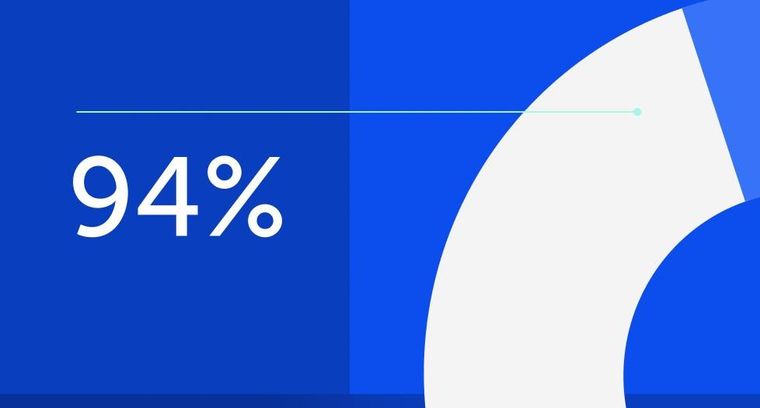
94% of researchers rate our articles as excellent or good
Learn more about the work of our research integrity team to safeguard the quality of each article we publish.
Find out more
ORIGINAL RESEARCH article
Front. Geochem., 31 August 2023
Sec. Organic Geochemistry
Volume 1 - 2023 | https://doi.org/10.3389/fgeoc.2023.1241784
This article is part of the Research TopicEmergence of Dynamic Membranes – Role of Terpenoids in the Evolution of Membrane Organization and RegulationView all 5 articles
The potential of C20 tricyclic and tetracyclic diterpane distributions in oils (and by extension, rock extracts) to aid the interpretation of sources of organic matter and depositional environments—spanning carbonate, marl, freshwater and saline lacustrine, normal marine and transitional—from Neoproterozoic to Neogene, is investigated using GC-MS and GC-MS-MS analysis of a range of oils of known origin. Contributions from gymnosperms are readily distinguished by abundant characteristic tricyclics and/or tetracyclics [e.g., 5β(H)-rimuane, 5β(H)-rosane, isopimarane and phyllocladanes]. Even at low levels, phyllocladane appears a reliable indicator of Carboniferous or younger source. A fairly uniform, limited range of diterpanes at relatively low abundance is observed in oils from other sources, with the 13β(H),14α(H)-cheilanthane often being the most abundant C20 diterpane associated with carbonates and marls. Other tricyclics include the previously proposed 8β-methyl-13α-ethylpodocarpane and a series of unidentified compounds, mostly sharing mass spectra with abundant fragment ions at m/z 123, 163 and 191, together with methyl (m/z 261), but not ethyl, loss from the molecular ion. This limited range of tricyclics suggests a common group of source organisms (probably bacterial) and or diagenetic transformation resulting in a few thermodynamically stable products. It may explain why pimarane is at most a trace component, despite pimaroids being widely occurring natural products. Where gymnosperms have made little contribution, C20 tetracyclic diterpanes are typically sparse and comprise beyerane, atisanes and possibly also 16α(H)-kaurane (which co-elutes with the first of the pair of atisane isomers), with beyerane usually the most abundant in terms of m/z 276→123 response. These compounds are not detected in oils from Neoproterozoic and Cambrian carbonates, but analysis of more samples is required to confirm this trend. Despite some caveats, diterpane distributions can provide useful information related to age and depositional environment as well as providing a tool for oil-oil correlation.
The terpenoid family is the largest group of natural products and there is considerable interest in it from the perspective of pharmacological applications. The most prolific producers are plants, fungi and marine invertebrates (Fischer et al., 2015; Reveglia et al., 2018; Karunanithi and Zerbe, 2019). In comparison, the bacterial terpenome is relatively small, but biochemical and genomic studies suggest that terpenoid biosynthesis among bacteria is widespread (Cane and Ikeda, 2012; Yamada et al., 2015; Dickschat, 2019; Rudolf et al., 2021). Our knowledge of bacterial terpene generation is growing rapidly, but establishing whether the relevant genes in specific bacteria are expressed is hampered by how successfully the microbes can be cultured. Active diterpene synthases have been identified in Actinomycetes, Streptomycetes, Nocardia and Rhizobium, where they are associated with organic matter degradation in soil, and they have also been found in the non-sulphur bacteria Chloroflexus and Rhodospirillum (Smanski et al., 2012).
Diterpenes have a variety of functions in plants, including growth regulation (Hanson, 1990), and signaling and protection against microbial or herbivore attack (Hammerbacher et al., 2019; Hunziker et al., 2021). Despite the ubiquity of diterpenes in plants, little is known about how their transport and focussing are controlled. In a recent molecular simulation study (Raza et al., 2023), it was found that model diterpenes permeated freely through plant membranes, so transport proteins may not be needed, as they are for other small molecules (Grotewold, 2004; Tomkins et al., 2021). In addition, permeability was greater for modelled membrane compositions of plants compared to those of animals, suggesting that plant membranes have adapted to facilitate low-energy transport processes for signaling molecules (Raza et al., 2023). Such passive transport across cell membranes is not without precedent in plants, having been recognized for lignin components (Vermass et al., 2019).
Abundant tricyclic and tetracyclic diterpanes in bitumen and oil are usually associated with woody gymnosperm contributions, and particularly conifer resinites (e.g., Noble et al., 1985a; Noble et al., 1985b; Noble et al., 1986; Simoneit et al., 1986; Weston et al., 1989; Philp, 1994; Killops et al., 1995; Keeling and Bohlmann, 2006). The most reliable of such gymnosperm indicators are thought to be the tetracyclanes beyerane (Bey), phyllocladane (Phyl) and kaurane (Kau), the structures of which are shown in Figure 1A. The precursors of these hydrocarbons are formed via the action of various cyclase enzymes on geranylgeranyl diphosphate (GGPP; e.g., Bohlmann et al., 1998), and the corresponding alkenes occur widely among the gymnosperms (Otto and Wilde, 2001), the first reliable fossil records of which date to the Pennsylvanian (Gymnosperm Database, 2023). However, abundant beyerane, phyllocladane and kaurane have been observed in Early Carboniferous coals, which points to an earlier origin for these compounds, possibly from pteridophytes (Disnar and Harouna, 1994) or progymnosperms. Whereas phyllocladane appears limited to Carboniferous and younger deposits, kaurane and beyerane have been reported in some rare, cuticle-rich, Devonian coals from China (Sheng et al., 1992; Song et al., 2017).
FIGURE 1. (A) C20 tricyclic and tetracyclic diterpanes identified in oils. IUPAC nomenclature adopted; note C-numbering schemes in red depend upon whether cheilanthane (C25) or isoagathane (C20) is adopted as parent compound. Rosane and rimuane have 5α(H) stereochemistry (e.g., Herz and Mirrington, 1965), but geochemically stable isomers are believed to be 5β(H), conferring a trans A-B junction (Zinniker, 2005). B = basic skeletal form produced by bacteria. (B) Some possible structures for unidentified tricyclic diterpanes (B = known from bacteria). (C) Origin of major fragment ions from isoagathane (N), 8β-methyl-13α-ethylpodocarpane (J) and possible structure for L. Ions in red more abundant than blue.
Fungi are known to produce tetracyclic diterpenoids of the kaurane, phyllocladane and aphidicolane skeletal families (Quin et al., 2014), and a modified biosynthetic route to kaurene has been recognized in the symbiotic nitrogen-fixing Bradyrhizobium japonicum (Morrone et al., 2009). Both kaurene and atiserene (i.e., the unsaturated form of atisane; Figure 1A) have been observed among terpenoids from the soil bacterium Streptomyces platensis (Smanski et al., 2011 and refs therein). Kaurene is a key intermediate in the pathway to gibberellins, compounds important in reproduction and growth in fungi and bacteria, as well as in plants (Salazar-Cerezo et al., 2018; Dickschat, 2019; Hedden, 2020). Kaurene can be converted by oxidative metabolism into beyerene and atiserenes, but not phyllocladene, which is synthesised by plants in a different pathway from GGPP, via copalyl diphosphate (MacMillan and Beale, 1999). Interconversion of kaurene, beyerene, atiserene and cyclo-seco-atiserenes can occur as a result of clay/acid-catalyzed rearrangement during diagenesis (Zinniker, 2005; Hong and Tantillo, 2010; Hanson, 2018).
Among gymnosperm derived tricyclic diterpanes, 5β(H)-rimuane, 5β(H)-rosane and isopimarane (Rim, Ros and iPim, respectively, Figure 1A), or combinations of them, can be abundant, but they have also been reported in oils from pre-Silurian marine sources (Peters et al., 2005), indicating that microbial origins are possible. 5β(H)-Rosane [or its enantiomer, 8β(H),9α(Me),10β(H)-rimuane] has been identified in sediments from lake Sokorte Dika (Kenya) and suggested to be of algal origin, based on its C isotopic composition (Huang et al., 1997). The abietane (Ab, Figure 1A) skeleton is common among, but not restricted to, coniferous diterpanes. Terrestrial and marine fungi are known to produce pimaroids (Sun et al., 2012; Quin et al., 2014; Reveglia et al., 2018), but differentiating fungal contributions from those of the terrestrial plants with which they are associated is likely to be problematical.
