for Columbus. Here have been studies through the years that have compared urban water chemistry to samples from the same river taken in agricultural portions of the watershed. We utilize a series of data from the upper, agricultural corridor of the Olentangy River and urban sources to establish the geochemical evolution of a river flowing through an urban area. Like other urban studies, the Olentangy River is substantially affected by urban water sources that alter the composition of the dissolved load as it travels through Columbus, Ohio.
6 Conclusion
In this study we sampled the Olentangy River and the discharge of two urban outfalls to measure the chemistry of urban waters feeding the Olentangy. We identified the shift in chemistry of the river as it travels from an agricultural to an urban environment. Findings demonstrate evidence of freshwater salinization affecting the Olentangy as urban runoff with elevated Na+, Cl+ and other major ions flow into the river along the urban corridor. We show that water interaction with impervious surfaces does not only happen on the ground surface, but also in the subsurface, through interaction with concrete pipe networks that have the potential to increase Ca2+, Mg2+, H4SiO4, SO42−, and K+ through weathering processes. Municipal water addition and combined storm overflow also contributed to the concentration and ion abundance of the dissolved load. The isotopic composition of waters and corresponding dilution patterns in these outfalls indicate that the water residence time varies, but during non-event sampling, the waters in the system were well mixed from multiple sources and reflected a dampened precipitation signature of recent events. Finally, we demonstrate that the agricultural headwaters still have notable control on the nutrient composition of the Olentangy River, where NO3− concentrations showed an elevated response to spring fertilizer application while outfalls did not.
The evolution of the river from an agricultural to urban corridor exhibits the fate of many rivers around the world, where the ion abundance is altered from a “natural” signature that is formulated primarily by weathering of soils, tills, and bedrock to an anthropogenic signature that is controlled by the chemistry of runoff that has interacted with the urban landscape and sewage effluent (Fitzpatrick et al., 2007). Studies documenting the progression of chemical change in urban and agricultural landscapes are essential to quantify the degree and rate of surface water geochemical change. Opportunities for future work in the Columbus metropolitan region and other urban areas include high resolution and event-based sampling of outfalls, river, and groundwaters to disentangle water sources and corresponding chemical contribution to urban rivers. Numerous water sources and restraints on information regarding the flow and residence time of waters in subsurface pipes hinder chemical source attribution. High resolution, daily, sampling of these systems will provide appropriate information to inform mixing models and quantify source water contributions to urban rivers. This type of data will support research investigating the source of water to subsurface systems and the transformation of water as it carries runoff through an urban karst landscape and contributes to both surface and groundwaters.
Data availability statement
All geochemical data presented in this manuscript are available in Supplementary Table 1. These data have also been made available on the Consortium of Universities for the Advancement of Hydrologic Science, Inc. HydroShare (https://www.hydroshare.org/) under the following resource: Smith et al. (2024).
Author contributions
DS: Writing–review and editing, Writing–original draft, Visualization, Investigation, Formal Analysis, Data curation. SW: Writing–review and editing, Investigation, Formal Analysis, Data curation. AR: Writing–original draft, Investigation, Formal Analysis, Data curation. AC: Writing–review and editing, Conceptualization. WL: Writing–review and editing, Writing–original draft, Supervision, Resources, Methodology, Investigation, Conceptualization.
Funding
The author(s) declare that financial support was received for the research, authorship, and/or publication of this article. A portion of AR’s contribution to this work was supported by the McKenzie-Breecher Undergraduate Scholarship Fund administered by the Byrd Polar and Climate Research Center. Support for Picarro Analyzer model L2103-i was provided by NSF GEO EAR/IF 1707989.
Acknowledgments
We thank the McKenzie-Breecher Undergraduate Scholarship Fund administered by the Byrd Polar and Climate Research Center for partial support of AR while she completed this research for her undergraduate thesis. Thanks to Ms. Najette Kanoun for her help regarding information on subsurface flow on The Ohio State University Campus. We are grateful to Dr. Christopher Gardner for his assistance in data collection. We Thank Dr. David Long for his continued and long-term support of earth scientists applying their knowledge and expertise to urban environmental problems.