Isoagathenediol has been identified in Rhodospirillum rubrum (Chuck and Barrow, 1995); it is a potential precursor of isoagathane, which is the equivalent of the C20 member of the 13β(H),14α(H)-cheilanthanes (Figure 1A). Although the cheilanthanes are not strictly diterpanes, the C20 member fits the general definition of one. These compounds are ubiquitous in petroleum and often dominate hydrocarbons extracted from tasmanites (e.g., Australian Permian samples; Simoneit et al., 1990). In a detailed GC-MS analysis of the bitumen extracted from Latrobe tasmanites (Late Carboniferous-Early Permian, Tasmania), a range of tricyclic diterpanes was observed, with mass spectra being obtained on the 10 most abundant C20 compounds (Greenwood and George, 1999). Other than the C20 member (16,17,18,19,20-pentanor-13β(H),14α(H)-cheilanthane; 20C, Figure 1A), cheilanthanes were not identified, but one of the other diterpanes appears to be 8β-methyl-13α-ethylpodocarpane (based on its relative retention time and mass spectrum; Figure 1A for structure). The latter compound was one of a major proposed series of 8β-methyl-13α-n-alkylpodocarpanes, in addition to 13β(H),14α(H)-cheilanthanes, observed in a Precambrian fossil oil from North China (Wang and Simoneit, 1995). It was initially thought that the cheilanthanes in tasmanites were biosynthesized by the prasinophyte alga Tasmanites, the presence of which in the sedimentary record dates back to the Precambrian (Dutta et al., 2006). Indeed, laser micropyrolysis-GC-MS of Tasmanites microfossils in Latrobe shales has been found to yield cheilanthanes (Greenwood et al., 2000). However, pyrolytic analysis of isolated, Silurian-Devonian, prasinophyte fossils from Turkey has shown that cheilanthanes are not always produced by Tasmanites, but they can be found in another prasinophyte genus, Leiosphaeridia (Dutta et al., 2006). Cheilanthanes cannot be formed from squalene because its constituent farnesyl units are linked tail-to-tail. The most likely precursor of the common C19–C30 series is all-trans hexaprenol diphosphate (Renoux and Rohmer, 1986; Ribeiro et al., 2007); it is common in bacteria but related tricyclic triterpenes have yet to be identified in organisms (Tao et al., 2022). So the chemotaxonomy of cheilanthanes remains ambiguous, although extant sources of likely precursors are being sought (Liyanage, 2023).
A variety of tricyclic structures has been identified among bacterial diterpenoids, with ring sizes ranging from four to 9 C atoms (Rudolf et al., 2021 and references therein), but among the most stable thermodynamically are likely to be those based on the perhydrophenanthrene skeleton. Pimarane/isopimarane and isoagathane structures (Figure 1A) have been reported (e.g., Smanski et al., 2012 and references therein) and some others are shown in Figure 1B. Cyanobacterial diterpenoids discovered to date include abietenes in Microcoleous lacustris, noscomin in Nostoc commune (the ‘noscomane’ skeleton of which is shown in Figure 1B) and tolypodiol (potentially another source of isoagathane) in Tolypothrix nodosa (Pattanaik and Lindberg, 2015 and references therein). Tricyclic diterpenes, like tetracyclics, can undergo acid-catalyzed rearrangement, such as the interconversion of C-1 methyl configuration in the rimuadiene-isopimaradiene and rosadiene-pimaradiene isomeric pairs (Zinniker, 2005).
From the above synopsis, it can be concluded that tri- and tetracyclic diterpanes can potentially be found in a variety of depositional settings and derive from diverse sources, but usually in relatively minor abundance when not associated with dominantly gymnosperm contributions. The potential for clay/acid-catalyzed rearrangement and interconversion of cyclic diterpene skeletons during diagenesis must also be recognized, mirroring those of carbocations during biosynthesis (Peters, 2010). This article examines what information about source/environment can be obtained from the distribution of tricyclic and tetracyclic diterpanes when higher plants are not the main contributors. The study expands and elaborates upon on work presented at IMOG 2017 (Killops et al., 2017), which used GC-MS-MS to determine the distributions of C20 tricyclic and tetracyclic diterpanes in oils from source rocks of known age and depositional environment.
Samples, listed in Table 1, comprise 56 oils from sources of varying age, from Neoproterozoic to Neogene, and from a range of depositional environments, spanning carbonate/evaporitic, normal marine, lacustrine (freshwater and hypersaline) and swamp/mire/deltaic settings. Confidence in oil-source correlation is high, ranked from 1 for well-understood petroleum systems in Table 1, through 2 where multiple biomarker parameters and characteristics are consistent with a specific age, to 3 where there is general compatibility backed up by a few specific parameters. Oil window maturity ranges of the study sample suite are assessed by multiple parameters as early (∼0.5–0.7% vitrinite reflectance), peak (∼0.7–0.9% VR) and late (∼0.9–1.1%).
TABLE 1. Study samples and associated ages and depositional environments of their source rocks. Confidence of oil-source correlation (Correl.): 1 = well established petroleum system; 2 = based on combination of molecular characteristics and known potential source units; 3 = based on key biomarker parameters. Oil window maturity ranges (Mat.) estimated from combinations of molecular parameters: E = early (∼0.5–0.7% VR); peak (∼0.7–0.9% VR); late (∼0.9–1.1% VR). References (Ref.): 1) Perkins et al., 2023; 2) Słowakiewicz et al., 2020; 3) Abbassi et al., 2016; 4) Killops, 1996; 5) Killops et al., 1994; 6) Justwan et al., 2006; 7) Al Ghammari et al., 2021; 8) Hoshino et al., 2017; 9) Fowler et al., 1986; 10) Rullkötter et al., 1986; 11) Reed et al., 1986; 12) Creaney and Allan, 1990; 13) Killops et al., 2014; 14) Grassmann et al., 2005; 15) Underhill and Stonely, 1988; 16) England, 2009; 17) Scott et al., 2021; 18) Chung et al., 1992; 19) Riediger et al., 1997; 20) Véron, 2005; 21) Yang and Schulz, 2019; 22) Wavrek et al., 1998; 23) Eichentopf et al., 2017; 24) Curiale et al., 1985; 25) Podruski et al., 1988; 26) Clegg et al., 1997; 27) Biteau et al., 2006.
Solutions of each oil in small amounts of dichloromethane were treated by addition of freshly activated Cu blades to remove elemental S, and then asphaltenes were precipitated by addition of excess pentane (∼40x). Subsequent fractionation was achieved by medium pressure liquid chromatography (MPLC; after Radke et al., 1980), using a silica gel 100 (63–200 μm; Merck) pre-column (previously activated at 600°C for 2 h) and LiChroprep® Si 60 (40–63 μm; Merck) main column (previously activated at 120°C for 2 h under helium flow). Approximately 30 mg of maltenes, diluted to 1 mL in n-hexane, was injected, yielding an aliphatic fraction upon elution with n-hexane and an aromatic fraction by back-flushing the main column with n-hexane. Fractions were concentrated to ∼1 mL using an automated evaporation system (Turbovap®). N-alkanes were removed from aliphatic fractions (∼50 mg in ∼7 mL toluene) by addition of 5 Å molecular sieve (∼2 g, previously activated by heating at 450°C for 16 h) and refluxing (∼20 min), followed by cold rinsing (∼40 min), under nitrogen, using a Soxtec™ instrument.
Gas chromatography-mass spectrometry-mass spectrometry (GC-MS-MS) of branched/cyclic aliphatic hydrocarbon fractions employed a TSQ™9000 (Thermo Scientific™) quadrupole instrument, with Ar collision gas at an energy of 15 eV, monitoring molecular ion to m/z 123 and other major fragment ion transitions for C20 tricyclic and tetracyclic diterpanes. A 60 m CP-Sil 5 CB-MS column with an i.d. of 0.25 mm and a film thickness 0.25 μm was used with a temperature programme of 50°C (1 min isothermal) to 115°C at 20°C/min and then at 2°C/min to 325°C (20 min isothermal). The same instrument, column and temperature programme were used for full mass range (m/z 50–300) analyses to obtain mass spectra of previously unidentified diterpanes and for general screening of biomarkers in selected ion recording (SIR) mode. Well-known gymnosperm-derived diterpanes were identified by comparison of relative retention times and characteristic fragment ion responses with reported data (e.g., Noble et al., 1986; Weston et al., 1989; Zinniker, 2005).
There is potential for confusion of structures and the position of methyl groups, not least because C-numbering schemes differ depending upon the basic structure adopted for nomenclature (as shown for isoagathane, podocarpane and cheilanthane in Figure 1A). Diterpane stereochemistries in Figure 1A conform to IUPAC recommendations (IUPAC, 2022). The most stable (least strained) configuration at a ring junction is where the H atom or methyl group on the pair of bridging C atoms are on opposite sides of the bond with respect to the rings (i.e., trans). The IUPAC structure for rosane involves H and methyl on the same side of the A-B ring junction (cis), in a 5α(H),10α(H) configuration. The more stable 5β(H),10α(H) arrangement is present in geological samples [5β(H)-rosane], as shown in Figure 1A. Its enantiomer, in which the configuration at every chiral centre is inverted (C-5, C-8, C-9, C-10, and C-13), can be called 8β(H),9α(Me),10β(H)-rimuane. Huang et al. (1997) could not distinguish between this pair of enantiomers because they share identical chemical properties and cannot be resolved on achiral GC stationary phases. Enantiomeric pairs are not shown in Figure 1A but are likely to be present in geological samples.
The distributions of C20 tricyclic and tetracyclic diterpanes in oils from source rocks of varying age and depositional setting are shown in the partial m/z 276→123 and m/z 274→123 chromatograms in Figures 2–4.
FIGURE 2. C20 tricyclic (m/z 276→123; red) and tetracyclic (m/z 274→123; blue) diterpane distributions in oils from source rocks deposited in terrestrial/deltaic environments. Signal intensities are normalized to highest peak in each pair of transitions. See Table 2 for key to compound abbreviations.
FIGURE 3. C20 tricyclic (m/z 276→123; red) and tetracyclic (m/z 274→123; blue) diterpane distributions in oils from marine shales. Signal intensities are normalized to highest peak in each pair of transitions. See Table 2 for key to compound abbreviations.
FIGURE 4. C20 tricyclic (m/z 276→123; red) and tetracyclic (m/z 274→123; blue) diterpane distributions in oils from marine carbonates/marls. Signal intensities are normalized to highest peak in each pair of transitions. See Table 2 for key to compound abbreviations.
There is no obvious impact of maturity differences on diterpane distributions for the small numbers of oils that correspond to early or late oil window, upon comparison with the bulk of peak generation maturity. Most biomarkers are generated in the early to peak maturity range (∼0.6–0.9% vitrinite reflectance; Wilhelms and Larter, 2004) and accumulations represent the sum of charges over a range of source-rock maturity, so the observed maturity represents a mean weighted in favour of the highest generation rate of the compounds used in molecular maturity determination. Hence most study samples fall into the peak oil window range. An advantage of using oils is that they better represent the average composition of a source rock than individual rock extracts, particularly for coaly units (Killops et al., 1995, 2008).
The distributions of C20 tricyclic diterpanes in oils from source rocks of varying age and depositional setting are shown in partial m/z 276→123 chromatograms in Figures 2–4. Traditional indicators of gymnosperm contributions—5β(H)-rimuane, 5β(H)-rosane and isopimarane—are only abundant in oils originating from coals and some of the deltaic and lacustrine shales (m/z 276→123 chromatograms in Figure 2). The relative abundance of individual diterpanes can vary significantly, depending upon the major families of contributing gymnosperms (Killops et al., 1995; Otto and Wilde, 2001; Diefendorf et al., 2019), as demonstrated by the dominance of either the tetracyclic 16β(H)-phyllocladane (βPhyl) or the tricyclic isopimarane (iPim) in the coal-sourced oils in Figure 2. The variability in abundance of gymnosperm markers in oils associated with deltaic and lacustrine sources in Figure 2 is most likely controlled by transport of plant debris to the depositional site and its subsequent degree of preservation.
Abietane (Ab) appears to be present at low levels in the coal-sourced oils and is seemingly the main component in the corresponding small peaks in other samples, based on multiple GC-MS-MS transitions for eight oils of varying source age and environment (7, 8, 17, 18, 20, 35, 44, and 51; Table 1), which were selected to test the accuracy of peak identifications based on m/z 276→123 responses. The presence of abundant 5β(H)-rimuane, 5β(H)-rosane and isopimarane in the pair of Late Cretaceous coal sourced oils (7 and 8) was confirmed. Under the conditions employed, the m/z 276→247 response factors relative to m/z 276→123 were ∼100% for 5β(H)-rimuane and 5β(H)-rosane, but ∼60% for isopimarane. These response factors indicate that small amounts of all three diterpanes are present in NSO-1 (oil 35, Figure 5). The oil from a Paleogene deltaic shale (17, Figure 5) contains a significant amount of 5β(H)-rosane, but only traces of 5β(H)-rimuane and isopimarane. It has a m/z 276→123 peak corresponding to iPim that is larger than expected from the m/z 276→247 response (Figure 5), suggesting a co-eluant is present. Small peaks at the relative retention times of the three diterpanes can be observed in the m/z 276→123 mass chromatograms of the Neoproterozoic-Cambrian (18 and 44) and Ordovician oils (20 and 51) in Figure 5, but there is no corresponding m/z 276→247 signal, suggesting that other compounds are responsible. Consequently, there is no evidence of the presence of 5β(H)-rimuane, 5β(H)-rosane or isopimarane among the study oils from sources older than Devonian.
FIGURE 5. Identification of 5β(H)-rimuane, 5β(H)-rosane and isopimarane using response factors from the combination of m/z 276→247 and 276→123 transitions. Small peaks (●) in m/z 276→123 chromatograms of Paleozoic oils (20 and 51) do not appear to have any contribution from these traditional gymnosperm markers. A peak (○) in the m/z 276→123 chromatogram of the Paleogene deltaic sample (17) is only partially accounted for by isopimarane.
A small signal at the retention time of 5β(H)-rosane in oil sample 2 (Figure 2), even if genuine, could derive from the Mid Jurassic marine shale source component rather than the Devonian lacustrine. Similarly small signals are recorded for Late Devonian oil 24 and Late Devonian-Early Carboniferous oil 26, both related to marine shales (Figure 3), and also for Late Carboniferous oil 13 originating from deltaic shale (Figure 2) may be attributable to 5β(H)-rosane, but on its own it cannot be considered conclusive, given the potential origin from rearrangement of pimaroids (Otto and Wilde, 2001). However, dominance of 5β(H)-rosane among gymnosperm-derived diterpanes in oils is not unknown, as evinced by some oils and associated coals within Paleocene-Eocene strata in the Assam Basin, E. India (Rudra et al., 2017). Whether it is characteristic of Devonian coal-forming flora remains to be established.
Oils from marine shales, carbonates and marls of all ages contain much lower abundances of a fairly uniform range of mostly unidentified tricyclic diterpanes in m/z 276→123 chromatograms (G–M, X and Y) than those associated with coals, with limited occurrences of gymnosperm indicators as minor components (Figures 3, 4). These oils mostly exhibit a dominance of tricyclics over tetracyclics; the latter can be below the detection threshold. The C20 13β(H),14α(H)-cheilanthane (20C) is often relatively abundant, particularly so in the carbonate/marl oils. This is not surprising, because cheilanthanes in general are known to be abundant in high salinity environments, such as hypersaline lacustrine and marine carbonate/evaporite settings (de Grande et al., 1993). There is potential for a contribution of 20C from bacterial isoagathene-containing diterpenoids, additional to that from the source(s) of the wider cheilanthane series. This may account for the variation in the relative abundance of 20C among the tricyclic diterpanes in oils from marine shales (Figure 3) as well as enrichment in oils from evaporitic sources (Figure 4).
Peaks H, J, M and 20C are usually the most abundant in Figures 3, 4, suggesting that the diterpanes either derive from the same widespread group of organisms, among which bacteria are obvious candidates, or represent the most stable products of diagenetic alteration of what may be a wide range of diterpene precursors from an equally wide range of organisms. Mass spectra of the previously unidentified tricyclics and possible structural inferences are discussed in Section 3.2.3. Among tricyclics known to synthesized by bacteria are cassane, cleisthantane and noscomane (Figure 1B), but neither their mass spectra nor their GC relative retention times appear to have been reported.
Peak H approximately corresponds to the relative retention time of pimarane (Pim), as reported by Zinniker (2005) but its mass spectrum (Figure 6) lacks the characteristic m/z 247 fragment ion. Minimal m/z 276→247 responses indicate that pimarane is no more than a trace component in any of the eight samples subjected to detailed GC-MS-MS analysis (Figure 7A), including the coal-associated oils. So, despite the ubiquity of pimaroid-type skeletons among known microbial diterpenes, pimarane seems not to be a diagenetic product in most sedimentary environments. Zinniker (2005) observed only very low relative abundance of the compound from the m/z 276→247 transition for a Beaufort Sea, coal-sourced oil, but higher relative abundance in the Merced Formation and oil from the San Joaquin Valley (North California). Another rare report of relatively abundant pimarane (again from the m/z 276→247 transition) is in a Late Permian Mongolian coal (Peters et al., 2005). Pimarane gives the lowest response factor for the m/z 276→247 transition of 5β(H)-rimuane, pimarane, 5β(H)-rosane and isopimarane (Zinniker, 2005), but m/z 123 responses from traditional selected ion recording GC-MS analysis are fairly similar for these four diterpanes. Small peaks in the relative retention window of H/pimarane can be observed in such m/z 123 mass chromatograms of coaly samples in the literature, often slightly broader than expected for a single compound, as observed in this study. An example is provided by an Early Eocene, NE Indian coal (Figure 9 of Chattopadhyay and Dutta, 2014). This may be caused by closely eluting peaks H* and H we observe in oils from coaly sources (Section 3.2.3).
FIGURE 6. Mass spectra of compounds labelled G–N in m/z 276→123 chromatograms, as obtained from Neoproterozoic marine shale sourced oil (sample 19; Figure 8).
FIGURE 7. (A) Selected GC-MS-MS transition intensities for co-eluants in peak H in Gippsland (8), NSO-1 (35) and Huqf (18) oils. (B) Intensities of m/z 276 to selected product ions relative to m/z 276→123 intensity for peak H in oils of varying age and depositional environment, showing apparent effect of varying gymnosperm contribution.
Among possible diagenetic transformations of diterpane precursors which may explain the virtual absence of pimarane is acid-catalyzed rearrangement (Hall and Oehlschlager, 1972; Zinniker, 2005). Cyclization of pimarene via carbocation rearrangement to yield tetracyclics such as beyerene is a known biosynthetic pathway (e.g., scheme 10 of Peters, 2010), which might have an equivalent among potential diagenetic modifications.
A factor possibly impacting the range and abundance of C20 tricyclic diterpanes observed in oils and rock extracts is competition between aromatization and reduction of precursors. Anoxia tends to favour reduction to diterpanes, whereas oxidizing conditions generally leads to aromatization via oxidation and decarboxylation/dehydration (Diefendorf et al., 2014 and references therein). Determining the extent to which varying degrees of aromatization may affect diterpane distributions in oils is problematic, because specificity tends to be lost with methyl group elimination during aromatization. Solvent extract studies of the Zeit Formation (Eocene, Saxony) have provided evidence for the preferential diagenetic formation of diterpanes in conifer fossils, whereas aromatic species dominate the host sediment (Otto and Simoneit, 2001). Our results are consistent with this model in that diterpanes are most abundant where coaly sources, rich in preserved gymnosperm macerals, are involved.
There is no clear evidence that alteration of triterpenoids is a source of the ubiquitous unidentified tricyclics. The similar distributions in the relatively immature Latrobe tasmanite analysed by Greenwood and George (1999) to those in the diverse oils in our study suggests the absence of significant rupture of ring systems of alkyl side-chains during catagenesis. Although hopanoid origins would satisfy the requirement for widespread occurrence of precursors, these compounds preferentially undergo cleavage of the C ring at the 8(14) C-C bond, and ultimately tending to yield the dicyclic members of the drimane family (Schmitter et al., 1982; Alexander et al., 1984; Wang et al., 1990). Any A,B,C-ring fragment that might be formed would likely share the basic isoagathane/cheilanthane structure.