Conflict of interest
The authors declare that the research was conducted in the absence of any commercial or financial relationships that could be construed as a potential conflict of interest.
The author(s) declared that they were an editorial board member of Frontiers, at the time of submission. This had no impact on the peer review process and the final decision.
Publisher’s note
All claims expressed in this article are solely those of the authors and do not necessarily represent those of their affiliated organizations, or those of the publisher, the editors and the reviewers. Any product that may be evaluated in this article, or claim that may be made by its manufacturer, is not guaranteed or endorsed by the publisher.
Supplementary material
The Supplementary Material for this article can be found online at: https://www.frontiersin.org/articles/10.3389/fgeoc.2024.1475109/full#supplementary-material
References
Berner, E. K., and Berner, R. A. (2012). Global environment. 2nd Edn. Princeton: Princeton University Press.
Bird, D. L., Groffman, P. M., Salice, C. J., and Moore, J. (2018). Steady-state land cover but non-steady-state major ion chemistry in urban streams. Environ. Sci. Technol. 52, 13015–13026. doi:10.1021/acs.est.8b03587
Bonneau, J., Fletcher, T. D., Costelloe, J. F., and Burns, M. J. (2017). Stormwater infiltration and the “urban karst” – a review. J. Hydrology 552, 141–150. doi:10.1016/j.jhydrol.2017.06.043
Carey, A. E., Welch, S. A., and Lyons, W. B. (2016). “Urban dust and central Ohio precipitation,” in Dust 2016 conference.
Castiblanco, E. S., Groffman, P. M., Duncan, J., Band, L. E., Doheny, E., Fisher, G. T., et al. (2023). Long-term trends in nitrate and chloride in streams in an exurban watershed. Urban Ecosyst. 26, 831–844. doi:10.1007/s11252-023-01340-0
CFAES (2023). CFAES weather system. Columbus: Ohio. Available at: https://weather.cfaes.osu.edu//(Accessed January 5, 2023).
Chambers, L. G., Chin, Y. P., Filippelli, G. M., Gardner, C. B., Herndon, E. M., Long, D. T., et al. (2016). Developing the scientific framework for urban geochemistry. Appl. Geochem. 67, 1–20. doi:10.1016/j.apgeochem.2016.01.005
Chung, A. H., Elliott, E. M., Bain, D. J., Thomas, B. F., River, M., Nim, C. J., et al. (2023). Riverine nitrogen source and yield in urban systems. Front. Ecol. Environ. 21, 461–468. doi:10.1002/fee.2679
City of Columbus (2022). Consumer confidence report. Available at: https://www.columbus.gov/Services/Public-Utilities/Information-for-Customers/Current-Consumer-Confidence-Report/CCR-Archives (Accessed July 19, 2024).