To help facilitate the attribution of biological sources to previously unidentified diterpanes, full mass range GC-MS analysis of oil sample 19 (Table 1) was performed; this oil having one of the highest relative abundances of these compounds among the study samples. It should lack any contribution from gymnosperm-derived diterpanes, because it is believed to originate from an Ediacaran anoxic marine shale, based on biomarker characteristics such as: C29/(C27–C29) steranes 0.90; Pr/Ph 0.99; 28,30-dinorhopane/17α-hopane 0.91; DBT/P 0.09; 24-iso-propyl/24-n-propyl cholestanes 0.96; A-ring methylated steranes dominated by 3-methyl isomers; and lack of detectable dinosteroids (aliphatic and triaromatic). A comparison of the total ion current (TIC) and m/z 276 and 123 chromatograms from this analysis with the corresponding GC-MS-MS m/z 276→123 chromatogram is shown in Figure 8. Mass spectra for compounds G–N are shown in Figure 6. These compounds exhibit effectively identical relative retention times and mass spectra to the C20 tricyclic diterpanes reported in Latrobe tasmanite by Greenwood and George (1999), whose labelling system is used here to aid comparison. Peak N (as shown in Figure 8) corresponds to the C20 13β(H),14α(H)-cheilanthane (20C) and J is the previously proposed 8β-methyl-13α-ethylpodocarpane (8M13EPod; Wang and Simoneit, 1995). In oils from younger sources, J can contain a pair of co-eluting compounds, the resulting composite mass spectrum seems to explain why it was not considered to represent 8M13EPod in the tasmanite analyzed by Greenwood and George (1999). The other compounds remain unidentified, although suggestions of possible structural elements to account for major fragment ions have been made by Greenwood and George (1999).
FIGURE 8. C20 tricyclic diterpanes in Neoproterozoic marine shale sourced oil (sample 19) as determined by (A) m/z 276→123 transition from GC-MS-MS and (B) TIC, m/z 276 and m/z 123 from full mass range GC-MS analysis (intensities relative to TIC in parentheses). See Table 2 for key to abbreviations and Figure 6 for mass spectra.
None of the mass spectra in Figure 6 exhibit fragment ions corresponding to the facile loss of ethyl (m/z 247) or iso-propyl (m/z 233) groups from the C ring, which characterize the pimarane and abietane structural types, respectively (Zinniker, 2005). Molecular ions at m/z 276 and loss of a single methyl group (m/z 261) are observed in all of the mass spectra, often accompanied by abundant fragment ions at m/z 123, 163 and 191. Such a pattern could suggest an A ring structure identical to that of pimarane/isopimarane (Zinniker, 2005), with a pair of methyl groups at C-4 and one at C-10 (Figure 1A). Among possible candidates are cassane, cleisthantane, noscomane and staminane (Figure 1B), although their mass spectra are unknown. The A-ring fragment from such structures is represented by m/z 123, whereas m/z 191 can originate from an A-B fragment, as in cheilanthanes (Heissler et al., 1984), or from a B-C fragment (involving neutral loss of the A ring from the molecular ion), as in the 8β-methyl-13α-n-alkylpodocarpanes (Wang and Simoneit, 1995). In the latter series, further loss of the C-13 alkyl group from the C ring of the m/z 191 fragment, as shown in Figure 1C, results in the sequence of major ions at m/z (135+n14), where n = 0, 1, 2, 3 …, confirming that the alkyl group on the C-ring forms part of this fragment (e.g., in the C20 member, n = 4, resulting in a m/z 191 fragment ion). This process has been suggested to account for the m/z 163 ion in the mass spectra of pimarane/isopimarane (Noble, 1986; Zinniker, 2005). A likely contributing factor to the significant m/z 247 response in pimarane/isopimarane is the stability of the tertiary ion (involving the methyl group at C-13) formed upon ethyl loss.
Interpretation of diterpane mass spectra is complicated by the profound influence of the position of substituents and stereochemistry, as demonstrated by a base peak at m/z 123 in 8β,13α-dimethylpodocarpane but at m/z 191 in 16-nor-isoagathane (Aquino Neto et al., 1983), these being C19 compounds differing only in the position and stereochemistry of a C-ring methyl group. Stereochemical differences alone can result in variations in major fragment ion responses in cheilanthane isomers, with the m/z 191/123 ratio being significantly greater in the 13β-methyl than the 13α isomers (Aquino Neto, 1986). Adjacent 8β-methyl and 14β-alkyl groups on the C-ring yields a m/z 191 base peak, regardless of whether or not there is a 13α-methyl group (e.g., isoagathane, N, Figure 6), whereas the presence of both 8β-methyl and 13α-alkyl groups, without an alkyl group at C-14, results in a m/z 123 base peak (e.g., 8β-methyl-13α-ethylpodocarpane, J, Figure 6). Because of such complexities, our suggestions of structures compatible with the mass spectra in Figure 6 are limited.
It is possible that the compounds responsible for peaks I and M are isomers of J. Peak K may be structurally related to M, although the dominance of the m/z 123 fragment might be explained by its formation from both A and C rings, such as appears possible in noscomane (Figure 1B). Peak L has major fragment ions at m/z 149 (base peak), 137 and 177 (Figure 6), which might result from a similar structure to I and M, but in which a methyl group from the B-C rings is relocated to the A ring (Figure 1C). The m/z 123 fragment from L is of relatively low abundance compared to the other major diterpanes, so the compound is quantitatively of more significance than suggested by its signal in the m/z 276→123 transition (Figure 8). Peak G may be affected by co-elution. Its mass spectrum is dominated by fragment ions at m/z 137 and 191 (Figure 6), which might be explained by a structure similar to L. Although it has a major m/z 191 fragment ion, it elutes too early to represent one of the four potential isomers of the C20 cheilanthane (Chicarelli et al., 1988), as previously noted by Greenwood and George (1999).
Two very closely eluting C20 tricyclanes are commonly present in peak H, resulting in some broadening of m/z 123 SIR and 276→123 MS-MS responses. The slightly earlier eluting of the pair of compounds (H*, Figure 7A), characterized by an intense m/z 276→163 signal, is more prominent in the Late Cretaceous, coal-sourced oils (7 and 8), but a definitive mass spectrum could not be obtained because of its low abundance and additional co-elution problem with a C19 sterane. The later-eluting compound (H of Greenwood and George, 1999) dominates in the Neoproterozoic-Cambrian oils (18 and 44). The other four oils are consistent with intermediate mixtures (Figure 7B). Consequently, care is required when attributing an identity to the peak in m/z 123 or m/z 276→123 chromatograms. From the data provided by extended GC-MS-MS analysis, the major peaks M and 20C appear to result from single compounds, without any obvious co-elutants.
Peak J elutes on the leading edge of 5α(H),14β(H)-androstane (14βAnd; Bender et al., 2015), when the latter is present, as in the Neoproterozoic-Cambrian samples. In such samples it corresponds to 8β-methyl-13α-ethylpodocarpane, based on its reported relative retention time and mass spectrum (Figures 6, 8A, respectively; Wang and Simoneit, 1995). However, in the coal-sourced oils a different, unidentified compound is present, in which m/z 163 dominates the transitions monitored and the m/z 123 and 261 responses are depressed, as in the mass spectrum reported for J by Greenwood and George (1999). Reliable mass spectra could not be obtained for the early eluting peaks X and Y.
The C20 tetracyclic diterpanes present a simpler distribution pattern than the tricyclics. In oils sourced by coals and some from Tertiary deltaic shales (16 and 17) in which gymnosperm contributions are inferred to be significant, tetracyclic diterpanes are at least as abundant as tricyclics and often dominated by 16β(H)-phyllocladane (βPhyl) in Figure 2. The second eluting of the pair of (16R)-14,15-cyclo-8,14-seco-atisane isomers (csAti2) identified by Zinniker (2005) appears to be present, at very low relative abundance, in the oils from coals and Eocene deltaic shale 16, based on relative retention time. Presence of the earlier eluting seco-atisane isomer (csAti1) could not be confirmed in any sample because of its reported co-elution with atisane isomer Ati1 (Zinniker, 2005). Sample 1, from an Eocene hypersaline lacustrine shale, appears to contain abundant 16β(H)-kaurane, rather than the thermodynamically more stable 16α(H) isomer, which is consistent with its early oil-window maturity [e.g., for 5α,14α,17α-24-ethylcholestane, 20S/(20S+20R) = 32%].
The relatively enriched tetracyclanes in general, together with the presence of gymnosperm-related 16β-phyllocladane, in NSO-1 oil (sample 35, Figure 3), may reflect a minor contribution from Mid Jurassic coaly sources, which is more prominent in the Sigyn Field oil from the Norwegian North Sea (sample 14, Figure 2). NSO-1 represents Oseberg B-18 production oil, from Mid Jurassic (Brent) reservoir at ∼3 km depth. Within the study set, the presence of C20 tetracyclic diterpanes in samples predating the appearance of gymnosperms is limited to traces of Kau-Ati-Bey combination in the Ordovician marine shale oil (sample 20, Figure 3). Whether the diterpanes in this oil are augmented by those from a younger source rock must be considered. Contributions to marine shale, carbonate and marl source rocks in general from kaurene precursors to microbial gibberellins is a possibility, if they are sufficiently abundant. Spores and pollens may represent another source of gibberellin precursors.
The upper Devonian Munindalen cannel coals from Svalbard are rich in spores, primarily from lycopsids, but have been found to contain no detectable Kau-Ati-Bey, unlike the neighbouring Pyramiden Carboniferous humic coals (Blumenberg et al., 2018). In contrast, the cuticular liptobiolite Devonian coals from China, primarily derived from lycopsids, do contain Kau-Ati-Bey, and what appear to be 17-nor and possibly dinor homologues (Song et al., 1997; Song et al., 2022), but the nor-phyllocladanes suggested to be present by Sheng et al. (1992) appear to be misidentified, so the earliest sedimentary occurrence of phyllocladane remains at Late Mississippian (Versteegh and Riboulleau, 2010). The biological origin of the Devonian diterpanes is unclear; it may be lycopsid cutinite, or the result of microbial activity or even other plant types, such as the progymnosperms (Rothwell, 1996; Retallack, 2021). Devonian oils in our data set exhibiting minor Kau-Ati-Bey are from carbonate (sample 45, Figure 4), marine shale (sample 24, Figure 3) and mixed lacustrine and marine shale (sample 2, Figure 2). It is possible that the diterpanes in the last sample originate from the Mid Jurassic marine shale contribution to the Beatrice Field oil, rather than the co-sourcing Devonian lacustrine unit, as noted for a possible trace of 5β(H)-rosane in that oil (Section 3.2.2).
In oils not dominated by gymnosperm contributions, tetracyclics are mostly minor components or undetectable, although their m/z 274→123 responses can sometimes rival those of equally sparse tricyclic m/z 276→123 signals, such as for beyerane in the Jurassic marine shale sourced samples 29 and 35 in Figure 3. Small amounts of what appear to be phyllocladane isomers are present in the Triassic marine carbonate (46), otherwise Mesozoic carbonates and marls contain only traces of beyerane (Bey), atisanes (Ati1 and Ati2) and a previously unidentified compound, Z (Figure 4), which is discussed below. Oils from older carbonate and marl sources are devoid of these tetracyclics, except for the Mid Devonian sample (45) from the Western Canada Basin (Figure 4). The oils derived from marine shales exhibit broadly similar distributions to those from carbonates and marls (Figure 3).
Relative responses for the m/z 274 to 123, 245 and 259 transitions suggest that beyerane, where detected in five of the eight oils subjected to extended GC-MS-MS analysis (7, 8, 17, 20, and 35), does not suffer from significant co-elution problems as far as those responses are concerned (Figure 9). However, a closely eluting, unidentified compound affects the m/z 274→189 response. Similar evaluation of 16α(H)-kaurane (αKau) and the pair of atisane isomers (Ati1 and Ati2) was inconclusive because of the potential co-elution of αKau with Ati1 and of Ati2 with the earlier eluting of a pair of (16R)-14,15-cyclo-8,14-seco-atisane isomers (csAti1; Zinniker, 2005). However, there is no obvious evidence for significant contributions from compounds with distinctly different mass spectra.
FIGURE 9. Identification of beyerane using response factors from the combination of m/z 274→123, 274→245 and 274→259 transitions. The m/z 274→189 chromatogram shows the adverse effects of a closely eluting, unidentified compound.
Clay-catalyzed inter-conversion of kaurene, beyerene and atiserene has been observed in the laboratory and suggested to account for sedimentary distributions of βKau, Ati2 and Bey (Zinniker, 2005). The common occurrence of these three tetracyclics in the study samples could be argued to provide circumstantial evidence of such diagenetic control, although it may well be superimposed on varying contributions of individual tetracyclics related to differences in microbial communities.
Compound Z is a C20 tetracyclane with an intense m/z 203 fragment ion, and it shares the mass spectrometric characteristics of 5α(H),14β(H)-androstane (Figure 10), so we suggest it is a homologue of that compound. The additional methyl group is likely to be found on the A-ring, because if it were on the D-ring the response of m/z 203 would be reduced and m/z 217 enhanced. This proposition is based on the observation of Tökés and Djerassi (1969) that 90% of the dominant m/z 203 signal originates from loss of the A-ring, because the absence of a side-chain at C-17 (which is present in regular steranes) confers greater stability on the D-ring, so the m/z 217 fragment ion is only minor. No higher homologues could be conclusively identified. The A-ring methyl group is suggested to be most likely at C-4, given that Z was detected in post-Paleozoic oils only, the methyl steranes of which are generally dominated by 4-methyl species.
FIGURE 10. Suggested presence of androstanes in NSO-1 oil (sample 35). (A) Partial m/z 203, 217 and molecular ion chromatograms for C19–C21 tetracyclanes (see Table 2 for abbreviations). (B) Mass spectra of peaks 19B [5α(H),14β(H)-androstane] and Z [probably 4α-methyl-5α(H),14β(H)-androstane].
Whereas the C20 tricyclic and tetracyclic diterpanes are the main focus of this study, it is important to recognize that other diterpanes can be relatively abundant in oils and sediments, sometimes as the main diterpanes. Examples are shown in Figure 11. Among C19 diterpanes, nor-isopimaranes and nor-phyllocladanes can be present, where gymnosperms contribute. Fichtelite, which can also be called 18-nor-abietane, was not positively identified; it is at most a trace compound in the sample set (Fic, Figure 11) and suffers from co-elution problems when monitored by its m/z 123 SIR response.
FIGURE 11. Examples of C19 tri- and tetracyclic and C20 dicyclic terpane occurrence in relation to C20 tri- and tetracyclic diterpanes. See Table 2 for peak identifications.
8β(H)-Labdane can be a major component in m/z 123 mass chromatograms in any environment during the Phanerozoic. Another, as yet unidentified, C20 bicyclane, W, elutes just before it, as noted by Zinniker (2005). Its mass spectrum appears to be similar to that of 8β(H)-labdane, although with a more prominent m/z 123 base peak (Figure 12). Several C19 tricyclic diterpanes were detected in the oil from Ediacaran marine shale (19), including 8β,13α-dimethylpodocarpane, the mass spectrum of which has previously been reported (Aquino Neto et al., 1983; Wang and Simoneit, 1995). Another pair of C19 tricyclics appear to correspond to peaks D and F of Greenwood and George (1999), although unambiguous mass spectra could not be obtained for D, because of co-eluants X and G, or for peak F, which co-elutes with dominant 19C in oil 19 (Figure 12). Of the other C19 tricyclic peaks, it is possible that B and C could be homologues of K and M, respectively, with loss of a methyl group from the C-ring, based on their mass spectra and relative retention characteristics in relation to the podocarpanes A and J.
FIGURE 12. C20 dicyclic and C19 tricyclic diterpanes in Ediacaran marine shale sourced oil (19) as determined by full mass range GC-MS analysis (TIC plus m/z 123, 191, 262 and 278), together with mass spectra. See Table 2 for key to abbreviations.
Recognising oils from sources rich in gymnosperm remains is straightforward; they contain abundant C20 tricyclic and tetracyclic diterpanes in which a number of the following dominate: beyerane, phyllocladanes, kauranes, 5β(H)-rosane, 5β(H)-rimuane and isopimarane. Where gymnosperm contributions are less significant, abundances of C20 tricyclic and tetracyclic diterpanes are much reduced and it becomes more difficult to identify them positively and to attribute them to gymnosperm origins. Phyllocladane appears to be the most reliable gymnosperm marker among tetracyclics. Its earliest confirmed occurrence in the study oils corresponds to the Triassic, but in the literature reliable occurrences date as far back as the Carboniferous. It is still useful as an age indicator in oils where limited gymnosperm contributions are present.
The co-occurrence of 5β(H)-rosane, 5β(H)-rimuane and isopimarane, even at relatively low abundances, can provide indications of gymnosperm contributions. 5β(H)-Rosane may be present on its own, at relatively low levels, in study oils believed to be generated from non-coaly Late Devonian sources. If the occurrences are genuine, they pre-date the appearance of gymnosperms, so may be attributable to their forerunners/progenitors, such as the progymnosperms, but direct microbial production or rearrangement of microbial precursors, such as pimaradienes, cannot be discounted.
Beyerane, atisanes and possibly 16α(H)-kaurane (which co-elutes with the earlier eluting of the pair of atisane isomers) are present in many oils to which gymnosperms are highly unlikely to have contributed. Beyerane is often the most abundant, although all of them are at low concentrations, similar to tricyclic diterpanes. They appear to be absent from the Neoproterozoic-Cambrian carbonate oils, but analysis of further examples is required to increase confidence in this conclusion. The ubiquity of the beyerane-atisane-kaurane grouping in post-Cambrian oils that do not contain significant gymnosperm contributions is consistent with their previously proposed clay catalyzed interconversion during diagenesis. Microbial sources are possible, including bacteria, among which production of kaurene and atiserene is known.
Where gymnosperms have not made significant contributions to sources, the associated study oils broadly contain the same limited range of sparse C20 tricyclic diterpanes. These oils represent a range of depositional environments and hence communities of organisms, throughout the Phanerozoic, so varied initial inputs of diterpenes to sedimentary organic matter would be expected. That such diversity is not observed suggests that only a limited, common, group of organisms is responsible, and/or that diagenesis favours the formation of a small number of relatively stable diterpanes. The latter could explain why pimarane is seemingly rare, despite pimaroids occurring widely in nature. A similar distribution of tricyclics has previously been observed for immature tasmanites, suggesting that the pattern is broadly established by the end of diagenesis.
There are commonly four major, non-gymnosperm, tricyclic peaks in m/z 123 mass chromatograms. Of these, one is the C20 13β(H),14α(H)-cheilanthane, which can also be called isoagathane, which can have additional sources other than that yielding the cheilanthane series. Another tricyclic has been proposed to be 8β-methyl-13α-ethylpodocarpane, which appears to be the dominant member of a series with varying n-alkyl chain length at C-13. Most of the tricyclics share mass spectral characteristics of loss of a single methyl group (yielding m/z 261) and abundant fragment ions at m/z 123, 163 and 191, which could suggest the presence of the A ring structure present in pimarane/isopimarane, but with a different substitution pattern in the B/C rings that does not involve ethyl and methyl groups on the same C atom that would give rise to a m/z 247 signal.
With regard to depositional environments, abundant gymnosperm contributions appear to be associated with limited transport of plant material, which can include lakes as well as mires/swamps and deltas. Oils from carbonates and marls exhibit a greater relative abundance of the C20 13β(H),14α(H)-cheilanthane, consistent with the common correlation of abundant cheilanthanes with high salinity.
Among other diterpanes that can be relatively abundant in m/z 123 mass chromatograms is the C20 dicyclic 8β(H)-labdane, together with a slightly earlier eluting unidentified compound with a similar mass spectrum. In addition, there appear to be C19 homologues of some of the unidentified tricyclic diterpanes, together with 8,13-dimethylpodocarpane and the C19 13β(H),14α(H)-cheilanthane. Where gymnosperms have contributed significantly, 19-nor-isopimarane and 17-nor-phyllocladane can be abundant.
Overall, there is some potential for extending the use of diterpane distributions beyond identification of gymnosperm contributions, to aid interpretation of source rock ages and depositional environment. Even minor variability in distributions of tri- and tetracyclic diterpanes provides scope for application in local oil-oil and oil-source correlations.
All relevant data discussed are presented as chromatograms and mass spectra in the article.
SK interpreted the data and drafted the manuscript, AB and JW designed the study, ET, KU, and JW contributed to the analyses, MG provided samples from Oman. All authors contributed to the article and approved the submitted version.
The authors thank Shell Global Solutions International B. V., PDO and APT AS for approval to publish this study.