Connor, N. P., Sarraino, S., Frantz, D. E., Bushaw-Newton, K., and MacAvoy, S. E. (2014). Geochemical characteristics of an urban river: influences of an anthropogenic landscape. Appl. Geochem. 47, 209–216. doi:10.1016/j.apgeochem.2014.06.012
Craig, H. (1961). Isotopic variations in meteoric waters. Science 133, 1702–1703. doi:10.1126/science.133.3465.1702
Cruz, Y. D., Rossi, M. L., and Goldsmith, S. T. (2022). Impacts of road deicing application on sodium and chloride concentrations in Philadelphia region drinking water. Geohealth 6, e2021GH000538. doi:10.1029/2021GH000538
Dailey, K. R., Welch, K. A., and Lyons, W. B. (2014). Evaluating the influence of road salt on water quality of Ohio rivers over time. Appl. Geochem. 47, 25–35. doi:10.1016/j.apgeochem.2014.05.006
D’Aniello, A., Cimorelli, L., and Pianese, D. (2021). Leaking pipes and the urban karst: a pipe scale numerical investigation on water leaks flow paths in the subsurface. J. Hydrology 603, 126847. doi:10.1016/j.jhydrol.2021.126847
Davies, P. J., Wright, I. A., Jonasson, O. J., and Findlay, S. J. (2010). Impact of concrete and PVC pipes on urban water chemistry. Urban Water J. 7, 233–241. doi:10.1080/1573062X.2010.484502
Davis, S. N., Whittemore, D. O., and Fabryka-Martin, J. (1998). Uses of chloride/bromide ratios in studies of potable water. Ground Water 36, 338–350. doi:10.1111/j.1745-6584.1998.tb01099.x
Dugan, H. A., Rock, L. A., Kendall, A. D., and Mooney, R. J. (2023). Tributary chloride loading into Lake Michigan. Limnol. Oceanogr. Lett. 8, 83–92. doi:10.1002/lol2.10228
Dutton, A., Wilkinson, B. H., Welker, J. M., Bowen, G. J., and Lohmann, K. C. (2005). Spatial distribution and seasonal variation in 18 O/16 O of modern precipitation and river water across the conterminous USA, 4121–4146. doi:10.1002/hyp.5876
Environmental Protection Agency (2024a). National primary drinking water regulations. Available at: https://www.epa.gov/ground-water-and-drinking-water/national-primary-drinking-water-regulations#one (Accessed September 9, 2024).
Environmental Protection Agency (2024b). National recommended water quality criteria - aquatic life criteria table. Available at: https://www.epa.gov/wqc/national-recommended-water-quality-criteria-aquatic-life-criteria-table (Accessed July 14, 2024).
Eugster, H. P., and Jones, B. F. (1979). Behavior of major solutes during closed-basin brine evolution. Am. J. Sci. 279, 609–631. doi:10.2475/ajs.279.6.609
Fitzpatrick, M. L., Long, D. T., and Pijanowski, B. C. (2007). Exploring the effects of urban and agricultural land use on surface water chemistry, across a regional watershed, using multivariate statistics. Appl. Geochem. 22, 1825–1840. doi:10.1016/j.apgeochem.2007.03.047
Flint, E. M., Ascott, M. J., Gooddy, D. C., Stahl, M. O., and Surridge, B. W. J. (2023). Watermains leakage and outdoor water use are responsible for significant phosphorus fluxes to the environment across the United States. Glob. Biogeochem. Cycles 37. doi:10.1029/2022GB007614
Galvão, P., Hirata, R., Halihan, T., and Terada, R. (2017). Recharge sources and hydrochemical evolution of an urban karst aquifer, Sete Lagoas, MG, Brazil. Environ. Earth Sci. 76, 159. doi:10.1007/s12665-017-6482-3
Garcia-Fresca, B., and Sharp, J. M. (2005). “Hydrogeologic considerations of urban development: urban-induced recharge,” in Humans as geologic agents. Editors J. Ehlen, W. C. Haneberg, and R. A. Larson
Gardner, C. B., and Carey, A. E. (2004). Trace metal and major ion inputs into the Olentangy River from an urban storm sewer. Environ. Sci. Technol. 38, 5319–5326. doi:10.1021/es0497835
Gardner, C. B., Wichterich, C., Calero, A. E., Welch, S. A., Widom, E., Smith, D. F., et al. (2023). Carbonate weathering, phosphate fertilizer, and hydrologic controls on dissolved uranium in rivers in the US Corn Belt: disentangling seasonal geogenic- and fertilizer-derived sources. Sci. Total Environ. 861, 160455. doi:10.1016/j.scitotenv.2022.160455
Grider, A., Ponette-González, A., and Heindel, R. (2023). Calcium and ammonium now control the pH of wet and bulk deposition in Ohio, U.S. Atmos. Environ. 310, 119986. doi:10.1016/j.atmosenv.2023.119986
Han, R., and Xu, Z. (2022). Riverine hydrochemical characteristics of a typical karst urban watershed: major ion compositions, sources, assessment, and historical evolution. ACS Earth Space Chem. 6, 1495–1505. doi:10.1021/acsearthspacechem.2c00002
Hintz, W. D., Arnott, S. E., Symons, C. C., Greco, D. A., Mcclymont, A., Brentrup, J. A., et al. (2022a). Current water quality guidelines across North America and Europe do not protect lakes from salinization. Proc. Natl. Acad. Sci. U. S. A. 119, e2115033119. doi:10.1073/pnas.2115033119
Hintz, W. D., Fay, L., and Relyea, R. A. (2022b). Road salts, human safety, and the rising salinity of our fresh waters. Front. Ecol. Environ. 20, 22–30. doi:10.1002/fee.2433
Hobbie, S. E., Finlay, J. C., Janke, B. D., Nidzgorski, D. A., Millet, D. B., and Baker, L. A. (2017). Contrasting nitrogen and phosphorus budgets in urban watersheds and implications for managing urban water pollution. Proc. Natl. Acad. Sci. U. S. A. 114, 4177–4182. doi:10.1073/pnas.1618536114
Howden, N. J. K., Burt, T. P., Worrall, F., Whelan, M. J., and Bieroza, M. (2010). Nitrate concentrations and fluxes in the River Thames over 140 years (1868-2008): are increases irreversible? Hydrol. Process. 24, 2657–2662. doi:10.1002/hyp.7835
Kaushal, S. S., and Belt, K. T. (2012). The urban watershed continuum: evolving spatial and temporal dimensions. Urban Ecosyst. 15, 409–435. doi:10.1007/s11252-012-0226-7
Kaushal, S. S., Gold, A. J., Bernal, S., Johnson, T. A. N., Addy, K., Burgin, A., et al. (2018a). Watershed “chemical cocktails”: forming novel elemental combinations in Anthropocene fresh waters. Biogeochemistry 141, 281–305. doi:10.1007/s10533-018-0502-6
Kaushal, S. S., Groffman, P. M., Likens, G. E., Belt, K. T., Stack, W. P., Kelly, V. R., et al. (2005). Increased salinization of fresh water in the northeastern United States. Proc. Natl. Acad. Sci. 102, 13517–13520. doi:10.1073/pnas.0506414102
Kaushal, S. S., Likens, G. E., Pace, M. L., Haq, S., Wood, K. L., Galella, J. G., et al. (2019). Novel “chemical cocktails” in inland waters are a consequence of the freshwater salinization syndrome. Philosophical Trans. R. Soc. B Biol. Sci. 374, 20180017. doi:10.1098/rstb.2018.0017
Kaushal, S. S., Likens, G. E., Pace, M. L., Utz, R. M., Haq, S., Gorman, J., et al. (2018b). Freshwater salinization syndrome on a continental scale. Proc. Natl. Acad. Sci. U. S. A. 115, E574–E583. doi:10.1073/pnas.1711234115
Kaushal, S. S., Likens, G. E., Utz, R. M., Pace, M. L., Grese, M., and Yepsen, M. (2013). Increased river alkalinization in the eastern U.S. Environ. Sci. Technol. 47, 10302–10311. doi:10.1021/es401046s
Kaushal, S. S., Mayer, P. M., Shatkay, R. R., Maas, C. M., Cañedo-Argüelles, M., Hintz, W. D., et al. (2024). “Salinization of inland waters,” in Reference module in earth systems and environmental sciences (Elsevier).