Authors SK and KU were employed by Applied Petroleum Technology AS. Authors AB, ET, and JW were employed by Shell Global Solutions International B. V. Author MG was employed by Petroleum Development Oman.
All claims expressed in this article are solely those of the authors and do not necessarily represent those of their affiliated organizations, or those of the publisher, the editors and the reviewers. Any product that may be evaluated in this article, or claim that may be made by its manufacturer, is not guaranteed or endorsed by the publisher.
Abbassi, S., Edwards, D. S., George, S. C., Volk, H., Mahlstedt, N., di Primio, R., et al. (2016). Petroleum potential and kinetic models for hydrocarbon generation from the upper cretaceous to Paleogene Latrobe group coals and shales in the Gippsland basin, Australia. Org. Geochem. 91, 54–67. doi:10.1016/j.orggeochem.2015.11.001
Al-Ghammari, M., Siavalas, G., Bell, A., and Spaak, M. (2021). “Geochemical analysis of the Q source rocks of Oman: A 40-year-old mystery,” in Abstracts, 30th international meeting on organic Geochemistry (Montpelier. Available at: https://www.earthdoc.org/content/papers/10.3997/2214-4609.202134021.
Alexander, R., Kagi, R. I., Noble, R., and Volkman, J. K. (1984). Identification of some bicyclic alkanes in petroleum. Org. Geochem. 6, 63–72. doi:10.1016/0146-6380(84)90027-5
Aquino Neto, F. R. (1986). “Preferred fragmentation induced by degree of substitution and stereochemical effects on the mass spectra of tricyclic terpanes,” in Advances in mass spectrometry, Part B, proceedings of 10th international mass spectrometry conference. Editor J. F. D. Tedd (Chichester: John Wiley & Sons), 1349–1350.
Aquino Neto, F. R., Trendel, J. M., Restle, A., Connan, J., and Albrecht, P. A. (1983). “Occurrence and formation of tricyclic and tetracyclic terpanes in sediments and petroleum,” in Advances in organic Geochemistry 1981. Editor M. Bjorøyet al. (Chichester: John Wiley & Sons Ltd), 659–667.
Bender, M., Schmidtmann, M., Summons, R. E., Rullkötter, J., and Christoffers, J. (2015). A geomimetic approach to the formation and identification of fossil sterane biomarkers in crude oil: 18-nor-D-homo-Androstane and 5α,14β-androstane. Chem. Eur. J. 21, 12501–12508. doi:10.1002/chem.201502148
Biteau, J.-J., Le Marrec, A., Le Vot, M., and Masset, J.-M. (2006). The aquitaine basin. Pet. Geosci. 12 (3), 247–273. doi:10.1144/1354-079305-674
Blumenberg, M., Weniger, P., Kus, J., Scheeder, G., Piepjohn, K., Zindler, M., et al. (2018). Geochemistry of a middle Devonian cannel coal (Munindalen) in comparison with Carboniferous coals from Svalbard. Arktos 4 (4), 1–8. doi:10.1007/s41063-018-0038-y
Bohlmann, J., Meyer-Gauen, G., and Croteau, R. (1998). Plant terpenoid synthases: molecular biology and phylogenetic analysis. Proc. Natl. Acad. Sci. 95 (8), 4126–4133. doi:10.1073/pnas.95.8.4126
Cane, D. E., and Ikeda, H. (2012). Exploration and mining of the bacterial terpenome. Acc. Chem. Res. 45 (3), 463–472. doi:10.1021/ar200198d
Chattopadhyay, A., and Dutta, S. (2014). Higher plant biomarker signatures of early Eocene sediments of North eastern India. Mar. Petroleum Geol. 57, 51–67. doi:10.1016/j.marpetgeo.2014.04.004
Chicarelli, M. I., Neto, F., and Albrecht, P. (1988). Occurrence of four stereoisomeric tricyclic terpane series in immature Brazilian shales. Geochimica Cosmochimica Acta 52 (8), 1955–1959. doi:10.1016/0016-7037(88)90176-7
Chuck, J.-A., and Barrow, K. D. (1995). The isolation of isoagathalenediol: A tricyclic diterpene from the lipids of Rhodospirillum rubrum. Microbiology 141 (10), 2659–2663. doi:10.1099/13500872-141-10-2659
Chung, H. M., Wingert, W. S., and Claypool, G. E. (1992). “Geochemistry of oils in the northern viking graben: chapter 18,” in Giant oil and gas fields of the decade 1978-1988. Editor M. T. M. T. Halbouty (AAPG Mem), 54. doi:10.1306/M54555C18
Clegg, H., Horsfield, B., Stasiuk, L., Fowler, M., and Vliex, M. (1997). Geochemical characterisation of organic matter in keg river formation (elk point group, middle devonian), La crete basin, western Canada. Org. Geochem. 26 (11-12), 627–643. doi:10.1016/S0146-6380(97)00029-6
Creaney, S., and Allan, J. (1990). Hydrocarbon generation and migration in the Western Canada sedimentary basin. J. Brooks. Geol. Soc. Lond. Spec. Publ. 50, 189–202. doi:10.1144/GSL.SP.1990.050.01.9
Curiale, J. A., Cameron, D., and Davis, D. V. (1985). Biological marker distribution and significance in oils and rocks of the Monterey Formation, California. Geochimica Cosmochimica Acta 49 (1), 271–288. doi:10.1016/0016-7037(85)90210-8
de Grande, S. M. B., Aquino Neto, F. R., and Mello, M. R. (1993). Extended tricyclic terpanes in sediments and petroleums. Org. Geochem. 20 (7), 1039–1047. doi:10.1016/0146-6380(93)90112-O
Dickschat, J. S. (2019). Bakterielle diterpenbiosynthese. Angew. Chem. 131, 16110–16123. doi:10.1002/ange.201905312
Diefendorf, A. F., Freeman, K. H., and Wing, S. L. (2014). A comparison of terpenoid and leaf fossil vegetation proxies in Paleocene and Eocene Bighorn Basin sediments. Org. Geochem. 71, 30–42. doi:10.1016/j.orggeochem.2014.04.004
Diefendorf, A. F., Leslie, A. B., and Wing, S. L. (2019). A phylogenetic analysis of conifer diterpenoids and their carbon isotopes for chemotaxonomic applications. Org. Geochem. 127, 50–58. doi:10.1016/j.orggeochem.2018.11.007
Disnar, J. R., and Harouna, M. (1994). Biological origin of tetracyclic diterpanes, n-alkanes and other biomarkers found in Lower Carboniferous Gondwana coals (Niger). Org. Geochem. 21 (2), 143–152. doi:10.1016/0146-6380(94)90151-1
Dutta, S., Greenwood, P. F., Brocke, R., Schaefer, R. G., and Mann, U. (2006). New insights into the relationship between Tasmanites and tricyclic terpenoids. Org. Geochem. 37 (1), 117–127. doi:10.1016/j.orggeochem.2005.08.010
Eichentopf, H., Böcker, J., and Littke, R. (2017). Source rock characterisation of the rupelian fish shale (bodenheim Fm./Hochberg sbfm) – an organic geochemical profile from the clay pit unterfeld (rauenberg, Germany) in the upper rhine graben. Z. Dtsch. Ges. für Geowiss. 168 (2), 217–232. doi:10.1127/zdgg/2017/0112
England, M. L. (2009). Oil generation, migration and biodegradation in the wessex basin (dorset, UK). Doctoral dissertation, (Newcastle-upon-Tyne: Newcastle University).
Fischer, M. J. C., Rustenhloz, C., Leh-Louis, V., and Perrière, G. (2015). Molecular and functional evolution of the fungal diterpene synthase genes. BMC Microbiol. 15, 221. doi:10.1186/s12866-015-0564-8
Fowler, M. G., Abolins, P., and Douglas, A. G. (1986). Monocyclic alkanes in Ordovician organic matter. Org. Geochem. 10 (4-6), 815–823. doi:10.1016/S0146-6380(86)80018-3
Grassmann, S., Cramer, B., Delisle, G., Messner, J., and Winsemann, J. (2005). Geological history and petroleum system of the Mittelplate oil field, Northern Germany. Int. J. Earth Sci. 94, 979–989. doi:10.1007/s00531-005-0018-x
Greenwood, P. F., and George, S. C. (1999). Mass spectral characteristics of C19 and C20 tricyclic terpanes detected in Latrobe Tasmanite oil shale. Eur. J. Mass Spectrom. 5 (3), 221–230. doi:10.1255/ejms.278
Greenwood, P. F., Arouri, K. R., and George, S. C. (2000). Tricyclic terpenoid composition of Tasmanites kerogen as determined by pyrolysis GC-MS. Geochimica Cosmochimica Acta 64 (7), 1249–1263. doi:10.1016/S0016-7037(99)00326-9
Grotewold, E. (2004). The challenges of moving chemicals within and out of cells: insights into the transport of plant natural products. Planta 219:906–909. doi:10.1007/s00425-004-1336-0
Gymnosperm database (2023). Gymnosperm database. Available at: https://www.conifers.org/zz/gymnosperms.php.