Kaushal, S. S., Reimer, J. E., Mayer, P. M., Shatkay, R. R., Maas, C. M., Nguyen, W. D., et al. (2022). Freshwater salinization syndrome alters retention and release of chemical cocktails along flowpaths: from stormwater management to urban streams. Freshw. Sci. 41, 420–441. doi:10.1086/721469
Kaushal, S. S., Wood, K. L., Galella, J. G., Gion, A. M., Haq, S., Goodling, P. J., et al. (2020). Making “chemical cocktails” – evolution of urban geochemical processes across the periodic table of elements. Appl. Geochem. 119, 104632. doi:10.1016/j.apgeochem.2020.104632
Kelly, W. R., Panno, S. V., and Hackley, K. C. (2012). Impacts of road salt runoff on water quality of the Chicago, Illinois, region. Environ. and Eng. Geoscience 18, 65–81. doi:10.2113/gseegeosci.18.1.65
Kendall, C., and Coplen, T. B. (2001). Distribution of oxygen-18 and deuterium in river waters across the United States. Hydrol. Process. 15, 1363–1393. doi:10.1002/hyp.217
Lapointe, M., Rochman, C. M., and Tufenkji, N. (2022). Sustainable strategies to treat urban runoff needed. Nat. Sustain 5, 366–369. doi:10.1038/s41893-022-00853-4
Leslie, D. L., and Lyons, W. B. (2018). Variations in dissolved nitrate, chloride, and sulfate in precipitation, reservoir, and tap waters, Columbus, Ohio. Int. J. Environ. Res. Public Health 15, 1752. doi:10.3390/ijerph15081752
Lyons, W. B., Carey, A. E., Gardner, C. B., Welch, S. A., Smith, D. F., Szynkiewicz, A., et al. (2021). The geochemistry of Irish rivers. J. Hydrology Regional Stud. 37, 100881. doi:10.1016/j.ejrh.2021.100881
Lyons, W. B., Carey, A. E., Smith, D. F., Rankin, A., and Welch, S. A. (2024). ““Urban Kast” and the impacts of leaky pipes on groundwater and river chemistry,” in Groundwater and nature based solutions (Tullamore: International Association of Hydrologists - Irish Group).
Lyons, W. B., and Harmon, R. S. (2012). Why urban geochemistry. Elements 8, 417–422. doi:10.2113/gselements.8.6.417
Mackenzie, F. T., and Garrels, R. M. (1967). Equilibrium concepts in natural water systems: a symposium. Am. Chem. Soc. 67, 222. doi:10.1021/ba-1967-0067
Mindess, S., Young, J. F., and Darwin, D. (2003). Concrete. 2nd Edn. Upper Saddle River, NJ: Prentice Hall.
Müller, A., Österlund, H., Marsalek, J., and Viklander, M. (2020). The pollution conveyed by urban runoff: a review of sources. Sci. Total Environ. 709, 136125. doi:10.1016/j.scitotenv.2019.136125
National Land Cover Database (2019). 2019 CONUS land cover. Available at: https://www.usgs.gov/centers/eros/science/national-land-cover-database.
Neto, J. S. A, De la Torre, A. G., and Kirchheim, A. P. (2021). Effects of sulfates on the hydration of Portland cement – a review. Constr. Build. Mater. 279, 122428. doi:10.1016/j.conbuildmat.2021.122428
Oh, N. H., and Raymond, P. A. (2006). Contribution of agricultural liming to riverine bicarbonate export and CO2 sequestration in the Ohio River basin. Global biogeochemical cycles 20 (3). doi:10.1029/2005GB002565
Ohio Division of Geological Survey (2006). Bedrock geologic map of Ohio. Dep. Nat. Resour. Available at: https://ohiodnr.gov/wps/wcm/connect/gov/af200770-8656-455b-b41b-ee19ef48ef45/BG-1_8.5x11.pdf?MOD=AJPERES&CVID=ne.WWkh (Accessed May 19, 2023).
Pamuru, S. T., Forgione, E., Croft, K., Kjellerup, B. V., and Davis, A. P. (2022). Chemical characterization of urban stormwater: traditional and emerging contaminants. Sci. Total Environ. 813, 151887. doi:10.1016/j.scitotenv.2021.151887
Pickett, S. T. A., Cadenasso, M. L., Baker, M. E., Band, L. E., Boone, C. G., Buckley, G. L., et al. (2020). Theoretical perspectives of the Baltimore Ecosystem study: conceptual evolution in a social-ecological research project. Bioscience 70, 297–314. doi:10.1093/biosci/biz166
Rose, S. (2007). The effects of urbanization on the hydrochemistry of base flow within the Chattahoochee River Basin (Georgia, USA). J. Hydrology 341, 42–54. doi:10.1016/j.jhydrol.2007.04.019
Rossi, M. L., Kremer, P., Cravotta, C. A., Seng, K. E., and Goldsmith, S. T. (2023). Land development and road salt usage drive long-term changes in major-ion chemistry of streamwater in six exurban and suburban watersheds, southeastern Pennsylvania, 1999–2019. Front. Environ. Sci. 11. doi:10.3389/fenvs.2023.1153133
Saleh, H. M., and Eskander, S. B. (2020). “Innovative cement-based materials for environmental protection and restoration,” in New materials in civil engineering (Elsevier), 613–641. doi:10.1016/B978-0-12-818961-0.00018-1
Schoonover, J. E. (2005). Changes in chemical and physical properties of stream water across an urban-rural gradient in western Georgia.