Hall, S. F., and Oehlschlager, A. C. (1972). Cationic rearrangements and cyclizations of diterpenoid olefins. Tetrahedron 28 (12), 3155–3173. doi:10.1016/S0040-4020(01)93657-9
Hammerbacher, A., Coutinho, T. A., and Gershenzon, J. (2019). Roles of plant volatiles in defence against microbial pathogens and microbial exploitation of volatiles. Plant Cell. Environ. 42:2827–2843. doi:10.1111/pce.13602
Hanson, J. R. (2018). Skeletal rearrangements of rings C and D of the kaurene and beyerene tetracyclic diterpenoids. J. Chem. Res. 42 (4), 175–180. doi:10.3184/174751918X15233039624478
Hanson, J. (1990). The chemistry of the gibberellins. Nat. Prod. Rep. 7, 41–59. doi:10.1039/NP9900700041
Hedden, P. (2020). The current status of research on gibberellin biosynthesis. Plant Cell. Physiology 61 (11), 1832–1849. doi:10.1093/pcp/pcaa092
Heissler, D., Ocampo, R., Albrecht, P., Riehl, J.-J., and Ourisson, G. (1984). Identification of long-chain tricyclic terpene hydrocarbons (C21–C30) in geological samples. J. Chem. Soc. Chem. Commun. 8, 496–498. doi:10.1039/C39840000496
Herz, W., and Mirrington, R. N. (1965). Resin acids. V. Partial synthesis of (–)-rimuane. J. Org. Chem. 30 (9), 3195–3198. doi:10.1021/jo01020a079
Hong, Y. J., and Tantillo, D. J. (2010). Formation of beyerene, kaurene, trachylobane, and atiserene diterpenes by rearrangements that avoid secondary carbocations. J. Am. Chem. Soc. 132 (15), 5375–5386. doi:10.1021/ja9084786
Huang, Y., Peakman, T. M., and Murray, M. (1997). 8β,9α,10β-rimuane: A novel, optically active, tricyclic hydrocarbon of algal origin. Tetrahedron Lett. 38 (30), 5363–5366. doi:10.1016/S0040-4039(97)01173-8
Hunziker, P., Lambertz, S. K., Weber, K., Crocoll, C., Halkier, B. A., and Schulz, A. (2021). Herbivore feeding preference corroborates optimal defense theory for specialized metabolites within plants. Proc. Natl. Acad. Sci. U. S. A. 118, e2111977118, e2111977118. doi:10.1073/pnas.2111977118
IUPAC (2022). Recommendations on organic & biochemical nomenclature. Symbols & Terminology etc. Available at: https://iupac.qmul.ac.uk/.
Justwan, H., Dahl, B., and Isaksen, G. H. (2006). Geochemical characterisation and genetic origin of oils and condensates in the South Viking Graben, Norway. Mar. Petroleum Geol. 23 (2), 213–239. doi:10.1016/j.marpetgeo.2005.07.003
Karunanithi, P. S., and Zerbe, P. (2019). Terpene synthases as metabolic gatekeepers in the evolution of plant terpenoid chemical diversity. Front. Plant Sci. 10, 1166. doi:10.3389/fpls.2019.01166
Keeling, C. I., and Bohlmann, J. (2006). Diterpene resin acids in conifers. Phytochemistry 67 (22), 2415–2423. doi:10.1016/j.phytochem.2006.08.019
Killops, S. (1996). A geochemical perspective of oil generation in New Zealand basins, in 1996 NZ Pet. Conf. Proc. 1, 179–187. Available at: https://geodata.nzpam.govt.nz/report/pr5001.
Killops, S., Bishop, A., Tegelaar, E., Kleingeld, J., and Weijers, J. W. H. (2017) Diterpane distributions in oils in relation to source age and depositional environment. Poster presentation, 28th Internat. Mtg. Org. Geochem., Florence.
Killops, S. D., Mills, N., and Johansen, P. E. (2008). Pyrolytic assessment of oil generation and expulsion from a suite of vitrinite-rich New Zealand coals. Org. Geochem. 39 (8), 1113–1118. doi:10.1016/j.orggeochem.2008.01.017
Killops, S. D., Raine, J. I., Woolhouse, A. D., and Weston, R. J. (1995). Chemostratigraphic evidence of higher-plant evolution in the Taranaki Basin, New Zealand. Org. Geochem. 23 (5), 429–445. doi:10.1016/0146-6380(95)00019-B
Killops, S. D., Woolhouse, A. D., Weston, R. J., and Cook, R. A. (1994). A geochemical appraisal of oil generation in the Taranaki Basin, New Zealand. AAPG Bull. 78 (10), 1560–1585. doi:10.1306/A25FF21F-171B-11D7-8645000102C1865D
Killops, S., Stoddart, D., and Mills, N. (2014). Inferences for sources of oils from the Norwegian Barents Sea using statistical analysis of biomarkers. Org. Geochem. 76, 157–166. doi:10.1016/j.orggeochem.2014.07.011
Liyanage, T. (2023) “Finding a family for an orphan biomarker: searching for modern, biological sources of cheilanthane molecular fossils.” Doctoral dissertation (Canberra: Australian National University), submitted.
MacMillan, J., and Beale, M. H. (1999). “2.08 – diterpene biosynthesis,” in Comprehensive natural product chemistry. Editors D. Barton, K. Nakanishi, and O. Meth-Cohn (Amsterdam: Elsevier), Vol. 2, 217–243. doi:10.1016/B978-0-08-091283-7.00042-4
Morrone, D., Chambers, J., Lowry, L., Kim, G., Anterola, A., Bender, K., et al. (2009). Gibberellin biosynthesis in bacteria: separate ent-copalyl diphosphate and ent-kaurene synthases in Bradyrhizobium japonicum. FEBS Lett. 583 (2), 475–480. doi:10.1016/j.febslet.2008.12.052
Noble, R. A. (1986). A geochemical study of bicyclic alkanes and diterpenoid hydrocarbons in crude oils, sediments and coals. Doctoral dissertation. Australia: Perth: University of Western Australia.
Noble, R. A., Alexander, R., Kagi, R. I., and Nox, J. K. (1986). Identification of some diterpenoid hydrocarbons in petroleum. Org. Geochem. 10 (4–6), 825–829. doi:10.1016/S0146-6380(86)80019-5
Noble, R. A., Alexander, R., Kagi, R., and Knox, J. (1985a). Tetracyclic diterpenoid hydrocarbons in some Australian coals, sediments and crude oils. Geochimica Cosmochimica Acta 49 (10), 2141–2147. doi:10.1016/0016-7037(85)90072-9
Noble, R. A., Knox, J., Alexander, R., and Kagi, R. (1985b). Identification of teracyclic diterpene hydrocarbons in Australian crude oils and sediments. J. Chem. Soc. Chem. Commun., 32. –33. doi:10.1039/C39850000032
Otto, A., and Simoneit, B. R. T. (2001). Chemosystematics and diagenesis of terpenoids in fossil conifer species and sediment from the Eocene Zeitz formation, Saxony, Germany. Geochimica Cosmochimica Acta 65 (20), 3505–3527. doi:10.1016/S0016-7037(01)00693-7
Otto, A., and Wilde, V. (2001). Sesqui-di-and triterpenoids as chemosystematic markers in extant conifers – A review. Bot. Rev. 67 (2), 141–238. doi:10.1007/BF02858076
Pattanaik, B., and Lindberg, P. (2015). Terpenoids and their biosynthesis in cyanobacteria. Life 5, 269–293. doi:10.3390/life5010269
Perkins, J. R., Fraser, A. J., Muxworthy, A. R., Neumaier, M., and Schenk, O. (2023). Basin and petroleum systems modelling to characterise multi-source hydrocarbon generation: A case study on the inner moray firth, UK North Sea. Mar. Petroleum Geol. 151, 106180. doi:10.1016/j.marpetgeo.2023.106180
Peters, K. E., Walters, C. C., and Moldowan, J. M. (2005). The Biomarker Guide, Vol. 2. Cambridge: Cambridge University Press, 544–545.
Peters, R. J. (2010). Two rings in them all: the labdane-related diterpenoids. Nat. Prod. Rep. 27 (11), 1521–1530. doi:10.1039/c0np00019a
Philp, R. P. (1994). “Geochemical characteristics of oils derived predominantly from terrigenous source materials,” in Coal and coal-bearing strata as oil-prone source rocks? Editors A. C. Scott, and A. J. Fleet (London: Geological Society, Special Publication), 77, 71–92. doi:10.1016/j.cbpa.2012.03.002
Podruski, J. A., Barclay, J. E., Hamblin, A. P., Lee, P. J., Osadetz, K. G., Procter, R. M., et al. (1988). Conventional oil resources of Western Canada (light and medium). Part I: resource endowment. Geol. Surv. Can. Pap., 87–26.
Quin, M. B., Flynn, C. M., and Schmidt-Dannert, C. (2014). Traversing the fungal terpenome. Nat. Prod. Rep. 31 (10), 1449–1473. doi:10.1039/c4np00075g
Radke, M., Willsch, H., and Welte, D. H. (1980). Preparative hydrocarbon group type determination by automated medium pressure liquid chromatography. Anal. Chem. 52:406–411. doi:10.1021/ac50053a009
Raza, S., Miller, M., Hamberger, B., and Vermaas, J. V. (2023). Plant terpenoid permeability through biological membranes explored via molecular simulations. J. Phys. Chem. B 127 (5), 1144–1157. doi:10.1021/acs.jpcb.2c07209
Renoux, J.-M., and Rohmer, M. (1986). Enzymatic cyclization of all-trans pentaprenyl and hexaprenyl methyl esters by a cell-free system from the protozoan Tetrahymena pyriformis. The biosynthesis of scalarane and polycyclohexaprenyl derivatives. Eur. J. Biochem. 155 (1), 125–132. doi:10.1111/j.1432-1033.1986.tb09467.x
Retallack, G. J. (2021). Great moments in plant evolution. Proc. Natl. Acad. Sci. U. S. A. 118 (17), 21042561188–e2104256122. doi:10.1073/pnas.2104256118
Reveglia, P., Cimmino, A., Masi, M., Nocera, P., Berova, N., Ellestad, G., et al. (2018). Pimarane diterpenes: natural source, stereochemical configuration, and biological activity. Chirality 30 (10), 1115–1134. doi:10.1002/chir.23009
Ribeiro, N., Streiff, S., Heissler, D., Elhabiri, M., Albrecht-Gary, A. M., Atsumi, M., et al. (2007). Reinforcing effect of bi- and tri cyclopolyprenols on ‘primitive’ membranes made of polyprenyl phosphates. Tetrahedron 63 (16), 3395–3407. doi:10.1016/j.tet.2007.01.076
Riediger, C. L., Fowler, M. G., and Snowdon, L. R. (1997). Organic geochemistry of the Lower Cretaceous ostracode zone, a brackish/non-marine source for some lower Manville oils in southeastern Alberta, in Petroleum geology of the Cretaceous Mannville Group, western Canada, Can. Soc. Pet. Geol. Mem., 18, 93–101.