Shepley, M. G., Schmidt, N., Senior, M. J., Worthington, S. R. H., and Scheckenberger, R. B. (2020). Assessing “urban karst” effects from groundwater–storm sewer system interaction in a till aquitard. Groundwater 58, 269–277. doi:10.1111/gwat.12908
Silva, T. F. G., Beltrán, D., de Oliveira Nascimento, N., Rodríguez, J. P., and Mancipe-Muñoz, N. (2023). Assessing major drivers of runoff water quality using principal component analysis: a case study from a Colombian and a Brazilian catchments. Urban Water J. 20, 1555–1567. doi:10.1080/1573062X.2022.2029913
Smith, D. F., Saelens, E., Leslie, D. L., and Carey, A. E. (2021). Local meteoric water lines describe extratropical precipitation. Hydrol. Process. 35, e14059. doi:10.1002/hyp.14059
Smith, D. F., Welch, S. A., Rankin, A., Carey, A. E., and Lyons, W. B. (2024). Geochemistry of subterranean outfalls and the Olentangy River in Columbus Ohio. HydroShare. Available at: http://www.hydroshare.org/resource/852cd091aa994d13b0297c8eea20f522.
Smith, D. F., Wichterich, C. M., Carey, A. E., Welch, S. A., and Lyons, W. B. (2024). The δ18O and δ2H fingerprint of the Scioto River: a detailed look into seasonal and spatial variations in the context of Ohio rivers. Appl. Geochem. 162, 105882. doi:10.1016/j.apgeochem.2023.105882
Smith, J. S., Winston, R. J., Tirpak, R. A., Wituszynski, D. M., Boening, K. M., and Martin, J. F. (2020). The seasonality of nutrients and sediment in residential stormwater runoff: implications for nutrient-sensitive waters. J. Environ. Manag. 276, 111248. doi:10.1016/j.jenvman.2020.111248
Smith, J. S., Winston, R. J., Wituszynski, D. M., Tirpak, R. A., Boening-Ulman, K. M., and Martin, J. F. (2023). Effects of watershed-scale green infrastructure retrofits on urban stormwater quality: a paired watershed study to quantify nutrient and sediment removal. Ecol. Eng. 186, 106835. doi:10.1016/j.ecoleng.2022.106835
Soulsby, C., Birkel, C., and Tetzlaff, D. (2014). Assessing urbanization impacts on catchment transit times. Geophys. Res. Lett. 41, 442–448. doi:10.1002/2013GL058716
Steele, M. K., and Aitkenhead-Peterson, J. A. (2011). Long-term sodium and chloride surface water exports from the Dallas/Fort Worth region. Sci. Total Environ. 409, 3021–3032. doi:10.1016/j.scitotenv.2011.04.015
Steele, M. K., McDowell, W. H., and Aitkenhead-Peterson, J. A. (2010). “Chemistry of urban, suburban. And rural surface waters,” in Urban ecosystem ecology. Editors J. A. Aitkenhead-Peterson, and A. Volder, 297–339.
Stucker, J. D. (2013). The effects of urban land use type on low order stream geochemistry. Columbus, OH: The Ohio State University.