Rothwell, G. W. (1996). Pteridophytic evolution: an often underappreciated phytological success story. Rev. Palaeobot. Palynology 90 (3–4), 209–222. doi:10.1016/0034-6667(95)00084-4
Rudolf, J. D., Alsup, T. A., Xu, B., and Li, Z. (2021). Bacterial terpenome. Nat. Prod. Rep. 38 (38), 905–980. doi:10.1039/d0np00066c
Rudra, A., Dutta, S., and Raju, S. V. (2017). The Paleogene vegetation and petroleum system in the tropics: A biomarker approach. Mar. Petroleum Geol. 86, 38–51. doi:10.1016/j.marpetgeo.2017.05.008
Rullkötter, J., Meyers, P. A., Schaefer, R. G., and Dunham, K. W. (1986). Oil generation in the Michigan basin: A biological marker and carbon isotope approach. Org. Geochem. 10 (1-3), 359–375. doi:10.1016/0146-6380(86)90036-7
Salazar-Cerezo, S., Martínez-Montiel, N., García-Sánchez, J., Pérez-y-Terrón, R., and Martínez-Contreras, R. D. (2018). Gibberellin biosynthesis and metabolism: A convergent route for plants, fungi and bacteria. Microbiol. Res. 208, 85–98. doi:10.1016/j.micres.2018.01.010
Schmitter, J. M., Sucrow, W., and Arpino, P. J. (1982). Occurrence of novel tetracyclic geochemical markers: 8,14-seco-hopanes in a Nigerian crude oil. Geochimica Cosmochimica Acta 46 (11), 2345–2350. doi:10.1016/0016-7037(82)90206-X
Scott, M., Sylvester, P. J., and Wilton, D. H. C. (2021). A provenance study of upper jurassic hydrocarbon source rocks of the flemish pass basin and central ridge, offshore newfoundland, Canada. Minerals 11, 265. doi:10.3390/min11030265
Sheng, G., Simoneit, B. R. T., Leif, R. N., Chen, X., and Fu, J. (1992). Tetracyclic terpanes enriched in Devonian cuticle humic coals. Fuel 71 (5), 523–532. doi:10.1016/0016-2361(92)90149-I
Simoneit, B. R. T., Grimalt, J. O., Wang, T. G., Cox, R. E., Hatcher, P. G., and Nissenbaum, A. (1986). Cyclic terpenoids of contemporary resinous plant detritus and of fossil woods, ambers and coals. Org. Geochem. 10 (4–6), 877–889. doi:10.1016/S0146-6380(86)80025-0
Simoneit, B. R. T., Leif, R. N., Radler de Aquino Neto, F., Almeida Azevedo, D., Pinto, A. C., and Albrecht, P. (1990). On the presence of tricyclic terpane hydrocarbons in Permian Tasmanite algae. Naturwissenschaften 77 (8), 380–383. doi:10.1007/BF01135736
Słowakiewicz, M., Gluyas, J., Kowalski, A., Edwards, T., Słama, S., Mawson, M., et al. (2020). A new and working petroleum source rock on the UK Continental Shelf (Upper Permian, offshore Yorkshire). Mar. Petroleum Geol. 121, 104605. doi:10.1016/j.marpetgeo.2020.104605
Smanski, M. J., Peterson, R. M., Huang, S.-X., and Shen, B. (2012). Bacterial diterpene synthases: new opportunities for mechanistic enzymology and engineered biosynthesis. Curr. Opin. Chem. Biol. 16 (1–2), 132–141. doi:10.1016/j.cbpa.2012.03.002
Smanski, M. J., Yu, Z., Casper, J., Lin, S., Peterson, R. M., Chen, Y., et al. (2011). Dedicated ent-kaurene and ent-atiserene synthases for platensimycin and platencin biosynthesis. Proc. Natl. Acad. Sci. 108 (33), 13498–13503. doi:10.1073/pnas.1106919108
Song, D.-F., Wang, T.-G., Li, P., Zhang, M., Liu, A.-L., and Yan, J.-D. (2022). Petrology and organic geochemistry of the baishaping and damaidi devonian cutinitic liptobioliths, west of the kangdian uplift, China. Petroleum Sci. 19 (5), 1978–1992. doi:10.1016/j.petsci.2022.07.012
Song, D., Simoneit, B. R. T., and He, D. (2017). Abundant tetracyclic terpenoids in a Middle Devonian foliated cuticular liptobiolite coal from northwestern China. Org. Geochem. 107, 9–20. doi:10.1016/j.orggeochem.2017.02.010
Sun, L., Li, D., Tao, M., Chen, Y., Dan, F., and Zhang, W. (2012). Scopararanes C–G: new oxygenated pimarane diterpenes from the marine sediment-derived fungus Eutypella scoparia FS26. Mar. Drugs 10, 539–550. doi:10.3390/md10030539
Tao, H., Lauterbach, L., Bian, G., Chen, R., Hou, A., Mori, T., et al. (2022). Discovery of non-squalene triterpenes. Nature 606, 414–419. doi:10.1038/s41586-022-04773-3
Tökés, L., and Djerassi, C. (1969). Mass spectrometry in structural and stereochemical problems. CLXXVI. Course of the electron impact induced fragmentation of androstane. J. Am. Chem. Soc. 91 (18), 5017–5023. doi:10.1021/ja01046a014
Tomkins, M., Hughes, A., and Morris, R. J. (2021). An update on passive transport in and out of plant cells. Plant Physiol., 187:1973, 1984. doi:10.1093/plphys/kiab406
Underhill, J. R., and Stoneley, R. (1998). Introduction to the development, evolution and petroleum geology of the wessex basin, in development, Evolution and petroleum Geology of the wessex basin. J. R. Underhill. Geol. Soc. Lond. Spec. Publ. 133, 1–18. doi:10.1144/gsl.sp.1998.133.01.01
Vermaas, J. V., Dixon, R. A., Chen, F., Mansfield, S. D., Boerjan, W., Ralph, J., et al. (2019). Passive membrane transport of lignin-related compounds. Proc. Natl. Acad. Sci. 116, 23117–23123. doi:10.1073/pnas.1904643116
Véron, J. (2005). The alpine molasse basin: review of petroleum geology and remaining potential. Bull. Angew. Geol. 10 (1), 75–86. doi:10.5169/seals-225567
Versteegh, G. J. M., and Riboulleau, A. (2010). An organic geochemical perspective on terrestrialization. Geol. Soc. Lond. Spec. Publ. 339 (1), 11–36. doi:10.1144/SP339.3
Wang, T.-G., Simoneit, B. R. T., Philp, R. P., and Yu, C.-P. (1990). Extended 8β(H)-drimanes and 8,14-secohopane series in a Chinese boghead coal. Energy fuels. 4, 177–183. doi:10.1021/ef00020a009
Wang, T.-G., and Simoneit, B. R. T. (1995). Tricyclic terpanes in Precambrian bituminous sandstone from the eastern Yanshan region, North China. Chem. Geol. 120 (1–2), 155–170. doi:10.1016/0009-2541(94)00113-M
Wavrek, D. A., Curtiss, D. K., Guliyev, I. S., and Feyzullayev, A. A. (1998). “Maikop/diatom-productive series (!) petroleum system, south caspian basin, Azerbaijan,” in Abstracts, AAPG ann. Conv. (Salt Lake City. Abstract 90937.
Weston, R. J., Philp, R. P., Sheppard, C. M., and Woolhouse, A. D. (1989). Sesquiterpanes, diterpanes and other higher terpanes in oils from the Taranaki basin of New Zealand. Org. Geochem. 14 (4), 405–421. doi:10.1016/0146-6380(89)90006-5
Wilhelms, A., and Larter, S. (2004). “Shaken but not always stirred. Impact of petroleum charge mixing on reservoir geochemistry,” in Understanding petroleum reservoirs: Towards an integrated reservoir engineering and geochemical approach. Editors J. M. Cubitt, and W. A. England (Larter. Geol. Soc. Lond. Spec. Publ.), 237, 27–35.
Yamada, Y., Kuzuyama, T., Komatsu, M., Shin-ya, K., Omura, S., Cane, D. E., et al. (2015). Terpene synthases are widely distributed in bacteria. Proc. Natl. Acad. Sci. 112 (3), 857–862. doi:10.1073/pnas.1422108112
Yang, S., and Schulz, H.-M. (2019). Factors controlling the petroleum generation characteristics of Palaeogene source rocks in the Austrian Molasse Basin as revealed by principal component analysis biplots. Mar. Petroleum Geol. 99, 323–336. doi:10.1016/j.marpetgeo.2018.10.024
Keywords: tricyclic diterpanes, tetracyclic diterpanes, gymnosperms, diagenesis, terpenome
Citation: Killops SD, Bishop AN, Tegelaar EW, Urdal K, Ghammari MRK and Weijers JWH (2023) Sedimentary diterpane origins—inferences from oils of varying source depositional environment and age. Front. Geochem. 1:1241784. doi: 10.3389/fgeoc.2023.1241784
Received: 17 June 2023; Accepted: 14 August 2023;
Published: 31 August 2023.
Edited by:
Benjamin Nettersheim, University of Bremen, GermanyReviewed by:
Pierre Adam, UMR7177 Institut de Chimie de Strasbourg, FranceCopyright © 2023 Killops, Bishop, Tegelaar, Urdal, Ghammari and Weijers. This is an open-access article distributed under the terms of the Creative Commons Attribution License (CC BY). The use, distribution or reproduction in other forums is permitted, provided the original author(s) and the copyright owner(s) are credited and that the original publication in this journal is cited, in accordance with accepted academic practice. No use, distribution or reproduction is permitted which does not comply with these terms.
*Correspondence: S. D. Killops, c2tAYXB0LWludC5jb20=
†Present Address: A. N. Bishop, ConocoPhilips, Houston, TX, United States; E. W. Tegelaar, MultiPhase Analytics, The Hague, Netherlands
Disclaimer: All claims expressed in this article are solely those of the authors and do not necessarily represent those of their affiliated organizations, or those of the publisher, the editors and the reviewers. Any product that may be evaluated in this article or claim that may be made by its manufacturer is not guaranteed or endorsed by the publisher.
Research integrity at Frontiers
Learn more about the work of our research integrity team to safeguard the quality of each article we publish.