Stucker, J. D., and Lyons, W. B. (2017). Dissolved trace metals in low-order, urban stream water, Columbus, Ohio. Appl. Geochem. 83, 86–92. doi:10.1016/j.apgeochem.2016.12.003
Tao, S., Zhang, X., Xu, J., Pan, G., and Gu, F. (2021). Anthropogenic impacts on isotopic and geochemical characteristics of urban streams: a case study in Wuhan, China. Environ. Sci. Pollut. Res. 28, 39186–39198. doi:10.1007/s11356-021-13484-7
The Ohio State University (2024). GIS maps. Available at: https://gismaps.osu.edu/osumaps/Default.html? (Accessed January 6, 2024).
Tian, H., Xu, R., Pan, S., Yao, Y., Bian, Z., Cai, W. J., et al. (2020). Long-term trajectory of nitrogen loading and delivery from Mississippi river basin to the gulf of Mexico. Glob. Biogeochem. Cycles 34. doi:10.1029/2019GB006475
Toor, G. S., Occhipinti, M. L., Yang, Y. Y., Majcherek, T., Haver, D., and Oki, L. (2017). Managing urban runoff in residential neighborhoods: nitrogen and phosphorus in lawn irrigation driven runoff. PLoS One 12, e0179151. doi:10.1371/journal.pone.0179151
United Nations (UN) (2018). Population division (2018) world urbanization prospects: the 2018 revision.
United States Census Bureau (2021). Quick facts: Columbus, Ohio. Available at: https://www.census.gov/quickfacts/columbuscityohio.
USGS (2011). State boundaries. Available at: https://www.sciencebase.gov/catalog/item/52c78623e4b060b9ebca5be5 (Accessed May 31, 2023).
USGS (2023). National hydrography dataset. Available at: https://www.usgs.gov/national-hydrography/national-hydrography-dataset (Accessed May 14, 2023).
Welch, K. A., Lyons, W. B., Whisner, C., Gardner, C. B., Gooseff, M. N., Mcknight, D. M., et al. (2010). Spatial variations in the geochemistry of glacial meltwater streams in the Taylor Valley, Antarctica. Antarct. Sci. 22, 662–672. doi:10.1017/S0954102010000702
Wichterich, C. M., Welch, S. A., Gardner, C. B., Smith, D. F., and Lyons, W. B. (2024). Effects of agriculture and urbanization on the geochemistry of the Scioto River, Ohio. Appl. Geochem. 168, 106016. doi:10.1016/j.apgeochem.2024.106016
Wright, I. A., Davies, P. J., Findlay, S. J., and Jonasson, O. J. (2011). A new type of water pollution: concrete drainage infrastructure and geochemical contamination of urban waters. Mar. Freshw. Res. 62, 1355–1361. doi:10.1071/MF10296
Yang, Y. Y., and Toor, G. S. (2017). Sources and mechanisms of nitrate and orthophosphate transport in urban stormwater runoff from residential catchments. Water Res. 112, 176–184. doi:10.1016/j.watres.2017.01.039
Keywords: urban storm runoff, urban karst, weathering, river, anthropogenic effects
Citation: Smith DF, Welch SA, Rankin A, Carey AE and Lyons WB (2024) Geochemistry of urban waters and their evolution within the urban landscape. Front. Geochem. 2:1475109. doi: 10.3389/fgeoc.2024.1475109
Received: 02 August 2024; Accepted: 27 September 2024;
Published: 16 October 2024.
Edited by:
Nadia Valentina Martínez-Villegas, Instituto Potosino de Investigación Científica y Tecnológica (IPICYT), MexicoReviewed by:
Craig Allan, University of North Carolina at Charlotte, United StatesRichard Wilkin, U.S. Environmental Protection Agency, United States
Copyright © 2024 Smith, Welch, Rankin, Carey and Lyons. This is an open-access article distributed under the terms of the Creative Commons Attribution License (CC BY). The use, distribution or reproduction in other forums is permitted, provided the original author(s) and the copyright owner(s) are credited and that the original publication in this journal is cited, in accordance with accepted academic practice. No use, distribution or reproduction is permitted which does not comply with these terms.
*Correspondence: W. Berry Lyons, bHlvbnMuMTQyQG9zdS5lZHU=